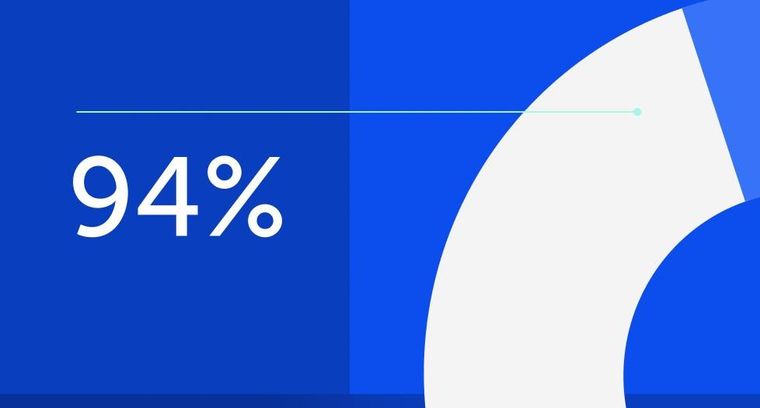
94% of researchers rate our articles as excellent or good
Learn more about the work of our research integrity team to safeguard the quality of each article we publish.
Find out more
ORIGINAL RESEARCH article
Front. For. Glob. Change, 14 February 2023
Sec. Tropical Forests
Volume 6 - 2023 | https://doi.org/10.3389/ffgc.2023.1024101
This article is part of the Research TopicBiochar from Forest Biomass as a Tool for Climate Change Mitigation and Degraded Soil ReclamationView all 5 articles
Drought and fire reduce productivity and increase tree mortality in tropical forests. Fires also produce pyrogenic carbon (PyC), which persists in situ for centuries to millennia, and represents a legacy of past fires, potentially improving soil fertility and water holding capacity and selecting for the survival and recruitment of certain tree life-history (or successional) strategies. We investigated whether PyC is correlated with physicochemical soil properties, wood density, aboveground carbon (AGC) dynamics and forest resistance to severe drought. To achieve our aim, we used an Amazon-wide, long-term plot network, in forests without known recent fires, integrating site-specific measures of forest dynamics, soil properties and a unique soil PyC concentration database. We found that forests with higher concentrations of soil PyC had both higher soil fertility and lower wood density. Soil PyC was not associated with AGC dynamics in non-drought years. However, during extreme drought events (10% driest years), forests with higher concentrations of soil PyC experienced lower reductions in AGC gains (woody growth and recruitment), with this drought-immunizing effect increasing with drought severity. Forests with a legacy of ancient fires are therefore more likely to continue to grow and recruit under increased drought severity. Forests with high soil PyC concentrations (third quartile) had 3.8% greater AGC gains under mean drought, but 33.7% greater under the most extreme drought than forests with low soil PyC concentrations (first quartile), offsetting losses of up to 0.68 Mg C ha–1yr–1 of AGC under extreme drought events. This suggests that ancient fires have legacy effects on current forest dynamics, by altering soil fertility and favoring tree species capable of continued growth and recruitment during droughts. Therefore, mature forest that experienced fires centuries or millennia ago may have greater resistance to current short-term droughts.
Despite the long-standing view that tropical rainforests are fire-free systems, there is increasing evidence that fires occurred in Amazonian forests before European colonization (Richards, 1973; Erickson, 2008). Fires in these wet environments depend on the combination of drought and ignition from human activity (Bush et al., 2008; França et al., 2020). Pre-Columbian fires were widespread and, in some areas, recurrent, with return intervals of hundreds of years (Sanford et al., 1985; Feldpausch et al., 2022). These ancient fires mostly spanned 7,000 to 250 years before present (BP), with an increase in fire frequency ∼1,500 to 500 years BP (Sanford et al., 1985; Santos et al., 2000; Goulart et al., 2017). Fires today are associated with deforestation and droughts (Silva et al., 2020; Silveira et al., 2020) and increased 74% in 2020 compared to 1998 in the Brazilian Amazon (INPE, 2021). Fragmented forests are at a greater risk of fire, especially in El Niño years, because fire spreads from forest edges to the interior of forests (Silva Junior et al., 2018, 2020). However, the long-term legacy of these fires on soil and vegetation remains unclear.
Forest fires produce pyrogenic carbon (PyC), which can act as an important proxy of ancient forest fire disturbances (Rehn et al., 2021). PyC is formed through the incomplete combustion of biomass (Bird et al., 2015). Some PyC can be lost as aerosols during burning (Bird et al., 2015) and through decomposition and erosion from steep slopes (Rumpel et al., 2006; Bird et al., 2015; Coppola et al., 2019). However, the remaining PyC is highly recalcitrant, because of its condensed aromatic structures and its physical and chemical associations with soil minerals (Lehmann et al., 2005; Brodowski et al., 2006), and can persist in the environment for millennia, including in soil (Bird et al., 2015). In the Amazon, there is a large stock of soil PyC formed in situ and by aerosol deposition (1.1 Pg in the top 30 cm alone) as a result of long-term PyC accumulation produced mostly by historical fires (Koele et al., 2017). Well-known examples of long-term PyC accumulation and amendment of soil fertility are the Amazon Dark Earth soils (ADE, Anthrosols, or Terra Preta de Índio), which are rich in PyC because of historical indigenous land management with fire and, as a result of this, have higher fertility than adjacent areas (Glaser, 2007; de Oliveira et al., 2020).
Fire can alter soil physicochemical properties. In addition to producing ash that can have a positive short-term effect on soil fertility, PyC produced by fires can also have positive long-term effects (Glaser et al., 2002). For example, the surface carboxylic groups on aromatic backbones can decrease aluminum toxicity and improve cation exchange capacity. Moreover, the porous structure of PyC can increase soil organic carbon and water holding capacity by reducing water percolation (Glaser et al., 2002). These changes potentially alleviate soil water deficits during drought events and improve soil fertility. Furthermore, forests rich in soil PyC (Amazon Dark Earth) have higher productivity (Aragão et al., 2009) and can allocate more carbon toward tree growth than forests in adjacent unburned areas (Doughty et al., 2014).
In recent decades, Amazonian forests have experienced an increase in drought frequency and severity (Lopes et al., 2016; Paredes-Trejo et al., 2021), reducing the net carbon sink (Brienen et al., 2015; Hubau et al., 2020) and causing net biomass losses (Feldpausch et al., 2016; Yang et al., 2018). However, the response to drought events can vary according to soil properties and plant functional traits such as wood density. Forests growing on more fertile soils and with lower wood density species experience faster tree turnover (Quesada et al., 2012), are often more vulnerable to severe drought (Feldpausch et al., 2016; Greenwood et al., 2017), and are consequently more vulnerable to fire (Berenguer et al., 2021). After fire events in Amazonian forests, severe structural and compositional changes frequently occur, with early successional tree species with low wood density establishing (Barlow and Peres, 2008; Berenguer et al., 2018). It remains unclear, however, if these changes leave a legacy on long-term (>100 years) forest carbon dynamics via changes induced in the soil by PyC or by selecting species and traits associated with fire and drought.
Here, we evaluate whether historical fires have left a legacy on soil and vegetation that affect how, over the past four decades, Amazonian forests have responded to drought. The aims of this research are: (i) to understand whether soil PyC concentration is associated with physicochemical soil properties and tree wood density, (ii) to determine whether there is an association between soil PyC and aboveground carbon (AGC) dynamics, (iii) to understand whether forests with higher soil PyC concentrations and more favorable soil physicochemical properties change AGC dynamics during severe droughts. We hypothesized that soil PyC as a proxy or legacy of ancient fires might influence Amazonian forests in three non-exclusive ways by: (1) improving soil fertility, tending to accelerate the turnover in Amazonian forests, (2) increasing water holding capacity, potentially conferring a higher resistance to droughts, and (3) favoring unique successional trajectories and species composition that reflects the compound legacy of ancient fire and drought disturbances (Figure 1).
