- 1Departamento de Ecología, Universidade Federal do Rio Grande do Norte, UFRN, Natal, Brazil
- 2Laboratório de Ecologia da Restauração, Universidade Federal do Rio Grande do Norte, UFRN, Natal, Brazil
- 3Departamento de Biologia Vegetal, Universidade Estadual de Campinas, UNICAMP, Campinas, Brazil
Plant functional strategies are well-established for low- and high-stress environments, such as rainforests and deserts. However, in environments with low- and high-stress level fluctuation within years, the relationship between plant functional strategies and their spatial distribution is still poorly understood. We aimed to answer: what are the relationships between above- and below-ground traits in the largest seasonally dry tropical forest in the Americas? Do the studied species form detectable groups from the functional perspective? If detectable, do functional groups present distinct spatial distributions across the domain, mediated by spatial heterogeneity of aridity? We sampled a range of 16 above- and below-ground traits from the 20 most common native tree species. We performed a PCA to understand the species' main coordinated trade-offs, a k-mean analysis to test for functional groups, and a Ripley's-K analysis followed by a GLS model to test spatial functional groups distribution through the aridity gradient. We found five coordinated trade-offs representing different aspects of the conservative-acquisitive strategy continuum. Drought-tolerance and avoidance mechanisms seem linked to the conservative-acquisitive gradient, where water storage is positively correlated with acquisitive strategies. Different from other seasonally dry regions, acquisitive strategies are not limited by aridity. The presence of short-term water storage traits might buffer rainfall fluctuations, allowing acquisitive species to occupy more arid regions. This study sheds new light on the functional complexity of species from Americas seasonally dry tropical forests, for the first time including the relationship of its below- and above-ground traits.
Introduction
How the plant functional strategies evolved to allow colonization and survival under different environmental conditions is a topic of worldwide interest in plant ecology (Kattge et al., 2011). General classification schemes have proposed that plant species should be distributed along a gradient of conservative to acquisitive strategies (Grime, 1977; Westoby, 1998; Diaz et al., 2016). Conservative species have a high capacity to handle limited resources in stressful conditions due to the presence of functional traits that minimize resource loss and acquisition rates (e.g., low specific leaf area, high wood densities, and narrow vessel diameter), leading to slow but safe growth patterns (Chave et al., 2000; Reich, 2014). Conversely, acquisitive plant species present coordinated functional traits that maximize plant resource acquisition rates, leading to a fast but vulnerable growth pattern (e.g., high specific leaf area, low wood densities, and wide vessel diameter). On a global scale, environments with high-stress conditions select for conservative species, while environments with low-stress levels select acquisitive plant species on a continuum of conservative-acquisitive growth strategies (Pierce et al., 2013; Reich, 2014).
However, some biomes, such as seasonally dry tropical forests (SDTF), have seasonal cycles alternating between high- and low-stress conditions within a year. Annually, the periods of high stress are characterized by several months of drought and high temperatures, while favorable periods are represented by shorter intervals of higher water availability and lower temperatures (Pennington et al., 2009). During the dry season, the abiotic stress exposes plants to conditions far from their physiological optimum (Domec et al., 2006; Martínez-Vilalta et al., 2014), substantially decreasing their survival and growth (Engelbrecht and Kursar, 2003). Although dry climates generally favor the establishment of conservative species (Cornwell and Ackerly, 2009; Costa-Saura et al., 2016), the rainy season can promote favorable conditions for the establishment of acquisitive species (Chesson et al., 2004).
Deciduousness is a common trait exhibited by trees in SDTF. By losing leaves at the beginning of the dry season, deciduous species limit their activity to the rainy season, avoiding embolism and hydraulic damage during drought (Poorter and Markesteijn, 2008; de Lima et al., 2021). To be able to maintain an active metabolism during stressful periods, late deciduous or evergreen species depend on traits and trait coordination to avoid the drying out of tissues. This has been produced through the selection of ecological strategies combining higher wood density (Eamus, 1999; Brodribb et al., 2003; Lima et al., 2012; Oliveira et al., 2015), lower hydraulic conductivity (Markesteijn et al., 2011a; Méndez-Alonzo et al., 2012), and smaller leaf size (Markesteijn et al., 2011b). On the contrary, for species with acquisitive characteristics to withstand seasonal drought and successfully photosynthesise quickly in the early rains, it is necessary not only for an early deciduousness but also traits that mitigate water loss during their activity, such as a higher water transport efficiency and higher efficiency in closing stomata (Markesteijn et al., 2011b; Sterck et al., 2011).
It may seem intuitive to expect that acquisitive species occurrence may be limited to moister sections along drought gradients (Engelbrecht et al., 2007; Frenette-Dussault et al., 2012; Esquivel-Muelbert et al., 2016). However, Sterck et al. (2011) found no relationship between the distribution of strategies along a drought gradient in mathematical models, and work conducted in situ, such as that of Pinho et al. (2019) and de Lima et al. (2021), showed that contrary to expected, acquisitive species in seasonally dry tropical forests might prevail at areas with higher aridity.
The seasonally dry tropical forest is a complex environment with important and large areas still insufficiently studied from a functional perspective, such as the largest SDTF of the Americas, the Caatinga (Leal et al., 2005; Albuquerque et al., 2012). This system holds vast biodiversity and a high level of species endemism, and is under great threat (Antongiovanni et al., 2018). In this sense, studies that elucidate the complexity and functional diversity of these environments are of extreme importance in terms of scientific novelty and support of ecological management. To fully understand the spectrum of functional strategies selected by this seasonal stress dynamic, it is necessary to consider multiple functional traits, their coordinated relations, and their spatial distribution. In this work, we studied 16 key morphological and biochemical functional traits of 20 ecologically important and abundant tree species of the Brazilian SDTF to respond to the following questions: (i) What are the relationships between leaf, stem, and root morphological and biochemical functional traits in Caatinga tree species? (ii) Do the studied species form detectable groups from the functional perspective? and (iii) If detectable, do functional groups present distinct spatial distributions across the Caatinga domain, mediated by variations in aridity? We expected to find an association between drought-avoidance and acquisitive strategies where species that present short canopy duration will also present a high specific leaf area, low wood density, high hydraulic weighted diameter, low water use efficiency, and a high specific root length representing the acquisitive side of the plants' functional spectrum. We also expect that conservative and acquisitive species will be evenly distributed throughout the domain, where in areas of higher aridity, acquisitive strategies will prevail, but their activity will be restricted to rainfall periods.
Methods
Study area
The study was conducted in the Caatinga Forest domain, the largest and most diverse seasonally dry tropical forest in the Americas (Pennington et al., 2009; Moro et al., 2016). The domain covers 826,411 km2, occupying 11% of the Brazilian territory (da Silva et al., 2017). The Caatinga is an exclusive Brazilian domain with rich soil and a high degree of endemic plant and animal species (Leal et al., 2005). Its vegetation varies in structure within the biome, from sparsely woody and cactaceous vegetation to forests. The Caatinga SDTF is fragmented, with approximately 50% of its original area suffering from degradation (Antongiovanni et al., 2018), while the remaining 50% continues in a state of chronic disturbance, caused by deforestation, urban infrastructure, grazing, and fire (Santos et al., 2015; Ribeiro et al., 2016; Antongiovanni et al., 2020).
Trait surveys were performed at the Açu National Forest (Rio Grande do Norte, Brazil). The National Forest encompasses 528 ha, where vegetation is characterized by trees varying in height between 2 and 15 m. The soil of this region is geologically characterized by crystalline plains, mostly flat in relief and rich in nutrients, although shallow and with a constant presence of stones (Moro et al., 2016). The vegetational structure of the National Forest region is known as the “São Francisco and Sertaneja depressions” (Silva and Souza, 2018). It is one of the driest regions of the domain, with a mean annual temperature of 24°C and a mean annual precipitation of 607 mm. In the last decade, the study site specifically presented on average during the rainy season 27.76°C (max 28.36°C and min 27.06°C) mean temperature and 84.09 mm/year (max 677.3 mm/year and min 221.6 mm/year) precipitation, and 27.58°C (max 28.07°C and min 27.07°C) mean temperature and 8.06 mm/year (max 113.1 mm/year and min 23.1 mm/year) precipitation during the dry season (data obtained from the Google Earth (sf) application of the Climate Research Unity database (Harris et al., 2014), with coordinates latitude −5.75 and longitude −36.75).
