- 1School of Science, Auckland University of Technology, Auckland, New Zealand
- 2Te Ara Poutama, Auckland University of Technology, Auckland, New Zealand
- 3Centre for Biodiversity and Biosecurity, School of Biological Sciences, University of Auckland, Auckland, New Zealand
Tree planting has long played a major role in the New Zealand Government’s approach to climate mitigation and is increasingly understood as important for climate adaptation. However, large-scale tree planting in Aotearoa New Zealand has been dominated by exotic species. Although there is growing public and expert support for using native species for forest revegetation in farm landscapes, there are two key barriers. First, the lack of ecological and economic data on native species performance in different environmental conditions. Second, policy and market-related mechanisms associated with carbon sequestration, such as the New Zealand Emissions Trading Scheme, favor the continuing use of exotic tree species, especially Pinus radiata, over native species. Consequently, there are strong incentives for exotic forests and insufficient financial support for natives, even when native forest re-establishment is often the preference of landowners, Indigenous peoples, and local communities. The AUT Living Laboratories Program is a long-term, transdisciplinary, experimental restoration research program aimed at addressing scientific, social, and economic knowledge gaps for native revegetation as a Nature-based Solution (NbS) on farmland soils. Here, we present the project design and establishment information from the three experimental restoration sites, which vary in native species composition, planting configuration, and environmental and socio-cultural context. Each site involves partnerships with Indigenous communities, specifically Ngāti Whātua Ōrākei, Ngāti Manuhiri, and Ngāti Pāoa, to value and embed mātauranga Māori as Indigenous knowledge. Monitoring carbon sequestration along with changes in ecological functions and outcomes, including native biodiversity, will be critical to ensure that large-scale tree-planting aligns with the government’s strategies for climate change, native biodiversity, and economic prosperity.
Introduction
Background
Nature-based Solutions (NbS) are increasingly appreciated as a vital aspect of well-integrated climate change strategies at local, regional, and global scales (Seddon et al., 2020b). Through the protection, restoration, management, and creation of biodiverse, multifunctional ecosystems, it is possible to produce ecological functions that address simultaneous challenges including climate change, biodiversity loss, and environmental amelioration (Cohen-Shacham et al., 2016; Seddon et al., 2020a,2021). In Aotearoa New Zealand, NbS has recently gained official endorsement through the development of the government’s Emissions Reduction Plan (New Zealand Government, 2022) and its draft National Adaptation Plan (Ministry for the Environment, 2022). Given the relative prominence of land-based activities in Aotearoa New Zealand’s emissions profile, the potential for agricultural NbS to contribute to climate change policy is especially promising (Miralles-Wilhelm, 2021; Simelton et al., 2021). In 2019, agriculture was responsible for 48.1% of Aotearoa New Zealand’s gross emissions (39.6 mt/CO2e), while the Land Use, Land-Use Change and Forestry (LULUCF) sector offsets about –33% by producing 27.4 mt/CO2e of carbon dioxide removals (Ministry for the Environment, 2021). By deploying NbS, this source of climate mitigation might also deliver a rich suite of co-benefits to address other policy objectives, such as native biodiversity protection, reparations for Indigenous peoples, and reducing the contribution of erosion and sedimentation to declining water quality.
However, multifunctional solutions to societal challenges require an integrated policy approach (Pörtner et al., 2021). At least until the implementation of new climate governance arrangements in 2019, the New Zealand Government has lacked coherence and coordination in its policy mix (Hall, 2020). Over the previous decade, climate policy was dominated by a single instrument, the Emissions Trading Scheme (NZ ETS), with climate mitigation as its target. As its price signal has risen, the NZ ETS has strengthened the incentive for exotic monoculture forests that sequester carbon more rapidly than native species, which entails a trade-off for other policy targets such as increasing indigenous biodiversity. In the second quarter of 2022, new NZ ETS registrations of post-1989 forest increased to more than 80,000 hectares, from an average of only 4,000 hectares per quarter from the 4 years up to the second quarter of 2021. Yet, of the 387,361 hectares registered as of 30th June 2022, only 11% was indigenous forest, and the remaining 89% in mixed exotic species (Te Uru Rākau, 2022). This strengthened financial incentive also builds upon a century-long preference for exotic plantation forestry for timber supply, again driven by the higher yields of exotic species. The result is an asymmetry of practical knowledge, where exotic species with commercial uses have been the subject of intensive scientific research, genetic improvement, and integration into economic and climate modeling (e.g., Burdon et al., 2008; Watt et al., 2010). By contrast, native species have been relatively neglected by scientific researchers, which disadvantages their incorporation into evidence-based policy. Furthermore, the failure to integrate Indigenous knowledge into policy development has meant that a valuable source of empirical and practical knowledge was neglected in policy and market design. Thus, while the need to accelerate the deployment of NbS is increasingly understood as critical and urgent by the New Zealand Government, there are a range of knowledge gaps and policy misalignments to overcome.
Monitoring long-term experimental forest restoration plantings—projects that aim to understand how ecological properties and processes change over multiple decades as forested ecosystems are restored in urban or farmland contexts—can be used to address these key knowledge gaps. While community-led native forest restoration projects have long contributed to woody revegetation (re-integrating of woody vegetation) within urban and production landscapes (Peters et al., 2015), very few of these were established as science experiments or have had baseline or ongoing measurements of either the planted trees or the ecosystems in which they are situated. Nonetheless, these activities have generated useful practical information regarding restoration practices and outcomes (Porteous, 1993), some of which is available, for instance, via local government or non-governmental organization websites (e.g., Tane’s Tree Trust1). Shorter term (multiple year) experimental and observational research studies have provided more detailed scientific insights into critical aspects of native forest restoration, including: survival and performance of planted trees (e.g., MacKay et al., 2011), direct seeding techniques and related seed and site preparation (e.g., Douglas et al., 2007; Paul et al., 2020), mammalian herbivore control and fencing (e.g., Dodd et al., 2011; Burns et al., 2012), successional trajectories and natural regeneration (e.g., Wallace et al., 2022), impacts of invasive species (Norton, 2009), and management interventions to improve native forest establishment and success (e.g., Forbes et al., 2020; Tulod and Norton, 2020), among others. However, there remains a paucity of longer-term restoration experiments in New Zealand to fill knowledge gaps regarding the performance and function of native species in agroecosystems, to track biodiversity and ecosystem function in the face of climatic change, and to support the ongoing development of NbS planning and policy frameworks.
The AUT Living Laboratories program
The AUT (Auckland University of Technology) Living Laboratories program has been established as a multi-decadal, transdisciplinary, experimental restoration research program aimed at addressing scientific, social, and economic knowledge gaps for native revegetation as NbS on previously farmed soils. Drawing on post-normal science and its emphasis on cultivating a plurality of knowledges in the face of complex, urgent challenges (Funtowicz and Ravetz, 1993), each AUT Living Laboratories project is co-produced by an extended peer community (Meisch et al., 2022) which includes landowners and Indigenous partners. Thus, the program aims to produce and mobilize knowledge, both from Western scientific and Indigenous paradigms, to support the use of NbS as a strategic contribution to climate action, biodiversity improvement, and decolonization. The overarching purpose of the program is to address the question: How can ecological experiments be designed and implemented in Aotearoa New Zealand to support holistic approaches to revegetation that address multiple challenges, especially climate change and biodiversity loss? Underpinning this question are the contextual elements that make the experiments place-based, in terms of its implications for Indigenous and local communities and endemic native species.
In this paper, we present the experimental design and monitoring methodology for three long-term restoration experiments being established within the AUT Living Laboratories program. Building on existing knowledge regarding native restoration practice in New Zealand and globally, an overall aim of the Living Laboratories experiments is to gain new and useful insights into how native species composition, planting configuration, and environmental context influence the rate of restoration of mature, late-successional native forest ecosystems on lands with a multi-decadal legacy of pastoral farming. Mature native forest is multi-tiered and contains self-sustaining populations of tree species that traditionally occur in the canopy/emergent tiers of mature, podocarp-broadleaved forests typical of the upper North Island of Aotearoa New Zealand. These systems are resilient (Peterson et al., 1998; Gunderson, 2000), have ecological integrity (McGlone et al., 2020; Griffiths et al., 2021), have high species occupancy (Lee et al., 2005), and are dominated by indigenous species. The program also aims to characterize and quantify the socio-cultural and economic contexts of the restoration experiments to understand their respective roles in establishing successful restoration outcomes on previously farmed land. For example, we will be recording the costs of setting up each site, including the number and type of ongoing interventions, such as weeding, needed to establish the site and plantings to enable future economic analysis.
From an ecological science perspective, the experiments are aimed at collecting a comprehensive dataset to address a range of questions relevant to native restoration and the implementation of NbS in New Zealand farm landscapes, including: (1) Does planting late-successional species at the same time as nurse species accelerate succession? (2) How does microhabitat variation (e.g., soil chemistry, moisture, and compaction) affect tree seedling establishment and growth rate? (3) How does distance from existing native seed sources affect natural woody plant regeneration? (4) How does the composition of the late-successional and nurse species affect seedling establishment, tree growth, and canopy closure rates? (5) How do characteristics of the restoration plantings, such as planting density and environmental context, affect biodiversity and ecosystem functioning? New and emerging research questions will be accommodated as the three restoration experiments begin to mature over the next 10–50 years through the integration of sub-experiments or observational studies within the foundational experimental design. Comparisons of collected data and results against other restoration datasets from across New Zealand will be undertaken to enable generalization to other contexts across the country.
Critically, the experiments were developed based on the establishment of genuine partnerships with Indigenous Māori communities to create opportunities for the embedding of mātauranga Māori as Indigenous knowledge. More generally, the project aims to lay the foundations for a knowledge base to support NbS in Aotearoa New Zealand, and to address the obstacles that currently face the upscaling of NbS, by taking a holistic approach to restoration that integrates social science, partnerships with land stakeholders and ecological science (e.g., Lyver et al., 2015; Fleischman et al., 2020). Thus, the AUT Living Laboratories program is intended to reduce project risks for landowners, accelerate learning curves to decrease costs of tree planting and maintenance, improve understanding of the wider ecological benefits of native afforestation, and enhance the legitimacy of NbS by creating partnership approaches with Māori who hold mana whenua (ancestral links) with the land. This knowledge will inform the design of policies and economic instruments that might upscale NbS further.
Living Laboratories experimental forest restoration sites
The AUT Living Laboratories program comprises three long-term restoration sites established in New Zealand’s upper North Island (Figure 1). Each site differs in social context, environmental context, planted species, and experimental treatments (Tables 1, 2). The treatments encompass replicate groups of mature, canopy-dominant (late-successional), and nurse (early successional) tree species across each site that will be subjected to different experimental conditions or manipulations. No exotic species were introduced, neither as experimental controls nor as nurse species to facilitate succession, because site partners expressed a strong preference for native species only. All sites are being maintained on an ongoing basis beyond the initial site setup, with the goal of establishing biodiverse forest patches. This will involve in-filling of seedlings that do not initially establish, weed control, stock and/or feral pest mammal control, and the application of specific superimposed treatments, such as irrigation. The costs of all maintenance activities are being recorded, so the longer-term economic cost of the range of restoration approaches can be compared over time. This is especially relevant at one site, Te Muri, where the per hectare setup cost was varied by planting seedlings at different densities.
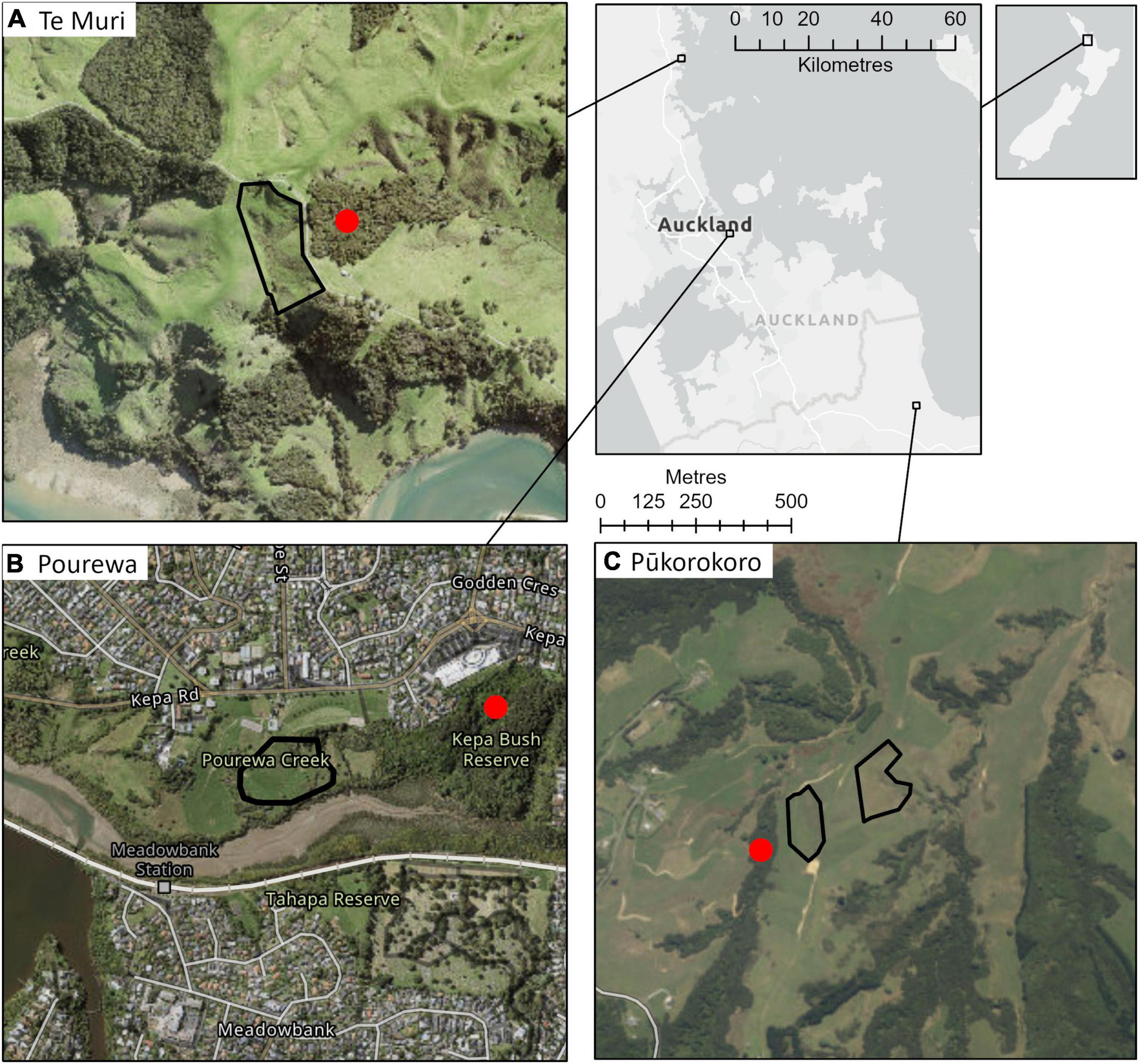
Figure 1. AUT Living Laboratories program forest restoration experiment site locations within the context of the greater Auckland Region, and within Aotearoa New Zealand. The sites are: (A) Te Muri (3.8 ha), (B) Pourewa (2.1 ha), and (C) Pūkorokoro (3.2 ha). The red dots indicate adjacent reference sites, which will be monitored for a subset of ecosystem functions (Table 3) at a similar temporal frequency to the experimental sites to enable comparisons.
