- 1Department of Biology, Virginia Commonwealth University, Richmond, VA, United States
- 2United States Department of Agriculture Forest Service, Southern Research Station, New Ellenton, SC, United States
- 3Pacific Northwest National Laboratory, Joint Global Change Research Institute, College Park, MD, United States
The capacity of forests to resist structural change and retain material legacies–the biotic and abiotic resources that persist through disturbance–is crucial to sustaining ecosystem function after disturbance. However, the role of forest structure as both a material legacy and feature supporting carbon (C) cycling stability following disturbance has not been widely investigated. We used a large-scale disturbance manipulation to ask whether legacies of lidar-derived canopy structures drive 3-year primary production responses to disturbance. As part of the Forest Resilience Threshold Experiment (FoRTE) in northern Michigan, USA we simulated phloem-disrupting disturbances producing a range of severities and affecting canopy trees of different sizes. We quantified the legacies of forest structure using two approaches: one measuring the change in structure and primary production from pre-to post-disturbance and the second estimating resistance as log transformed ratios of control and treatment values. We found that total aboveground wood net primary production (ANPPw) was similar across disturbance severities as legacy trees rapidly increased rates of primary production. Experiment-wide, the disturbance had limited effects on change in mean structural complexity values; however, high variance underscored large differences in the magnitude and direction of complexity's response at the plot-scale. Plot-scale structural complexity, but not vegetation area index (VAI), resistance strongly predicted ANPPw resistance while temporal VAI and structural complexity changes did not. We conclude that the presence of material legacies in the form of forest structure may affect primary production stability following disturbance and that how legacies are quantified may affect the interpretation of disturbance response.
Introduction
Forests of the upper Great Lakes region have been strong carbon (C) sinks for over a century (Pan et al., 2011), but partial disturbances from pests, pathogens, and extreme weather threaten to diminish their capacity to sequester C (Running, 2008; Hicke et al., 2012; Seidl et al., 2014a). These partial disturbances are increasing in spatial extent in the region (Cohen et al., 2016), restructuring forests in ways that differ from historical stand-replacing disturbances. Unlike severe disturbance, partial or moderate severity disturbances may cause patchy, species-specific tree mortality or eliminate entire plant functional groups and, consequently, dramatically reshape forest structure while producing gradients of disturbance severity across forest landscapes (Atkins et al., 2020). Yet, the degree to which these resulting changes in forest structure correspond with C cycling responses to partial disturbance, including net primary production (NPP), is unclear (Fahey et al., 2016; Gough et al., 2020; Grigri et al., 2020).
Conceptual and analytical frameworks for interpreting how disturbance affects forest structure and function include two complementary ecological constructs, that of “material legacies” (sensu Johnstone et al., 2016) and “ecosystem stability” (sensu Hillebrand et al., 2018). The term “material legacy” represents the ecological memory of an environment and encompasses biotic and abiotic resources that are retained through disturbance and may support compositional and functional recovery (Franklin et al., 2000; Royo et al., 2010; Johnstone et al., 2016). Studies of material legacies have emphasized surviving tree species' abundances and vegetation spatial arrangement (Turner et al., 1998; Seidl et al., 2014b; Meigs and Keeton, 2018; Engelken et al., 2020); coarse downed and standing woody debris (Meigs and Keeton, 2018; Taboada et al., 2018; Engelken et al., 2020); and soil seed bank and seed persistence (Turner et al., 1998; Johnstone et al., 2016; Harris et al., 2021). These material legacies are sometimes calculated as the change in mass or abundance of a resource following disturbance, with some disturbances reducing (e.g., number of live stems) and others increasing (e.g., downed woody debris) resources in response to disturbance (Taboada et al., 2018). Concurrently, a complementary ecosystem stability literature provides guidance on defining and characterizing structural and functional responses to disturbance (Mathes et al., 2021). Among the metrics of ecosystem stability, “resistance” describes the direction and magnitude of structural and functional changes that immediately follow disturbance and is calculated as the log ratio of pre-post or control-treatment responses to disturbance (Hillebrand et al., 2018; Radchuk et al., 2019). Here, resistance is a relative measure and, consequently, normalizes for site differences that influence the absolute magnitude of structural or functional change and may otherwise obscure disturbance response patterns (Mathes et al., 2021). Thus, these conceptual and analytical frameworks provide complementary but not redundant approaches to quantifying and interpreting material legacies and their response to disturbance and may yield different results.
Applying these complementary ecological frameworks, we used a large-scale disturbance manipulation to ask: do the material legacies of canopy structure mediate 3-year above ground wood net primary production (ANPPw) responses to disturbance? Our analysis is part of the Forest Resilience Threshold Experiment (FoRTE), which used stem-girdling to attain 0, 45, 65, and 85% gross defoliation (i.e., disturbance severity) levels and two disturbance types, one targeting large canopy trees and one targeting smaller canopy trees (Figure 2). We focused on two canopy structural measures that are strongly coupled with ANPPw at our site and elsewhere: vegetation area index (VAI) and canopy rugosity (Hardiman et al., 2011; Fotis et al., 2018; Gough et al., 2019). VAI is a single, dimensionless value that expresses the number of wood and leaf layers per unit of ground area, while canopy rugosity is an integrative measure of structural complexity summarizing the horizontal and vertical variability in canopy-interior vegetation distribution (Hardiman et al., 2011). We focus on these biomass-dependent canopy structural measures because they are: sensitive to disturbance (Atkins et al., 2020), correlated with primary production (Gough et al., 2019), and meet the definition of “material legacies” (sensu Johnstone et al., 2016) but have not been studied in this context. Our specific objectives were to evaluate: (1) the separate contributions of the subcanopy and upper canopy to ANPPw after disturbance; (2) how VAI and canopy rugosity respond to different disturbance severities and treatment types, and (3) whether canopy structural and ANPPw changes and resistances are related. We hypothesized that there would be a significant rise in subcanopy ANPPw and a corresponding decline in upper canopy ANPPw as girdled-tree growth slowed and eventually ceased and subcanopy vegetation exhibited competitive release (Stuart-Haëntjens et al., 2015). We further hypothesized that VAI would be more sensitive to disturbance than canopy rugosity because vegetation area measures almost universally decline following tree mortality, while structural complexity displays more variable responses to disturbance (Fahey et al., 2016; Meigs and Keeton, 2018; Haber et al., 2020; Gough and Tallant, 2022). Finally, we hypothesized that ANPPw's response to disturbance would be more strongly coupled with canopy rugosity rather than VAI change or resistance because of primary production's relatively stronger tie to structural complexity (Hardiman et al., 2013; Gough et al., 2020).