We used data of forest dynamics from 95 plots encompassing 432 censuses from a published dataset plus data obtained via ForestPlots.net (Hubau et al., 2020; ForestPlots.net et al., 2021; Figure 2). Censuses took place between 1981 and 2017, including drought and non-drought periods. Plot size ranged from 0.25 to 9 ha, with a mean size of 1.14 ha and total plot area of 108 ha. Census intervals ranged from 4 months to 5.6 years, and plots have been monitored for an average of 15 years. For each census interval, we calculated Aboveground Biomass (AGB) gains caused by growth and recruitment, AGB losses from mortality and AGB net change as the difference between AGB gains and AGB losses. To calculate AGB, we used a community weighted mean wood density dataset available on ForestPlots.net that is derived from a global wood density database (Lopez-Gonzalez et al., 2011). This same database was used in the analyses which include community weighted mean wood density. AGB rates were transformed into Aboveground Carbon (AGC) stocks using the ratio 2:1 (IPCC, 2006). We excluded censuses with an interval length longer than twice the mean value of all censuses (>5.77 years) from our analysis. We exclude these censuses because long intervals are less likely to detect any effects of drought. All plots are in mature lowland forest (< 1,500 m above sea level), in non-ADE sites, without known recent fire disturbances or selective logging (i.e., from 1981) and represent a climatic and edaphic variation gradient across the Amazon Basin.
Figure 2. Spatial distribution of the plots analyzed. Note that locations are approximate and displayed to reduce overlapping points where multiple plots are sampled. The size of the gray dots is proportional to the mean concentration of PyC in the 0–30 cm interval at each site. Amazon regions are defined following Feldpausch et al. (2011).
Soil samples were collected from the same plots as the forest inventory data (Figure 2) at depths of 0–5, 5–10, 10–20, and 20–30 cm as part of past studies (Koele et al., 2017; Quesada et al., 2020) and this study with soil samples taken between 2017 and 2019. In plots ≥1 ha we sampled a minimum of five different locations across the plot, and in plots <1 ha we sampled three locations. Then, we combined the samples in each plot by depth to result in one sample per plot per depth. For each sample, we measured the total organic carbon (TOC), pyrogenic carbon (PyC), and non-PyC fraction of the organic carbon (OC). The hydrogen pyrolysis technique (HyPy) (Ascough et al., 2009) was used to quantify the PyC fraction of TOC, which represents stable polycyclic aromatic carbon with a ring number of >7 (Meredith et al., 2013). However, there is potentially more PyC that is not quantified by this technique since PyC produced by forests fires includes a variable fraction of PyC with an aromatic ring size of <7 that is not quantified by this technique. The non-PyC fraction of organic carbon (OC) is the difference between TOC and PyC and will include low temperature PyC not quantified by HyPy (if present).
We calculated the weighted mean for all depths to estimate the PyC and non-PyC OC concentrations in the soil surface (0–30 cm). The statistical analyses use continuous values; for Figure 5 we assigned each plot to one of two PyC classes based on the PyC median distribution: “Low”– values smaller than 0.048% and “High”– values equal or higher than 0.048%.
Figure 3. Relationship between soil PyC, physicochemical soil properties and wood density. (A) Soil fertility (log scale), (B) organic carbon (log scale) (%), (C) wood density (g cm– 3), (D) sand (%), (E) clay (%), (F) silt (%). Shading denotes 95% confidence intervals of the linear models. Soil PyC is log transformed.
Figure 4. Relationship between AGC dynamics and PyC (log-scale) for (A) AGC gains, (B) AGC losses and (C) AGC net change. Dashed lines represent non-significant relationships between variables and the shading denotes the 95% confidence interval from our GLMM.
Figure 5. The relationship between soil PyC and (A) AGC gains, (B) AGC losses and (C) AGC net change with drought severity (MCWD anomaly). Blue lines and dots represent plots classified as low percentage of PyC in soil (<0.048%) and red as high percentage of PyC in soil (≥0.048%). Solid lines represent significant relationships between MCWD anomalies and AGC dynamics, whilst different slopes between blue and red lines represent a significant interaction between soil PyC and MCWD anomaly in the best model (p < 0.05). Dashed lines represent non-significant relationships between variables. Shading denotes the 95% confidence interval. The MCWD anomaly is log transformed.
The Maximum Cumulative Water Deficit (MCWD) is a well-established metric to indicate water stress in forests (Aragão et al., 2007). MCWD is calculated from the cumulative difference in precipitation and evapotranspiration using an average evapotranspiration (E) for lowland moist tropical forests of approximately 100 mm per month (da Rocha et al., 2004; von Randow et al., 2004). Consequently, if the monthly precipitation (P) is lower than 100 mm, the forest enters a state of water deficit (WDef), which can accumulate over time (CWD). To calculate CWD, we used monthly precipitation data from the Climate Hazards Group InfraRed Precipitation with Stations (CHIRPS) from January 1981 until December 2017 (Funk et al., 2015). This dataset integrates satellite data with in situ rainfall gauge station measurements to produce gridded rainfall time series, and performs well across several regions of the world (Duan et al., 2016; Katsanos et al., 2016; Verdin et al., 2016; Perdigón-Morales et al., 2018). In the Brazilian Amazon, the CHIRPS dataset explained 73% of the observed rainfall in field gauge stations with a root mean square error below 15 mm per month (Anderson et al., 2018). The following rule was applied to calculate the CWD per plot (p) for each month (n), using the evapotranspiration fixed at 100 mm month–1:
The most negative CWD value among all months in each calendar year was taken as the MCWD. Subsequently, we calculated MCWD for each census interval, which refers to the most negative CWD during the census interval. The mean annual MCWD from 1981 to 2017 for each plot was used as the baseline to compute MCWD anomalies (Z-score) for each census interval, normalized by the standard deviation (σ). Censuses with anomalies ≤−1.65 were classified as severe drought events, based on a confidence level of 90% (Aragão et al., 2018).
Soil physicochemical data derived from plot samples were provided by ForestPlots.net (ForestPlots.net et al., 2021). We used data for surface (0–30 cm) soil including soil texture (silt, clay, sand fractions), total P, pH and exchangeable cations (K, Mg, Ca). The soil samples were all analyzed following the protocol described in Quesada et al. (2010).
All soil fertility variables were centered, scaled and condensed into one axis using a principal component analysis (PCA) using the R package FactoMineR (Husson et al., 2016). The first eigenvalue explained 73.3% of the variance and was used as a single soil fertility variable in the analysis, visualized using the R package factoextra (Kassambara and Mundt, 2017) (Supplementary Figure 1 and Supplementary Table 1).
To evaluate the relationship between soil PyC and soil fertility, OC, wood density and soil texture we used Pearson’s correlation tests. We applied a natural log transformation to soil PyC, soil fertility and OC to meet assumptions for data normality.
We applied a generalized linear mixed effects model (GLMM) to evaluate the relationship between soil PyC and AGC gains, losses and net change. To account for census replication per plot and for regional differences across the Amazon Basin, we used the plot code nested in plot clusters as a random intercept effect in the models, with a fixed slope. The plot clusters refer to plot codes that are located within the same local group and are represented by the same three first letters of the plot codes (Lopez-Gonzalez et al., 2011).