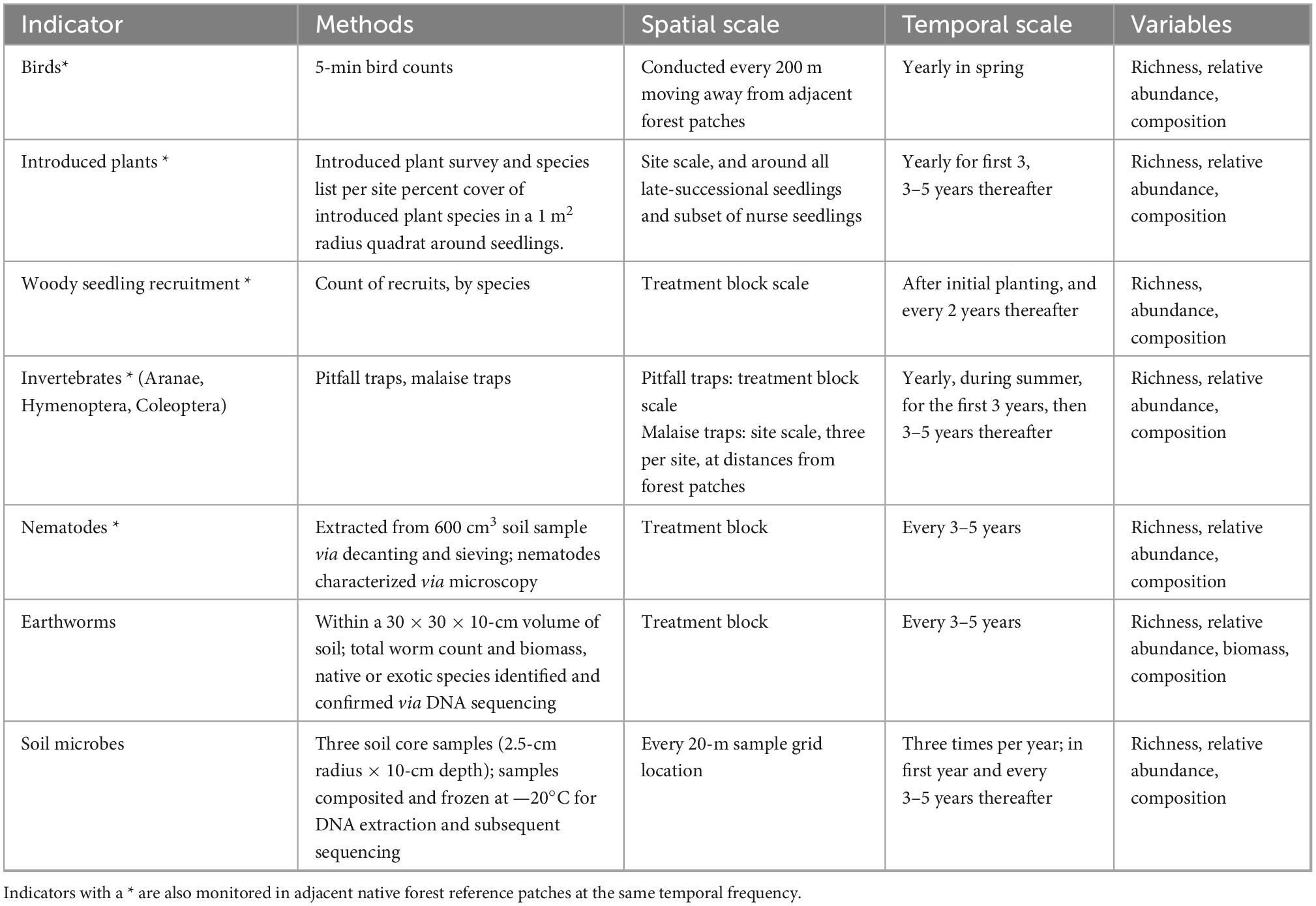
Table 3. Biodiversity indicator monitoring design and methodology for the AUT Living Laboratories program experimental sites.
Experimental site 1. Te Muri
This is a 3.8-ha site situated within Te Muri Regional Park (Figure 1A); Ngāti Manuhiri is mana whenua (ancestral people) in this area. In pre-human times, this land was covered by kauri (Agathis australis)—podocarp-broadleaved forest (Singers et al., 2017). Since human arrival and subsequent forest clearance (e.g., Wardle, 1991), the area was used for farming beef and cattle, until 2019, when the area was retired from grazing. Today, small patches of native forest surround the experimental site, including some representation of the original forest type, and other modified mixed-broadleaved native forest types that reflect different levels of human modification and disturbance. The establishment of the Te Muri site, in the winter of 2020, followed protocols commonly used across Aotearoa New Zealand by restoration groups and local and regional government councils. These methods use weed spraying in advance of planting and during seedling establishment; a small area within the site was left unsprayed as a comparison; herbicide spraying in this region is particularly useful for killing kikuyu (Cenchrus clandestinus), a highly competitive invasive grass. The experimental site is situated in a small upland gully characterized by several ephemeral stream channels draining into a sedge-dominated wetland area in the lower parts of the gully. The main experimental effects at this site (Figure 2) varying across treatment blocks are: (1) the identity of the late-successional tree species, (2) the spatial pattern of the late-successional plantings, (3) the density of seedlings in the restoration planting, and (4) the impact of summer irrigation on survival and growth of planted seedlings (Table 1). The several small, regenerating native forest patches nearby are also being monitored as reference sites.
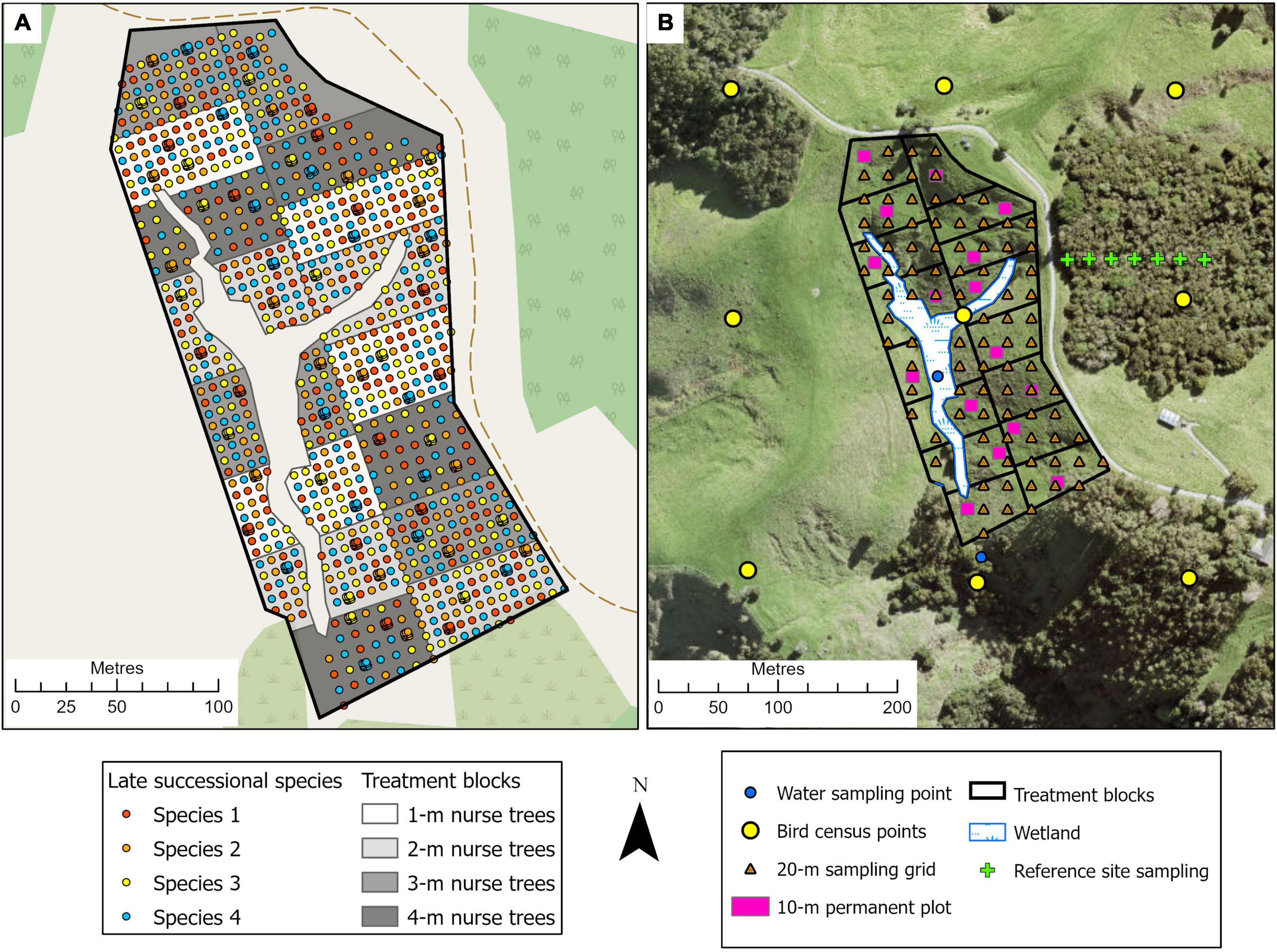
Figure 2. Illustration of the tree planting, treatment block, and site monitoring design for the Te Muri experimental site. (A) The treatments involve mixed-species nurse trees planted at four densities; across the site, four late-successional canopy species are planted singly, and in clusters of nine seedlings 1-m apart, in a randomized design (see Tables 1, 2). Survival and performance of both target and nurse tree species are monitored through time. (B) Monitoring design, at the landscape scale (e.g., bird observations, water quality), at the treatment block scale, within 10 × 10-m permanent plots (e.g., decomposition, natural seedling recruitment), and at the 20-m sampling grid scale (e.g., soil chemistry, soil microbes).
Experimental site 2. Pourewa
This 2.1-ha site (Figure 1B) is located on a south-facing slope of Pourewa Valley in the middle of the City of Auckland (McArthur, 2017). Prior to human settlement, and similar to the Te Muri site, the vegetation of the area was comprised of kauri, podocarp, and broadleaved forest (Singers et al., 2017); the area was subsequently cleared of its original vegetation after the settlement of Māori (the Ngāti Whātua Orākei hapū/sub-tribe) around the mid-1300s, and then colonial Europeans from the 1600s. By the 1950s, the land on which this experimental site sits had been confiscated from iwi (tribal) ownership by the New Zealand government and used for farmland, eventually becoming a horse-riding club for c. 30 years. In 2017, an extensive area of the Pourewa Valley was returned to local iwi and is currently undergoing both development and ecological restoration, the latter including the AUT Living Laboratories Pourewa experimental site. The initial plantings at this site were undertaken in 2019 but were greatly affected by a severe drought in the summer of 2019–2020 (Figure 3); thus, further plantings were carried out in 2020 and completed in 2021. Adjacent to this site is a 13.6-ha council-managed reserve, Kepa Bush Reserve, the largest patch of native forest (coastal broadleaved forest) on the densely urbanized central Auckland isthmus; this reserve is also being monitored as a reference site (see Table 3 for monitored indicators). The main experimental effects at the Pourewa site (Figure 4) varying across treatment blocks are: (1) the identity of the late-successional tree species, (2) the spatial pattern of the late-successional plantings, (3) the provision of food for birds via the nurse species composition, and (4) the distance from a nearby forest patch (Table 1).