Species and individual selection
We selected 20 native tree species out of the total pool of 40 species registered for the National Forest of Açu (Lira et al., 2010; de Amorim et al., 2016), although the domain contains more than 886 tree species registered (BFG, 2015). All tree species selected (Table 1) are abundant in the National Forest and present a wide distribution over the domain, with some species extending their distribution to neighboring domains. We marked 15 individual trees from each species in a circular area of 1 km diameter range. The trees met the criteria of being an adult (with a robust structure of diameter (minimum 30 cm of diameter at soil height) and height (minimum of 3 m), and reproductive for at least 7 years) and being isolated from other trees to ensure that the samples taken from below ground were collected from the specifically selected tree. From all individuals marked, we randomly selected three trees from each species to be sampled for trait collection and measurement.
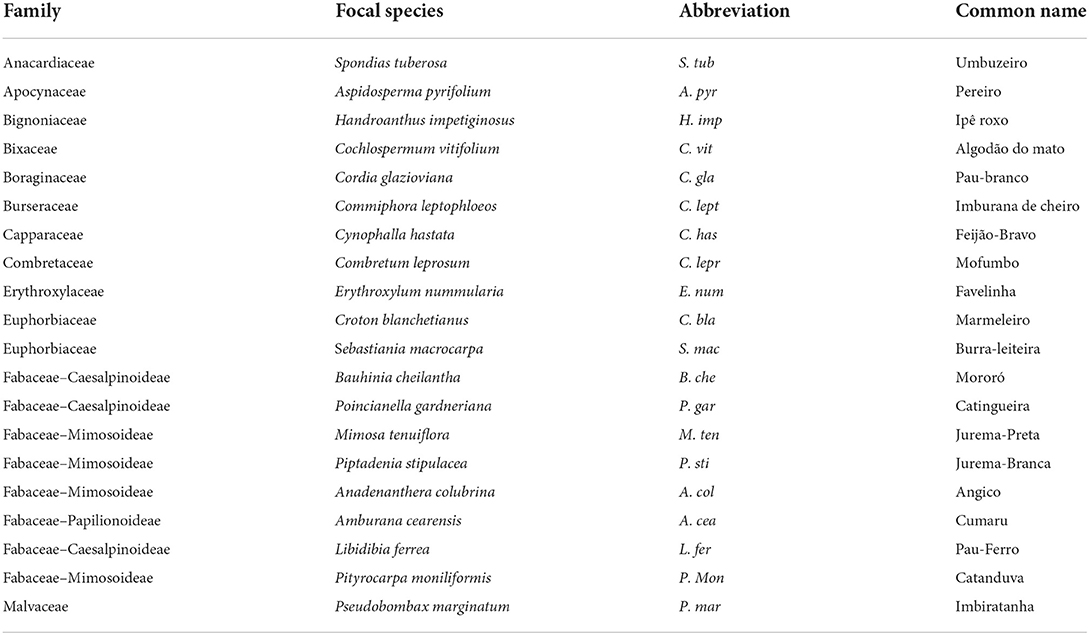
Table 1. Information of scientific name and popular name of the studied trees from the Caatinga seasonally dry tropical forest.
Trait selection and measurements
To encompass above- and below-ground structures, we sampled the following leaf, stem, and root traits (see all traits and respective values in Table 2):
Structural traits
The two structural traits representing plant size, height, and canopy diameter, were obtained in the field using a measuring tape. Plant height was recorded using a vertical measurement of the tree from the soil to the top of the canopy. Canopy diameter was established by measuring two perpendicular canopy widths directed north and west, respectively. Both canopy measurements were subsequently averaged to calculate the canopy diameter.
Leaf traits
For leaf area (LA) and specific leaf area (SLA), three fully developed sun-exposed leaves were sampled from each tree and stored in plastic bags to maintain leaf moisture for image scanning. Fresh leaf areas were scanned with a Hp Officejet 4,500 desktop scanner. The petiole was excluded because it can influence leaf weight and not represent the photosynthetic area (Pérez-Harguindeguy et al., 2013). The leaves were dried for 48 h at 60°C and weighed. Leaf area (LA) was calculated using an ImageJ program (Schneider et al., 2012), and the specific leaf area (SLA) was calculated using the dimensions of the fresh leaf area divided by its dry weight. For C:N ratio and Leaf ∂13C isotope, three leaves per individual were sampled. The leaves were dried for 48 h at 60°C, macerated, encapsulated, and sent to UC Davis Laboratory for chemical and isotopic analysis, respectively. Data on leaf phenological patterns as the number of months that tree species were dormant, without any leaves in their canopy were obtained from the literature (Machado et al., 1997; Lima and Rodal, 2010; Lima et al., 2012; Souza et al., 2014). If species where reported in more than one publication, we calculated an average value per species.
Wood traits
The largest branch above the main stem (50 mm of diameter on average) was selected to be sampled to minimize plant injuries. After sampling the entire branch, two sub-samples were made. The first sample was stored in a plastic bag to maintain humidity for the measurements of bark density, wood density, bark and wood water storage capacity, and bark thickness. Bark was detached from its wood, and the fresh volume of both structures was obtained using water displacement. Bark and wood were submerged for 48 h, re-weighed to obtain saturated weight, and then, subsequently dried at 70°C for 1 week, and weighed again to obtain the dry weight. Bark and wood densities were calculated by dividing the dry weight by fresh volume. Bark and wood water storage capacities were calculated using the difference between the saturated and dry weight divided by dry weight. Bark thickness was calculated by averaging two perpendicular lengths measured from the vascular cambium to the external part of the fresh bark.
The second sample was stored in a paper bag to measure the hydraulic weighted diameter, which reflects the conductance of conduits, calculated through , where D is the diameter of each vessel and N is the number of vessels measured. Samples were naturally dried and polished with different grains of sandpaper until the anatomical structures were evident to measure the vessels. Images of all samples were obtained using a stereo microscope Nikon SMZ1500. For each sample, the diameters of 100 vessels were measured using ImagePro Plus software, guided by Scholz et al. (2013) method.
Root traits
For each individual, we dug (40 cm radius, 20 cm of depth) around the stem of each tree and carefully removed all the soil until the roots were exposed. To obtain the specific root area (SRA), calculated as the fresh root surface area divided by root dry mass (cm3-g2), we sampled roots with a diameter smaller than 1 mm. The samples were stored in zip locks, inside a cooler bag to avoid moisture loss. The fresh root area was scanned with a Hp Officejet 4,500 desktop scanner, and measured with the free software ImageJ. Subsequently, the root samples were dried at 70°C for 1 week and weighed to obtain their dry mass. To obtain the root volume, all roots larger than 1 mm had their largest diameter (D) and length (L) measured. Its volume (V) was calculated for each root individually, using the cylinder formula (V = π.r2.L). The total root volume per individual was calculated by adding all measurements. The average of all root volumes of each tree species represented the species root volume. The root density trait was calculated by dividing root dry mass by root fresh volume (g/cm3). To do so, we sampled one root, larger than 10 mm in diameter, and calculated its volume using the water displacement method. The samples were dried at 70°C for 1 week and weighed to obtain the root dry mass. See what the traits' names, units, and the respective physiological function of each trait are responsible for in Supplementary Table 1.
Statistical analysis
To establish coordinated trade-offs between species traits, we performed a Principal Component Analysis with the 16 traits sampled. After data normalization procedures, we used the principal function of the “psych” package (Revelle, 2018) and the varimax argument for axis adjustment. The number of significant axes was evaluated by a chi-squared test. We considered interpretable and relevant ecological variables, the ones with scores higher than 0.5 following (Hair et al., 2014).