Methods
Study site
Our study is part of the Forest Resilience Threshold Experiment (FoRTE) at the University of Michigan Biological Station (UMBS) in the northern lower peninsula of Michigan, USA (45.56°N, 84.68°W). Mean annual temperature was 7.2°C and mean annual precipitation is 75.4 cm in 2021 (NOAA, 2022), “Climate at a Glance”). The forests at our site are primarily 100-year-old secondary forests positioned on a gently sloping glacial outwash landscape. The upper canopy is composed of naturally declining early successional species: bigtooth and trembling aspen (Populus grandidentata and P. tremuloides) and paper birch (Betula papyrifera). Ascendent later successional species include red oak (Quercus rubra), eastern white pine (Pinus strobus), sugar maple (Acer saccharum), red maple (Acer rubrum), and American beech (Fagus grandifolia). The subcanopy is primarily composed of red maple, red oak, American beech, sugar maple, eastern white pine, serviceberry (Amelenchior spp.), red pine (Pinus resinosa), striped maple (Acer pennsylvanicum), and balsam fir (Abies balsamea). Throughout, we categorize forest strata as upper canopy > 8 cm diameter at breast height (DBH) and subcanopy 1- 8 cm DBH. Prior to the initiation of experimental treatment, the upper canopy leaf area in each subplot was estimated using the site or region-specific equations relating DBH to leaf area.
FoRTE is a replicated manipulation of disturbance severity and source. To simulate a phloem-disrupting disturbance, we stem-girdled ~3,600 canopy trees experiment-wide on May 2019. Stem-girdled trees were scored at 1 m height with a chainsaw and a 10 cm-wide strip of bark removed with a pry bar. Trees were girdled irrespective of tree species to simulate indiscriminate disturbances from generalist insect defoliators and phloem feeders.
Treatment replicates were nested within four distinct “land ecosystem types” that have been categorized by the unique soil, biota, landform, and climate (Pearsall et al., 1995), and are broadly representative of dominant ecosystems in the region (Nave et al., 2019; Figure 1A). While each of the land ecosystem types shares disturbance histories and overlapping tree species, the community composition, soils, structural complexity, and net primary production in each replicate differ (Hardiman et al., 2011; Scheuermann et al., 2018), allowing for inference beyond a single forest type. Each treatment replicate contained four 0.5-ha whole-plots, which were randomly assigned a disturbance severity of 0, 45, 65, or 85% gross defoliation based on previously established allometries relating stem diameter to leaf area index. Whole-plots were then bisected from north to south into two split-plots, which were designated either a top-down or bottom-up treatment type. We stem girdled the largest diameter trees first in the top-down treatment and the smallest first in the bottom-up treatment until disturbance severity (gross defoliation) targets were met (Figure 2); thus, the disturbance severity treatments also yielded variable stem diameter distributions and densities, approximating a range of structural changes associated with phloem-disrupting insects (Gough et al., 2020). Tree mortality from stem girdling generally occurred within 3 years, following the decline of root non-structural carbohydrates and functioning (Gough et al., 2010). Within split-plots, 0.1 ha circular subplots with a 5 m treatment buffer around its perimeter were established and used as the experimental unit throughout. In total, there were 32 subplots nested within 4 replicates (Figure 1A).
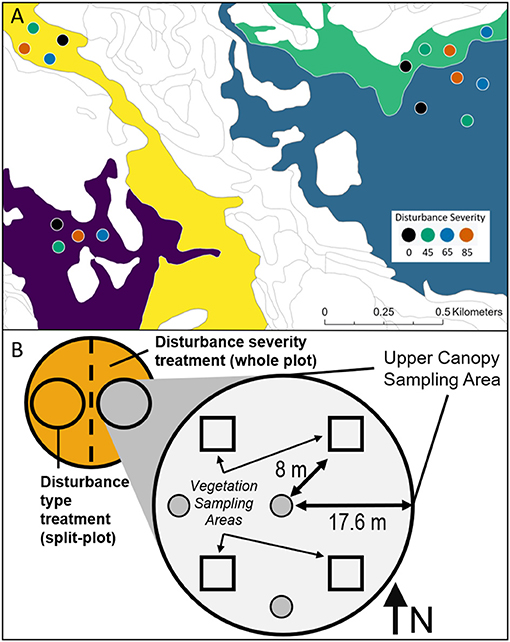
Figure 1. Map of Forest Threshold Resilience Experiment (FoRTE) replicates (A) and split-plot design (B). Replicates, which are used as experimental blocks, represent four different land ecosystem types and are designated by color with non-experimental land left white. Within each replicate, there are four randomly assigned disturbance severities representing 0, 45, 65, and 85% gross defoliation, which are designated by color (A). Each plot is likewise bisected into two subplots, with each half randomly assigned either top-down or bottom-up disturbance type (B). The subplot is used as the experimental unit throughout the experiment.
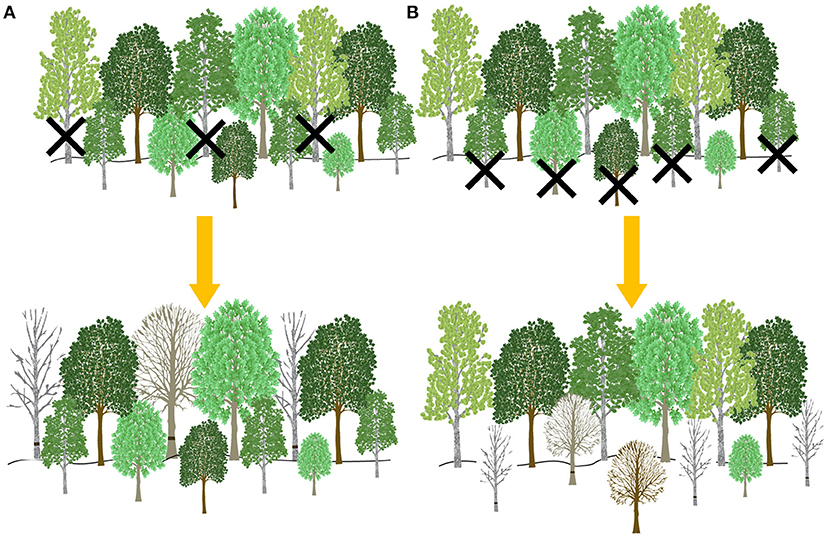
Figure 2. A representation of the two treatment types used in each subplot. In the top-down treatment type, the largest upper canopy trees (>8 cm) were girdled until the designated disturbance severity target (either 0, 45, 65, or 85% gross defoliation) was reached (A). In the bottom-up treatment type, the smallest upper canopy trees (>8 cm) were girdled until the designated disturbance severity target was reached (B). The bottom half of the figure illustrates the predicted remnant forest structure 3 years after disturbance initiation.