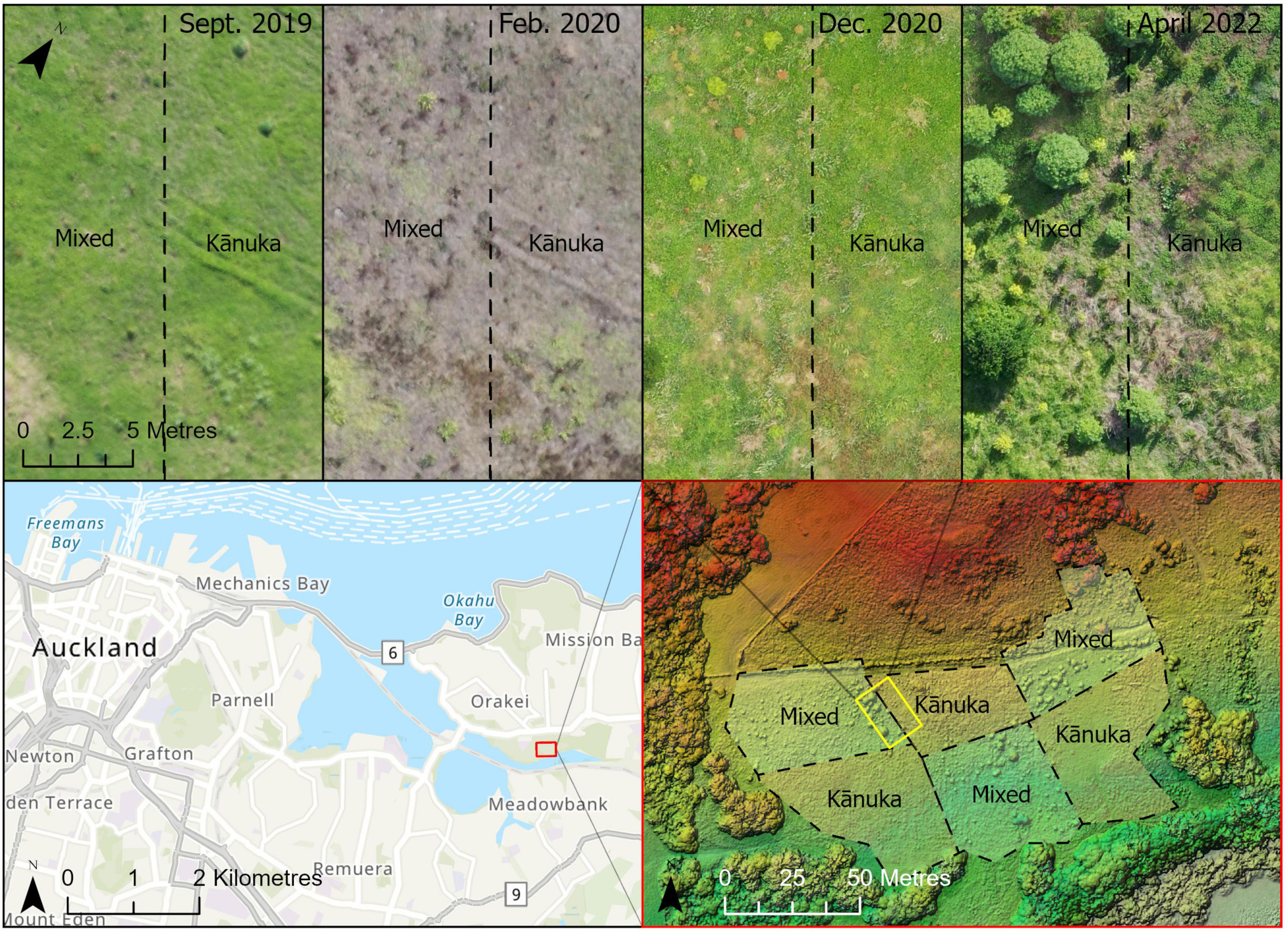
Figure 3. An illustration, for the Pourewa experimental site, of the use of UAV (drone) captured imagery for site monitoring. In the (top) half of the figure, spatio-temporal variation in weedy vegetation (e.g., grass cover), drought effects (e.g., Feb 2020), and seedling growth can be tracked in different treatment blocks; note that seedlings have become visible in the 2022 imagery. The (bottom right panel) illustrates how photogrammetric techniques can be applied to the images to produce digital surface models (DSMs), capturing variation in topography, and in the taller vegetation where it exists, across the sites.
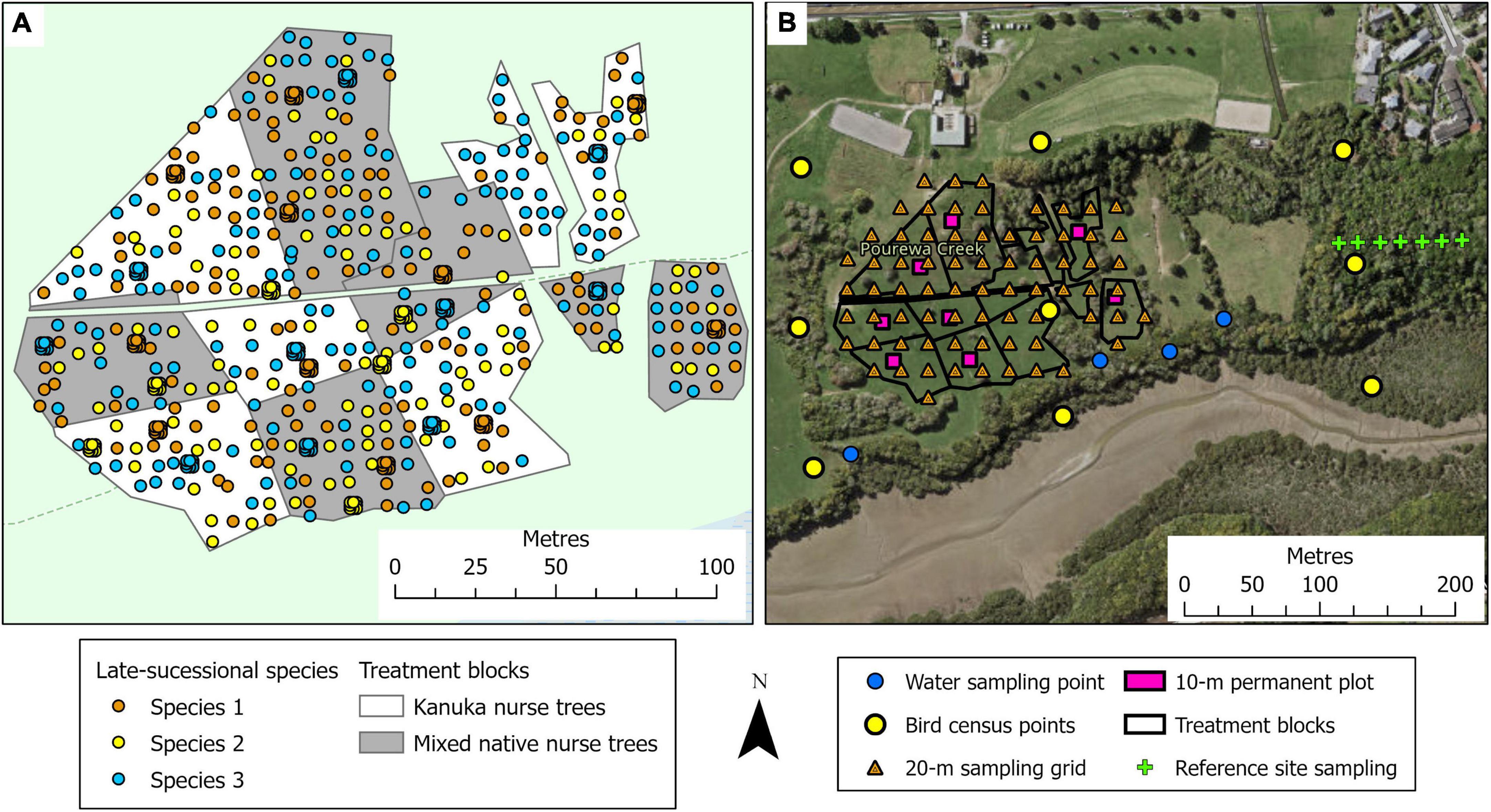
Figure 4. Illustration of the tree planting, treatment block, and site monitoring design for the Pourewa experimental site. (A) The treatments involve two types of nurse tree compositions: kānuka and mixed-native species, all planted at a 1.5-m spacing; across the site, late-successional canopy species are planted singly at a 6-m spacing, and in clusters of nine seedlings 1-m apart, in a randomized design (see Tables 1, 2). Survival and performance of both target and nurse tree species are monitored through time. (B) Monitoring design, at the landscape scale (e.g., bird observations, water quality), at the treatment block scale, within 10 × 10-m permanent plots (e.g., decomposition, natural seedling recruitment), and at the 20-m sampling grid scale (e.g., soil chemistry, soil microbes).
Experimental site 3. Pūkorokoro
This 3.2-ha site, located in the northeast of the Waikato region in the North Island of New Zealand, adjacent to the Auckland region, is on a privately owned, steep, coastal property (Figure 1C). In pre-human times, this area would have been covered in coastal forest communities. It is currently primarily managed as a dairy farm. Similar to the Te Muri site, the restoration experimental planting in winter 2022 at the Pūkorokoro site followed commonly used restoration techniques, including weed spraying and fencing off the site from livestock. The main experimental effects (Figure 5) at this site varying across treatment blocks are: (1) the identity of the late-successional tree species, (2) the drought tolerance of nurse species, (3) the stature of nurse species, (4) aspect, (5) pot sizes of nursery stock, and (6) seedling initial heights from nursery stock (Table 1).
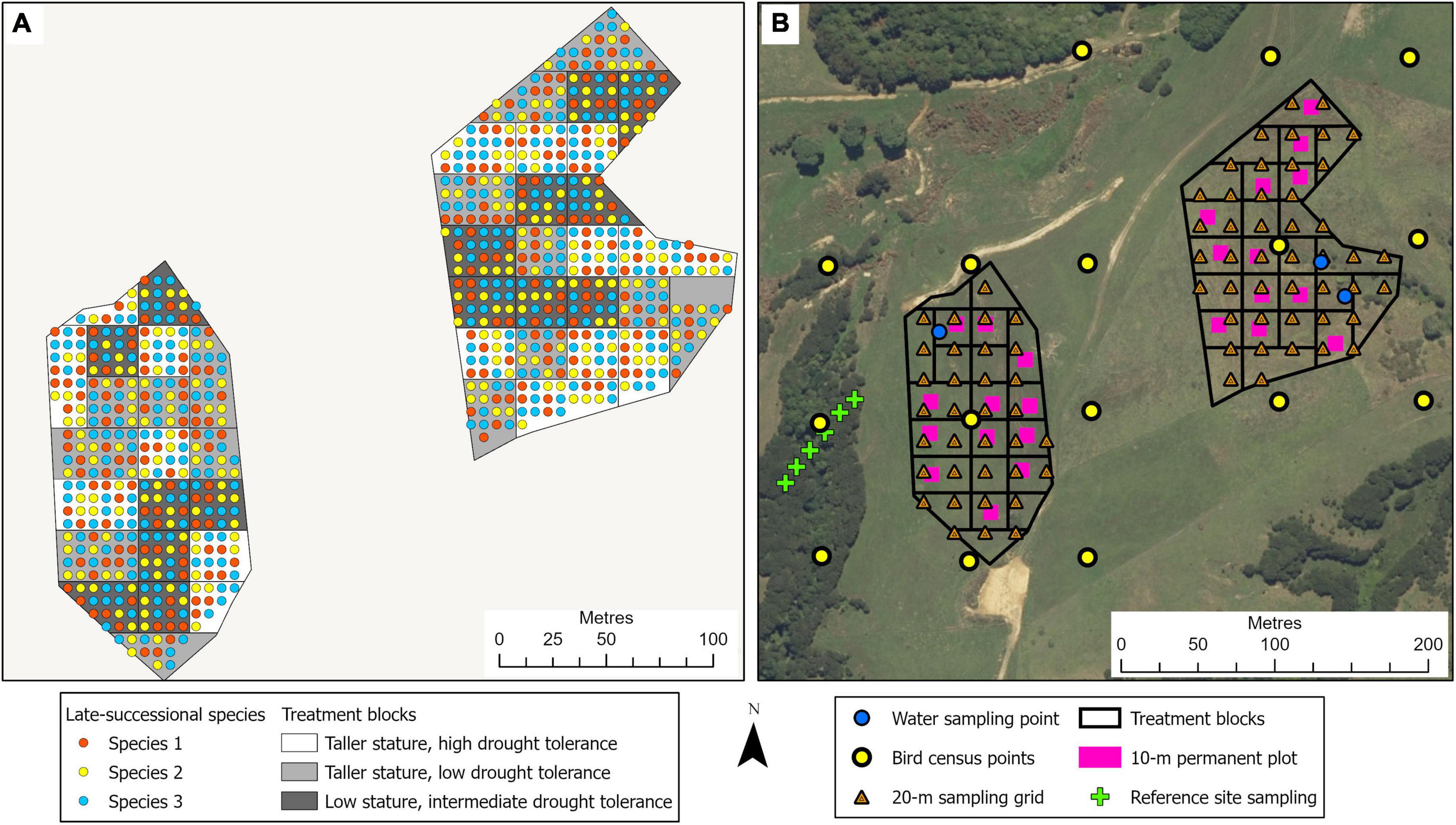
Figure 5. Illustration of the tree planting, treatment block, and site monitoring design for the Pūkorokoro experimental site. (A) The treatments involve three mixtures of native nurse tree species, comprising differences in stature and drought tolerance, planted at 1.5-m spacing; across the site, four late-successional canopy species are planted singly at a 6-m spacing, in a randomized design (see Tables 1, 2). Survival and performance of both target and nurse tree species are monitored through time. (B) Monitoring design, at the landscape scale (e.g., bird observations, water quality), at the treatment block scale, within 10 × 10-m permanent plots (e.g., decomposition, natural seedling recruitment), and at the 20-m sampling grid scale (e.g., soil chemistry, soil microbes).