We used a dataset with all species and their trait values to test if the functional traits formed discrete functional groups. We performed a k-means analysis using the cascadeKM function of the “vegan” package (Oksanen et al., 2018) after data normalization procedures, using a log scale for the variables SLA, SRA, and water storage capacity and squared root for wood density. The model was run with 1,000 iterations using the default Calinski criteria (Dimitriadou et al., 2002). To test differences in the values of functional traits between the groups established by k-means analysis, we performed a MANOVA, followed by standardized individual linear models to test which traits differed between functional groups.
To understand the distribution of plant functional strategies in the domain, we used the species occurrence data gathered by Silva and Souza (2018). We grouped species based on the two functional groups previously established by k-means analysis. We then extract the coordinates of each species' presence belonging to the conservative and acquisitive group formed by k-means results, and plot over the domain map obtained from worldclim containing mean precipitation and mean temperatures data with a resolution of 2.5 min. We calculated the aridity index according to Quan et al. (2013), dividing the mean precipitation by the mean temperatures and adding a constant number of 33 (AI = precipitation/mean temperature + 33). We built the map of functional groups distribution over the annual aridity index availability by using the QGis software. To test if the distribution of functional groups was related to environmental stress, we accessed the data of Caatinga mean annual rainfall (mm) from Worldclim (Fick and Hijmans, 2017), considering 30 years of data between 1970 and 2000s, and average temperature.
To test if there is a spatial association between two sets of points or whether they are distributed in space independently over the Caatinga, we performed Ripley's K-test, using the k12fun function from the “ads” R package. We tested our model against the null hypothesis of independence once we were interested in understanding if there is spatial aggregation/repulsion between functional groups (Pelissier and Goreaud, 2015). The confidence limits were established by 1,000 Monte Carlo simulations.
To test if species from distinct functional groups occur at distinct aridity levels, we first extracted the aridity index values of all geographic points in which the species of each group occurred. We also extract aridity index values from 120 random geographic points to test the group found against values of randomly distributed occurrences. We, then, tested the data for spatial autocorrelation using the Moran.I function from the “ape” package (Paradis and Schliep, 2018). Because our data presented spatial autocorrelation, we performed a generalized least-squared analysis using the gls function from the “nlme” package (Pinheiro et al., 2019). This analysis tests if there is a difference in the aridity values between groups, incorporating the latitude and longitude coordinates to remove the spatial bias of species occurrence (Morans I-test, Observed = −0.004, expected = −0.002, sd = 0.048, p = 0.95) (see analysis details in Supplementary Figure 1). The first variable is used as the intercept by the package default for a matter of comparison between variables. All geospatial procedures were made using the “rdgal” (Bivand et al., 2019) and “raster” (Hijmans, 2019) packages.
Results
Caatinga traits, trade-offs, and strategies
Trait coordination of 20 Caatinga tree species (Table 2) resulted in five significant axes, explaining 38, 18, 17, 15, and 13% of the data variation (Table 3, see trait values in Supplementary Table 2). The first axis (Figure 1) was positively correlated with acquisitive trait values such as high leaf area, high hydraulic weighted diameter, high bark thickness, and storage capacity and negatively correlated with conservative traits such as high tissue densities of wood, root, and bark. The second axis was positively correlated with acquisitive traits such as larger stature, canopy diameter, and low C:N leaf ratio. The third axis was positively correlated with species with high SLA and longer periods without leaves. The fourth axis was positively correlated to a high volume of root occupied per soil ground unit and high bark density and negatively correlated to high bark water storage capacity. The fifth axis was positively correlated with high leaf area and high water use efficiency represented by Leaf ∂13C.
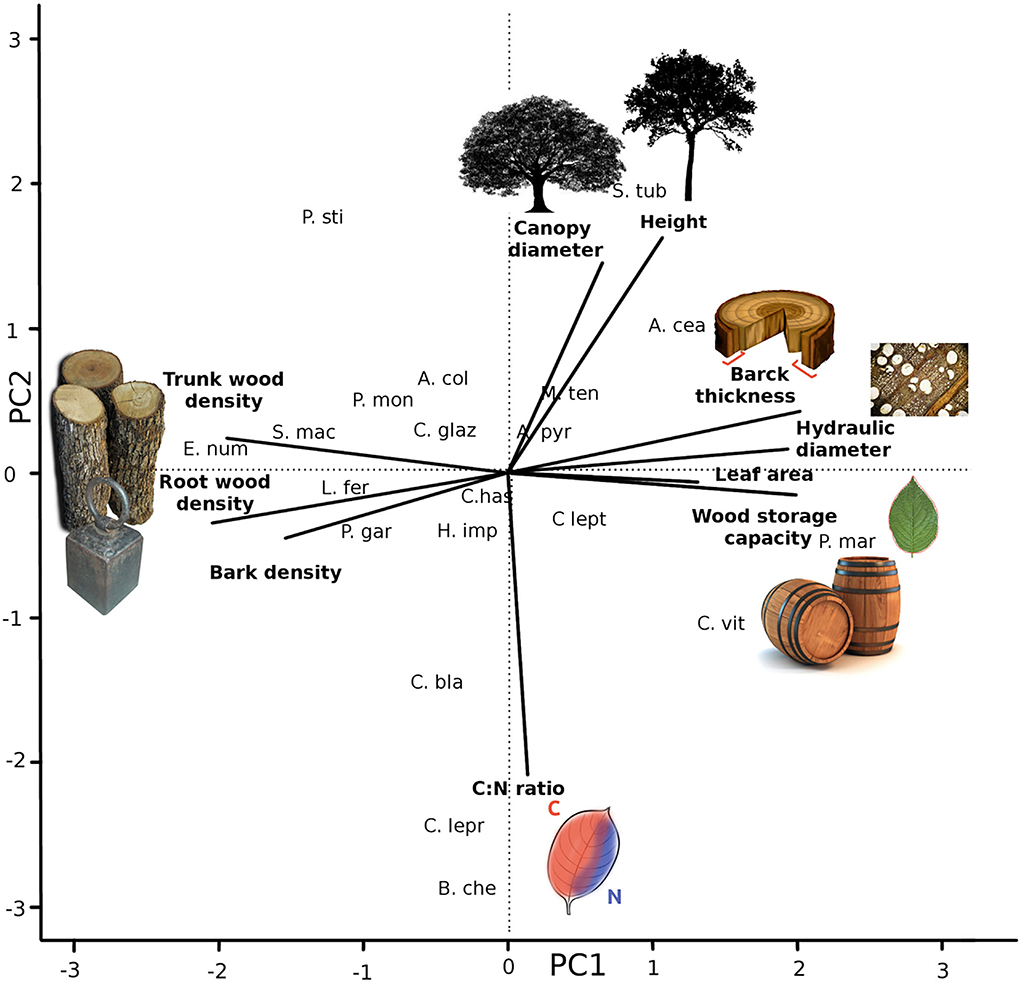
Figure 1. Coordinated trade-offs based on PCA analysis. Results of the two first axis of a PCA analysis with selected trait scores higher than 0.5. On PC1 Leaf area, Wood density, Wood storage capacity, Bark density, Bark thickness, hydraulic weighted diameter capacity, and Root density. PC2 is represented by Canopy diameter and Leaf C:N ratio. The analysis was performed with 20 tree species of Caatinga SDTF. The full name of the species can be found in Table 1.
Functional groups
Caatinga tree species were divided into two main functional groups according to k-means analysis. The first group was represented by four species: Spondias tuberosa, Pseudobombax marginatum, Cochlospermum vitifolium, and Commiphora leptophloeos, characterized by higher height, higher canopy diameter, low tissue density, high water storage capacity, high bark thickness, high hydraulic weighted diameter capacity, and high SRA (i.e., acquisitive group, Figure 2). The second group included 16 species characterized by lower height, lower canopy diameter, higher number of months with canopy holding leaves, higher tissue density, low storage capacity, low bark thickness, low hydraulic weighted diameter, and low Specific root area (SRA) (i.e., conservative group, Figure 2). The value of traits differed between groups (df = 1, pillai = 0.911, f = 21.84, p < 0.001), but see individual linear models testing each trait values between functional groups in Supplementary Table 3.