Aboveground wood net primary production
Subcanopy, upper canopy, and total aboveground wood net primary production (ANPPw) were calculated using protocols detailed by Grigri et al. (2020) and Atkins et al. (2021). Our approach used repeated measurements of DBH to infer the woody biomass increment from 1 year to the next. In the spring of 2018, all upper canopy and subcanopy trees were censused and identified to the species level. Dendrometer bands were installed at 1.3 m height on ~25% of all (girdled and non-girdled) upper canopy trees (n = 666) in the summer of 2018. Dendrometer bands were fitted atop a thin, mechanically shaved band of outer bark to ensure an even and snug fit. The bands are made of 1.27 cm-wide steel tape with a 5.08 cm stainless steel spring and stickers indicating the change in circumference of each tree. Each year, dendrometer bands were read at least once in summer and the following autumn, when stem growth has paused for the dormant season (Gough et al., 2009).
Daily species-specific relative growth rates (RGR, cm day−1) were estimated for each subplot (Grigri et al., 2020). When there was no relationship between RGR and DBH (p > 0.05), a mean subplot and species-specific RGR value were applied to un-banded trees to estimate their annual DBH increment. When subplot-level RGR varied by DBH (p < 0.05), RGR of the unbanded trees was modeled using regression equations that adjust for the effect of diameter.
Once the annual DBH increment was estimated separately for all banded and unbanded upper canopy trees, site-specific allometries were used to calculate aboveground wood biomass (kg) (Cooper, 1981; Gough et al., 2008). Annual aboveground wood biomass increments of the upper canopy were then used to estimate ANPPw (kg C ha−1year−1) by scaling the sum of woody biomass growth per subplot to the hectare and multiplying by 0.48, the site-specific C fraction, to convert biomass to C mass (Gough et al., 2008).
In the subcanopy, annual DBH measurements were used to measure diameter growth. Species-level censuses were conducted in 2019 in one quarter of each subplot (0.025 ha) to estimate the stem density of the subcanopy and four 2 × 2 m vegetation sampling areas were established in each subplot (Figure 1B). Within vegetation sampling areas, two individuals were tagged for repeated DBH measurements. In instances where there were fewer than two subcanopy class trees within a vegetation sampling area, the subcanopy tree nearest to the center of the vegetation sampling area was selected and measured instead. Repeated DBH measurements of the tagged subcanopy trees in the summer and autumn of each year were used to calculate the annual increment of growth. Subcanopy woody biomass was calculated using annual growth increment and site-specific allometries, accounting for subcanopy stem density in each subplot.
Total subplot ANPPw was calculated as the sum of upper canopy and subcanopy ANPPw with uncertainty calculated as the standard error of mean annual ANPPw among subplots (Gough et al., 2008).
Forest structure
We focused our analysis on canopy structural metrics derived from Light Detection and Ranging (lidar) technology that is tied to net primary production at our site and others (Gough et al., 2019). A portable canopy lidar (PCL) was used in the summers of 2018–2021 to map horizontal and vertical vegetation arrangements in each subplot. The PCL consists of a metal frame that is worn at 1 m above ground level, mounted with a laser with a maximum pulse frequency of 2,000 Hz (Riegl LD90 3100 VHS; Riegl USA, Inc., Orlando, Florida). Measurements were taken in each subplot in two perpendicular 40 m transects one running north-south and one running east-west. Structural metrics were derived from the two-dimensional hit-grids using forestr in R (Atkins et al., 2018). For full mathematical derivations of structural metrics, we refer readers to Atkins et al. (2018). Briefly, VAI is a vegetation cover metric, summarizing the number of leaf and wood layers per unit of ground area. Canopy rugosity is a multi-dimensional canopy structural complexity measure, mathematically defined as the product of horizontal and vertical vegetation density variances (Hardiman et al., 2011). Canopy rugosity's spatially integrative properties are strongly tied to growth-limiting resource-use efficiency and acquisition, and production (Gough et al., 2020).
Structural and primary production resistance and changes
We used two separate approaches to calculate shifts in ANPPw and canopy structure following disturbance. The first calculated subplot changes (δ) over time (before and after disturbance) in canopy structure and ANPPw by subtracting mean subplot values in 2018 or 2019 (ANPPw only) from those of 2021, following a conventional change-based approach to quantify material legacies (Taboada et al., 2018). A second approach utilized a stability framework (sensu Mathes et al., 2021), estimating resistance as the log response ratio of control vs. treatment VAI, canopy rugosity, or ANPPw. Positive resistance and δ values signal a net increase in canopy structural material legacies or ANPPw following disturbance, while negative values signal their net decline. We employ both approaches because a standardized convention for estimating how material legacies respond to disturbance is lacking, and each approach has inherent strengths and limitations. For example, change-based approaches do not require control, but they are influenced by factors other than disturbance, such as inter-annual climate and site variation. More recently introduced stability measures, including resistance, provide relative, normalized expressions of disturbance response akin to effect size and may reveal patterns that are otherwise obscured by additional sources of variability (Hillebrand et al., 2018); however, such stability measures may not be desirable when absolute responses are of interest.
Statistical analysis
For categorical comparisons of mean ANPPw by disturbance severity, year, and canopy strata, we used a time-series split plot analysis of variance (ANOVA). ANPPw was log transformed to meet the assumption of normality across all canopy strata. Levene's test was used to test for homogeneity of variance; assumptions of equal variance were met in the upper canopy, but not in the subcanopy, where variance increased with increasing disturbance severity. Pairwise comparisons of ANPPw were conducted using Fisher's least significant difference across time and disturbance severity. We used two-way ANOVA to compare mean changes and resistances of VAI and canopy rugosity by disturbance treatment. Assumptions of normality and equal variance were checked using a Shapiro-Wilkes test and Levene's test, respectively. We used simple linear regression to evaluate whether total ANPPw change and resistance correlate with canopy rugosity and VAI change and resistance, respectively. An analysis of covariance (ANCOVA) was performed to test for interactions between disturbance severity and (top-down/bottom-up) type. Assumptions of normality and homogeneity were conducted using visual inspection of plots of residuals. A significance level of 0.05 was applied for all analyses. All statistical analyses were conducted using R software (v4.0.2) using agricolae (v1.3-3, de Mendiburu, 2020) package.
Results
Aboveground wood net primary production
Compensatory growth fully sustained ANPPw after disturbance, even at the highest severity level. Mean upper canopy ANPPw did not differ among disturbance severities or treatment types (p > 0.05, Figure 3A; Supplementary Table S1), comprising 95 and 82% of total ANPPw in 2019 and 2021, respectively. In contrast, subcanopy ANPPw increased with rising disturbance severity, contributing a larger proportion over time to total ANPPw (p < 0.05, Figure 3B; Supplementary Table S1). Mean subcanopy ANPPw at the 85% disturbance severity level averaged 127 kgC ha−1 year−1 in 2019 and 777 kgC ha−1 year−1 in 2021, a 5-fold increase. In 2021, the contribution of the subcanopy to total ANPPw reached 6, 17, 23, and 27% of total ANPPw in the control, 45, 65, and 85% gross defoliation levels, respectively. Thus, subcanopy and canopy material legacies responded dynamically and completely to offset the effects of rising disturbance severity, resulting in no significant differences in total ANPPw among disturbance treatments (p > 0.05; Figure 3C).