To evaluate the relationship of soil PyC on the change in AGC dynamics with drought severity, we filtered our database to only include censuses classified as severe drought (MCWD anomalies ≤−1.65σ) (Feldpausch et al., 2016). In this analysis, we also applied a GLMM using the same random effect cited above. Soil PyC, severe MCWD anomalies, OC, soil fertility, wood density and interaction between variables were used as predictors to evaluate differences in AGC dynamics according to drought severity. We applied a square-root transformation of AGC loss, and a log transformation of soil PyC and MCWD anomalies to ensure a normal distribution of fitted residuals. All variables were standardized to allow comparisons between model outputs. We applied a backwards stepwise selection procedure and selected the best models according to the lowest Akaike Information Criterion corrected for sample size (AICc) values using the MuMIn package (Barton, 2020). To evaluate spatial autocorrelation that could bias the models’ coefficients, we used the models’ residual to plot spline correlograms with 95% confidence interval based on 1,000 bootstrap resamples using the spline.correlog function in the package ncf (Bjørnstad and Falck, 2001). In order to understand whether the effects of soil PyC were driven by non-PyC organic carbon soil amelioration, we re-ran the models with OC instead of soil PyC. All GLMM models were performed using the lme4 package (Bates et al., 2018). All statistical analyses were conducted using R statistical software version 4.0.3 (R Core Team, 2020).
Soil PyC was significantly correlated with all measured variables. We found highly significant positive correlations between soil PyC with soil fertility (PCA axis-1) (r = 0.49, p < 0.001), OC (r = 0.30, p = 0.003), clay (r = 0.52, p < 0.001) and silt (r = 0.34, p = 0.002), and a negative correlation between soil PyC with wood density (r = −0.33, p < 0.001) and sand (r = −0.6, p < 0.001) (Figure 3).
Our results showed no significant relationship between soil PyC and AGC gains, AGC losses or AGC net change (Figure 4 and Supplementary Table 2). There was no significant autocorrelation in the model residuals and thus no spatial bias in the model results (Supplementary Figure 2).
To investigate the effect of PyC on AGC dynamics during drought events, we selected only censuses that had experienced a severe drought (i.e., MCWD anomalies ≥−1.65σ). Soil PyC, MCWD anomalies, OC, soil fertility, wood density, and interactions between variables were used as predictors to evaluate AGC dynamics with drought severity (Supplementary Table 3). The best models selected to explain AGC dynamics based on AICc values are reported in Table 1. Spline correlograms of the best models showed no significant spatial autocorrelation in the model residuals (Supplementary Figure 3). Wood density and the interaction of soil PyC with MCWD anomalies were significant predictors of AGC gains (i.e., woody productivity) during severe droughts. Our results showed lower rates of AGC gains in forests with greater wood density. We also found a significant interaction between soil PyC and MCWD anomalies on AGC gains (p = 0.041), with the slope between AGC gains and MCWD anomalies becoming less negative with increasing soil PyC (Table 1).
Table 1. Parameter estimates for the selected models explaining AGC (gains, losses, and net change) during severe droughts (σ ≤ −1.65).
We found the difference in AGC gains between forests with PyC concentrations lower and those with PyC concentrations greater than the median (0.048%) to increase with drought severity, as AGC gains significantly declined at a greater rate in low soil PyC forests compared with high soil PyC forests (Figure 5). We compared events around the mean MCWD anomaly (∼−2σ, p < 0.046) and the most severe MCWD anomaly (∼−4.3σ, p < 0.001) for forests with the first quartile of observed soil PyC concentration (0.038%) to forests with the third quartile of observed soil PyC concentration (0.056%), which was ∼1.5 times greater. Our models suggest that forests with the third quartile of observed soil PyC concentrations have 3.8% greater AGC gains under mean drought conditions, but 33.7% greater AGC gains under the most extreme drought conditions compared with forests with the first quartile of observed soil PyC concentrations. These results may translate to a difference in the gain of up to 0.68 Mg C ha–1yr–1 during a most extreme drought.
The best model to explain AGC losses also included WD and the interaction between soil PyC and MCWD anomalies. However, the effect size did not significantly differ from zero. We could not explain any of the variance in AGC net change during severe droughts with the tested variables (Table 1). We also found the non-PyC OC had no significant effect on AGC gain and loss with drought severity when tested instead of soil PyC (Supplementary Table 4).
Drought drives major reductions in the rate of biomass growth and increases tree mortality across Amazonia (Feldpausch et al., 2016; Aleixo et al., 2019). This is the first study to analyse the relationship between soil PyC, acting as a proxy of ancient fire legacies, and AGC dynamics in Amazonian forests. Overall, we found that soil PyC does not have a significant effect on the rates of AGC dynamics (Figure 4 and Supplementary Table 2). However, under severe droughts, the impact of drought on AGC gains is significantly greater in forests with low soil PyC compared to those with high soil PyC concentrations (Figure 5 and Table 1). This pattern, though, did not affect the overall AGC net change during severe droughts. Our results also show that soil PyC is positively correlated with soil fertility and clay and silt content (Figures 3A, E, F). Moreover, we found a negative correlation between soil PyC and tree wood density (Figure 3C). Our research highlights the potential importance of ancient fire legacies on forest soils and long-term forest succession, a basis for further investigations of the repercussions of ancient fires across Amazonia.
Our findings indicate that soil PyC is associated with increased soil fertility (Figure 3A). Studies of ADE and biochar (PyC) addition indicate that PyC can improve soil fertility via several mechanisms (Glaser et al., 2001; Czimczik and Masiello, 2007). PyC has a polycyclic aromatic structure and can persist in the environment for centuries to millennia (Bird et al., 2015). Therefore, PyC can reduce aluminum toxicity by increasing pH, increase cation exchange capacity, and improve water holding capacity (Glaser et al., 2001; Czimczik and Masiello, 2007). The ADE are historical indigenous lands where fire was frequently used as a land-management tool. Covering only 3% of Amazonia (McMichael et al., 2014), these small-scale areas (e.g., 0.5–300 ha in size) (Paz-Rivera and Putz, 2009) are more fertile and richer in soil PyC than adjacent areas (Glaser et al., 2002; Liang et al., 2006; Glaser, 2007). Our study highlights the impact of soil PyC-associated changes in soil fertility for non-ADE soils, representing the remaining 97% of the Amazon Basin, where we found a positive correlation between PyC and soil fertility.
We quantified total resistant PyC, representing PyC produced in situ by local fires and PyC that may have been deposited by aeolian transport from remote fires. The aerosol-derived PyC is derived semi-continuously from ancient to modern fires and fluxes have recently been estimated (fossil fuel + biomass burning) at approximately 6 kg km–2 yr–1 in Amazonia (Coppola et al., 2019). Given this rate, it would still take approx. 27,000 years to accumulate the estimated store of 1.1 Pg (0–30 cm) of PyC in the Amazon Basin from aerosol deposition alone (Koele et al., 2017). This suggests that the majority of the PyC analyzed derives from ancient local fires. Atmospheric transport, over centennial-scales, may also have been important, and these processes combined may have improved soil fertility over time.
Soil texture is a key determinant of soil fertility. There is a large range of physical and chemical soil properties across the Amazon Basin that vary according to gradients of pedogenic development, where nutrient pools are lowest in the most weathered soils (Quesada et al., 2010). We found the same pattern for soil PyC, with soil PyC concentration negatively correlated with soil sand content (Figure 3D), and occurring in highly weathered soils. Because of macropore predominance, sandy soils are more aerated and, consequently, destabilize PyC through high rates of oxidation and potentially greater rates of vertical translocation (Major et al., 2010). On the other hand, soils that experienced less weathering and, consequently, have more clay and silt, showed a positive association with PyC (Figures 3E, F) because of lower PyC oxidation rates. Our results corroborate other studies showing that soils with >50% clay content have significantly more PyC (Reisser et al., 2016). Moreover, clay-rich soils present more opportunities for organo-mineral interactions, helping to stabilize PyC (Sørensen, 1972; Six et al., 2002; Reisser et al., 2016).