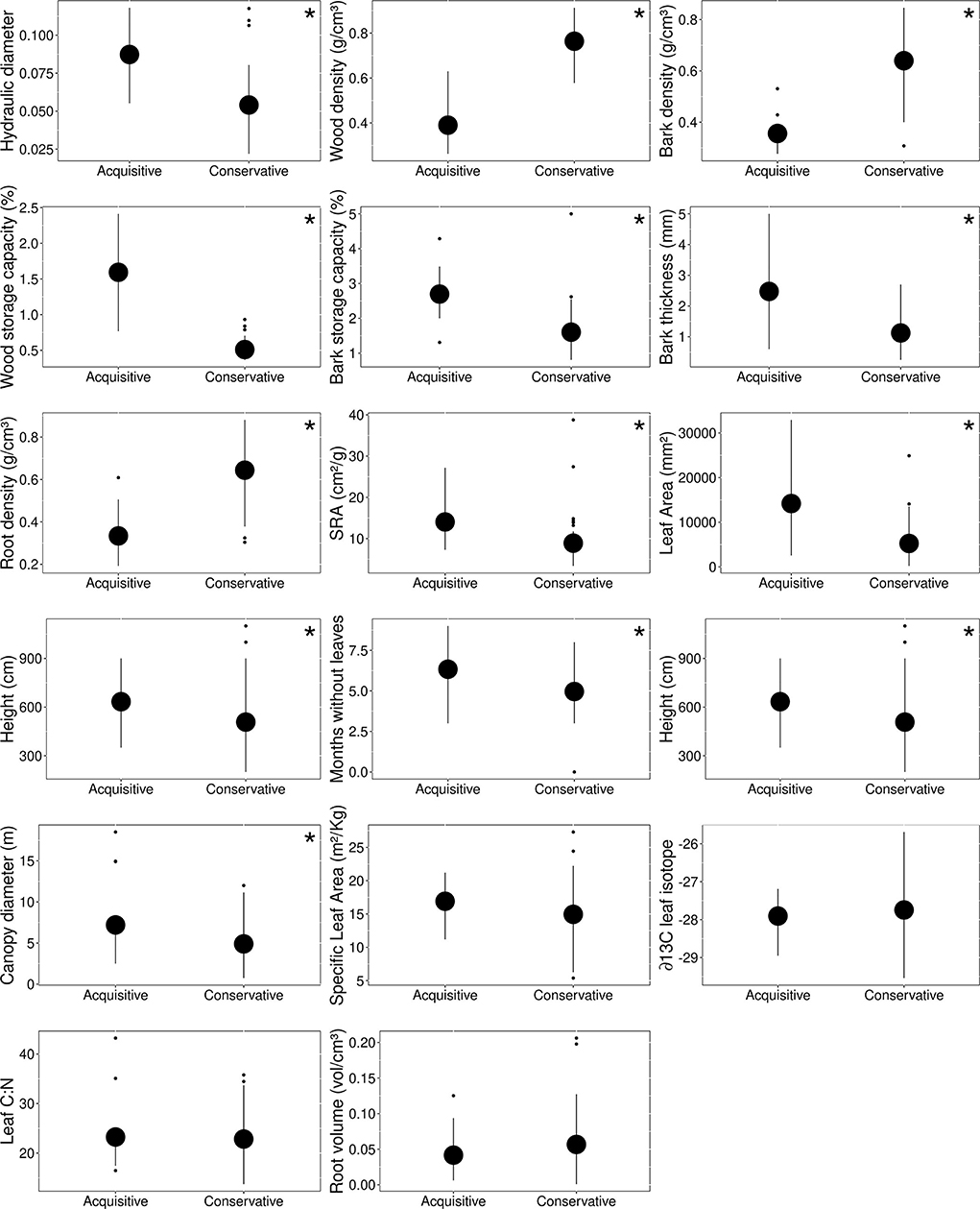
Figure 2. Functional group trait values. The mean and standard deviation of the functional attributes of conservative and acquisitive groups were found based on Calinski's criteria. Traits that differ between groups by GLM models are represented by *, see all analysis values in Supplementary Table 3.
Functional groups' spatial distribution
The aridity index varied over the domain from 7.14 to 28.79. The lowest aridity region was found at the border of the Caatinga with the northern part of the Cerrado domain, and the highest aridity region occurred at the center of the Caatinga domain. The acquisitive and conservative functional groups are distributed independently of one another along the Caatinga domain (Figure 3, and graphical analysis in Supplementary Figure 2). However, the regions in which acquisitive species occur presented slightly higher aridity (Table 4) than the regions where conservative species occur.
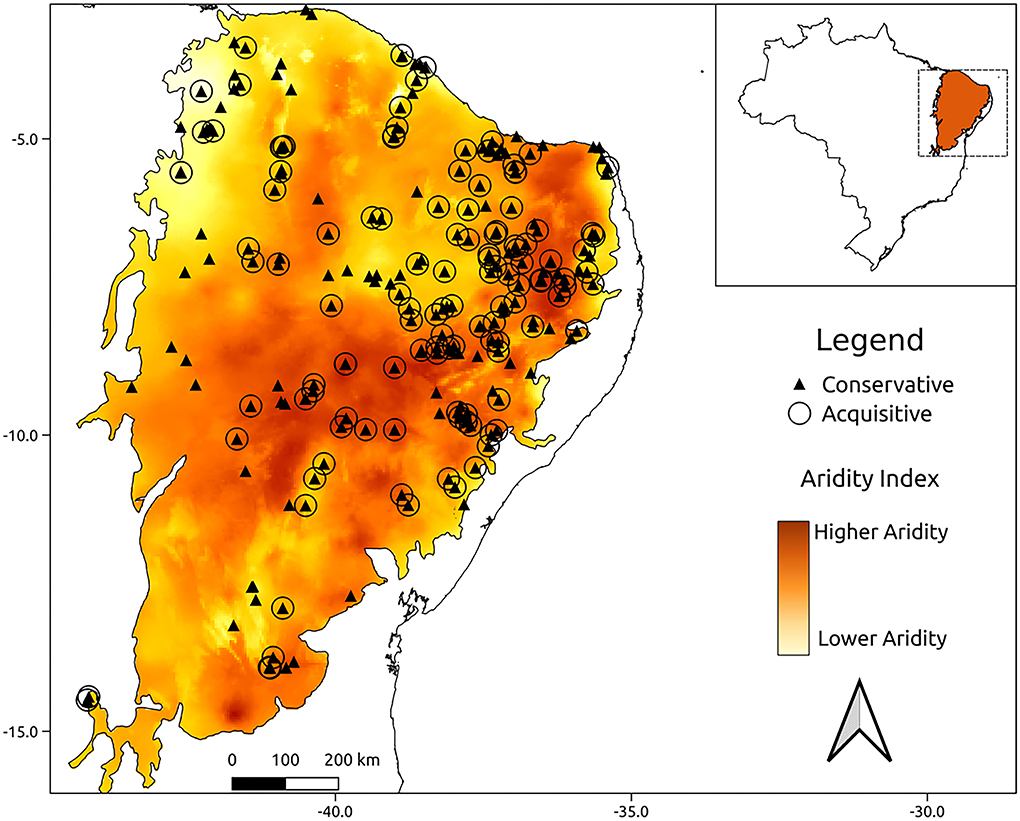
Figure 3. Spatial distribution of functional groups along the Caatinga biome. The acquisitive functional group is represented by O and the conservative functional group is represented by ▴. The colors indicate the aridity index calculated for 30 years, from 1970 to 2000, the dark colors indicate greater aridity areas and the light colors represent lower aridity areas.
Discussion
In this work, we found that the Caatinga tree species exhibit different strategies that build the acquisitive-conservative continuum. In general, we found a classic spectrum of acquisitive species presenting lower wood density above and below ground, thicker bark, higher hydraulic weighted diameter, larger leaf area, and higher water storage capacity both for bark and wood. On the other hand, we also found classic conservative species presenting a higher wood density above and below ground, thinner bark, smaller hydraulic weighted diameter, smaller leaf area, and lower water storage capacity both for bark and wood. The two groups of strategies were distinct in terms of their functional traits, but other trait combinations such as water storage that together overcome water loss could expand the distribution of acquisitive species, helping plants to survive during wet and dry seasons. As a consequence, the acquisitive plant group does not seem to be spatially limited by aridity, on the contrary, this group co-occurs with conservative strategy trees across a wide aridity gradient throughout the biome.