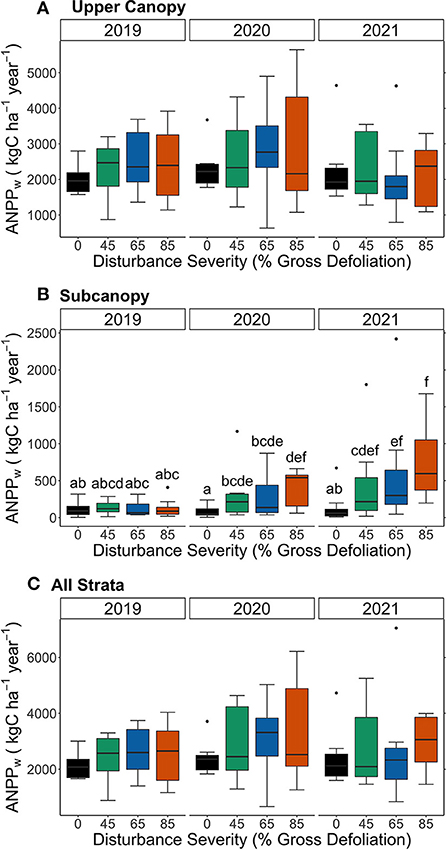
Figure 3. Box plots representing aboveground wood net primary production (ANPPw) in the upper canopy (A), subcanopy (B), and sum of both upper canopy and subcanopy (C). Middle horizontal lines represent the median ANPPw, boxes represent the interquartile ranges, and the whiskers represent the maximum and minimum subplot values for ANPPw. Colored boxes represent disturbance severity, or percent gross defoliation, which is repeated in each stratum for years 2019–2021. Non-overlapping letters in (B) indicate significant pairwise differences between disturbance severities (p < 0.05). In the upper canopy and both strata combined, there was no significant effect of disturbance severity (A,C).
Canopy structural response to disturbance
Mean VAI displayed greater change over time and less resistance to disturbance treatments than canopy rugosity. VAI declined significantly from 2018 (pre-disturbance) to 2021 at 65% (p = 0.02) and 85% (p = 0.005) disturbance severity levels (Figure 4A). Mean VAI resistance was only significantly different from 0 in the 65 % disturbance severity treatment where mean resistance was −0.30 ± 0.12 (p = 0.04, Figure 4B). In contrast, there were no significant changes in canopy rugosity from 2018 to 2021 at any disturbance severity (p = 0.07) or between top-down/bottom up disturbance types (p = 0.08, Figure 4C). Mean canopy rugosity resistance was comparable among disturbance severity and types, averaging 0.08 ± 0.09 (p > 0.05, Figure 4D). Change-based and resistance metrics, therefore, suggest that mean (experiment-wide) VAI legacies were eroded, while material legacies associated with structural complexity remained relatively unchanged.
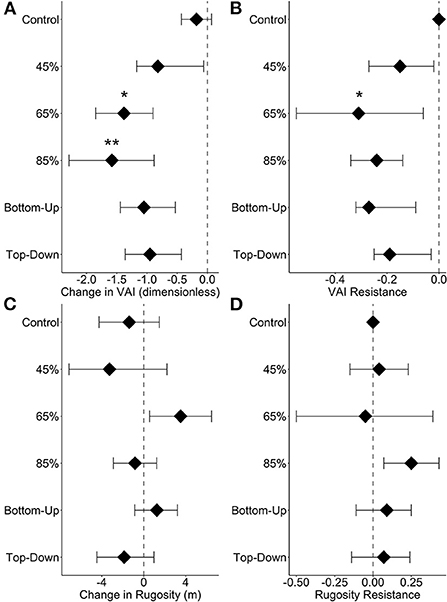
Figure 4. Comparisons of mean vegetation area index (VAI) from 2018 to 2021 (A) and VAI resistance (the log ratio of experimental VAI resistance compared to control VAI resistance) (B). Then, comparisons of mean canopy rugosity from 2018 to 2021 (C) and rugosity resistance (the log ratio of experimental rugosity resistance compared to control rugosity resistance) (D). Bars represent 95% confidence intervals. Significant differences from the control plots are indicated by a single star (p < 0.05) or two stars (p < 0.01) (A, C). Vertical lines at 0.0 indicate no change over time in panels A and C; vertical lines at 0.0 indicate resistance values equal to the control in panels B and D.
Structure-production resistance and change relationships
At the subplot scale, post-disturbance relationships between ANPPw and canopy structure differed for VAI and canopy rugosity, and were dependent upon whether change or resistance measures were assessed. Subplot ANPPw change was not correlated with either VAI (p = 0.98, Figure 5A) or canopy rugosity (p = 0.37, Figure 5C) changes from 2018 to 2021. In contrast, ANPPw resistance was positively related to canopy rugosity resistance (Adj. R2 = 0.56, p < 0.001, Figure 5D), but not VAI resistance (Adj. R2 = 0.02, p = 0.23, Figure 5B), irrespective of disturbance severity or treatment type. These results suggest that extensive subplot variation in structure and ANPPw associated with pre-existing differences in site productivity, biomass, soils, and vegetation communities rather than systematic responses to disturbance severity or type drove interactions between structure and production.