We also found a negative correlation between wood density and soil PyC (Figure 3C). A previous large-scale fire experiment has already shown that tropical soft-wood trees are at greater risk of death from fire, in part because tree species with low wood density are less likely to close wounds post-fire (Balch et al., 2015). Moreover, wood density had a significant positive relationship with tree survival rates in areas burned repeatedly, where increasing wood density by 0.8 g cm–3 can enhance tree-level survival probability by 15% (Brando et al., 2012). Note that only one previous work has investigated the relationship between soil PyC and wood density and did not find a significant tendency (Massi et al., 2017). However, it included fewer than half the number of plots used in our study.
It is well established that wood density is negatively correlated with soil fertility (Baker et al., 2004; Quesada et al., 2012). We found a strong correlation between soil PyC and soil fertility (Figure 3A), and other previous studies have shown soil PyC can increase soil fertility as discussed previously in this section. Despite not being able to separate the origin of soil PyC (aerosol deposition or locally produced) in our analyses, we hypothesize that forests with high soil PyC concentrations added by aerosol deposition are more likely to have low wood density. This may be because soil PyC can increase soil fertility, leading to forests with fast stem turnover (Quesada et al., 2012). Wood density is also known to be related to successional stage and thus disturbance history. Meanwhile, forests with high concentrations of locally produced soil PyC may have experienced ancient fire disturbances in situ. Low wood density in these forests with high concentrations of soil PyC may be an indicator of disturbance and an earlier successional state, rather than merely because of changes in soil fertility. We attribute these differences to ancient fires because there was no evidence of recent disturbance events, although it should be noted that modern records of disturbance only go back <100 years.
Whilst we found correlations between soil PyC and soil fertility, soil texture and tree wood density, we were unable to directly test causal relationships in this study and these results should therefore be interpreted with caution. It should be noted that the highest concentrations of soil PyC were found in the West Amazon (Figure 2) that also has the most fertile soils, the lowest sand content and the lowest stand-level wood density (Quesada et al., 2012).
Our results do not show a relationship between soil PyC and AGC dynamics when analyzed across all census intervals. This result may be related to low concentrations of soil PyC found in the majority of the plots analyzed in this study. A study from eastern and southern Amazonia showed that biomass and forest composition had legacy effects in forests on or near ADE soils with high soil PyC and a history of ancient fires, which in general presented higher aboveground biomass (de Oliveira et al., 2020). It is likely that soil PyC has stronger relationships at high concentrations since the effect of PyC on soil fertility and water holding capacity is likely to be small at the concentrations found in this study (Glaser et al., 2002; Glaser, 2007). Moreover, disturbances in ADE are likely to be much greater (de Oliveira et al., 2020) and therefore they may still have differences in species composition and traits that would not have persisted in forests that were less disturbed.
Drought events can occur because of natural processes that are related to changes in sea surface temperature, e.g., Atlantic Multidecadal Oscillations, El Niño Southern Oscillations and Pacific Decadal Oscillations (Marengo and Espinoza, 2016; Aragão et al., 2018). However, anthropogenic actions such as land-use and land-cover changes and greenhouse gas emissions may exacerbate the intensity of droughts by changing patterns of large-scale atmospheric circulation (Spracklen and Garcia-Carreras, 2015; Llopart et al., 2018). Drought events in the Amazon are becoming more frequent, prolonged and intense as a result of both globally and locally driven climate change (Malhi et al., 2008; Dubreuil et al., 2012; Bonini et al., 2014). Amazonian forests are vulnerable to drought, which causes biomass losses and reduced forest productivity, enough to temporarily reverse a large multi-decadal aboveground carbon sink into mature forest biomass (Phillips et al., 2009; Feldpausch et al., 2016; Anderson et al., 2018; Gatti et al., 2021).
Our results show that during extreme drought events, forests with greater soil PyC have significantly higher rates of AGC gain compared to forests with lower soil PyC, with this difference increasing with drought severity (33.7% greater gains under the most extreme drought conditions) (Figure 5). These results may be driven by the capacity of soil PyC to hold more water in the soil (Glaser et al., 2002; de Melo Carvalho et al., 2014), alleviating the effects of severe droughts, and allowing trees to continue to grow under drought conditions. This significant effect on AGC gains during drought events in forests with higher soil PyC compared to those with lower soil PyC, may also be related to soil fertility, since forests with high concentrations of soil PyC are also more fertile soils. The drought immunization response is associated with the PyC fraction of TOC and not the non-PyC fraction of the organic carbon, since we found no significant relationship between OC and changes in AGC gains and losses with drought severity. Our models also suggest that PyC rather than soil fertility drives the patterns we observed. This indicates that the responses we found are potentially related to the effects of ancient fire on soil and vegetation (Quesada et al., 2012).
Some studies have shown that during extreme droughts, old-growth forests can lose more AGC than forests disturbed by past fires (Brando et al., 2014; Berenguer et al., 2021). Since old-growth forests have trees with higher wood density than those in disturbed forests, the death of few large trees in an old-growth forest can cause a greater amount of carbon loss than in disturbed forests (Brando et al., 2014; Berenguer et al., 2021). Therefore, our results may demonstrate that forests with a stronger history of fires, here identified by high concentrations of soil PyC, can be more resistant to current drought events. These forests may have lower overall biomass resulting from an establishment of long-term successional species, and consequently do not show a significant reduction in biomass growth during drought events.
In contrast to finding a significant relationship between PyC and AGC gains, we were unable to explain any of the variance in AGC losses (Table 1). Berenguer et al. (2021) found that forests increase the carbon loss rates for up to 3 years after a drought, and consequently, forest inventories taken in the first year after a drought may not detect the full impact of drought on tropical vegetation. Since many of our census intervals did not capture 3 years of post-drought dynamics, we may be failing to capture any effect of PyC on long-term carbon losses. Moreover, high spatial and temporal heterogeneity in tree mortality may prevent a signal from being detected in this study (Johnson et al., 2016; Pugh et al., 2020). Many of our plots were small (≤1 ha) or had short census intervals (<2 years), making it difficult to estimate AGC losses precisely. Much larger datasets may be needed to separate a trend from the large natural variance in tree mortality. Despite an increase in AGC gains, we are not able to detect an increase in AGC net change. Since spatial variation in carbon stocks depends more on losses than gains (Johnson et al., 2016; Hubau et al., 2020; Pugh et al., 2020), the caveats outlined above are also likely to hold true for the AGC net change data.
It is critical to understand the impacts of ancient fire on contemporary forest dynamics. Our results highlight the importance of understanding ancient fire regimes when predicting how species composition and carbon storage will change as a result of drought. Our results suggest ancient fires may influence current forest dynamics by altering soil fertility and favoring tree species capable of continued growth and recruitment during droughts. A major shift in the frequency and intensity of fires and droughts has occurred this century, bringing large uncertainties for future predictions of the carbon cycle (Aragão et al., 2007; Aragão et al., 2018; Silva Junior et al., 2019). This increase in fire represents a new fire regime for the Amazon (Aragão et al., 2018; Silveira et al., 2020). These fires bring several impacts to tropical rainforests such as changes in forest structure, species composition and carbon dynamics (Sato et al., 2016; Prestes et al., 2020; Silva et al., 2020; Pontes-Lopes et al., 2021). Forests that burned 30 years ago still have ∼25% less aboveground biomass than unburned forests (Silva et al., 2018), showing these impacts can persist over decades. From our analysis using soil PyC as a proxy and legacy of ancient fires, it is possible that forests which burned centuries ago may still not have recovered to their pristine state but instead continue to exhibit some attributes associated with recently disturbed or secondary forests (Berenguer et al., 2018; de Oliveira et al., 2020; Heinrich et al., 2021). Our study, however, suggests that mature forests that experienced fires centuries or millennia ago have greater resistance to short-term droughts, as a consequence of ancient fires. Further studies and experiments are needed to identify whether the effects of PyC on fertility and/or water holding capacity drive the patterns we observe, or whether PyC simply acts as a proxy of ancient fires.