Coordinated trade-offs and plant strategies
Trees with widespread distribution in the Caatinga Seasonally Dry Tropical Forest present multiple strategies that sustain the acquisitive-stress tolerant continuum of plant strategies. The first of these dimensions (represented by axis PC1) embraces the well-known trade-off between vulnerable but fast-growing tissues on the acquisitive side vs. safe but slow-growing tissues on the conservative side (Grime, 1977; Diaz et al., 2016).
In tropical forests, this trade-off is expected to be found when studying above- and below-ground traits (Borchert, 1994; Stratton et al., 2000; Baraloto et al., 2011; Fortunel et al., 2012; Vleminckx et al., 2021). However, these traits are not often measured in seasonally dry tropical forests (Roumet et al., 2016; Kong et al., 2019). Our results show that these patterns also apply to SDTF, agreeing with the works of Pineda-García et al. (2015), who developed an SDTF with similar pluviosity and temperature that found the same pattern for root traits.
The hydraulic weighted diameter, a water-related trait that is also rarely studied in such forests and has been fundamental to explaining the coordination of species trait strategies (Zanne et al., 2010; Méndez-Alonzo et al., 2013; Reich, 2014). The greater hydraulic weighted diameter was related to the acquisitive side of the spectrum. Similar results were found in a study conducted in a dry forest with rainfall and temperature equivalent to the Caatinga at the island of Lana'i—Hawaii (Stratton et al., 2000), Colombia (González et al., 2021), and Mexico (Markesteijn et al., 2011b). Additionally, wood water storage capacity was positively correlated with more acquisitive strategies. This pattern was detected in Neotropical savannas by Scholz et al. (2007) and in a dry tropical forest by Pineda-García et al. (2013), where more acquisitive trees could use stored water to sustain their metabolism during sporadic drought periods over the wet season. Oliveira et al. (2021) have proposed that water-related traits tend to be associated with the conservative-acquisitive spectrum in the tropics, which was shown by Markesteijn et al. (2011a) and Méndez-Alonzo et al. (2012) and here, we confirm empirically this hypothesis for SDTF, highlighting the importance of studying traits that are linked to water use and storage to better understand plant strategies.
The second trait combination dimension (represented by axis PC2) revealed that plants with larger height and canopy size are the ones that have higher nitrogen leaf content (lower C/N ratio), which is an indicator of higher photosynthetic capacity. The third dimension (represented by axis PC3) reveals that plants with high photosynthetic capacity (higher SLA) compensate for their higher water loss through transpiration by losing their leaves for longer periods (Grime, 1977; Poorter et al., 2006). Acquisitive species that have higher growth rates are also susceptible to high water losses through leaves, lower resistance to xylem embolism, and a higher likelihood of dehydration (Eamus, 1999; Pérez-Ramos et al., 2013; Eller et al., 2018). Therefore, this strategy coordinates with other fundamental survival mechanisms in the Caatinga Tropical Dry Forest, namely the ability to avoid or tolerate drought periods (Pineda-García et al., 2013; Pinho et al., 2019). Acquisitive species present short canopy duration (e.g., species maintain their leaves for 3–5 months); by losing their leaves, they decrease water loss during dry periods, likely reducing the mortality risk. Conservative species (low SLA values), on the other hand, have low water loss through their leaves, greater resistance to xylem embolism, and a lower likelihood of dehydration (Lima et al., 2012). Consequently, these species can safely hold their canopy leaves for longer periods (e.g., 7 months per year, including species that never lose their leaves) resisting drought (Brodribb et al., 2003; Lima et al., 2018).
The fourth and fifth dimensions (Axis PC4 and PC5) represent the traits that increase water acquisition or reduce water loss. The fourth axis shows that species that have a high bark density and therefore restricted water storage, have a greater root volume in the soil, increasing their water acquisition capacity. The fifth axis shows that species that have a larger leaf area, which may consequently lead to higher water loss, have greater stomatal regulation (∂C13 less negative), which is a compensation to avoid water loss.
The importance of short-term storage
The Caatinga Dry Tropical Forest is a complex and unique environment, being one of the semiarid systems with the highest rainfall unpredictability (Garreaud et al., 2008). Despite the ability of the species to lose their leaves avoiding periods of drought, they have to deal with the gaps in rainfall, even during the rainy season. In environments where the rainy season is not predictable, short-term water storage traits, such as bark and wood structures, can be of great importance to ensure the functioning of many physiological processes (Borchert, 1994; Poorter et al., 2014; Rosell et al., 2014; Loram-Lourenço et al., 2020). It is important to point out that bark tissues had a water storage capacity 40% higher than wood tissue. Although bark water storage may represent short-term water availability compared to other specific storage organs, it can play an important role for plant species with acquisitive strategies (Rosell et al., 2014). Short-term water storage structures are easily accessed and can act as a water buffer, maintaining water flux, and decreasing the risk of plant damage or embolism due to dehydration (Stratton et al., 2000; Pérez-Ramos et al., 2013). Thus, short-term water storage might be an essential mechanism by which plants with acquisitive strategies can be successful in semiarid lands, and may also be an important trait to be studied in other types of ecosystems.
Spatial distribution of species strategies
Our results indicate that species with acquisitive strategies are not limited by aridity and, therefore can persist in drier areas of the Caatinga forest (Pinho et al., 2019). Recent findings indicate that this might be a general pattern for the Caatinga domain (de Oliveira et al., 2020). We found four plant strategies that could explain how acquisitive tree species overcome water stress in higher aridity areas: (1) by increasing root allocation, they increase their ability to rapidly acquire water during the limited growing season; (2) by increasing water storage in bark and wood, they increase their capacity to acquire water during sporadic dry periods; (3) by having higher control of their stomatal closure, they avoid water loss; and (4) by losing their leaves, they avoid water stress (de Lima et al., 2021). Additionally, the strategy of rapid growth and acquisitive behavior during the rainy season and leaf loss during the dry season allows these species to maintain viable populations in areas subject to considerable drought periods, as our results on the spatial distribution of functional groups suggest. The rainy season in the SDTF is short and irregular (Garreaud et al., 2008; da Silva et al., 2017). A greater capacity to acquire available water for short periods may be competitively advantageous to conservative species, which need longer periods for water acquisition (Reich, 2014). The acquired water can be stored in the soft tissues, ensuring photosynthesis (Poorter et al., 2014) and preventing embolism (Stratton et al., 2000) during the absence of rain. Finally, the rapid response of leaf loss at the end of the rainy season can give the acquisitive species a rapid dormancy process, avoiding drought stress (Eamus, 1999).
Future perspectives
Our work considers a unique set of multiple above- and below-ground traits measured in 20 of the most widespread tree species of this biome. Future works should increase the number of trees studied to better represent the overall 886 tree species occurring in the Caatinga domain (BFG, 2015). This broader study would allow the comparison of functional traits between rare and widespread tree species. Future works should also focus on increasing sampling efforts to address the contribution of intraspecific variation to overall trait differences, as well as increasing sampling localities to enhance the robustness of plant strategy predictions for such diverse and complex systems. Additionally, future climate change scenarios predict more sparse and reduced rainfall in this region, which may significantly affect the distribution of these species across the domain. In this sense, studies that investigate how tree community traits will be affected by future climate scenarios are of extreme importance (Santos et al., 2014).
Data availability statement
The original contributions presented in the study are included in the article/Supplementary material, further inquiries can be directed to the corresponding author.
Author contributions
MF, GG, and AS conceived the ideas, designed methodology, analyzed the data, and contributed to the writing of the manuscript. MF and GG collected the data. MF and RO performed lab analysis and writing of the manuscript. All authors contributed to the article and approved the submitted version.
Funding
This study was financed in part by the Coordenação de Aperfeiçoamento de Pessoal de Nível Superior, Brazil (CAPES), Finance Code 001 and by the Brazilian National Research Council—PELD grant (441684/2020-3), and research fellowship PQ granted to GG (316924/2021-0). RO received a CNPq productivity scholarship.