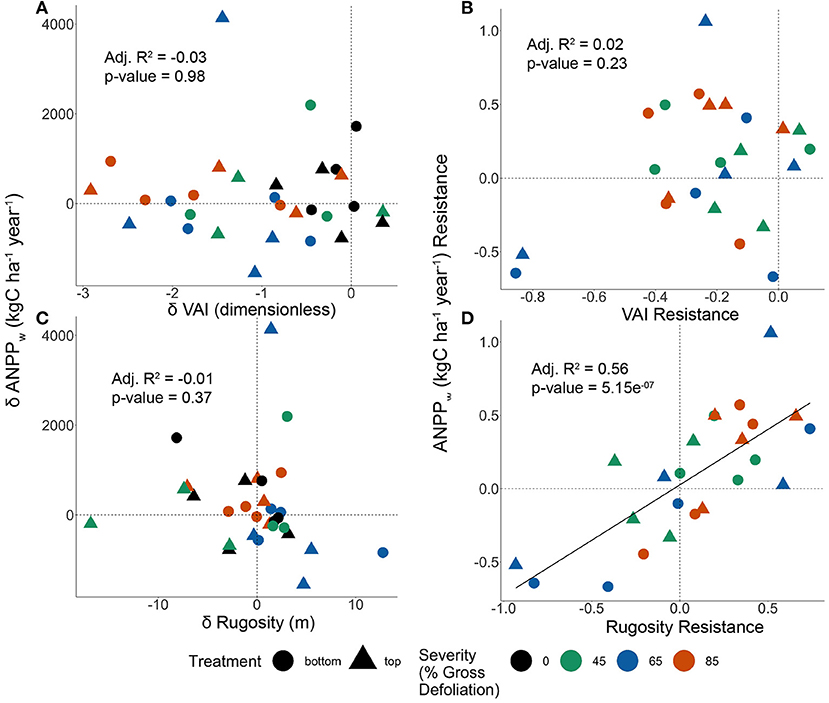
Figure 5. Linear regressions relating change in vegetation area index (VAI) to change in aboveground wood net primary production (ANPPw) (A); VAI resistance to ANPPw resistance (B); change in canopy rugosity to change in ANPPw (C); and rugosity resistance to ANPPw resistance (D). A solid trendline is included when a significant relationship is present (p < 0.05) (A,C). Where changes in structural metrics over time are shown, a decrease in structural metric (x < 0) over time represents structures that existed before disturbance that remain 3 years after, while an increase in structural metric (x > 0) over time represents structures that were generated by disturbance (B,D). Where structural resistance is shown, a decrease in structural metric relative to the control (x < 0) is designated as net negative resistance, while an increase in structural resistance (x > 0) is designated as net positive resistance.
Discussion
Our analysis provides insight into the relationships between forest structural material legacies and ANPPw in the years following experimental disturbance varying by severity and source. Three years after stem-girdling, total ANPPw remained virtually unchanged, despite significant losses of VAI at higher disturbance severities. The growth of legacy subcanopy and upper canopy trees fully compensated for losses in ANPPw, even at the highest disturbance severities. Experiment-wide, mean VAI declined in response to high severity disturbance treatments, while canopy rugosity was less sensitive to disturbance; however, large subplot-to-subplot variation points to substantial small-scale disturbance response differences. Subplot canopy rugosity and ANPPw, resistance values were correlated, irrespective of disturbance treatment. These findings indicate that forest structural material legacies sustained total ANPPw following disturbance in three synergistic ways, maintaining upper canopy ANPPw, stimulating subcanopy ANPPw at levels commensurate with the degree of disturbance severity, and supporting ANPPw resistance through the retention or accrual of structural complexity.
A key finding is that the growth of legacy upper canopy and subcanopy vegetation fully offset the declining growth and mortality of stem-girdled trees, stabilizing total ANPPw at gross defoliation levels of 85%. Moreover, the top-down and bottom-up disturbances exhibited comparably high levels of ANPPw resistance, indicating that structural legacies originating from different locations within the canopy compensated equally well for phloem-disruption. While system-wide production was stable, the mechanisms underlying this stability changed over time. In the first year following disturbance, girdled and ungirdled trees exhibited comparable radial stem growth (Grigri et al., 2020, Figure 3A), a phenomenon associated with the accumulation of non-structural carbohydrates above the girdle following phloem-disruption (Regier et al., 2010; Mei et al., 2015). As the growth of girdled trees tapered, production in the upper canopy was sustained by healthy tree growth. At the same time, we observed a significant compensatory rise in subcanopy ANPPw (Figure 3B), likely stimulated by a gradual multi-year increase in canopy gap formation and increased light availability, especially at the highest severities (Hanson and Lorimer, 2007; Campbell et al., 2009; Muscolo et al., 2014; Stuart-Haëntjens et al., 2015; Fahey et al., 2016). The subcanopy ANPPw response intensified over time, lagging the initiation of disturbance. These results indicate that the canopy stratum and physiology underlying compensatory growth was dynamic and sufficiently flexible to overcome progressive tree declines over time across a range of disturbance severities and types. However, the high ANPPw stability that we observed may not occur when tree mortality unfolds more rapidly (Breshears and Allen, 2002) or when crucial material legacies are completely eliminated (Harvey et al., 2016). Instead, our findings highlight the adaptive response of forests in the event of slowly-unfolding disturbances caused by phloem-feeding insects or defoliating disturbances from pests and pathogens.
We found that canopy rugosity and VAI exhibited different degrees of change and resistance following disturbance. Aligned with our hypothesis, we observed a decline in VAI, especially at the highest disturbance severities, as tree decline caused gradual defoliation and crown degradation. At the highest disturbance severities (65 and 85% gross defoliation), VAI declined, as expected, in response to treatments targeting different gross defoliation levels, similar to disturbance responses elsewhere (Kashian et al., 2005; Peters et al., 2013). The resulting change over time and reduction relative to the control in VAI forced experiment-wide declines in this measure of vegetation quantity across disturbance treatments (Figures 4A,B). In contrast, the number of subplots gaining and losing canopy complexity (i.e., rugosity) was approximately balanced, irrespective of disturbance severity or treatment type (Figure 4C). As a result, mean canopy rugosity experiment-wide changed little but exhibited high variability at the smaller spatial scale (Figure 5C). Our findings that disturbance exerts variable effects on small spatial-scale canopy rugosity are congruent with studies demonstrating that moderate severity disturbances can enhance or erode structural complexity by introducing or decreasing, respectively, variation in the 3-dimensional arrangement of vegetation (Meigs and Keeton, 2018; Peterson, 2019; Atkins et al., 2020; Gough and Tallant, 2022). In our study, the effects of disturbance on complexity were not systematic, however, suggesting that additional site factors such as pre-disturbance productivity, biomass, and community composition interacted with disturbance to reshape structure. Together, these findings suggest the definition of material legacies be expanded to acknowledge that disturbance may precipitate net losses (e.g., number of live stems) or increases (e.g., coarse woody debris) of pooled individuals and resources with ties to ecosystem functioning (Johnstone et al., 2016).