The datasets presented in this article are not readily available but the raw data supporting the conclusions of this article will be available under reasonable request to the authors. Requests to access the datasets should be directed to TF, VC5SLkZlbGRwYXVzY2hAZXhldGVyLmFjLnVr.
LV, TF, LC, and LA conceived and designed the study. LV, LC, LP, CQ, MB, EO contributed to the soil sample collection for fertility and PyC analyses. LV performed the statistical analysis and wrote the manuscript with important contributions from all authors. All authors contributed to the forest inventories data collection and reviewed and approved the manuscript.
This research was supported by the Coordination of Improvement of Personnel in Higher Education, Brazil (CAPES) grant to LV (No. 88881.128127/2016-01), CAPES-Science without Borders grant to TF (PVE 177/2012), and NERC (NE/N011570/1). The Amazon forest plots in the RAINFOR network analyzed here were established, identified and measured with support from many colleagues and grants mentioned elsewhere. This research was also supported by the NERC/FAPESP grants “BIO-RED” (NE/N012542/1 and 2012/51872-5), “ECOFOR” (NE/K016431/1 and 2012/51509-8), “ARBOLES” (NE/S011811/1), the Royal Society for the Global Challenge Award “FORAMA” (ICA/R1/180100), the National Council for Science and Technology Development of Brazil (CNPq) grants Cerrado/Amazonia Transition Long-Term Ecology Project (PELD/441244/2016-5 and PELD/441572/2020-0), the Rede Amazônia Sustentável Long-term Ecology Project (PELD-RAS; 441659/2016-0 and 441573/2020-7), the PPBio Phytogeography of Amazonia/Cerrado Transition Project (CNPq/PPBio/457602/2012-0), FATE Project (CNPq 458022/2013-6), FAPESP (2016/21043-8), CNPq/INCT 4067/2022-0, and productivity grants (PQ1) to BMJ, BM, LA (CNPq 314416/2020-0). This study was carried out as a collaborative effort of the ForestPlots.net meta-network, a cyber-initiative developed at the University of Leeds that unites contributing scientists and their permanent plot records from the world’s tropical forests. This manuscript is supported by ForestPlots.net Research Project #97, led by LV.
We thank the Large-Scale Biosphere-Atmosphere Program for logistical and infrastructure support during field measurements in Belterra. Additionally, we also thank Paulo Graça and Toby Gardner for data availability.
The authors declare that the research was conducted in the absence of any commercial or financial relationships that could be construed as a potential conflict of interest.
All claims expressed in this article are solely those of the authors and do not necessarily represent those of their affiliated organizations, or those of the publisher, the editors and the reviewers. Any product that may be evaluated in this article, or claim that may be made by its manufacturer, is not guaranteed or endorsed by the publisher.
The Supplementary Material for this article can be found online at: https://www.frontiersin.org/articles/10.3389/ffgc.2023.1024101/full#supplementary-material
Aleixo, I., Norris, D., Hemerik, L., Barbosa, A., Prata, E., Costa, F., et al. (2019). Amazonian rainforest tree mortality driven by climate and functional traits. Nat. Clim. Change 9, 384–388. doi: 10.1038/s41558-019-0458-0
Anderson, L. O., Ribeiro Neto, G., Cunha, A. P., Fonseca, M. G., Mendes de Moura, Y., Dalagnol, R., et al. (2018). Vulnerability of Amazonian forests to repeated droughts. Philos. Trans. R. Soc. Lond. B Biol. Sci. 373, 20170411. doi: 10.1098/rstb.2017.0411
Aragão, L. E. O. C., Malhi, Y., Metcalfe, D. B., Silva-Espejo, J. E., Jimenez, E., Navarrete, D., et al. (2009). Above- and below-ground net primary productivity across ten Amazonian forests on contrasting soils. Biogeosciences 6, 2759–2778. doi: 10.5194/bg-6-2759-2009
Aragão, L. E. O. C., Malhi, Y., Roman-Cuesta, R. M., Saatchi, S., Anderson, L. O., and Shimabukuro, Y. E. (2007). Spatial patterns and fire response of recent Amazonian droughts. Geophys. Res. Lett. 34:L07701. doi: 10.1029/2006GL028946
Aragão, L. E., Anderson, L. O., Fonseca, M. G., Rosan, T. M., Vedovato, L. B., Wagner, F. H., et al. (2018). 21st Century drought-related fires counteract the decline of Amazon deforestation carbon emissions. Nat. Commun. 9:536. doi: 10.1038/s41467-017-02771-y
Ascough, P. L., Bird, M. I., Brock, F., Higham, T. F. G., Meredith, W., Snape, C. E., et al. (2009). Hydropyrolysis as a new tool for radiocarbon pre-treatment and the quantification of black carbon. Quat. Geochronol. 4, 140–147. doi: 10.1016/j.quageo.2008.11.001
Baker, T. R., Phillips, O. L., Malhi, Y., Almeida, S., Arroyo, L., Di Fiore, A., et al. (2004). Variation in wood density determines spatial patterns in Amazonian forest biomass. GCB 10, 545–562. doi: 10.1111/j.1365-2486.2004.00751.x
Balch, J. K., Brando, P. M., Nepstad, D. C., Coe, M. T., Silvério, D., Massad, T. J., et al. (2015). The susceptibility of southeastern Amazon forests to fire: Insights from a large-scale burn experiment. Bioscience 65, 893–905. doi: 10.1093/biosci/biv106
Barlow, J., and Peres, C. A. (2008). Fire-mediated dieback and compositional cascade in an Amazonian forest. Philos. Trans. R. Soc. Lond. B Biol. Sci. 363, 1787–1794. doi: 10.1098/rstb.2007.0013
Bates, D., Maechler, M., Bolker, B., Walker, S., Christensen, R. H. B., Singmann, H., et al. (2018). Package ‘lme4’. Version 1:437.