One of the main long-term research aims at the three sites is to enable the embedding of additional “sub-experiments.” Such sub-experiments might, for example, comprise student research projects, involve visiting researchers, or simply be undertaken to test specific hypotheses that emerge as the restoration sites mature. For instance, we are planning to superimpose three sub-experiments onto the basic experimental design at two of the sites that are testing: (1) the efficacy of using native ferns as ground cover to facilitate weed control and natural seedling recruitment; (2) the relative performance of drought- and non-drought-hardened native seedlings planted into the sites; and (3) the performance of small subsets of the planted seedlings under two different spring/summer irrigation regimes.
Baseline measurements and ongoing monitoring
Ecological monitoring protocols have been established at the three experimental sites, to gather data to test the temporal effects of experimental forest restoration treatments on biodiversity and ecosystem functioning (Tables 3, 4). The protocols are designed to: (1) Track the establishment and growth of all planted old-growth trees, and a representative subsample of nurse trees, and determine the possible drivers of their survival and performance at the tree scale and within the context of the experimental treatment blocks; and (2) Measure and monitor key indicators of above- and below-ground ecosystem function and dynamics occurring with forest development on these sites, and in the context of the experimental treatments.
The initial baseline measurements are “targeted monitoring” (sensu Sparrow et al., 2020) of each site. These measurements provide intensive pre-experiment data against which site changes, planned as ongoing “surveillance monitoring” (sensu Sparrow et al., 2020), can be tracked at each site. Facets of biodiversity and ecosystem functioning that are being monitored within the experiment include: the planted trees, introduced plants, native plant regeneration, birds, terrestrial invertebrates, seed dispersal, litter and debris, soil microbes, soil invertebrates, soil biological activity, decomposition, soil physicochemistry, and water chemistry. For the monitored biodiversity components, we will compute a range of biodiversity indices that can be used to characterize, compare, and contrast the overall biodiversity quality (sensu Feest et al., 2010) within among sites through time. Regularly captured UAV (drone)-based aerial imagery enables the creation of very high resolution 2–2.5-cm pixel resolution) “orthomosaic” snapshots (mosaicked imagery corrected for topography) of the experimental sites. Monitoring data are geographically positioned within the context of the UAV imagery to a high level of accuracy (± 1 m). All data generated by the project are to be open access. We actively seek research collaborations to join the project by adding sub-experiments or additional biodiversity and ecosystem monitoring.
The spatial and temporal scale at which each ecosystem indicator is monitored within our protocols accounts for several factors, including the expected scale of spatiotemporal variation, the costs of field sampling or lab processing, and the logistical practicality of continuing a particular sampling rigor and frequency 20 or more years into the future. As a result, the design includes data collection at three different spatial scales (e.g., Figure 2): (i) within a single permanent, 10 × 10-m plot positioned within each experimental treatment area using randomly generated coordinates (hereafter, “treatment block” monitoring) (ii) on a regular, 20 × 20-m sampling grid overlaid across the entire site, or (iii) at a site scale, or at the scale of comparison between the experimental site and an adjacent reference site. For example, decomposition and soil invertebrate activity are monitored at the treatment block scale because we wish to understand how these taxa respond to the different experimental treatments. In contrast, monitoring the variability in physicochemical properties of soils at fine spatial scales is costly and logistically infeasible; thus, here we monitor soil properties on a 20 × 20-m sampling grid scale and interpolate these quantities into spatially continuous GIS layers, consistent with other plot-based, long-term forest ecology approaches (e.g., Baldeck et al., 2013). Temporally, monitoring is to be repeated according to the expected temporal scale of variation. Sampling is conducted to track seasonal variation, e.g., bird communities (summer, winter), initial short-term changes, e.g., seedling establishment (annually), and changes that occur over longer time scales, e.g., tree growth (every 3–5 years).
Tree survival and performance monitoring
Baseline data for the late-successional trees are aimed at quantifying the survival probabilities of the different species across the three sites in relation to the initial starting condition of each tree, including: (1) seedling height and basal diameter, (2) the amount of dieback within 1 year of planting (from any cause, but most likely from drought or damage at planting), and (3) whether or not it has resprouted (yes/no) from the previous dieback within 1–2 years of planting. All late-successional seedlings are individually tagged, GPS-positioned, and measured annually for the first 3 years, followed by a 3–5-year monitoring cycle.
These measurements will be used to calculate woody biomass accumulation and, therefore, carbon sequestration rates for each species at each site and treatment. These measurements can be compared to environmental variables recorded at sites, such as soil moisture and physicochemical properties. Because all late-successional trees will be individually monitored and spatially referenced, we will be able to analyze individual tree establishment success and performance over time, in relation to continuous environmental variables and categorical treatment effects (e.g., nurse tree spacing or composition), using more sophisticated modeling methods such as mixed-effects regression analysis or spatially explicit growth and competition models (e.g., Uriarte et al., 2004). Thus, the granularity of data analysis for tree growth/survival will be at the tree scale, precluding the need to summarize and analyze the data at the treatment block level using more standard ANOVA-based designs, thereby increasing statistical power (Gotelli and Ellison, 2013) and the ability to disentangle microhabitat effects.
Because the numbers of nurse species trees are much greater than that of the late-successional species, monitoring of the former at the individual tree level is on a stratified subsample that represents all species, experimental treatments, and replicates. Protocols match those used for the late-successional trees, including permanently tagging individuals so they can be tracked through time. The selection of nurse trees is centered on the late-successional trees; at every fourth late-successional tree, the rapid and easy-to-conduct Point-Centered Quarter (PCQ) method (e.g., Wainscott, 2015) is used to identify the nearest four nurse trees, which are then permanently marked. Growth data for these trees will be related to that for late-successional trees to assess fine-scale ecological interactions and the effects of environmental factors.
In Aotearoa New Zealand, non-native plant species, including many invasive weeds, are numerous and known to have detrimental effects on native ecosystems (Lovegrove et al., 2002) and their restoration (Norton, 2009). For example, “kikuyu” (Cenchrus clandestinus) is a grass invasive in northern New Zealand that can often dominate restoration sites in early phases prior to canopy closure, forming thick swards that can have a range of impacts on seedlings and young trees (Forbes and Craig, 2013). The presence of non-native plant species is monitored at a site level by compiling a list of all introduced plant species on an annual basis; this ensures novel invasive species can be controlled at sites before they become well-established. At a finer scale, the effects of introduced plants on native plant establishment, survival, and ongoing growth are monitored by recording the presence and cover of introduced plant species in 1-m2-radius quadrats around all late-successional seedlings and the monitored subset of nurse seedlings.
Monitoring at the treatment block scale
Natural plant regeneration
Native and non-native woody plants will colonize and establish within the experimental areas over time, depending on the distance from nearby seed sources. Once the planted seedlings are well established at each site (after 2 years), the number, and identity of, naturally regenerating woody seedlings will be recorded within 2 × 2-m sub-plots established at the center and four corners of 10 × 10-m treatment block plots. As the restoration sites age, and both the planted and naturally regenerating seedlings get larger, monitoring will include counts within the 10 × 10-m plots. These data will be related to the wider species pool available at adjacent reference sites (see “Monitoring of adjacent forest patches” section, below) and that disperse to the site (see “Bird monitoring” section, below), to help understand the potential for native and non-native plant species establishment in association with restoration, in relation to distance from seed sources.
Ground-active invertebrates
Invertebrates are important indicators of ecosystem condition (Green, 2000; Gerlach et al., 2013) and changes in condition through time (Borges et al., 2021). Monitoring ground-active invertebrate communities by targeted trapping of key indicator taxa, such as carabid beetles (Carabidae) and spiders (Aranae), can be used to assess biodiversity, habitat quality, and ecosystem function in space and over time (Ward and Larivière, 2004; Case et al., 2020; Montgomery et al., 2021). At all three AUT Living Laboratories sites, pitfall trapping is carried out at the treatment unit scale, within the permanent 10 × 10-m plots, following standard protocols for environmental monitoring in Aotearoa New Zealand (Sherley and Stringer, 2016).
Soil invertebrates
Earthworms and nematodes are recognized indicators of soil quality and ecosystem condition (Blair et al., 1997; Pulleman et al., 2012). Earthworms, as eco-engineers, contribute to nutrient cycling and soil formation (Van Groenigen et al., 2014) and earthworm community composition generally reflects ecosystem condition and the level of human modification (e.g., Paoletti, 1999; Fusaro et al., 2018). Likewise, Nematoda play a key ecological role in soil nutrient cycling, are sensitive to human-caused disturbance, and their community structure therefore reflects aspects of soil condition (Lu et al., 2020). Within the AUT Living Laboratories experimental sites, earthworms and nematodes are sampled within each permanent, 10 × 10-m plots associated with each experimental treatment unit (e.g., Figure 2). All earthworms are extracted from a 25-cm3 soil sample excavated at a random distance (cm) of up to 5 m and direction (compass bearing) from the plot center; this method ensures that the same sample area is not excavated more than once. For each sample, the number of individual earthworms is recorded and total earthworm biomass is measured; all earthworms are then stored in ethanol for subsequent DNA barcoding to determine taxon identities. For nematode sampling, an aggregate of 500 grams of soil will be taken from five random samples up to 5-m from the earthworm sample locations. For each sample, nematodes will be extracted using decanting and sieving methods following standard protocols (Hallmann and Subbotin, 2018); using microscopy, nematode morphotypes and their relative abundances will be estimated, and samples stored for potential later DNA-based community analyses (e.g., Bogale et al., 2020).
Litter layer, organic layer, and fine woody debris
The amount and types of litter and woody debris produced by tree species within the experimental sites will increase as the restoration plantings develop and mature (e.g., Grant et al., 2007). Litter and organic layers are formed, and woody debris of different sizes accumulate, as trees form into a more mature, closed canopy forest (Facelli and Pickett, 1991). The depths of the litter and organic layers, and the relative biomass contributions from litter, organic soil, and woody debris, are indicative therefore of forest ecosystem development and of the rate of nutrient and carbon cycling within treatment zones and can impact on the biodiversity of local, ground-dwelling organisms (Rondeux and Sanchez, 2010). Litter, organic layer soil, and woody debris will be sampled at small subplots within the 10 × 10-m permanent sample plots within treatment zones. Sampling of litter and organic matter involves excavating these from one, 50 × 50-cm area randomly located within the permanent plot, recording the depth of each, and determining the relative biomass via drying and weighing. Fine woody debris (<5 cm diameter) will be collected from within one of the five, 2 × 2-m subplots and subsequently weighed to calculate a mass per unit area. Fine litter and organic matter will be first surveyed 2 years post-planting and every 2 years thereafter.
Soil biological activity
The “bait lamina probe” method is used as an inexpensive and easy to use test for evaluating relative biological activity in the top 5–10 cm of the soil and at the soil surface (e.g., Welsch et al., 2019). The method quantifies the amount of bait substrate, pre-inserted into holes on a plastic probe partially buried in the soil, that has been consumed by soil macrofauna after a given time period. Four probes are buried in the top of the soil, with one bait hole showing above the surface, in each of the five, 2 × 2 m sub-plots located within the 10 × 10-m permanent sample plots associated with treatment blocks. After 90 days, the probes are excavated, and the relative number of holes consumed on each probe provides an index of soil biological activity over that period; this method will be repeated annually for the first 2 years and every 3–5 years thereafter.
Decomposition
Organic matter decomposition plays a critical role in the flow of energy and matter in an ecosystem and is therefore a central component of carbon and nutrient cycling processes. The rate of decomposition (carbon turnover) impacts on organic carbon accumulation in the upper soil layers through time and, as a monitored indicator, reflects changes in litter accumulation and quality, local environmental conditions (e.g., moisture and temperature), and in the decomposer faunal communities as the forest restoration progresses (Ehrenfeld and Toth, 1997). The “tea bag index (TBI)” experimental decomposition method (Keuskamp et al., 2013) is used here to quantify the rate of soil organic matter decomposition at the AUT Living Laboratories experimental restoration sites. Within the 10 × 10-m permanent treatment block plots, five pairs of green and rooibos tea bags are buried at an 8-cm soil depth, one pair in each 2 × 2-m sub-plot, for 30 days. Following the standard method, values for k (decomposition rate) and S (stability) are calculated using the five replicate samples per treatment block (e.g., Welsch et al., 2019). This bioassay will be repeated at a temporal frequency of every 3–5 years.
Monitoring at the 20 × 20-m, regular grid scale
Soil physicochemical properties
Mineral soil physical and chemical properties are measured for sample points on a 20-m grid across the three experimental sites. Soil properties provide critical information on soil fertility and condition. Monitoring these properties through time provides quantitative data that can be used to track changes in soil quality as the restored forest develops (Gatica-Saavedra et al., 2017). Soil physical property measurements comprise soil compaction, bulk density, and soil moisture, while chemical property measurements comprise soil pH, total carbon and nitrogen, organic carbon, mineralizable nitrogen, Olsen phosphorous, calcium, magnesium, sodium, cation exchange capacity, and total base saturation. Soil compaction is measured using a soil penetrometer (Eijkelkamp Hand Penetrometer) which measures the relative pressure required to force a probe down through the soil at different depth increments. Soil moisture is collected using a handheld soil moisture probe (Campbell Scientific Hydrosense II). Soil bulk density is measured using a copper cup of a known volume to sample soil from the top 10 cm of the mineral soil which is then dried and weighed. The collection, storage, and analyses for soil chemistry follow standard procedures (e.g., Carter and Gregorich, 2008); all chemical analyses are carried out by a certified laboratory. The temporal intensity of sampling for the different physical and chemical properties is every 3–5 years.