Lastly, we found that ANPPw's resistance to disturbance was directly coupled with the resistance of canopy rugosity but not VAI, indicating that small spatial scale variation in structural complexity strongly predicted the response of a key ecosystem function. Previous work has shown positive relationships between wood or total NPP and leaf area index (LAI) (Scheuermann et al., 2018), canopy rugosity (Hardiman et al., 2011; Fotis et al., 2018; Gough et al., 2019, 2021a), tree density and size (Seidl et al., 2012), and species diversity (Silva Pedro et al., 2015); however, our analysis is among the first to show that the normalized disturbance responses (i.e., resistances) of complexity and primary production parallel one another. The significance of this finding is three-fold. First, it supports theoretical expectations (Mathes et al., 2021) that the use of normalized log ratios provides a more sensitive test of disturbance response than pre- to post- comparisons because the relativized (treatment:control) response eliminates variability associated with the difference in response magnitudes of the variable of interest (Hillebrand et al., 2018). This normalization may have been particularly relevant to the context of our study, which encompassed landscape ecosystems varying substantially in pre-disturbance composition and productivity (Gough et al., 2021b). Secondly, and related, change metrics incorporate temporal variability that is not driven solely by disturbance, whereas normalized resistance metrics generated by comparing treatment and control responses account for year-to-year variation explained by other factors such as climate (Mathes et al., 2021). Third, in the application, these findings suggest that forest stands managed for enhanced structural complexity could sustain higher rates of primary production following disturbance (Evans and Perschel, 2009; Puettmann et al., 2009; D'Amato et al., 2011; D'amato and Palik, 2021). As a result, indices of canopy structural complexity may be useful guide for the adaptive management of disturbance response (Fahey et al., 2016).
Conclusion
We conclude that material legacies in the form of canopy structure play a significant role in the stabilization of wood net primary production following a phloem-girdling disturbance. Our analysis shows that forests exhibiting high structural resistance may be more functionally resistant to moderate-to-severe slow-acting disturbances, but that the measure of canopy structure (i.e., VAI vs. canopy rugosity) and the analytical approach employed (temporal change vs. resistance) are influential. In addition, we found that an intact subcanopy was critical for compensatory growth to offset primary losses in real-time as upper canopy tree growth waned (Stuart-Haëntjens et al., 2015; Fahey et al., 2016), underscoring the importance of material legacies in the form of an intact vegetated subcanopy. Finally, we advocate for an expanded definition of “material legacies” that includes stand-to-landscape scale changes in pooled biotic or abiotic materials. This broader definition is compatible with the basic tenets of material legacy theory (sensu Johnstone et al., 2016) and would acknowledge that materials and resources crucial to ecosystem functioning may decrease or increase following disturbance (Taboada et al., 2018). Such broader inclusion would parallel recent extensions of stability theory, which acknowledge the potential for negative and positive resistances (Hillebrand et al., 2018; Mathes et al., 2021), for example, in the case of higher primary production following disturbance (Stuart-Haëntjens et al., 2015; Williams et al., 2017). We suggest that continued dialogue and interaction among and within the complementary scholarly communities studying material legacies and stability theory will help expand the relevance and application of these frameworks; standardize analytical frameworks; and harmonize terminology and concepts.
Data availability statement
The datasets presented in this study can be found in online repositories. The names of the repository/repositories and accession number(s) can be found in the article/Supplementary Material.
Author contributions
Original draft preparation: KN. Supervision: CG. Data analysis and methodology: JA and MG. Conceptualization and editing: BB-L. All authors have read and agreed to the published version of this manuscript.
Funding
This research was supported by the National Science Foundation, Division of Environmental Biology, Award 1655095. We thank the University of Michigan Biological Station for logistical support.
Acknowledgments
The authors would like to acknowledge the help with field work, especially from Alex Marini, Tess Belleville, Cameron Clay, and Carol-Anne Petit for assistance with data collection. We would also like to thanks the Burt Lake Band of Ottawa and Chippewa Indians, Lisa Haber, Kayla Mathes, and Laura Hickey for their direct and indirect support of this project.
Conflict of interest
The authors declare that the research was conducted in the absence of any commercial or financial relationships that could be construed as a potential conflict of interest.
Publisher's note
All claims expressed in this article are solely those of the authors and do not necessarily represent those of their affiliated organizations, or those of the publisher, the editors and the reviewers. Any product that may be evaluated in this article, or claim that may be made by its manufacturer, is not guaranteed or endorsed by the publisher.
Supplementary material
The Supplementary Material for this article can be found online at: https://www.frontiersin.org/articles/10.3389/ffgc.2022.941851/full#supplementary-material
References
Atkins, J. W., Agee, E., Barry, A., Dahlin, K. M., Dorheim, K., Grigri, M. S., et al. (2021). The fortedata R package: open-science datasets from a manipulative experiment testing forest resilience. Earth System Science Data 13, 943–952. doi: 10.5194/essd-13-943-2021
Atkins, J. W., Bohrer, G., Fahey, R. T., Hardiman, B. S., Morin, T. H., Stovall, A. E. L., et al. (2018). Quantifying vegetation and canopy structural complexity from terrestrial LiDAR data using the forestr r package. Methods Ecol. Evol. 9, 2057–2066. doi: 10.1111/2041-210X.13061
Atkins, J. W., Bond-Lamberty, B., Fahey, R. T., Haber, L. T., Stuart-Haëntjens, E., Hardiman, B. S., et al. (2020). Application of multidimensional structural characterization to detect and describe moderate forest disturbance. Ecosphere 11, 3156. doi: 10.1002/ecs2.3156
Breshears, D. D., and Allen, C. D. (2002). The importance of rapid, disturbance-induced losses in carbon management and sequestration. Global Ecol. Biogeogr. 11, 1–5. doi: 10.1046/j.1466-822X.2002.00274.x
Campbell, J., Alberti, G., Martin, J., and Law, B. E. (2009). Carbon dynamics of a ponderosa pine plantation following a thinning treatment in the northern Sierra Nevada. Forest Ecol. Manag. 257, 453–463. doi: 10.1016/j.foreco.2008.09.021
Cohen, W. B., Yang, Z., Stehman, S. V., Schroeder, T. A., Bell, D. M., Masek, J. G., et al. (2016). Forest disturbance across the conterminous United States from 1985-2012: The emerging dominance of forest decline. Forest Ecol. Manag. 360, 242–252. doi: 10.1016/j.foreco.2015.10.042
Cooper, A. W. (1981). “Above-ground biomass accumulation and net primary production during the first 70 years of succession in populus grandidentata stands on poor sites in northern lower Michigan,” in Forest Succession. Springer Advanced Texts in Life Sciences, eds D.C. West, H. H. Shugart, and D. B. Botkin (New York, NY: Springer). doi: 10.1007/978-1-4612-5950-3_21
D'Amato, A. W., Bradford, J. B., Fraver, S., and Palik, B. J. (2011). Forest management for mitigation and adaptation to climate change: insights from long-term silviculture experiments. Forest Ecol. Manag. 262, 803–816. doi: 10.1016/j.foreco.2011.05.014
D'amato, A. W., and Palik, B. J. (2021). Building on the last “new” thing: exploring the compatibility of ecological and adaptation silviculture. NRC Res. Press 51, 172–180. doi: 10.1139/cjfr-2020-0306
de Mendiburu, F. (2020). Agricolae: Statistical Procedures for Agricultural Research. R package version 1.3-3. Available online at: https://CRAN.R-project.org/package=agricolae (accessed June 06, 2021).