Berenguer, E., Lennox, G. D., Ferreira, J., Malhi, Y., Aragão, L. E. O. C., Barreto, J. R., et al. (2021). Tracking the impacts of El Niño drought and fire in human-modified Amazonian forests. Proc. Natl. Acad. Sci. U.S.A. 118:e2019377118. doi: 10.1073/pnas.2019377118
Berenguer, E., Malhi, Y., Brando, P., Cardoso Nunes Cordeiro, A., Ferreira, J., França, F., et al. (2018). Tree growth and stem carbon accumulation in human-modified Amazonian forests following drought and fire. Philos. Trans. R. Soc. B Biol. Sci. 373:20170308. doi: 10.1098/rstb.2017.0308
Bird, M. I., Wynn, J. G., Saiz, G., Wurster, C. M., and McBeath, A. (2015). The pyrogenic carbon cycle. Annu. Rev. Earth Planet. Sci. 43, 273–298. doi: 10.1146/annurev-earth-060614-105038
Bjørnstad, O. N., and Falck, W. (2001). Nonparametric spatial covariance functions: Estimation and testing. Environ. Ecol. Stat. 8, 53–70. doi: 10.1023/A:1009601932481
Bonini, I., Rodrigues, C., Dallacort, R., Marimon Junior, B. H., and Carvalho, M. A. C. (2014). Rainfall and deforestation in the municipality of Colíder, southern Amazon. Rev. Brasil. Meteorol. 29, 483–493. doi: 10.1590/0102-778620130665
Brando, P. M., Balch, J. K., Nepstad, D. C., Morton, D. C., Putz, F. E., Coe, M. T., et al. (2014). Abrupt increases in Amazonian tree mortality due to drought–fire interactions. Proc. Natl. Acad. Sci. U.S.A. 111, 6347–6352. doi: 10.1073/pnas.1305499111
Brando, P. M., Nepstad, D. C., Balch, J. K., Bolker, B., Christman, M. C., Coe, M., et al. (2012). Fire-induced tree mortality in a neotropical forest: The roles of bark traits, tree size, wood density and fire behavior. Glob. Chang. Biol. 18, 630–641. doi: 10.1111/j.1365-2486.2011.02533.x
Brienen, R. J. W., Phillips, O. L., Feldpausch, T. R., Gloor, E., Baker, T. R., Lloyd, J., et al. (2015). Long-term decline of the Amazon carbon sink. Nature 519, 344–348. doi: 10.1038/nature14283
Brodowski, S., John, B., Flessa, H., and Amelung, W. (2006). Aggregate-occluded black carbon in soil. Eur. J. Soil Sci. 57, 539–546. doi: 10.1111/j.1365-2389.2006.00807.x
Bush, M. B., Silman, M. R., McMichael, C., and Saatchi, S. (2008). Fire, climate change and biodiversity in Amazonia: A Late-Holocene perspective. Philos. Trans. R. Soc. Lond. B Biol. Sci. 363, 1795–1792. doi: 10.1098/rstb.2007.0014
Coppola, A. I., Seidel, M., Ward, N. D., Viviroli, D., Nascimento, G. S., Haghipour, N., et al. (2019). Marked isotopic variability within and between the Amazon River and marine dissolved black carbon pools. Nat. Commun. 10:4018. doi: 10.1038/s41467-019-11543-9
Czimczik, C. I., and Masiello, C. A. (2007). Controls on black carbon storage in soils. Global Biogeochem. Cycles 21:GB3005. doi: 10.1029/2006GB002798
da Rocha, H. R., Goulden, M. L., Miller, S. D., Menton, M. C., Pinto, L. D. V. O., de Freitas, H. C., et al. (2004). Seasonality of water and heat fluxes over a tropical forest in eastern Amazonia. Ecol. Appl. 14, 22–32. doi: 10.1890/02-6001
de Melo Carvalho, M., de Holanda Nunes Maia, A., Madari, B., Bastiaans, L., Van Oort, P., Heinemann, A., et al. (2014). Biochar increases plant-available water in a sandy loam soil under an aerobic rice crop system. Solid Earth 5, 939–952. doi: 10.5194/se-5-939-2014
de Oliveira, E. A., Marimon-Junior, B. H., Marimon, B. S., Iriarte, J., Morandi, P. S., Maezumi, S. Y., et al. (2020). Legacy of Amazonian dark earth soils on forest structure and species composition. Global Ecol. Biogeogr. 29, 1458–1473. doi: 10.1111/geb.13116
Doughty, C. E., Metcalfe, D. B., da Costa, M. C., de Oliveira, A. A. R., Neto, G. F. C., Silva, J. A., et al. (2014). The production, allocation and cycling of carbon in a forest on fertile terra preta soil in eastern Amazonia compared with a forest on adjacent infertile soil. Plant Ecol. Divers. 7, 41–53. doi: 10.1080/17550874.2013.798367
Duan, Z., Liu, J., Tuo, Y., Chiogna, G., and Disse, M. (2016). Evaluation of eight high spatial resolution gridded precipitation products in Adige Basin (Italy) at multiple temporal and spatial scales. Sci. Total Environ. 573, 1536–1553. doi: 10.1016/j.scitotenv.2016.08.213
Dubreuil, V., Debortoli, N., Funatsu, B., Nédélec, V., and Durieux, L. (2012). Impact of land-cover change in the Southern Amazonia climate: A case study for the region of Alta Floresta, Mato Grosso, Brazil. Environ. Monit. Assess. 184, 877–891. doi: 10.1007/s10661-011-2006-x
Erickson, C. L. (2008). “Amazonia: The historical ecology of a domesticated landscape,” in The handbook of South American archaeology, eds H. Silverman and W. H. Isbell (New York, NY: Springer New York), 157–183. doi: 10.1007/978-0-387-74907-5_11
Feldpausch, T. R., Banin, L., Phillips, O. L., Baker, T. R., Lewis, S. L., Quesada, C. A., et al. (2011). Height-diameter allometry of tropical forest trees. Biogeosciences 8, 1081–1106. doi: 10.5194/bg-8-1081-2011
Feldpausch, T. R., Carvalho, L., Macario, K. D., Ascough, P. L., Flores, C. F., Coronado, E. N. H., et al. (2022). Forest fire history in Amazonia inferred from intensive soil charcoal sampling and radiocarbon dating. Front. For. Glob. Change 5:815438. doi: 10.3389/ffgc.2022.815438
Feldpausch, T. R., Phillips, O. L., Brienen, R. J. W., Gloor, E., Lloyd, J., Lopez-Gonzalez, G., et al. (2016). Amazon forest response to repeated droughts. Global Biogeochem. Cycles 30, 964–982. doi: 10.1002/2015GB005133
ForestPlots.net, Blundo, C., Carilla, J., Grau, R., Malizia, A., and Malizia, L. (2021). Taking the pulse of Earth’s tropical forests using networks of highly distributed plots. Biol. Conserv. 260:108849. doi: 10.1016/j.biocon.2020.108849
França, F. M., Benkwitt, C. E., Peralta, G., Robinson, J. P. W., Graham, N. A. J., Tylianakis, J. M., et al. (2020). Climatic and local stressor interactions threaten tropical forests and coral reefs. Philos. Trans. R. Soc. Lond. B Biol. Sci. 375:20190116. doi: 10.1098/rstb.2019.0116
Funk, C., Peterson, P., Landsfeld, M., Pedreros, D., Verdin, J., Shukla, S., et al. (2015). The climate hazards infrared precipitation with stations—a new environmental record for monitoring extremes. Sci. Data 2:150066. doi: 10.1038/sdata.2015.66
Gatti, L. V., Basso, L. S., Miller, J. B., Gloor, M., Gatti Domingues, L., Cassol, H. L. G., et al. (2021). Amazonia as a carbon source linked to deforestation and climate change. Nature 595, 388–393. doi: 10.1038/s41586-021-03629-6
Glaser, B. (2007). Prehistorically modified soils of central Amazonia: A model for sustainable agriculture in the twenty-first century. Philos. Trans. R. Soc. Lond. B Biol. Sci. 362, 187–196. doi: 10.1098/rstb.2006.1978
Glaser, B., Haumaier, L., Guggenberger, G., and Zech, W. (2001). The ‘Terra Preta’ phenomenon: A model for sustainable agriculture in the humid tropics. Naturwissenschaften 88, 37–41. doi: 10.1007/s001140000193
Glaser, B., Lehmann, J., and Zech, W. (2002). Ameliorating physical and chemical properties of highly weathered soils in the tropics with charcoal - a review. Biol. Fertil. Soils 35, 219–230. doi: 10.1007/s00374-002-0466-4
Goulart, A. C., Macario, K. D., Scheel-Ybert, R., Alves, E. Q., Bachelet, C., Pereira, B. B., et al. (2017). Charcoal chronology of the Amazon forest: A record of biodiversity preserved by ancient fires. Quat. Geochronol. 41, 180–186. doi: 10.1016/j.quageo.2017.04.005
Greenwood, S., Ruiz-Benito, P., Martínez-Vilalta, J., Lloret, F., Kitzberger, T., Allen, C. D., et al. (2017). Tree mortality across biomes is promoted by drought intensity, lower wood density and higher specific leaf area. Ecol. Lett. 20, 539–553. doi: 10.1111/ele.12748
Heinrich, V. H. A., Dalagnol, R., Cassol, H. L. G., Rosan, T. M., de Almeida, C. T., Silva Junior, C. H. L., et al. (2021). Large carbon sink potential of secondary forests in the Brazilian Amazon to mitigate climate change. Nat. Commun. 12:1785. doi: 10.1038/s41467-021-22050-1
Hubau, W., Lewis, S. L., Phillips, O. L., Affum-Baffoe, K., Beeckman, H., Cuní-Sanchez, A., et al. (2020). Asynchronous carbon sink saturation in African and Amazonian tropical forests. Nature 579, 80–87. doi: 10.1038/s41586-020-2035-0
Husson, F., Josse, J., Le, S., Mazet, J., and Husson, M. F. (2016). Package ‘FactoMineR’. R package 96:698.