Soil biota
Extraction and sequencing of genetic material from belowground samples can be used for monitoring changes in the belowground biotic components of ecosystems over a range of timescales (Mathieu et al., 2020). Soil samples can be used in a range of ways: (1) targeted sequencing of certain taxa, such as microbial communities, comprising fungi, archaea, and bacteria, can be used to monitor soil health (Hermans et al., 2017; Astudillo-García et al., 2019), (2) metagenomic sequencing can be used to quantify the functional potential of soil biota (Hermans et al., 2020), and (3) comparisons of environmental DNA (eDNA) can be used to monitor the diversity of bacteria, archaea, fungi, slime molds, and other soil organisms over time (Lear et al., 2018). At all three of the AUT Living Laboratories sites, 10-cm soil cores have been collected at all soil sample points (20 × 20-m grid) and frozen at –20°C as baseline samples for temporal comparison. These will be repeated seasonally (late summer, winter, and spring) every 3–5 years. Root tip sampling can be used to monitor fungal communities associated with the roots of vascular plants; ectomycorrhizal fungal communities are relatively easy to sample and are crucial in plant growth and survival. Thus, in addition to soil samples, root tip sampling of the only ectomycorrhizal plant added to all three experimental sites, kānuka (Kunzea spp.), will be undertaken to monitor the colonization of the restoration plantings and changes in belowground biodiversity over time. Root tips will be excavated and samples removed for molecular analysis every 3–5 years, following protocols recommended for sampling ectomycorrhizal fungi, e.g., Gehring et al. (1998). These samples will be subjected to molecular analysis to identify the symbiotic fungal taxa (Martin, 2007; Janowski et al., 2019).
Monitoring at the site-to-landscape scale
Birds
Native and non-native birds disperse seed, contribute to food web interactions and other ecosystem functions, and are an important component of biodiversity (García et al., 2014; Coux et al., 2021). Bird communities at the experimental sites are monitored seasonally using standard 5-min bird count protocols (e.g., Bibby et al., 2000; Hartley and Greene, 2012), which have been used historically, including at Pourewa, where comparisons over time and with nearby sites can be made. These protocols are consistent with those applied by government agencies and thus will be comparable to monitoring data for the region (e.g., Elliott et al., 2010). The protocol involves recording the identities and number of birds heard and seen over 5 min at monitoring points at least 200 m apart. These data will be used to track changes in bird biodiversity, such as abundance, number of native species, and number of exotic species over time, as the plantings mature. In addition, artificial perches with seed traps will be established at each site and seed rain monitored periodically to understand the role of birds in native seedling recruitment and introduced plant invasion at each site. Animal ethics approval will be sought prior to undertaking this monitoring.
Flying invertebrates
Malaise trapping (Matthews and Matthews, 2017) is useful for monitoring the presence of key flying invertebrates, such as Hymenoptera, including bees and wasps. Three Malaise traps will be deployed at each site annually for several weeks during mid- to late-summer within the nearest woody vegetation patch and within the planted area at two different distances from the nearby woody patch. Standard New Zealand protocols will be used (Sherley and Evans, 2016) and the three samples will be used to assess differences in native invertebrate biodiversity between the restoration planting and nearby woody vegetation. Depending on the number of taxa present, identifications of recognizable taxonomic units (RTUs) to species level may be limited to a set of focal taxa that are tracked through time.
Water chemistry
Portions of the three experimental sites each drain into at least one intermittently flowing stream or area of standing water (e.g., wetland; e.g., Figure 2). Changes in biotic, chemical, and physical constituents of these water bodies through time can be indicative of ecosystem changes occurring uphill as the new forest patches develop. We follow a standard protocol used for water quality monitoring by community groups, government bodies, and land managers across New Zealand (the “Wai Care” protocol2) using a suite of standard measures of water quality, including pH, water chemistry, dissolved oxygen, turbidity, and macroinvertebrate indicators. The temporal frequency of sampling is three times per year, every 2 years.
Drone/UAV imagery
Color UAV imagery (2–5-cm pixel resolution) covering each experimental site is collected three times per year. Flights are conducted as automated 3D waypoint surveys using UAV flight planning and control software (UgCS 4.6, SPH Engineering) providing repeatable spatial coverage and detail. Overlapping UAV imagery provides opportunities for generating a “dense point cloud” dataset using structure-from-motion methods (Smith et al., 2016) that can be further processed to create GIS-ready RGB orthomosaics along with high-resolution digital elevation models (DEM–elevation at the ground surface) and a digital surface models (DSM—elevation at top of vegetation) at for each image collection period. These data are very useful for tracking topographic variation and vegetation structural changes through time (e.g., Figure 3). Due to the very high resolution of the data, the composition and spatial distribution of the native and exotic herbaceous and woody vegetation can be classified and tracked, providing a way to remotely monitor the planted trees, once they reach an adequate size (3–4 years old).
Monitoring of adjacent forest reference patches
At each location, adjacent forest patches (Figure 1—red dots) will be monitored for biodiversity and ecosystem functioning in comparable ways to establish reference, unmanipulated baseline data. This will be especially useful for temporal comparisons, allowing spatial and temporal variation to be teased apart. The focus initially on these locations is on basic biodiversity data including plant species composition, soil and water quality sampling, and terrestrial invertebrate surveys (Table 3). In each reference patch, these variables will be monitored every 20 m along a transect moving inward from the patch edge nearest the experimental site to the patch center (e.g., Figure 2B). Measurements will be conducted at the same temporal frequency as for the experimental site sampling.
Partnerships
A foundational aim of the AUT Living Laboratories program experiments was co-design of the research with mana whenua (Indigenous peoples in Aotearoa New Zealand, with tribal authority over a region). Good kāwanatanga (governance) of research should enhance relationships and promote cooperation and power sharing in a relationship of equals, co-designing, and co-determining opportunities of which their impact meets the needs of mana whenua. The goal was to enable a sense of control and protection throughout all parts of the program—from experimental design to decisions around data collection and control of access. There are a range of both international and domestic frameworks regarding the rights of Māori as partners in scientific research from the United Nations Declaration on the Rights of Indigenous peoples to Indigenous Data Sovereignty agenda. However, first and foremost, as Te Tiriti o Waitangi (Healy et al., 2012) partners with mana whenua, partnership must enable rangatiratanga (sovereignty) and mana motuhake (self-determination) and offer possibilities for mutually defined success (Kukutai and Taylor, 2016).
The approaches to partnerships varied at the different experimental sites. The Pourewa project was initiated directly with Ngāti Whātua o Orākei through a prior invitation to collaborate. Planning for this project emerged out of a series of hui (meetings). The AUT Livings Laboratories program successfully entered into a collaboration agreement with Ngāti Whātua o Orākei where clear guidelines were agreed upon detailing the nature of the relationship. A significant part of the agreement was acknowledging Ngāti Whātua mātauranga-a-iwi (regional-specific mātauranga Māori) as equal to conventional science and ensuring that any activity on the Pourewa site is conducted with appropriate tikanga (protocols) in accordance with Ngāti Whātua protocols and a plan was made regarding the sharing of any mātauranga that would emerge from this project. While discussions for this project resulted in the decision that information could be shared openly at this site, this is not a given at all potential sites, and, in general, researchers should be cognizant of ongoing conversations and developments regarding best practice for collection and governance of any and all Indigenous data (e.g., CARE principles3). Such conversations in this case were facilitated by regular meetings with iwi (tribe) environmental managers, the appointment of a kaumatua (respected elder) from the organization representing Ngāti Whātua o Orākei, and a commitment within the science team to align the program’s outcomes with tribal aspirations. Working in such a way also provided the opportunity for AUT Living Laboratories researchers to engage with Ngāti Whātua o Orākei giving them rich exposure and experience to their reo (language) and tikanga (protocols), and cultural nuances and histories of that particular region. In a relationship of equals where utu (reciprocity) enables the upholding of each partner’s mana (authority), the benefits then become two-way, thus, developing capability and building capacity in research for Ngāti Whātua o Orākei and the AUT Living Laboratories program team. For example, in establishing the experiment at this site, we integrated iwi (tribal) requirements regarding the selection of species deemed ecologically and culturally appropriate for the location and followed protocols for how soil and vegetation were to be treated, including not applying any chemical herbicides at the site.
The Te Muri project emerged out of negotiations with Auckland Council who selected the experimental site after a process of identifying viable options on council land. Ngāti Manuhiri has ancestral links to the Te Muri site and Auckland Council has a co-governance plan for that area. However, because Auckland Council acted as an intermediary, the establishment of a direct partnership between AUT and Ngāti Manuhiri was slower and suffered from miscommunication. The third site, Pūkorokoro, involves a partnership with a tauiwi (non-Māori) organization, Te Whangai Trust, as well as a formal agreement Ngāti Pāoa which has mana whenua in that area. Currently, work is still underway to establish formal collaboration agreements with mana whenua for these two sites.
Good partnership aims to restore the balance between Māori and the Crown. Wherever possible, researchers should directly co-design research with mana whenua to give communities a sense of control and protection throughout the entire process. For example, in this program, the question of data sovereignty is dependent on the partnership agreement; the agreement is for open access in the case of Ngāti Whātua o Orākei. Adequate time, care, and resources need to be assigned to ensure projects remain culturally safe, the research uncompromised, and the mana (power and authority) of iwi (tribes) is protected and enhanced. From a partnerships perspective, direct engagement with Māori organizations has led to more meaningful and successful relationships and outcomes within this research program, because a reliance on third-party exposes the project to risks of mismanagement, especially when co-governance arrangements are not adequately instantiated.
Relevance for NbS policy and planning
Aotearoa New Zealand was the second country in the world to implement an emissions trading scheme (in 2008), and the first to include forestry in its uniquely broad sectoral coverage (Leining et al., 2019). The objective of the NZ ETS, as an incentivization instrument, was to produce the least-cost emissions reductions through the exchange of emission units (NZUs) that represent a “right to emit” one ton of carbon dioxide equivalents. The NZU price produces a disincentive for emitters who must surrender units equivalent to their obligations, as well as an incentive for forest owners who are the only non-government suppliers of units (Leining, 2022). Under current settings, the government also supplies NZUs through regular auctions and free allocations to emissions-intensive trade-exposed businesses. In the early phases of the NZ ETS, the NZU price was low due to various design features; however, it has increased significantly due to successive reforms (Hall, 2020)—from as low as NZ$0.66 in secondary markets in May 2013 to an unprecedented peak of over NZ$85 in early 2022. There is evidence that afforestation is highly responsive to the ETS incentive; thus, as long as carbon prices remain high, the NZ ETS will likely continue to be a primary mechanism driving significant land-use change, largely involving the establishment of forests on farmland (Ministry for the Environment, 2020).
However, this outcome is not necessarily positive, neither in terms of climate change strategy nor other policy objectives, such as biodiversity. Central to this is the nature of the ETS incentive, which relates solely to carbon sequestration. The greater the sequestration rate, the larger the volume of units received, and therefore the greater the potential for revenue (depending on when the recipient sells the units). Thus, the ETS incentivizes fast-growing trees, with an opportunity cost for planting slower-growing species. Profitability is also increased if establishment costs are low. This has tended to favor monocultures of exotic Pinus radiata, which has the advantage of being fast growing in a range of settings, as well as cheap to establish due to the industrial scale of commercial forestry. This historical path-dependency is reinforced by carbon markets which favor the same attributes of rapid growth. This situation has led to critical misalignments in how plantation forestry and ecosystem restoration is treated by climate policy. First, there is a lack of policy coherence among the various land-related objectives that government is concerned with, especially between climate mitigation, climate adaptation, and biodiversity. Second and consequently, there is a risk of perverse impacts from climate policy, and in particular the potential for maladaptive outcomes due to the sole focus on carbon sequestration. Third, there are transition risks with respect to securing and sustaining the social license for land use change. Fourth, the policy mix is not comprehensive: there is insufficient support for biodiversity and landscape resilience which might counterbalance the incentives for climate mitigation, e.g., erosion control in extreme weather events. And finally, there is a significant reliance on exotic monocultures, which needs to be overcome to produce alternative outcomes.
From a short-term climate mitigation perspective, Pinus radiata and other exotic species such as redwoods (Sequoia sempervirens) and eucalypts (Eucalyptus spp.) clearly have utility. However, from a climate resilience perspective, large-scale monocultural forests are not necessarily optimal (Sacco et al., 2021), which may justify concerns regarding farm-scale conversions in New Zealand at scales of 100–1,000 s of hectares. In principle, forest resilience is positively correlated with biodiversity and ecosystem function, both in terms of species diversity and uneven-aged structures that promote ecological succession (Suryaningrum et al., 2021). Consequently, species diversity in forest ecosystems is generally seen as an adaptive strategy (Hisano et al., 2018; Höltermann, 2020), because even-aged monocultures are susceptible to catastrophic loss from pests and diseases (Liu et al., 2018). Climate mitigation policies that incentivize the latter are therefore at risk of maladaptive outcomes (Anderegg et al., 2020). In light of New Zealand’s unique geography and industry, further research is needed on the particular risks to exotic plantations in New Zealand (Macinnis-Ng et al., 2021), but uncertainty warrants a precautionary approach to future climate-related risks like windthrow and wildfire (Moore and Watt, 2015; Melia et al., 2022). For unharvested forests (otherwise known as carbon farming), these risks may increase if active forest management is not undertaken, which may occur if forest managers lack sufficient cash reserves when sequestration rates slow and cashflow diminishes. On the other hand, landowners who want to use native species for farmland revegetation activities face uncertain outcomes and returns on investment, largely due to major deficits in published ecological knowledge for New Zealand endemic tree species and their effective uses in agroecosystem contexts (Case and Ryan, 2020; but see Kimberley et al., 2021).