Engelken, P. J., Benbow, M. E., and McCullough, D. G. (2020). Legacy effects of emerald ash borer on riparian forest vegetation and structure. Forest Ecol. Manag. 457, 117684. doi: 10.1016/j.foreco.2019.117684
Evans, A. M., and Perschel, R. (2009). A review of forestry mitigation and adaptation strategies in the Northeast U.S. Climatic Change 96, 167–183. doi: 10.1007/s10584-009-9569-3
Fahey, R. T., Stuart-Haëntjens, E. J., Gough, C. M., de La Cruz, A., Stockton, E., Vogel, C. S., et al. (2016). Evaluating forest subcanopy response to moderate severity disturbance and contribution to ecosystem-level productivity and resilience. Forest Ecol. Manag. 376, 135–147. doi: 10.1016/j.foreco.2016.06.001
Fotis, A. T., Morin, T. H., Fahey, R. T., Hardiman, B. S., Bohrer, G., and Curtis, P. S. (2018). Forest structure in space and time: biotic and abiotic determinants of canopy complexity and their effects on net primary productivity. Agric. Forest Meteorol. 250, 181–191. doi: 10.1016/j.agrformet.2017.12.251
Franklin, J. F., Lindenmayer, D., MacMahon, J. A., McKee, A., Magnuson, J., Perry, D. A., et al. (2000). Threads of continuity. Conserv. Biol. Pract. 1, 8–16. doi: 10.1111/j.1526-4629.2000.tb00155.x
Gough, C. M., Atkins, J. W., Bond-Lamberty, B., Agee, E. A., Dorheim, K. R., Fahey, R. T., et al. (2021a). Forest structural complexity and biomass predict first-year carbon cycling responses to disturbance. Ecosystems 24, 699–712. doi: 10.1007/s10021-020-00544-1
Gough, C. M., Atkins, J. W., Fahey, R. T., and Hardiman, B. S. (2019). High rates of primary production in structurally complex forests. Ecology 100, 1–6. doi: 10.1002/ecy.2864
Gough, C. M., Atkins, J. W., Fahey, R. T., Hardiman, B. S., and LaRue, E. A. (2020). Community and structural constraints on the complexity of eastern North American forests. Global Ecol. Biogeogr. 29, 2107–2118. doi: 10.1111/geb.13180
Gough, C. M., Bohrer, G., Hardiman, B. S., Nave, L. E., Vogel, C. S., Atkins, J. W., et al. (2021b). Disturbance-accelerated succession increases the production of a temperate forest. Ecol. Appl. 31, e02417. doi: 10.1002/eap.2417
Gough, C. M., Flower, C. E., Vogel, C. S., Dragoni, D., and Curtis, P. S. (2009). Whole-ecosystem labile carbon production in a north temperate deciduous forest. Agric. Forest Meteorol. 149, 1531–1540. doi: 10.1016/j.agrformet.2009.04.006
Gough, C. M., and Tallant, J. (2022). Inferring the effects of partial defoliation on the carbon cycle from forest structure: challenges and opportunities Boreal Water Flux (PhD Thesis).
Gough, C. M., Vogel, C. S., Hardiman, B., and Curtis, P. S. (2010). Wood net primary production resilience in an unmanaged forest transitioning from early to middle succession. Forest Ecol. Manag. 260, 36–41. doi: 10.1016/j.foreco.2010.03.027
Gough, G., Vogel, C., Schmid, H., and Curtis, P. (2008). Controls on annual forest carbon storage: lessons from the past and predictions for the future. BioScience 58, 609–622. doi: 10.1641/B580708
Grigri, M. S., Atkins, J. W., Vogel, C., Bond-Lamberty, B., and Gough, C. M. (2020). Aboveground wood production is sustained in the first growing season after phloem-disrupting disturbance. Forests 11, 1–19. doi: 10.3390/f11121306
Haber, L. T., Fahey, R. T., Wales, S. B., Correa Pascuas, N., Currie, W. S., Hardiman, B. S., et al. (2020). Forest structure, diversity, and primary production in relation to disturbance severity. Ecol. Evol. 10, 4419–4430. doi: 10.1002/ece3.6209
Hanson, J. J., and Lorimer, C. G. (2007). Forest structure and light regimes following moderate wind storms: implications for multi-cohort management. Ecol. Appl. 17, 1325–1340. doi: 10.1890/06-1067.1
Hardiman, B. S., Bohrer, G., Gough, C. M., Vogel, C. S., and Curtis, P. S. (2011). The role of canopy structural complexity in wood net primary production of a maturing northern deciduous forest. Ecology 92, 1818–1827. doi: 10.1890/10-2192.1
Hardiman, B. S., Gough, C. M., Halperin, A., Hofmeister, K. L., Nave, L. E., Bohrer, G., et al. (2013). Maintaining high rates of carbon storage in old forests: a mechanism linking canopy structure to forest function. Forest Ecol. Manag. 298, 111–119. doi: 10.1016/j.foreco.2013.02.031
Harris, L. B., Drury, S. A., and Taylor, A. H. (2021). Strong legacy effects of prior burn severity on forest resilience to a high-severity fire. Ecosystems 24, 774–787. doi: 10.1007/s10021-020-00548-x
Harvey, B. J., Donato, D. C., and Turner, M. G. (2016). High and dry: post-fire tree seedling establishment in subalpine forests decreases with post-fire drought and large stand-replacing burn patches. Global. Ecol. Biogeogr. 25, 655–669. doi: 10.1111/geb.12443
Hicke, J. A., Allen, C. D., Desai, A. R., Dietze, M. C., Hall, R. J., Hogg, E. H. T., et al. (2012). Effects of biotic disturbances on forest carbon cycling in the United States and Canada. Global Change Biol. 18, 7–34. doi: 10.1111/j.1365-2486.2011.02543.x
Hillebrand, H., Langenheder, S., Lebret, K., Lindström, E., Östman, Ö., and Striebel, M. (2018). Decomposing multiple dimensions of stability in global change experiments. Ecol. Lett. 21, 21–30. doi: 10.1111/ele.12867
Johnstone, J. F., Allen, C. D., Franklin, J. F., Frelich, L. E., Harvey, B. J., Higuera, P. E., et al. (2016). Changing disturbance regimes, ecological memory, and forest resilience. Front. Ecol. Environ. 14, 369–378. doi: 10.1002/fee.1311
Kashian, D. M., Turner, M. G., and Romme, W. H. (2005). Variability in leaf area and stemwood increment along a 300-year lodgepole pine chronosequence. Ecosystems 8, 48–61. doi: 10.1007/s10021-004-0067-1
Mathes, K. C., Ju, Y., Kleinke, C., Oldfield, C., Bohrer, G., Bond-Lamberty, B., et al. (2021). A multidimensional stability framework enhances interpretation and comparison of carbon cycling response to disturbance. Ecosphere 12, 1–12. doi: 10.1002/ecs2.3800
Mei, L., Xiong, Y., Gu, J., Wang, Z., and Guo, D. (2015). Whole-tree dynamics of non-structural carbohydrate and nitrogen pools across different seasons and in response to girdling in two temperate trees. Oecologia 177, 333–344. doi: 10.1007/s00442-014-3186-1
Meigs, G. W., and Keeton, W. S. (2018). Intermediate-severity wind disturbance in mature temperate forests: legacy structure, carbon storage, and stand dynamics. Ecol. Appl. 28, 7980815. doi: 10.1002/eap.1691
Muscolo, A., Bagnato, S., Sidari, M., and Mercurio, R. (2014). A review of the roles of forest canopy gaps. J. Forestry Res. 25, 725–736. doi: 10.1007/s11676-014-0521-7
Nave, L., Marín-Spiotta, E., Ontl, T., Peters, M., and Swanston, C. (2019). Soil carbon management. Global Change Forest Soils. 36, 215–257. doi: 10.1016/B.978-0-444-63998-1.00011-2
NOAA (2022). National Centers for Environmental information, Climate at a Glance: Global Mapping. Available online at: https://www.ncdc.noaa.gov/cag/ (accessed March 24, 2022).