IPCC (2006). “Chapter 4 forest land,” in IPCC Guidelines for national greenhouse gas inventories, eds H. S. Eggleston, L. Buendia, K. Miwa, T. Ngara, and K. Tanabe (Geneva: IPCC).
Johnson, M. O., Galbraith, D., Gloor, M., De Deurwaerder, H., Guimberteau, M., Rammig, A., et al. (2016). Variation in stem mortality rates determines patterns of above-ground biomass in Amazonian forests: Implications for dynamic global vegetation models. Global Change Biol. 22, 3996–4013. doi: 10.1111/gcb.13315
Kassambara, A., and Mundt, F. (2017). Factoextra: Extract and visualize the results of multivariate data analyses. R package version 1, 337–354.
Katsanos, D., Retalis, A., and Michaelides, S. (2016). Validation of a high-resolution precipitation database (CHIRPS) over Cyprus for a 30-year period. Atmos. Res. 169, 459–464. doi: 10.1016/j.atmosres.2015.05.015
Koele, N., Bird, M., Haig, J., Marimon-Junior, B. H., Marimon, B. S., Phillips, O. L., et al. (2017). Amazon Basin forest pyrogenic carbon stocks: First estimate of deep storage. Geoderma 306, 237–243. doi: 10.1016/j.geoderma.2017.07.029
Lehmann, J., Liang, B., Solomon, D., Lerotic, M., Luizão, F., Kinyangi, J., et al. (2005). Near-edge X-ray absorption fine structure (NEXAFS) spectroscopy for mapping nano-scale distribution of organic carbon forms in soil: Application to black carbon particles. Global Biogeochem. Cycles 19:GB1013. doi: 10.1029/2004GB002435
Liang, B., Lehmann, J., Solomon, D., Kinyangi, J., Grossman, J., O’Neill, B., et al. (2006). Black carbon increases cation exchange capacity in soils. Soil Sci. Soc. Am. J. 70, 1719–1730. doi: 10.2136/sssaj2005.0383
Llopart, M., Reboita, M. S., Coppola, E., Giorgi, F., Da Rocha, R. P., and De Souza, D. O. (2018). Land use change over the Amazon Forest and its impact on the local climate. Water 10:149. doi: 10.3390/w10020149
Lopes, A. V., Chiang, J. C. H., Thompson, S. A., and Dracup, J. A. (2016). Trend and uncertainty in spatial-temporal patterns of hydrological droughts in the Amazon basin. Geophys. Res. Lett. 43, 3307–3316. doi: 10.1002/2016GL067738
Lopez-Gonzalez, G., Lewis, S., Burkitt, M., and Phillips, O. (2011). ForestPlots.net: A web application and research tool to manage and analyse tropical forest plot data. J. Veg. Sci. 22, 610–613. doi: 10.1111/j.1654-1103.2011.01312.x
Major, J., Lehmann, J., Rondon, M., and Goodale, C. (2010). Fate of soil-applied black carbon: Downward migration, leaching and soil respiration. Global Change Biol. 16, 1366–1379. doi: 10.1111/j.1365-2486.2009.02044.x
Malhi, Y., Roberts, J. T., Betts, R. A., Killeen, T. J., Li, W. H., and Nobre, C. A. (2008). Climate change, deforestation, and the fate of the Amazon. Science 319, 169–172. doi: 10.1126/science.1146961
Marengo, J. A., and Espinoza, J. C. (2016). Extreme seasonal droughts and floods in Amazonia: Causes, trends and impacts. Int. J. Climatol. 36, 1033–1050. doi: 10.1002/joc.4420
Massi, K. G., Bird, M., Marimon, B. S., Marimon, B. H., Nogueira, D. S., Oliveira, E. A., et al. (2017). Does soil pyrogenic carbon determine plant functional traits in Amazon Basin forests? Plant Ecol. 218, 1047–1062. doi: 10.1007/s11258-017-0751-9
McMichael, C., Palace, M., Bush, M., Braswell, B., Hagen, S., Neves, E., et al. (2014). Predicting pre-Columbian anthropogenic soils in Amazonia. Proc. R. Soc. B Biol. Sci. 281:20132475. doi: 10.1098/rspb.2013.2475
Meredith, W., Ascough, P. L., Bird, M. I., Large, D. J., Snape, C. E., Song, J., et al. (2013). Direct evidence from hydropyrolysis for the retention of long alkyl moieties in black carbon fractions isolated by acidified dichromate oxidation. J. Anal. Appl. Pyrolysis. 103, 232–239. doi: 10.1016/j.jaap.2012.11.001
Paredes-Trejo, F., Barbosa, H. A., Giovannettone, J., Lakshmi Kumar, T. V., Thakur, M. K., and de Oliveira Buriti, C. (2021). Long-term spatiotemporal variation of droughts in the Amazon River basin. Water 13:351. doi: 10.3390/w13030351
Paz-Rivera, C., and Putz, F. E. (2009). Anthropogenic soils and tree distributions in a lowland forest in Bolivia. Biotropica 41, 665–675. doi: 10.1111/j.1744-7429.2009.00521.x
Perdigón-Morales, J., Romero-Centeno, R., Pérez, P. O., and Barrett, B. S. (2018). The midsummer drought in Mexico: Perspectives on duration and intensity from the CHIRPS precipitation database. Int. J. Climatol. 38, 2174–2186. doi: 10.1002/joc.5322
Phillips, O. L., Aragao, L. E., Lewis, S. L., Fisher, J. B., Lloyd, J., Lopez-Gonzalez, G., et al. (2009). Drought sensitivity of the Amazon rainforest. Science 323, 1344–1347. doi: 10.1126/science.1164033
Pontes-Lopes, A., Silva, C. V. J., Barlow, J., Rincón, L. M., Campanharo, W. A., Nunes, C. A., et al. (2021). Drought-driven wildfire impacts on structure and dynamics in a wet Central Amazonian forest. Proc. R. Soc. B Biol. Sci. 288:20210094. doi: 10.1098/rspb.2021.0094
Prestes, N.C.CdS, Massi, K. G., Silva, E. A., Nogueira, D. S., de Oliveira, E. A., Freitag, R., et al. (2020). Fire effects on understory forest regeneration in Southern Amazonia. Front. For. Glob. Change 3:10. doi: 10.3389/ffgc.2020.00010
Pugh, T. A. M., Rademacher, T., Shafer, S. L., Steinkamp, J., Barichivich, J., Beckage, B., et al. (2020). Understanding the uncertainty in global forest carbon turnover. Biogeosciences 17, 3961–3989. doi: 10.5194/bg-17-3961-2020
Quesada, C. A., Lloyd, J., Schwarz, M., Patiño, S., Baker, T. R., Czimczik, C., et al. (2010). Variations in chemical and physical properties of Amazon forest soils in relation to their genesis. Biogeosciences 7, 1515–1541. doi: 10.5194/bg-7-1515-2010
Quesada, C. A., Paz, C., Oblitas Mendoza, E., Phillips, O. L., Saiz, G., and Lloyd, J. (2020). Variations in soil chemical and physical properties explain basin-wide Amazon forest soil carbon concentrations. Soil 6, 53–88. doi: 10.5194/soil-6-53-2020
Quesada, C. A., Phillips, O. L., Schwarz, M., Czimczik, C. I., Baker, T. R., Patino, S., et al. (2012). Basin-wide variations in Amazon forest structure and function are mediated by both soils and climate. Biogeosciences 9, 2203–2246. doi: 10.5194/bg-9-2203-2012