Although observational data are frequently used as a basis for modeling the outcomes of NbS (e.g., Hutchison et al., 2018; Chausson et al., 2020), long-term ecological experiments (e.g., Lindenmayer et al., 2012) are crucial for achieving a detailed understanding of the causal processes linking carbon, biodiversity and ecosystem functioning (e.g., Scherer-Lorenzen et al., 2007; Gottschall et al., 2022) associated with woody, non-production vegetation (Case et al., 2020). This is because large variability in both tree growth rates across environmental gradients and in the response of biodiversity and ecosystem functions to environmental management make outcomes unpredictable (e.g., Grossman et al., 2018; Yuan et al., 2020); in addition, most restoration activity is designed for achieving successful plant establishment, not for understanding the underlying ecological processes and functions such as nutrient cycling, interaction webs (pollination, predation, competition, dispersal), decomposition, carbon sequestration, and water cycling. Thus, in the context of supporting NbS planning and policy, long-term experimental data enable insights into how restored forest ecosystems are responding to ongoing climatic changes relative to key ecological, social, cultural, and economic NbS performance indicators (e.g., see Simelton et al., 2021). Such data might also, for example, inform the development of possible payment schemes for biodiversity value, generating a key leverage point for better integrated policy (Hall and Lindsay, 2021). Around the world, a variety of economic instruments are being developed and implemented with the aim of providing landowners a means to realize monetary benefits from creating and maintaining biodiverse ecosystem components on their farms (Brears, 2022). Improved understanding in Aotearoa New Zealand of how native species contribute to desirable ecological functions, especially ecological resilience, can therefore contribute to policy design.
The incentivization of exotic over native species through climate mitigation policy has implications for Māori, the Indigenous people of Aotearoa New Zealand. Historical deforestation and other types of land use change, including drainage of wetlands and peatlands, are inseparable from the injustices of colonization, in particular the alienation of Māori from ancestral land and associated loss of decision-making power (Koroi, 2021). This entails specific breaches of Te Tiriti o Waitangi (the Treaty of Waitangi), a key agreement made between the Crown and many Māori tribes in 1840, which promised to Māori “te tino rangatiratanga o o ratou wenua o ratou kainga me o ratou taonga katoa,” or “the full exclusive and undisturbed possession of their Lands and Estates Forests Fisheries and other properties” in the English version; a direct translation of the Māori version to English is “the unqualified exercise of their chieftainship over their lands, villages and all their treasures.” Consequently, the rehabilitation of native ecosystems can be seen as a complement to decolonization, as the exercise of an “ethic of restoration” (Jackson, 2020) that strives to address colonial injustices. Māori who have ancestral connections (mana whenua) to a particular location have relationships of mutual care for endemic species, which relates to the idea of whakapapa, the interconnectedness of things, including between human and non-human entities (Roberts, 2013). These relationships create a duty of care known as kaitiakitanga, commonly translated as guardianship or stewardship, but entails a more sophisticated sense of obligation and reciprocity (Kawharu, 2000). However, Māori perspectives vary regarding how to dispense these duties and the emphasis placed, for example, on use of natives and exotic species to achieve long-term objectives in different contexts or to meet varying socio-economic objectives (e.g., Wannan, 2022).
Thus, there is an urgent societal need to address the interlocking challenges of climate mitigation, adaptation and biodiversity loss. As such, inquiry-based research within the AUT Living Laboratories research program is conceived as post-normal science to improve the quality of knowledge production where “facts [are] uncertain, values in dispute, stakes high and decisions urgent” (Funtowicz and Ravetz, 1993). Because of the complexity of native ecosystems and the long timeframes for establishment, existing knowledge gaps cannot be filled within the timeframes in which policymakers must act, especially in advance of the 2030 Nationally Determined Contributions under the Paris Agreement. Consequently, the production of knowledge must operate in a post-normal mode: first, by working with an extended peer community which is open to Indigenous expertise (i.e., mātauranga Māori) and also lay knowledges of landowners (Meisch et al., 2022); and second, by acknowledging that appropriate experimental projects will be “out in the world” as manifestations of the climate- and nature-positive actions that they strive to inform. For example, the inclusion of an exotic species comparison in the research design would be typical of a normal experimental approach; however, such controls were not implemented because landowners did not want exotic tree species. Thus, through co-design of research sites, the environmental aspirations of landowners took priority over idealized research design. This reflects a post-normal approach to “living laboratories” which are embedded in complex, interconnected natural and human systems.
Conclusion
Nature-based Solutions are presented as “place-based partnerships between people and nature,” with the conservation and enhancement of biodiversity at its core (Seddon et al., 2021); the implementation of NbS is therefore necessarily local in scale, specific to the context and needs of a given region, people, and situation. Indeed, the adoption of NbS as a framework, particularly as a model for climate change mitigation and adaptation, has gained significant traction and momentum in the last 5 years (Seddon, 2022). One of the large attractions of frameworks such as that offered by NbS is that it espouses a holistic approach to addressing the twin crises of biodiversity and climate change, and is naturally aligned with the caretaking mindsets of local indigenous peoples toward their lands and waters (e.g., Reed et al., 2022). The AUT Living Laboratories program was established recognizing the need for such holistic thinking in a bicultural Aotearoa New Zealand, given that the country is experiencing an intersection of crises, debates, and challenges in land and water management; this program also recognizes that empirical evidence will be necessary to fill critical knowledge gaps via transdisciplinary research and to provide a way to legitimize NbS as a useful toolset for both understanding and mitigating the impacts of a worsening climate crisis (Seddon, 2022).
Specifically, the AUT Living Laboratories program has been designed as a long-term teaching and research resource, a source of practical knowledge for using the restoration of native vegetation to address societal challenges such as climate change mitigation and adaptation and biodiversity loss. In addition to understanding parameters for restoration success in local environments, we seek to address the lack of understanding of how ecological processes and functions change over time within restoration sites. These processes include nutrient cycling and soil health, soil erosion, biological interaction webs, connectivity and maintenance of native plant and animal populations, suppression or enhancement of weed and pest animal spread, changes in water quality (nutrient run off), and quantity (water capture). Thus, the experiments endeavor to optimize restoration protocols for biodiversity and ecosystem functioning and NbS outcomes, as referenced against existing indicator frameworks. Cross-disciplinary research within the program will ultimately aim to explore human-nature interactions including human wellbeing and their connections to land and biodiversity, Indigenous people’s empowerment, and economic advantage to landowners such as carbon credits and other long-term financial benefits.
In developing the scientific design and ecosystem measurement and monitoring protocols for the AUT Living Laboratories experiments, we have drawn on a range of local and global scientific evidence, examples, and resources. These include those provided by international intergovernmental bodies tasked with development of global biodiversity restoration science work programs and policies (e.g., the Intergovernmental Science-Policy Platform on Biodiversity and Ecosystem Services—IPBES). The restoration indicators used in our protocols have been aligned against those described in the Society for Ecological Restoration Australasia (SERA) guidelines for Australia (Standards Reference Group [SERA], 2021) and the Society for Ecological Restoration international principles and standards (McDonald et al., 2016). Indeed, we ensured adherence to the key principles of ecological restoration, including starting with strong partnerships, proceeded by incorporating relevant scientific and traditional knowledge, benchmarking against native forest reference systems, and the use of measurable indicators that reflect ecosystem recovery through time (Gann et al., 2019). We also aimed to achieve complementarity and a level of standardization, and uniqueness, compared with other forest restoration experiments being undertaken globally (e.g., the TreeDivNet experimental network4), particularly those aiming to investigate relationships between biodiversity and ecosystem function in agroecosystems (e.g., Perring et al., 2012; Schwarz et al., 2021).
In sum, our experimental design is intended as place-based and context-specific, to be guided by the knowledge demands of urgent public problems (Clarkson, 2022). By exploring how to establish legitimacy with landowners and local Māori communities, and by seeking to enhance public understanding of the values that native vegetation uniquely provides, the program creates post-normal “laboratories” that are open to the world, that are self-aware of their impacts on local ecosystems and people, and responsive to wider societal challenges.
Data availability statement
The original contributions presented in this study are included in the article, further inquiries can be directed to the corresponding authors.
Author contributions
HB and BC led the organization and wrote the manuscript. All authors contributed to writing the manuscript and approved the submitted version.
Funding
This work was funded by Auckland University of Technology and by the New Zealand Ministry for Primary Industries—Te Uru Rakau (TUR_1BT_2020_038). Both funders were not involved in the research design or the research itself.
Acknowledgments
We thank landowners and mana whenua contacts at the three sites for their land access permission, collaboration, and advice including Pourewa contacts Dane Tumahai, Rob Small, Tom Irvine, Jamie Cook, Wyatt Dooley, Marara van Buuren, and Mervyn Kerehoma (Ngāti Whātua Ôrākei). Te Muri contacts Nicola MacDonald, Mook Hohneck, and Delma O’Kane (Ngāti Manuhiri) and Jason Maguiness, Amy Waldmann, and Jared Anderson (Auckland Council); and Pūkorokoro contacts Glen Tupuhi (Ngāti Pāoa), Adrienne Dalton, Gary Dalton, Marc Dalton, and Nicola Pritchard (Te Whangai Trust). We also thank a large number of students and collaborators who have contributed to the project design, setup, monitoring, and sample processing: Adam Forbes, David Norton, Stacey Bryan, Paul Brierly, Shanta Budha-Magar, Kathrin Bolstad, Heather Braid, Feby Suryaningrum, Angus Keen, Riley Nolan, Benjamin Colin, Sarah Bishop, Evan Brown, Cate Ryan, Luke Liddell, Timothy Curran, Manjula Kularathna, Paul Michael (Fern Factor Ltd.), Cassie Newman, Talia Blewitt, Andrew Hart, Sydney Curtis-Wilson, Tom Subritzky, Lynda Burnside, Danielle Burling-Claridge, Chantoya Isaksson, Tarn Gillman, Mahinaarangi Marriner, Brittney Barnard, Claire Ellis, Cory Palmer, Ellis Nimick, Katherine Masters, Ross Silby, Gabriel Beattie (Plant More Trees Ltd.), Blake Worsfold (Rural Design), Jane Straka (Scrub), and Athira Sreeja (Te Whangai Trust). Other volunteers who have assisted with implementation and monitoring are Parnell Rotary Club, Ōrākei School, St Joseph’s School Ōrākei, Randwick Park School, INSPIRASI Indonesia Young Leaders Program, Rotary Science Challenge, St Chad’s Scout Group, Auckland Council staff, Auckland University of Technology Library staff, and all the Auckland University of Technology students who have volunteered. We thank two reviewers, whose comments have significantly improved this article.
Conflict of interest
The authors declare that the research was conducted in the absence of any commercial or financial relationships that could be construed as a potential conflict of interest.
Publisher’s note
All claims expressed in this article are solely those of the authors and do not necessarily represent those of their affiliated organizations, or those of the publisher, the editors and the reviewers. Any product that may be evaluated in this article, or claim that may be made by its manufacturer, is not guaranteed or endorsed by the publisher.
Footnotes
- ^ https://www.tanestrees.org.nz/resources/
- ^ https://waicare.org.nz
- ^ https://www.gida-global.org/care,WAI262-wai262.nz
- ^ https://treedivnet.ugent.be/experiments.html
References
Anderegg, W. R. L., Trugman, A. T., Badgley, G., Anderson, C. M., Bartuska, A., Ciais, P., et al. (2020). Climate-driven risks to the climate mitigation potential of forests. Science 368:6497. doi: 10.1126/science.aaz7005
Astudillo-García, C., Hermans, S. M., Stevenson, B., Buckley, H. L., and Lear, G. (2019). Microbial assemblages and bioindicators as proxies for ecosystem health status: potential and limitations. Appl. Microbiol. Biotechnol. 103, 6407–6421. doi: 10.1007/s00253-019-09963-0
Baldeck, C. A., Harms, K. E., Yavitt, J. B., John, R., Turner, B. L., Valencia, R., et al. (2013). Soil resources and topography shape local tree community structure in tropical forests. Proc. R. Soc. B: Biol. Sci. 280:20122532. doi: 10.1098/rspb.2012.2532
Bibby, C. J., Burgess, N. D., Hill, D. A., and Mustoe, S. (2000). Bird census techniques, 2nd Edn. London: Academic Press.
Blair, J. M., Bohlen, P. J., and Freckman, D. W. (1997). Soil invertebrates as indicators of soil quality. Methods Assess. Soil Qual. 49, 273–291. doi: 10.2136/sssaspecpub49.c16
Bogale, M., Baniya, A., and DiGennaro, P. (2020). Nematode identification techniques and recent advances. Plants 9:1260. doi: 10.3390/plants9101260
Borges, F. L. G., da Rosa Oliveira, M., de Almeida, T. C., Majer, J. D., and Garcia, L. C. (2021). Terrestrial invertebrates as bioindicators in restoration ecology: a global bibliometric survey. Ecol. Indic. 125:107458. doi: 10.1016/j.ecolind.2021.107458
Brears, R. (2022). Financing nature-based solutions: exploring public, private, and blended finance models and case studies. Cham: Palgrave Macmillan. doi: 10.1007/978-3-030-93325-8
Burdon, R. D., Carson, M. J., and Shelbourne, C. J. A. (2008). Achievements in forest tree genetic improvement in Australia and New Zealand 10: pinus radiata in New Zealand. Aust. For. 71, 263–279. doi: 10.1080/00049158.2008.10675045
Burns, B., Innes, J., and Day, T. (2012). “The use and potential of pest-proof fencing for ecosystem restoration and fauna conservation in New Zealand,” in Fencing for conservation: restriction of evolutionary potential or a riposte to threatening processes?, eds M. J. Somers and M. W. Hayward (Berlin: Springer Science + Business Media), 65–90. doi: 10.1007/978-1-4614-0902-1_5
Carter, M. R., and Gregorich, E. G. (2008). Soil sampling and methods of analysis. Boca Raton: CRC press. doi: 10.1201/9781420005271
Case, B. S., Pannell, J. L., Stanley, M. C., Norton, D. A., Brugman, A., Funaki, M., et al. (2020). The roles of non-production vegetation in agroecosystems: a research framework for filling process knowledge gaps in a social-ecological context. People Nat. 2, 292–304. doi: 10.1002/pan3.10093
Case, B., and Ryan, C. (2020). An analysis of carbon stocks and net carbon position for New Zealand sheep and beef farmland. Auckland: Auckland University of Technology.