Pan, Y., Birdsey, R. A., Fang, J., Houghton, R., Kauppi, P. E., Kurz, W. A., et al. (2011). A large and persistent carbon sink in the world's forests. Science 333, 988–993. doi: 10.1126/science.1201609
Pearsall, D., Barnes, B. V., Zogg, G. R., Lapin, M., and Ring, R. R. (1995). Landscape Ecosystems of the University of Michigan Biological Station Vol. 66. Ann Arbor, MI: School of Natural Resources and Environment.
Peters, E. B., Wythers, K. R., Bradford, J. B., and Reich, P. B. (2013). Influence of disturbance on temperate forest productivity. Ecosystems 16, 95–110. doi: 10.1007/s10021-012-9599-y
Peterson, C. J. (2019). Damage diversity as a metric of structural complexity after forest wind disturbance. Forests. 10, 85. doi: 10.3390/f10020085
Puettmann, K., Coates, K., and Messier, C. (2009). A Critique of Silviculture: Managing for Complexity. Washington, DC: Island Press.
Radchuk, V., Laender, F., Cabral, J. S., Boulangeat, I., Crawford, M., Bohn, F., et al. (2019). The dimensionality of stability depends on disturbance type. Ecol. Lett. 22, 674–684. doi: 10.1111/ele.13226
Regier, N., Streb, S., Zeeman, S. C., and Frey, B. (2010). Seasonal changes in starch and sugar content of poplar (Populus deltoides × nigra cv. Dorskamp) and the impact of stem girdling on carbohydrate allocation to roots. Tree Physiol. 30, 979–987. doi: 10.1093/treephys/tpq047
Royo, A. A., Stout, S. L., deCalesta, D. S., and Pierson, T. G. (2010). Restoring forest herb communities through landscape-level deer herd reductions: is recovery limited by legacy effects? Biol. Conserv. 143, 2425–2434. doi: 10.1016/j.biocon.2010.05.020
Running, S. W. (2008). Ecosystem disturbance, carbon, and climate. Science 321, 652–653. doi: 10.1126/science.1159607
Scheuermann, C. M., Nave, L. E., Fahey, R. T., Nadelhoffer, K. J., and Gough, C. M. (2018). Effects of canopy structure and species diversity on primary production in upper Great Lakes forests. Oecologia 188, 405–415. doi: 10.1007/s00442-018-4236-x
Seidl, R., Rammer, W., and Spies, T. A. (2014a). Disturbance legacies increase the resilience of forest ecosystem structure, composition, and functioning Europe PMC Funders Group. Ecol. Appl. 24, 2063–2077. doi: 10.1890/14-0255.1
Seidl, R., Schelhaas, M.-J., Rammer, W., and Verkerk, P. J. (2014b). Increasing forest disturbances in Europe and their impact on carbon storage. Nature Climate Change 4, 806–810. doi: 10.1038/nclimate2318
Seidl, R., Spies, T. A., Rammer, W., Steel, E.A., Pabst, R.J. and Olsen, K. (2012). Multi-scale drivers of spatial variation in old-growth forest carbon density disentangled with lidar and an individual-based landscape model. Ecosystems 15, 1321–1335. doi: 10.1007/s10021-012-9587-2
Silva Pedro, M., Rammer, W., and Seidl, R. (2015). Tree species diversity mitigates disturbance impacts on the forest carbon cycle. Oecologia 177, 619–630. doi: 10.1007/s00442-014-3150-0
Stuart-Haëntjens, E. J., Curtis, P. S., Fahey, R. T., Vogel, C. S., and Gough, C. M. (2015). Net primary production of a temperate deciduous forest exhibits a threshold response to increasing disturbance severity. Ecology 96, 2478–2487. doi: 10.1890/14-1810.1
Taboada, A., Fernández-García, V., Marcos, E., and Calvo, L. (2018). Interactions between large high-severity fires and salvage logging on a short return interval reduce the regrowth of fire-prone serotinous forests. Forest Ecol. Manag. 414, 54–63. doi: 10.1016/j.foreco.2018.02.013
Turner, M. G., Baker, W. L., Peterson, C. J., and Peet, R. K. (1998). Factors influencing succession: lessons from large, infrequent natural disturbances. Ecosystems 1, 511–523. doi: 10.1007/s100219900047
Keywords: disturbance, material legacy, ecosystem stability, resistance, net primary production, lidar—remote sensing, canopy structure, rugosity
Citation: Niedermaier KM, Atkins JW, Grigri MS, Bond-Lamberty B and Gough CM (2022) Structural complexity and primary production resistance are coupled in a temperate forest. Front. For. Glob. Change 5:941851. doi: 10.3389/ffgc.2022.941851
Received: 11 May 2022; Accepted: 27 June 2022;
Published: 19 July 2022.
Edited by:
Siyan Ma, University of California, Berkeley, United StatesReviewed by:
Marcy Litvak, University of New Mexico, United StatesMark Vanderwel, University of Regina, Canada
Copyright © 2022 Niedermaier, Atkins, Grigri, Bond-Lamberty and Gough. This is an open-access article distributed under the terms of the Creative Commons Attribution License (CC BY). The use, distribution or reproduction in other forums is permitted, provided the original author(s) and the copyright owner(s) are credited and that the original publication in this journal is cited, in accordance with accepted academic practice. No use, distribution or reproduction is permitted which does not comply with these terms.
*Correspondence: Kerstin M. Niedermaier, bmllZGVybWFpa20mI3gwMDA0MDt2Y3UuZWR1