Rehn, E., Rowe, C., Ulm, S., Woodward, C., and Bird, M. (2021). A late-Holocene multiproxy fire record from a tropical savanna, eastern Arnhem Land, Northern Territory, Australia. Holocene 31, 870–883. doi: 10.1177/0959683620988030
Reisser, M., Purves, R. S., Schmidt, M. W. I., and Abiven, S. (2016). Pyrogenic carbon in soils: A literature-based inventory and a global estimation of its content in soil organic carbon and stocks. Front. Earth Sci. 4:80. doi: 10.3389/feart.2016.00080
Richards, P. W. (1973). The tropical rain forest. Sci. Am. 229, 58–68. doi: 10.1038/scientificamerican1273-58
Rumpel, C., Chaplot, V., Planchon, O., Bernadou, J., Valentin, C., and Mariotti, A. (2006). Preferential erosion of black carbon on steep slopes with slash and burn agriculture. Catena 65, 30–40. doi: 10.1016/j.catena.2005.09.005
Sanford, R. L. Jr., Saldarriaga, J., Clark, K. E., Uhl, C., and Herrera, R. (1985). Amazon rain-forest fires. Science 227, 53–55. doi: 10.1126/science.227.4682.53
Santos, G. M., Gomes, P. R. S., Anjos, R. M., Cordeiro, R. C., Turcq, B. J., Sifeddine, A., et al. (2000). 14C AMS dating of fires in the central Amazon rain forest. Nuclear Instr. Methods Phys. Res. Sec. B 172, 761–766. doi: 10.1016/S0168-583X(00)00234-2
Sato, L. Y., Gomes, V. C. F., Shimabukuro, Y. E., Keller, M., Arai, E., dos-Santos, M. N., et al. (2016). Post-fire changes in forest biomass retrieved by airborne LiDAR in Amazonia. Remote Sens. 8:839. doi: 10.3390/rs8100839
Silva Junior, C. H. L., Anderson, L. O., Silva, A. L., Almeida, C. T., Dalagnol, R., Pletsch, M. A. J. S., et al. (2019). Fire responses to the 2010 and 2015/2016 Amazonian droughts. Front. Earth Sci. 7:97. doi: 10.3389/feart.2019.00097
Silva Junior, C. H. L., Aragão, L. E. O. C., Fonseca, M. G., Almeida, C. T., Vedovato, L. B., and Anderson, L. O. (2018). Deforestation-induced fragmentation increases forest fire occurrence in central Brazilian Amazonia. Forests 9:305. doi: 10.3390/f9060305
Silva Junior, C. H. L., Aragão, L., Anderson, L., Fonseca, M., Shimabukuro, Y., Vancutsem, C., et al. (2020). Persistent collapse of biomass in Amazonian forest edges following deforestation leads to unaccounted carbon losses. Sci. Adv. 6:eaaz8360. doi: 10.1126/sciadv.aaz8360
Silva, C. V. J., Aragão, L. E. O. C., Young, P. J., Espirito-Santo, F., Berenguer, E., Anderson, L. O., et al. (2020). Estimating the multi-decadal carbon deficit of burned Amazonian forests. Environ. Res. Lett. 15:114023. doi: 10.1088/1748-9326/abb62c
Silva, C. V. J., Aragao, L., Barlow, J., Espirito-Santo, F., Young, P. J., Anderson, L. O., et al. (2018). Drought-induced Amazonian wildfires instigate a decadal-scale disruption of forest carbon dynamics. Philos. Trans. R. Soc. Lond. B Biol. Sci. 373:20180043. doi: 10.1098/rstb.2018.0043
Silveira, M. V. F., Petri, C. A., Broggio, I. S., Chagas, G. O., Macul, M. S., Leite, C. C. S. S., et al. (2020). Drivers of fire anomalies in the Brazilian Amazon: Lessons learned from the 2019 fire crisis. Land 9:516. doi: 10.3390/land9120516
Six, J., Conant, R. T., Paul, E. A., and Paustian, K. (2002). Stabilization mechanisms of soil organic matter: Implications for C-saturation of soils. Plant Soil 241, 155–176. doi: 10.1023/A:1016125726789
Sørensen, L. H. (1972). Stabilization of newly formed amino acid metabolites in soil by clay minerals. Soil Sci. 114, 5–11. doi: 10.1097/00010694-197207000-00002
Spracklen, D., and Garcia-Carreras, L. (2015). The impact of Amazonian deforestation on Amazon basin rainfall. Geophys. Res. Lett. 42, 9546–9552. doi: 10.1002/2015GL066063
Verdin, A., Funk, C., Rajagopalan, B., and Kleiber, W. (2016). Kriging and local polynomial methods for blending satellite-derived and gauge precipitation estimates to support hydrologic early warning systems. IEEE Trans. Geosci. Remote Sens. 54, 2552–2562. doi: 10.1109/TGRS.2015.2502956
von Randow, C., Manzi, A. O., Kruijt, B., de Oliveira, P. J., Zanchi, F. B., Silva, R. L., et al. (2004). Comparative measurements and seasonal variations in energy and carbon exchange over forest and pasture in South West Amazonia. Theor. Appl. Climatol. 78, 5–26. doi: 10.1007/s00704-004-0041-z
Keywords: historical fires, soil fertility, wood density, carbon sequestration, soil pyrogenic carbon, water deficit, forest composition
Citation: Vedovato LB, Carvalho LCS, Aragão LEOC, Bird M, Phillips OL, Alvarez P, Barlow J, Bartholomew DC, Berenguer E, Castro W, Ferreira J, França FM, Malhi Y, Marimon B, Marimon Júnior BH, Monteagudo A, Oliveira EA, Pereira LO, Pontes-Lopes A, Quesada CA, Silva CVJ, Silva Espejo JE, Silveira M and Feldpausch TR (2023) Ancient fires enhance Amazon forest drought resistance. Front. For. Glob. Change 6:1024101. doi: 10.3389/ffgc.2023.1024101
Received: 21 August 2022; Accepted: 06 January 2023;
Published: 14 February 2023.
Edited by:
William H. McDowell, University of New Hampshire, United StatesReviewed by:
Joseph Yavitt, Cornell University, United StatesCopyright © 2023 Vedovato, Carvalho, Aragão, Bird, Phillips, Alvarez, Barlow, Bartholomew, Berenguer, Castro, Ferreira, França, Malhi, Marimon, Marimon Júnior, Monteagudo, Oliveira, Pereira, Pontes-Lopes, Quesada, Silva, Silva Espejo, Silveira and Feldpausch. This is an open-access article distributed under the terms of the Creative Commons Attribution License (CC BY). The use, distribution or reproduction in other forums is permitted, provided the original author(s) and the copyright owner(s) are credited and that the original publication in this journal is cited, in accordance with accepted academic practice. No use, distribution or reproduction is permitted which does not comply with these terms.
*Correspondence: Laura B. Vedovato, bHYyODdAZXhldGVyLmFjLnVr
Disclaimer: All claims expressed in this article are solely those of the authors and do not necessarily represent those of their affiliated organizations, or those of the publisher, the editors and the reviewers. Any product that may be evaluated in this article or claim that may be made by its manufacturer is not guaranteed or endorsed by the publisher.
Research integrity at Frontiers
Learn more about the work of our research integrity team to safeguard the quality of each article we publish.