Chausson, A., Turner, B., Seddon, D., Chabaneix, N., Girardin, C. A., Kapos, V., et al. (2020). Mapping the effectiveness of nature-based solutions for climate change adaptation. Glob. Chang Biol. 26, 6134–6155. doi: 10.1111/gcb.15310
Clarkson, B. D. (2022). Reversing biodiversity decline in Aotearoa New Zealand. Policy Q. 18, 61–70. doi: 10.26686/pq.v18i2.7576
Cohen-Shacham, E., Walters, G. M., Maginnis, S., and Janzen, C. (2016). “Nature-based solutions to address global societal challenges,” in Nature-based solutions to address global societal challenges, eds E. Cohen-Shacham, G. Walters, C. Janzen, and S. Maginnis (Switzerland: International Union for Conservation of Nature). doi: 10.2305/IUCN.CH.2016.13.en
Coux, C., Donoso, I., Tylianakis, J. M., García, D., Martínez, D., Dehling, D. M., et al. (2021). Tricky partners: native plants show stronger interaction preferences than their exotic counterparts. Ecology 10:e03239. doi: 10.1002/ecy.3239
Dodd, M., Barker, G., Burns, B., Didham, R., Innes, J., King, C., et al. (2011). Resilience of New Zealand indigenous forest fragments to impacts of livestock and pest mammals. N Z. J. Ecol. 35, 83–95.
Douglas, G. B., Dodd, M. B., and Power, I. L. (2007). Potential of direct seeding for establishing native plants into pastoral land in New Zealand. N Z. J. Ecol. 31, 143–153.
Ehrenfeld, J. G., and Toth, L. A. (1997). Restoration ecology and the ecosystem perspective. Restore. Ecol. 5, 307–317. doi: 10.1046/j.1526-100X.1997.00544.x
Elliott, G. P., Wilson, P. R., Taylor, R. H., and Beggs, J. R. (2010). Declines in common, widespread native birds in a mature temperate forest. Biol. Conserv. 143, 2119–2126. doi: 10.1016/j.biocon.2010.05.022
Facelli, J. M., and Pickett, S. T. (1991). Plant litter: its dynamics and effects on plant community structure. Bot. Rev. 57, 1–32. doi: 10.1007/BF02858763
Feest, A., Aldred, T. D., and Jedamzik, K. (2010). Biodiversity quality: a paradigm for biodiversity. Ecol. Indic. 10, 1077–1082. doi: 10.1016/j.ecolind.2010.04.002
Fleischman, F., Basant, S., Chhatre, A., Coleman, E. A., Fischer, H. W., Gupta, D., et al. (2020). Pitfalls of Tree Planting Show Why We Need People-Centered Natural Climate Solutions. BioScience 70, 947–950. doi: 10.1093/biosci/biaa094
Forbes, A. R., and Craig, J. L. (2013). Assessing the role of revegetation in achieving restoration goals on Tiritiri Matangi Island. N Z. J. Ecol. 37, 343–352.
Forbes, A. S., Wallace, K. J., Buckley, H. L., Case, B. S., Clarkson, B. D., and Norton, D. A. (2020). Restoring mature-phase forest tree species through enrichment planting in New Zealand’s lowland landscapes. N Z. J. Ecol. 44, 1–9. doi: 10.20417/nzjecol.44.10
Funtowicz, S. O., and Ravetz, J. R. (1993). Science for the post-normal age. Futures 25, 739–755. doi: 10.1016/0016-3287(93)90022-L
Fusaro, S., Gavinelli, F., Lazzarini, F., and Paoletti, M. G. (2018). Soil Biological Quality Index based on earthworms (QBS-e). A new way to use earthworms as bioindicators in agroecosystems. Ecol. Indic. 93, 1276–1292. doi: 10.1016/j.ecolind.2018.06.007
Gann, G. D., McDonald, T., Walder, B., Aronson, J., Nelson, C. R., Jonson, J., et al. (2019). International principles and standards for the practice of ecological restoration. Restor. Ecol. 27, S1–S46. doi: 10.1111/rec.13035
García, D., Martínez, D., Stouffer, D. B., and Tylianakis, J. M. (2014). Exotic birds increase generalization and compensate for native bird decline in plant–frugivore assemblages. J. Anim. Ecol. 83, 1441–1450. doi: 10.1111/1365-2656.12237
Gatica-Saavedra, P., Echeverría, C., and Nelson, C. R. (2017). Ecological indicators for assessing ecological success of forest restoration: a world review. Restor. Ecol. 25, 850–857. doi: 10.1111/rec.12586
Gehring, C. A., Theimer, T. C., Whitham, T. G., and Keim, P. (1998). Ectomycorrhizal fungal community structure of pinyon pines growing in two environmental extremes. Ecology 79, 1562–1572. doi: 10.1890/0012-9658(1998)079[1562:EFCSOP]2.0.CO;2
Gerlach, J., Samways, M., and Pryke, J. (2013). Terrestrial invertebrates as bioindicators: an overview of available taxonomic groups. J. Insect Conserv. 17, 831–850. doi: 10.1007/s10841-013-9565-9
Gotelli, N. J., and Ellison, A. M. (2013). A primer of ecological statistics, 2nd Edn. Massachusetts, USA: Sinauer Associates.
Gottschall, F., Cesarz, S., Auge, H., Kovach, K. R., Mori, A. S., Nock, C. A., et al. (2022). Spatiotemporal dynamics of abiotic and biotic properties explain biodiversity–ecosystem-functioning relationships. Ecol. Monogr. 92:e01490. doi: 10.1002/ecm.1490
Grant, C. D., Ward, S. C., and Morley, S. C. (2007). Return of ecosystem function to restored bauxite mines in Western Australia. Restor. Ecol. 15, S94–S103. doi: 10.1111/j.1526-100X.2007.00297.x
Green, C. (2000). Pitfall trapping for long-term monitoring of invertebrates. Ecol. Manag. 8, 73–93.
Griffiths, G. J., Khin, J., Landers, T. J., Lawrence, G., Ludbrook, M. R., and Bishop, C. D. (2021). Ecological integrity of forests in tāmaki makaurau/auckland 2009-2019. state of the environment reporting. Auckland: Auckland Council.
Grossman, J. J., Vanhellemont, M., Barsoum, N., Bauhus, J., Bruelheide, H., Castagneyrol, B., et al. (2018). Synthesis and future research directions linking tree diversity to growth, survival, and damage in a global network of tree diversity experiments. Environ. Exp. Bot. 152, 68–89. doi: 10.1016/j.envexpbot.2017.12.015
Gunderson, L. H. (2000). Ecological resilience—in theory and application. Annu. Rev. Ecol. Evol. Syst. 31, 425–439. doi: 10.1146/annurev.ecolsys.31.1.425
Hall, D. (2020). “Rhetoric and reality in New Zealand’s climate leadership?: ‘My generation’s nuclear-free moment,” in Climate governance across the globe, (Milton Park: Routledge), 82–100. doi: 10.4324/9781003014249-7
Hall, D., and Lindsay, S. (2021). Scaling climate finance: biodiversity instruments. Auckland: Mōhio Research.
Hallmann, J., and Subbotin, S. A. (2018). “Methods for extraction, processing and detection of plant and soil nematodes,” in Plant parasitic nematodes in subtropical and tropical agriculture, 3rd Edn, eds D. Coyne, J. Hallmann, and P. Timper (Germany: Sikora), 87–119. doi: 10.1079/9781786391247.0087
Hartley, L. and Greene, T. (2012). Incomplete counts – Five-minute bird counts, version 1.0. New Zealand:Department of Conservation Inventory
Healy, S., Huygens, I., and Murphy, T. (2012). Ngāpuhi speaks. Whangārei: Network Waitangi Whangārei.
Hermans, S. M., Buckley, H. L., Case, B. S., Curran-Cournane, F., Taylor, M., and Lear, G. (2017). Bacteria as emerging indicators of soil condition. Appl. Environ. Microbiol. 83, e2826–e2816. doi: 10.1128/AEM.02826-16
Hermans, S. M., Taylor, M., Grelet, G., Curran-Cournane, F., Buckley, H. L., Handley, K. M., et al. (2020). From pine to pasture: land use history has long-term impacts on soil bacterial community composition and functional potential. FEMS Microbiol. Ecol. 96:fiaa041. doi: 10.1093/femsec/fiaa041
Hisano, M., Searle, E. B., and Chen, H. Y. (2018). Biodiversity as a solution to mitigate climate change impacts on the functioning of forest ecosystems. Biol. Rev. 93, 439–456. doi: 10.1111/brv.12351
Höltermann, A. (2020). “Forests under a changing climate: increasing adaptability and resilience through more diversity and heterogeneity,” in How to balance forestry and biodiversity conservation: a view across Europe, eds F. Krumm, A. Schuck, and A. Rigling (Joensuu: European Forest Institute and Swiss).
Hutchison, C., Gravel, D., Guichard, F., and Potvin, C. (2018). Effect of diversity on growth, mortality, and loss of resilience to extreme climate events in a tropical planted forest experiment. Sci. Rep. 8:15443. doi: 10.1038/s41598-018-33670-x
Jackson, M. (2020). “Where to next? Decolonisation and the stories of the land,” in Imagining Decolonisation, eds R. Kiddle, B. Elkington, M. Jackson, O. R. Mercier, M. Ross, J. Smeaton, et al. (Wellington: Bridget Williams Books), 55–65. doi: 10.7810/9781988545783_5
Janowski, D., Wilgan, R., Leski, T., Karliński, L., and Rudawska, M. (2019). Effective molecular identification of ectomycorrhizal fungi: revisiting DNA isolation methods. Forests 10:218. doi: 10.3390/f10030218
Kawharu, M. (2000). Kaitiakitanga: a Māori anthropological perspective of the Māori socio-environmental ethic of resource management. J. Polyn. Soc. 109, 349–370.
Keuskamp, J. A., Dingemans, B. J. J., Lehtinen, T., Sarneel, J. M., and Hefting, M. M. (2013). Tea Bag Index: a novel approach to collect uniform decomposition data across ecosystems. Methods Ecol. Evol. 4, 1070–1075. doi: 10.1111/2041-210X.12097
Kimberley, M., Bergin, D., and Silvester, W. (2021). Carbon sequestration by native forest: setting the record straight. New Zealand: Tane’s Tree Trust.
Koroi, H. (2021). “In right relationship – whanaungatanga,” in Climate Aotearoa: what’s happening and what we can do about it, ed. H. Clark (Auckland: Allen & Unwin).
Kukutai, T., and Taylor, J. (2016). Indigenous data sovereignty: toward an agenda. Australian: ANU press. doi: 10.22459/CAEPR38.11.2016
Lear, G., Dickie, I., Banks, J., Boyer, S., Buckley, H. L., Buckley, T. R., et al. (2018). Methods for the extraction, storage, amplification and sequencing of DNA from environmental samples. N Z. J. Ecol. 42, 10A–50A. doi: 10.20417/nzjecol.42.9
Lee, W., McGlone, M., and Wright, E. (2005). “Biodiversity inventory and monitoring: a review of national and international systems and a proposed framework for future biodiversity monitoring by the Department of Conservation,” in Landcare research contract report LC0405/122, (Wellington: Landcare Research).
Leining, C. (2022). A guide to the new zealand emissions trading scheme: 2022 update. Wellington: Motu Economic and Public Policy Research.
Leining, C., Kerr, S., and Bruce-Brand, B. (2019). The New Zealand Emissions Trading Scheme: critical review and future outlook for three design innovations. Clim.Policy 20, 246–264. doi: 10.1080/14693062.2019.1699773
Lindenmayer, D. B., Likens, G. E., Andersen, A., Bowman, D., Bull, C. M., Burns, E., et al. (2012). Value of long-term ecological studies. Austral Ecol. 37, 745–757. doi: 10.1111/j.1442-9993.2011.02351.x
Liu, C. L. C., Kuchma, O., and Krutovsky, K. V. (2018). Mixed-species versus monocultures in plantation forestry: development, benefits, ecosystem services and perspectives for the future. Glob. Ecol. Conserv. 15:e00419. doi: 10.1016/j.gecco.2018.e00419
Lovegrove, T. G., Zeiler, C. H., Greene, B. S., Green, B. W., Gaastra, R., and MacArthur, A. D. (2002). “Alien plant and animal control and aspects of ecological restoration in a small ‘mainland island’: wenderholm Regional Park, New Zealand,” in Turning the tide: the eradication of invasive species. IUCN SSC Invasive Species Specialist Group, eds C. R. Veitch and M. N. Clout (Cambridge: IUCN Gland), 155–163.