Acknowledgments
We are thankful to RO's Lab members for supporting isotopic and chemical leaf analysis procedures. We thank the members of the Laboratório de Ecologia da Restauração (LER) for helping to collect plant material for trait analysis.
Conflict of interest
The authors declare that the research was conducted in the absence of any commercial or financial relationships that could be construed as a potential conflict of interest.
Publisher's note
All claims expressed in this article are solely those of the authors and do not necessarily represent those of their affiliated organizations, or those of the publisher, the editors and the reviewers. Any product that may be evaluated in this article, or claim that may be made by its manufacturer, is not guaranteed or endorsed by the publisher.
Supplementary material
The Supplementary Material for this article can be found online at: https://www.frontiersin.org/articles/10.3389/ffgc.2022.930099/full#supplementary-material
References
Albuquerque, U. P., de Lima Araújo, E., and El-Deir, A. C. A. (2012). Caatinga revisited: ecology and conservation of an important seasonal dry forest. ScientificWorldJournal 2012, 205182. doi: 10.1100/2012/205182
Antongiovanni, M., Venticinque, E. M., and Fonseca, C. R. (2018). Fragmentation patterns of the Caatinga drylands. Landsc. Ecol. 33, 1353–1367. doi: 10.1007/s10980-018-0672-6
Antongiovanni, M., Venticinque, E. M., Matsumoto, M., and Fonseca, C. R. (2020). Chronic anthropogenic disturbance on Caatinga dry forest fragments. J. Appl. Ecol. 57, 2064–2074. doi: 10.1111/1365-2664.13686
Baraloto, C., Rabaud, S., Molto, Q., Blanc, L., Fortunel, C., Hérault, B., et al. (2011). Disentangling stand and environmental correlates of aboveground biomass in Amazonian forests. Glob. Chang. Biol. 17, 2677–2688. doi: 10.1111/j.1365-2486.2011.02432.x
BFG (2015). Growing knowledge: an overview of seed plant diversity in Brazil. Rodriguesia 66, 1085–1113. doi: 10.1590/2175-7860201566411
Bivand, R., Tim, K., and Rowlingson, B. (2019). rgdal: Bindings for the 'Geospatial' Data Abstraction Library. R package version 1.4-4. Available online at: https://CRAN.R-project.org/package=rgdal
Borchert, R. (1994). Soil and stem water storage determine phenology and distribution of tropical dry forest trees. Ecology 75, 1437–1449. doi: 10.2307/1937467
Brodribb, T., Holbrook, N. M., and Gutiérrez, M. V. (2003). Hydraulic and photosynthetic co-ordination in seasonally dry tropical forest trees. Plant Cell Environ. 25, 1435–1444. doi: 10.1046/j.1365-3040.2002.00919.x
Chave, J., Coomes, D., Jansen, S., Lewis, S. L., Swenson, N. G., and Zanne, A. E. (2000). Towards a worldwide wood economics spectrum. Ecol. Lett. 12, 351–366. doi: 10.1111/j.1461-0248.2009.01285.x
Chesson, P., Gebauer, R. L. E., and Schwinning, S. (2004). Resource pulses, species interactions, and diversity maintenance in arid and semi-arid environments. Oecologia 141, 236–253. doi: 10.1007/s00442-004-1551-1
Cornwell, W. K., and Ackerly, D. D. (2009). Community assembly and shifts in plant trait distributions across an environmental gradient in coastal California. Ecol. Monogr. 79, 109–126. doi: 10.1890/07-1134.1
Costa-Saura, J. M., Martínez-Vilalta, J., Trabucco, A., Spano, D., and Mereu, S. (2016). Specific leaf area and hydraulic traits explain niche segregation along an aridity gradient in Mediterranean woody species. Perspect. Plant Ecol. Evolut. Syst. 21, 23–30. doi: 10.1016/j.ppees.2016.05.001
da Silva, M. C., Leal, I., and Tabarelli, M. (2017). Caatinga: The largest Tropical Dry Forest Region in South America. Springer International Publishing. doi: 10.1007/978-3-319-68339-3
de Amorim, L. D. M., de Oliveira, L., Oliveira, F. F. M., Camacho, R. G. V., and De Melo, J. I. M. (2016). Fabaceae na Floresta Nacional (FLONA) de Assú, semiárido potiguar, nordeste do Brasil. Rodriguesia 67, 105–123. doi: 10.1590/2175-7860201667108
de Lima, A. L., Rodal, M. J. N., Castro, C., Antonino, A. D., Melo, L., de Gonçalves-Souza, T., et al. (2021). Phenology of high- and low-density wood deciduous species responds differently to water supply in tropical semiarid regions. J. Arid Environ. 193, 104594. doi: 10.1016/j.jaridenv.2021.104594
de Oliveira, A. C. P., Nunes, A., Rodrigues, R. G., and Branquinho, C. (2020). The response of plant functional traits to aridity in a tropical dry forest. Sci. Total Environ. 747, 141–177. doi: 10.1016/j.scitotenv.2020.141177
Diaz, S., Kattge, J., Cornelissen, J. H., Wright, I. J., Lavorel, S., Dray, S., et al. (2016). The global spectrum of plant form and function. Nature 529, 167–171. doi: 10.1038/nature16489
Dimitriadou, E., Dolničar, S., and Weingessel, A. (2002). An examination of indexes for determining the number of clusters in binary data sets. Psychometrika 67, 137–159. doi: 10.1007/BF02294713
Domec, J. C., Scholz, F. G., Bucci, S. J., Meinzer, F. C., Goldstein, G., and Villalobos-Vega, R. (2006). Diurnal and seasonal variation in root xylem embolism in neotropical savanna woody species: impact on stomatal control of plant water status. Plant Cell Environ. 29, 26–35. doi: 10.1111/j.1365-3040.2005.01397.x
Eamus, D. (1999). Ecophysiological traits of deciduous and evergreen woody species in the seasonally dry tropics. Trends Ecol. Evol. 14, 11–16. doi: 10.1016/S0169-5347(98)01532-8
Eller, C. B., Barros, F. V., Bittencourt, P. R. L., Rowland, L., Mencuccini, M., and Oliveira, R. S. (2018). Xylem hydraulic safety and construction costs determine tropical tree growth. Plant Cell Environ. 41, 548–562 doi: 10.1111/pce.13106
Engelbrecht, B. M., and Kursar, T. A. (2003). Comparative drought-resistance of seedlings of 28 species of co-occurring tropical woody plants. Oecologia 136, 383–393. doi: 10.1007/s00442-003-1290-8
Engelbrecht, B. M. J., Comita, L. S., Condit, R., Kursar, T. A., Tyree, M. T., Turner, B. L., et al. (2007). Drought sensitivity shapes species distribution patterns in tropical forests. Nature 447, 80–83. doi: 10.1038/nature05747
Esquivel-Muelbert, A., Baker, T. R., Dexter, K. G., Lewis, S. L., Steege, H., Lopez-Gonzalez, G., et al. (2016). Seasonal drought limits tree species across the Neotropics. Ecography 39, 1–12. doi: 10.1111/ecog.01904
Fick, S. E., and Hijmans, R. J. (2017). WorldClim 2: new 1-km spatial resolution climate surfaces for global land areas. Int. J. Climatol. 37, 4302–4315. doi: 10.1002/joc.5086
Fortunel, C., Fine, P. V. A., and Baraloto, C. (2012). Leaf, stem and root tissue strategies across 758 Neotropical tree species. Funct. Ecol. 26, 1153–1161. doi: 10.1111/j.1365-2435.2012.02020.x
Frenette-Dussault, C., Shipley, B., Léger, J. F., Meziane, D., and Hingrat, Y. (2012). Functional structure of an arid steppe plant community reveals similarities with Grime's C-S-R theory. J. Veg. Sci. 23, 208–222. doi: 10.1111/j.1654-1103.2011.01350.x
Garreaud, R. D., Vuille, M., Compagnucci, R., and Marengo, J. (2008). Present-day South American climate. Palaeogeogr. Palaeoclimatol. Palaeoecol. 281, 180–195. doi: 10.1016/j.palaeo.2007.10.032
González, M. R., Posada, J. M., Carmona, C. P., Garzón, F., Salinas, V., Idárraga-Piedrahita, Á, et al. (2021). Diverging functional strategies but high sensitivity to an extreme drought in tropical dry forests. Ecol. Lett. 24, 451–463. doi: 10.1111/ele.13659
Grime, J. P. (1977). Evidence for the existence of three primary strategies in plants and its relevance to ecological and evolutionary theory. Am. Nat. 111, 1169–1194 doi: 10.1086/283244
Hair, J., Black, W., Babin, B., and Anderson, R. (2014). Multivariate Data Analysis. Edinburgh: Pearson Education limited.