Lu, Q., Liu, T., Wang, N., Dou, Z., Wang, K., and Zuo, Y. (2020). A review of soil nematodes as biological indicators for the assessment of soil health. Front. Agric. Sci. Eng. 7, 275–281. doi: 10.15302/J-FASE-2020327
Lyver, P. O. B., Wilmshurst, J. M., Wood, J. R., Jones, C. J., Fromont, M., Bellingham, P. J., et al. (2015). Looking back for the future: local knowledge and palaeoecology inform biocultural restoration of coastal ecosystems in New Zealand. Hum. Ecol. 43, 681–695. doi: 10.1007/s10745-015-9784-7
Macinnis-Ng, C., Mcintosh, A. R., Monks, J. M., Waipara, N., White, R. S. A., Boudjelas, S., et al. (2021). Climate-change impacts exacerbate conservation threats in island systems: new Zealand as a case study. Front. Ecol. Environ. 19, 216–224. doi: 10.1002/fee.2285
MacKay, D. B., Wehi, P. M., and Clarkson, B. D. (2011). Evaluating restoration success in urban forest plantings in hamilton. Auckland: Urban Habitats
Martin, K. J. (2007). Introduction to molecular analysis of ectomycorrhizal communities. Soil Sci. Soc. Am. J. 71, 601–610. doi: 10.2136/sssaj2006.0115
Mathieu, C., Hermans, S. M., Lear, G., Buckley, T. R., Lee, K. C., and Buckley, H. L. (2020). A systematic review of sources of variability and uncertainty in eDNA data for environmental monitoring. Front. Ecol. Evol. 8:135. doi: 10.3389/fevo.2020.00135
Matthews, R. W., and Matthews, J. R. (2017). The Malaise trap: its utility and potential for sampling insect populations. Gt. Lakes Entomol. 4:4.
McArthur, J. (2017). Auckland: rescaled governance and post-suburban politics. Cities 64, 79–87. doi: 10.1016/j.cities.2017.01.010
McDonald, T., Gann, G. D., Jonson, J., Dixon, K. W., Aronson, J., Decleer, K., et al. (2016). International standards for the practice of ecological restoration–including principles and key concepts. Washington, D.C: Society for Ecological Restoration.
McGlone, M. S., McNutt, K., Richardson, S. J., Bellingham, P. J., and Wright, E. F. (2020). Biodiversity monitoring, ecological integrity, and the design of the New Zealand Biodiversity Assessment Framework. N Z. J. Ecol. 44:3411. doi: 10.20417/nzjecol.44.17
Meisch, S. P., Bremer, S., Young, M. T., and Funtowicz, S. O. (2022). Extended Peer Communities: appraising the contributions of tacit knowledges in climate change decision-making. Futures 135:102868. doi: 10.1016/j.futures.2021.102868
Melia, N., Dean, S., Pearce, H. G., Harrington, L., Frame, D. J., and Strand, T. (2022). Aotearoa New Zealand’s 21st-century wildfire climate. Earth’s Future 10:e2022EF002853. doi: 10.1029/2022EF002853
Ministry for the Environment (2020). Marginal abatement cost curves analysis for New Zealand: potential greenhouse gas mitigation options and their costs. Wellington: Ministry for the Environment.
Ministry for the Environment (2021). New Zealand’s greenhouse gas inventory 1990-2019. Wellington: Ministry for the Environment.
Ministry for the Environment (2022). Draft national adaptation plan. Wellington: Ministry for the Environment.
Miralles-Wilhelm, F. (2021). Nature-based solutions in agriculture: sustainable management and conservation of land, water, and biodiversity. Rome: FAO and the Nature Conservancy.
Montgomery, G. A., Belitz, M. W., Guralnick, R. P., and Tingley, M. W. (2021). Standards and best practices for monitoring and benchmarking insects. Front. Ecol. Evol. 8:513. doi: 10.3389/fevo.2020.579193
Moore, J. R., and Watt, M. S. (2015). Modelling the influence of predicted future climate change on the risk of wind damage within New Zealand’s planted forests. Glob. Change Biol. 21, 3021–3035. doi: 10.1111/gcb.12900
New Zealand Government (2022). Te hau mārohi ki anamata: towards a productive, sustainable and inclusive economy: aotearoa New Zealand’s first emissions reduction plan. Wellington: Ministry for the Environment.
Norton, D. A. (2009). Species invasions and the limits to restoration: learning from the New Zealand experience. Science 325, 569–571. doi: 10.1126/science.1172978
Paoletti, M. G. (1999). The role of earthworms for assessment of sustainability and as bioindicators. Agric. Ecosyst. Environ. 74, 137–155. doi: 10.1016/S0167-8809(99)00034-1
Paul, T., Scott, M., Lennox, H., and Lord, J. (2020). Restoring wilding stands in the wakatipu basin by seeding native trees. report for the wakatipu beech restoration trust. Rotorua: Scion.
Perring, M. P., Standish, R. J., Hulvey, K. B., Lach, L., Morald, T. K., Parsons, R., et al. (2012). The Ridgefield Multiple Ecosystem Services Experiment: can restoration of former agricultural land achieve multiple outcomes? Agric. Ecosyst. Environ. 163, 14–27. doi: 10.1016/j.agee.2012.02.016
Peters, M. A., Hamilton, D., and Eames, C. (2015). Action on the ground: A review of community environmental groups’ restoration objectives, activities and partnerships in New Zealand. N. Z. J. Ecol. 39, 179–189.
Peterson, G., Allen, C. R., and Holling, C. S. (1998). Ecological resilience, biodiversity, and scale. Ecosystems 1, 6–18. doi: 10.1007/s100219900002
Porteous, T. (1993). Native forest restoration: a practical guide for landowners. Wellington: Queen Elizabeth the Second National Trust.
Pörtner, H. O., Scholes, R. J., Agard, J., Archer, E., Arneth, A., Bai, X., et al. (2021). Scientific outcome of the IPBES-IPCC co-sponsored workshop on biodiversity and climate change. Germany: IPBES,
Pulleman, M., Creamer, R., Hamer, U., Helder, J., Pelosi, C., Peres, G., et al. (2012). Soil biodiversity, biological indicators and soil ecosystem services—an overview of European approaches. Curr. Opin. Environ. Sustain. 4, 529–538. doi: 10.1016/j.cosust.2012.10.009
Reed, G., Brunet, N. D., McGregor, D., Scurr, C., Sadik, T., Lavigne, J., et al. (2022). Toward Indigenous visions of nature-based solutions: an exploration into Canadian federal climate policy. Clim. Policy 22, 514–533. doi: 10.1080/14693062.2022.2047585
Roberts, M. (2013). Ways of seeing: whakapapa. J. Soc. Anthropol. Cult. Stud. 10, 93–120. doi: 10.11157/sites-vol10iss1id236
Rondeux, J., and Sanchez, C. (2010). Review of indicators and field methods for monitoring biodiversity within national forest inventories Core variable: deadwood. Environ. Monitor. Assess. 164, 617–630. doi: 10.1007/s10661-009-0917-6
Sacco, A., Hardwick, L., Blakesley, D., Brancalion, P. H. S., Breman, E., Cecilio Rebola, L., et al. (2021). Ten golden rules for reforestation to optimize carbon sequestration, biodiversity recovery and livelihood benefits. Glob. Change Biol. 27, 1328–1348. doi: 10.1111/gcb.15498
Scherer-Lorenzen, M., Schulze, E. D., Don, A., Schumacher, J., and Weller, E. (2007). Exploring the functional significance of forest diversity: a new long-term experiment with temperate tree species (BIOTREE). Perspective Plant Ecol. Evol. Syst. 9, 53–70. doi: 10.1016/j.ppees.2007.08.002
Schwarz, J., Schnabel, F., and Bauhus, J. (2021). A conceptual framework and experimental design for analysing the relationship between biodiversity and ecosystem functioning (BEF) in agroforestry systems. Basic Appl. Ecol. 55, 133–151. doi: 10.1016/j.baae.2021.05.002
Seddon, N. (2022). Harnessing the potential of nature-based solutions for mitigating and adapting to climate change. Science 376, 1410–1416. doi: 10.1126/science.abn9668
Seddon, N., Daniels, E., Davis, R., Chausson, A., Harris, R., Hou-Jones, X., et al. (2020b). Global recognition of the importance of nature-based solutions to the impacts of climate change. Glob. Sustain. 3:E15. doi: 10.1017/sus.2020.8
Seddon, N., Chausson, A., Berry, P., Girardin, C. A. J., Smith, A., and Turner, B. (2020a). Understanding the value and limits of nature-based solutions to climate change and other global challenges. Philos. Trans. R. Soc. B 375:1794. doi: 10.1098/rstb.2019.0120
Seddon, N., Smith, A., Smith, P., Key, I., Chausson, A., Girardin, C., et al. (2021). Getting the message right on nature-based solutions to climate change. Glob. Change Biol. 27, 1518–1546. doi: 10.1111/gcb.15513
Sherley, G., and Evans, A. (2016). Invertebrates: Search and extraction methods, Version 1.1. Wellington: Department of Conservation.
Sherley, G., and Stringer, I. (2016). Invertebrates: pitfall trapping, Version 1.0. New Zealand: Department of Conservation.
Simelton, E., Carew-Reid, J., Coulier, M., Damen, B., Howell, J., Pottinger-Glass, C., et al. (2021). NBS Framework for Agricultural Landscapes. Front. Environ. Sci. 9:321. doi: 10.3389/fenvs.2021.678367
Singers, N. J., Osborne, B., Lovegrove, T., Jamieson, A., Boow, J., Sawyer, J. W. D., et al. (2017). Indigenous terrestrial and wetland ecosystems of Auckland. Auckland: Te Kaunihera o Tāmaki Makaurau.
Smith, M. W., Carrivick, J. L., and Quincey, D. J. (2016). Structure from motion photogrammetry in physical geography. Prog. Phys. Geogr. 40, 247–275. doi: 10.1177/0309133315615805
Sparrow, B. D., Edwards, W., Munroe, S. E., Wardle, G. M., Guerin, G. R., Bastin, J. F., et al. (2020). Effective ecosystem monitoring requires a multi-scaled approach. Biol. Rev. 95, 1706–1719. doi: 10.1111/brv.12636
Standards Reference Group [SERA] (2021). National standards for the practice of ecological restoration in Australia. Washington, DC: Society for Ecological Restoration Australasia.
Suryaningrum, F., Jarvis, R. M., Buckley, H. L., Hall, D., and Case, B. S. (2021). Large-scale tree planting initiatives as an opportunity to derive carbon and biodiversity co-benefits: a case study from Aotearoa New Zealand. New For. 53, 589–602 doi: 10.1007/s11056-021-09883-w
Te Uru Rākau (2022). Latest ETS Forest Land Statistics. Available online at: https://www.mpi.govt.nz/forestry/forestry-in-the-emissions-trading-scheme/news-and-changes-to-the-ets/ (accessed December 2, 2022).
Tulod, A. M., and Norton, D. A. (2020). Regeneration of native woody species following artificial gap formation in an early-successional forest in New Zealand. Ecol. Manag. Restore. 21, 229–236. doi: 10.1111/emr.12429
Uriarte, M., Condit, R., Canham, C. D., and Hubbell, S. P. (2004). A spatially explicit model of sapling growth in a tropical forest: Does the identity of neighbours matter? J. Ecol. 92, 348–360.
Van Groenigen, J. W., Lubbers, I. M., Vos, H. M., Brown, G. G., De Deyn, G. B., and Van Groenigen, K. J. (2014). Earthworms increase plant production: a meta-analysis. Sci. Rep. 4:6365. doi: 10.1038/srep06365
Wainscott, B. C. (2015). Surveying forest diversity and health using the point-centered quarter method. Proc. Assoc. Biol. Lab. Educ. 36:18.
Wallace, K. J., Clarkson, B. D., and Farnworth, B. (2022). Restoration trajectories and ecological thresholds during planted urban forest successional development. Forests 13:199. doi: 10.3390/f13020199
Wannan, O. (2022). Moves to limit pine would force landowners, Māori to forego ETS cash. New York, NY: Stuff.
Ward, D. F., and Larivière, M. C. (2004). Terrestrial invertebrate surveys and rapid biodiversity assessment in New Zealand: lessons from Australia. N Z. J. Ecol. 28, 151–159.
Watt, M., Palmer, D., Kimberley, M., Höck, B., Payn, T., and Lowe, D. (2010). Development of models to predict Pinus radiata productivity throughout New Zealand. Can. J. For. Res. 40, 488–499. doi: 10.1139/X09-207
Welsch, J., Songling, C., Buckley, H. L., Lehto, N. J., Jones, E. E., and Case, B. S. (2019). How many samples? Soil variability affects confidence in the use of common agroecosystem soil indicators. Ecol. Indic. 102, 401–409. doi: 10.1016/j.ecolind.2019.02.065
Keywords: tree planting, experimental restoration, climate change mitigation, climate adaptation, community engagement, ecosystem function, biodiversity, native forest
Citation: Buckley HL, Hall D, Jarvis RM, Smith V, Walker LA, Silby J, Hinchliffe G, Stanley MC, Sweeney AP and Case BS (2023) Using long-term experimental restoration of agroecosystems in Aotearoa New Zealand to improve implementation of Nature-based Solutions for climate change mitigation. Front. For. Glob. Change 5:950041. doi: 10.3389/ffgc.2022.950041
Received: 22 May 2022; Accepted: 22 December 2022;
Published: 09 January 2023.
Edited by:
Leland Kendall Werden, ETH Zürich, SwitzerlandReviewed by:
Alan Feest, University of Bristol, United KingdomEddie John Van Etten, Edith Cowan University, Australia
Copyright © 2023 Buckley, Hall, Jarvis, Smith, Walker, Silby, Hinchliffe, Stanley, Sweeney and Case. This is an open-access article distributed under the terms of the Creative Commons Attribution License (CC BY). The use, distribution or reproduction in other forums is permitted, provided the original author(s) and the copyright owner(s) are credited and that the original publication in this journal is cited, in accordance with accepted academic practice. No use, distribution or reproduction is permitted which does not comply with these terms.
*Correspondence: Hannah L. Buckley, SGFubmFoLkJ1Y2tsZXlAYXV0LmFjLm56; David Hall,
ZGF2aWQuaGFsbEBhdXQuYWMubno=