Harris, I., Jones, P. D., Osborn, T. J., and Lister, D. H. (2014). Updated highresolution grids of monthly climatic observations - the CRU TS3.10 Dataset. Int. J. Climatol. 34, 623–642. doi: 10.1002/joc.3711
Hijmans, R. J. (2019). raster: Geographic Data Analysis and Modeling. R package version 2.9-23. Available online at: https://CRAN.R-project.org/package=raster
Kattge, J., Díaz, S., Lavorel, S., Prentice, I. C., Leadley, P., Bönisch, G., et al. (2011). TRY—a global database of plant traits. Glob. Chang. Biol. 17, 2905–2935. doi: 10.1111/j.1365-2486.2011.02451.x
Kong, D., Wang, J., Wu, H., Valverde-Barrantes, O. J., Wang, R., Zeng, H., et al. (2019). Nonlinearity of root trait relationships and the root economics spectrum. Nat. Commun. 10, 1–9. doi: 10.1038/s41467-019-10245-6
Leal, I. R., Da Silva, J. M. C., Tabarelli, M., and Lacher, T. E. (2005). Changing the course of biodiversity conservation in the caatinga of northeastern Brazil. Conserv. Biol. 19, 701–706. doi: 10.1111/j.1523-1739.2005.00703.x
Lima, A. L. A., Barretto Sampaio, V., de Castro, C. C., Rodal, M. J. N., Antonino, A. C., and de Melo, A. L. (2012). Do the phenology and functional stem attributes of woody species allow for the identification of functional groups in the semiarid region of Brazil? Trees 26, 1605–1616. doi: 10.1007/s00468-012-0735-2
Lima, A. L. A., and Rodal, M. J. N. (2010). Phenology and wood density of plants growing in the semi-arid region of northeastern Brazil. J. Arid Environ. 74, 1363–1373. doi: 10.1016/j.jaridenv.2010.05.009
Lima, T., Carvalho, E., Martins, F., Miranda, R. S., Muller, C., Pereira, L., et al. (2018). Lignin composition is related to xylem embolism resistance and leaf retention time in trees in a tropical semiarid climate. New Phytol. 219, 1252–1262. doi: 10.1111/nph.15211
Lira, R., Maracajá, P., Miranda, M. A., da Sousa, D. D., de Melo, S. B., and Amorim, L. B. (2010). Estudo da composição florística arbóreo-arbustivo na Floresta Nacional de Açu no semi árido do RN Brasil. Agropecuária Científica Semi Árido 3, 23–30. Retrieved from http://150.165.111.246/ojs-patos/index.php/ACSA/article/view/28
Loram-Lourenço, L., Farnese, F., Sousa, L. F., Alves, R. D. F. B., Andrade, M. C. P., and Almeida, S. E. (2020). A structure shaped by fire, but also water: ecological consequences of the variability in bark properties across 31 species from the Brazilian Cerrado. Front. Plant Sci. 10, 1718. doi: 10.3389/fpls.2019.01718
Machado, C. S., Barros, L. M., and Sampaio, E. V. S. B. (1997). Phenology of caatinga species at Serra Talhada, PE, Northeastern Brazil. Biotropica 29, 57–68. doi: 10.1111/j.1744-7429.1997.tb00006.x
Markesteijn, L., Poorter, L., Bongers, F., Paz, H., and Sack, L. (2011a). Hydraulics and life history of tropical dry forest tree species: coordination of species' drought and shade tolerance. New Phytol 191, 480–495. doi: 10.1111/j.1469-8137.2011.03708.x
Markesteijn, L., Poorter, L., Paz, H., Sack, L., and Bongers, F. (2011b). Ecological differentiation in xylem cavitation resistance is associated with stem and leaf structural traits. Plant Cell Environ. 34, 137–148. doi: 10.1111/j.1365-3040.2010.02231.x
Martínez-Vilalta, J., Poyatos, R., Aguadé, D., Retana, J., and Mencuccini, M. (2014). A new look at water transport regulation in plants. New Phytol. 204, 105–115. doi: 10.1111/nph.12912
Méndez-Alonzo, R., Paz, H., Zuluaga, R. C., Rosell, J. A., and Olson, M. E. (2012). Coordinated evolution of leaf and stem economics in tropical dry forest trees. Ecology 93, 2397–2406. doi: 10.1890/11-1213.1
Méndez-Alonzo, R., Pineda-García, F., Paz, H., Rosell, J. A., and Olson, M. E. (2013). Leaf phenology is associated with soil water availability and xylem traits in a tropical dry forest. Trees 27, 745–754. doi: 10.1007/s00468-012-0829-x
Moro, M. F., Nic Lughadha, E., de Araújo, F. S., and Martins, F. R. (2016). A phytogeographical metaanalysis of the semiarid caatinga domain in Brazil. Bot. Rev. 82:91–148. doi: 10.1007/s12229-016-9164-z
Oksanen, J., Guillaume, G. L., Michael, F., Roeland, K., Legendre, P., Dan McGlinn, D., et al. (2018). vegan: Community Ecology Package. R package version 2.5-2. Available online at: https://CRAN.R-project.org/package=vegan
Oliveira, C. C., Zandavalli, R. B., de Lima, A. L. A., and Rodal, M. J. N. (2015). Functional groups of woody species in semi-arid regions at low latitudes. Austral. Ecol. 40, 40–49. doi: 10.1111/aec.12165
Oliveira, R. S., Eller, C. B., Barros, F. V., Hirota, M. B., Rum, M., et al. (2021). Linking plant hydraulics and the fast–slow continuum to understand resilience to drought in tropical ecosystems. New Phytol. 230, 904–923. doi: 10.1111/nph.17266
Paradis, E., and Schliep, K. (2018). ape 5.0: an environment for modern phylogenetics and evolutionary analyses in R. Bioinformatics 35, 526–528. doi: 10.1093/bioinformatics/bty633
Pelissier, R., and Goreaud, F. (2015). ads Package for R: A Fast Unbiased Implementation of the K-function Family for Studying Spatial Point Patterns in Irregular-Shaped Sampling Windows. Journal of Statistical Software 63:1-18. URL http://www.jstatsoft.org/v63/i06/.
Pennington, R. T., Lavin, M., and Oliveira-Filho, A. (2009). Woody plant diversity, evolution, and ecology in the tropics: perspectives from seasonally dry topical forests. Ann. Rev. Ecol. Evol. Syst. 40, 437–457. doi: 10.1146/annurev.ecolsys.110308.120327
Pérez-Harguindeguy, N., Díaz, S., Lavorel, S., Poorter, H., Jaureguiberry, P., and Bret-Harte, M. S. (2013). New handbook for standardized measurement of plant functional traits worldwide. Austral. J. Bot. 23, 167–234. doi: 10.1071/BT12225
Pérez-Ramos, I. M., Volaire, F., Fattet, M., Blanchard, A., and Roumet, C. (2013). Tradeoffs between functional strategies for resource-use and drought-survival in Mediterranean rangeland species. Environ. Exp. Bot. 87, 126–136. doi: 10.1016/j.envexpbot.2012.09.004
Pierce, S., Brusa, G., Vagge, I., and Cerabolini, B. E. L. (2013). Allocating CSR plant functional types: the use of leaf economics and size traits to classify woody and herbaceous vascular plants. Funct. Ecol. 27, 1002–1010. doi: 10.1111/1365-2435.12095
Pineda-García, F., Paz, H., and Meinzer, F. C. (2013). Drought resistance in early and late secondary successional species from a tropical dry forest: the interplay between xylem resistance to embolism, sapwood water storage and leaf shedding. Plant Cell Environ. 36, 405–418. doi: 10.1111/j.1365-3040.2012.02582.x
Pineda-García, F., Paz, H., Meinzer, F. C., and Angeles, G. (2015). Exploiting water versus tolerating drought: Water-use strategies of trees in a secondary successional tropical dry forest. Tree Physiol. 36, 208–217. doi: 10.1093/treephys/tpv124
Pinheiro, J., Bates, D., DebRoy, S., Sarkar, D., and R Core Team (2019). nlme: Linear and Nonlinear Mixed Effects Models_. R package version 3.1-141. Available online at: https://CRAN.R-project.org/package=nlme
Pinho, B. X., Tabarelli, M., Engelbrecht, B. M. J., Sfair, J., and Melo, F. P. L. (2019). Plant functional assembly is mediated by rainfall and soil conditions in a seasonally dry tropical forest. Basic Appl. Ecol. 40, 1–11. doi: 10.1016/j.baae.2019.08.002
Poorter, L., Bongers, L., and Bongers, F. (2006). Architecture of 54 moist-forest tree species: traits, trade-offs, and functional groups. Ecology 87, 1289–1301. doi: 10.1890/0012-9658(2006)87[1289:AOMTST]2.0.CO;2
Poorter, L., and Markesteijn, L. (2008). Seedling traits determine drought tolerance of tropical tree species. Biotropica 40, 321–331. doi: 10.1111/j.1744-7429.2007.00380.x
Poorter, L., Mcneil, A., Hurtado, V. H., Prins, H. H. T., and Putz, F. E. (2014). Bark traits and life-history strategies of tropical dry- and moist forest trees. Funct. Ecol. 28, 232–242. doi: 10.1111/1365-2435.12158
Quan, C., Han, S., Utescher, T., Zhang, C., and Liu, Y. S. C. (2013). Validation of temperature-precipitation based aridity index: paleoclimatic implications. Palaeogeogr. Palaeoclimatol. Palaeoecol. 386, 86–95. doi: 10.1016/j.palaeo.2013.05.008
Reich, P. B. (2014). The world-wide ‘fast–slow' plant economics spectrum: a traits manifesto. J. Ecol. 102, 275–301. doi: 10.1111/1365-2745.12211
Revelle, W. (2018). psych: Procedures for Personality and Psychological Research. Evanston, IL: Northwestern University. Available online at: https://CRAN.R-project.org/package=psych Version = 1.8.12.
Ribeiro, E. M. S., Santos, B. A., Arroyo-Rodríguez, V., Tabarelli, M., Souza, G., and Leal, I. R. (2016). Phylogenetic impoverishment of plant communities following chronic human disturbances in the Brazilian Caatinga. Ecology 97, 1583–1592. doi: 10.1890/15-1122.1
Rosell, J. A., Gleason, S., Méndez-Alonzo, R., Chang, Y., and Westoby, M. (2014). Bark functional ecology: evidence for trade offs, functional coordination, and environment producing bark diversity. New Phytol. 201:486–497. doi: 10.1111/nph.12541
Roumet, C., Birouste, M., Picon-Cochard, C., Ghestem, M., Osman, N., Vrignon-Brenas, S., et al. (2016). Root structure-function relationships in 74 species: Evidence of a root economics spectrum related to carbon economy. New Phytologist. 210, 815–826. doi: 10.1111/nph.13828
Santos, A., Tabarelli, M., Ribeiro, E. M. S., Arroyo-Rodriguez, V., Santos, B. A., Tabarelli, M., et al. (2015). Chronic anthropogenic disturbance drives the biological impoverishment of the Brazilian Caatinga vegetation. J. Appl. Ecol. 52, 611–620. doi: 10.1111/1365-2664.12420
Santos, M. G., Oliveira, M. T., Figueiredo, K. V., Falcão, H. M., Arruda, E. C. P., Almeida-Cortez, J., et al. (2014). Caatinga, the Brazilian dry tropical forest: can it tolerate climate changes? Theor. Exp. Plant Physiol. 26, 83–99. doi: 10.1007/s40626-014-0008-0
Schneider, C. A., Rasband, W. S., and Eliceiri, K. W. (2012). NIH Image to ImageJ: 25 years of image analysis. Nat Methods 9, 671–675. doi: 10.1038/nmeth.2089
Scholz, A., Klepsch, M., Karimi, Z., and Jansen, S. (2013). How to quantify conduits in wood? Front. Plant Sci. 4, 56. doi: 10.3389/fpls.2013.00056
Scholz, F. G., Bucci, S. J., Goldstein, G., Meinzer, F. C., Franco, A. C., and Miralles-Wilhelm, F. (2007). Biophysical properties and functional significance of stem water storage tissues in neotropical savanna trees. Plant Cell Environ. 30, 236–248. doi: 10.1111/j.1365-3040.2006.01623.x
Silva, A. C., and Souza, A. F. (2018). Aridity drives plant biogeographical sub regions in the Caatinga, the largest tropical dry forest and woodland block in South America. PLoS ONE 13, e0196130. doi: 10.1371/journal.pone.0196130
Souza, D. N., Valera Camacho, R. G., Miranda de Melo, J. I., Galdino da Rocha, L. N., and Silva, N. F. (2014). Estudo fenológico de espécies arbóreas nativas em uma unidade de conservação de caatinga no Estado do Rio Grande do Norte, Brasil. Biotemas 27:31. doi: 10.5007/2175-7925.2014v27n2p31
Sterck, F., Markesteijn, L., Schieving, F., and Poorter, L. (2011). Functional traits determine trade-offs and niches in a tropical forest community. Proc. Natl. Acad. Sci. U.S.A. 108, 20627–20632. doi: 10.1073/pnas.1106950108
Stratton, L., Goldstein, G., and Meinzer, F. C. (2000). Stem water storage capacity and efficiency of water transport: their functional significance in a Hawaiian dry forest. Plant Cell Environ. 23, 99–106. doi: 10.1046/j.1365-3040.2000.00533.x
Vleminckx, J., Fortunel, C., Valverde-Barrantes, O., Timothy Paine, C. E., Engel, J., Petronelli, P., et al. (2021). Resolving whole-plant economics from leaf, stem and root traits of 1467 amazonian tree species. Oikos 130, 1193–1208. doi: 10.1111/oik.08284
Westoby, M. (1998). A leaf-height-seed (LHS) plant ecology strategy scheme. Plant Soil 199, 213–227. doi: 10.1023/A:1004327224729
Keywords: below-ground traits, Caatinga, drought, functional strategies, bark-traits, seasonally dry topical forests, stressful environment
Citation: Fagundes MV, Souza AF, Oliveira RS and Ganade G (2022) Functional traits above and below ground allow species with distinct ecological strategies to coexist in the largest seasonally dry tropical forest in the Americas. Front. For. Glob. Change 5:930099. doi: 10.3389/ffgc.2022.930099
Received: 27 April 2022; Accepted: 07 September 2022;
Published: 03 October 2022.
Edited by:
Leonel Lopez-Toledo, Universidad Michoacana de San Nicolás de Hidalgo, MexicoReviewed by:
Bradley Christoffersen, The University of Texas Rio Grande Valley, United StatesLuciana F. Alves, University of California, Los Angeles, United States
Fernando Pineda García, National Autonomous University of Mexico, Mexico
Copyright © 2022 Fagundes, Souza, Oliveira and Ganade. This is an open-access article distributed under the terms of the Creative Commons Attribution License (CC BY). The use, distribution or reproduction in other forums is permitted, provided the original author(s) and the copyright owner(s) are credited and that the original publication in this journal is cited, in accordance with accepted academic practice. No use, distribution or reproduction is permitted which does not comply with these terms.
*Correspondence: Marina Vergara Fagundes, bWFydmVmYSYjeDAwMDQwO2dtYWlsLmNvbQ==