- 1School of Geography and Environmental Science, University of Southampton, Southampton, United Kingdom
- 2School of Biological Sciences, University of Southampton, Southampton, United Kingdom
Climate change is expected to adversely affect the crop yields and food security for many smallholder farmers in the tropics unless adaptive measures are implemented. Agroforestry ecosystem services, such as micro-climate buffering, have received growing attention from the academic and policy communities for alleviating the negative impacts of climate change on smallholders. These benefits imply that agroforestry could offer a suitable measure for adaptation to climate change. However, whether agroforestry systems themselves succumb to the adverse effects of climate change is often less studied in the agroforestry literature. Consequently, less is known about how climate change will impact agroforests. We conducted a systematic review, which included an evidence quality assessment, to examine the impacts of climate change on tropical agroforestry systems (TAFS). Based primarily on studies undertaking biophysical approaches, we found that climate change negatively impacts TAFS by reducing tree growth, intensifying tree-crop resource competition and reducing crop yields. However, the impacts on smallholder farmers are less clear due to limited evidence in the relevant literature. We found that the evidence supporting our findings is mostly “robust”, although “least robust” strength evidence was also commonly found. We conclude that to improve understanding of how climate change could affect the performance of TAFS as a social ecological system, more interdisciplinary studies are required. Furthermore, to improve the quality of evidence in the research field, studies should explore using mountain elevation gradients for climate analog analysis to perform the most robust study designs. We provide an interdisciplinary conceptual model, which considers the interactions and feedbacks between TAFS components noted from our review to predict the response of ecosystem services provisioning and farmers' wellbeing to climate change, to guide interdisciplinary studies using climate analog analysis.
Introduction
Agroforestry, termed tropical agroforestry systems (TAFS) in the tropics, involves the integration of trees into farmland for socio-economic and ecological benefits (Schroth et al., 2004). Using a broad definition of trees in agricultural land, TAFS cover around 12.3 million km2 of global farmland (approximately 25% of total agricultural land) with high coverage in Southeast Asia, Central America, eastern South America and central and coastal West Africa (Zomer et al., 2016). TAFS usually display greater animal and plant species per unit area and more canopy layers than other agroforestry systems (Pathak and Dagar, 2000). The types of agroforestry systems are defined by their structure (i.e., arrangement of components), function (i.e., acting as windbreaks), socio-economic purpose (i.e., commercial or subsistence), and ecological characteristics (i.e., ecological conditions that influence which agroforestry systems can be practiced) which pertain to the environment of a geographic region (Nair, 1993; Dagar and Tewari, 2017). The main types of TAFS include multipurpose trees on woodlots (community forests providing forest products) and cropland, taungya systems (short-term crops cultivated with cleared and re-planted forest) and homegardens (intermixing and layering of trees with plants and crops at different vertical canopy strata) (Table 1). Homegardens, are particularly diverse systems, usually exhibiting the richest biodiversity per unit area of all agroforests (Dagar and Tewari, 2017). The biotic features of a TAFS are influenced mainly by their environmental ecological and agroclimatic conditions (Atangana et al., 2014). For example, TAFS found in the humid highland tropics exhibit higher species richness than those in highland semiarid tropics due to differences in temperature and rainfall (Dagar and Tewari, 2017).
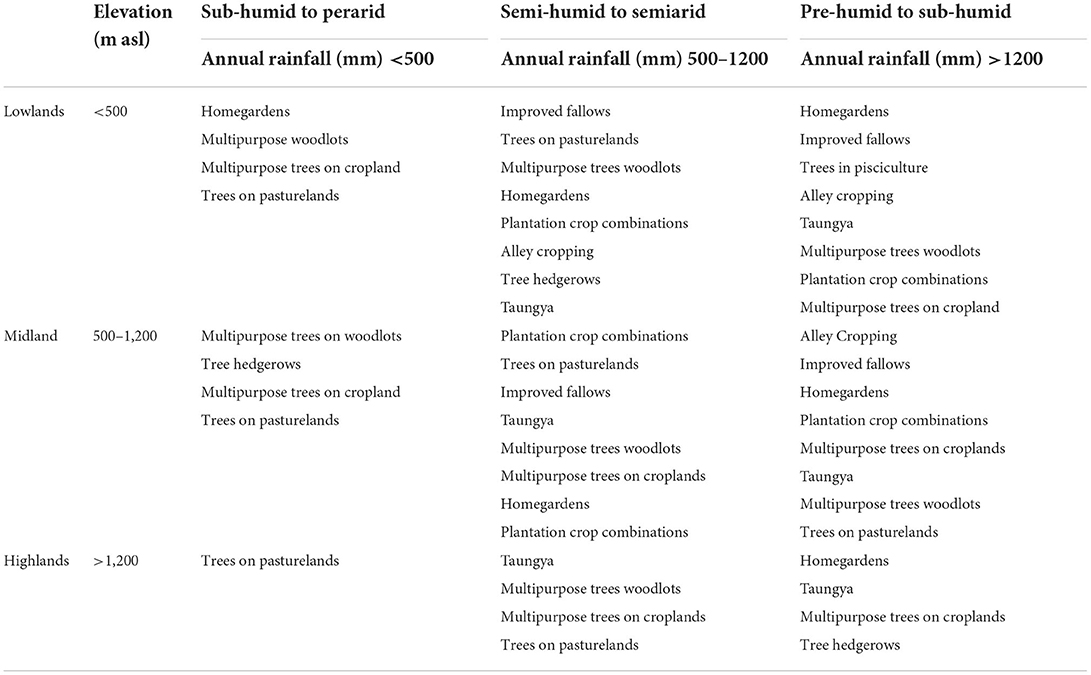
Table 1. Spread of the main types of tropical agroforestry systems based on supporting ecological conditions.
TAFS are important for the wellbeing of rural communities. These systems provide people with crops, fruits, and products which can be exchanged amongst communities to establish and reinforce social relationships (Blanckaert et al., 2004; Maroyi, 2009; Hashini Galhena et al., 2013). Such systems supporting indigenous crops and plants also support people's cultural values (Blanckaert et al., 2004; Linger, 2014). Homegardens, for example, enable people to manage traditional crops and plants belonging to their cultural history (Blanckaert et al., 2004), whilst coffee produced in TAFS allows people to partake in important religious coffee-related ceremonies within their communities (Linger, 2014). Regarding health and nutrition, TAFS provide diverse livestock products, fruit, and vegetable crops high in dietary micronutrients, calories, and protein (Talukder et al., 2000; de la Cerda and Mukul, 2008; Whitney et al., 2018). Other products, such as medicinal plants, treat diseases and illnesses (Hashini Galhena et al., 2013; Landreth and Saito, 2014). Returns for TAFS crops can be higher than open-field crops (Marsh, 1998) and provide income for even the poorest households (Mitchell and Hanstad, 2004; Hashini Galhena et al., 2013). In Sub-Saharan Africa, for example, TAFS crops can account for over 60% of household income (Okigbo, 1990). Such income enables households to improve their living standards, such as housing and cooking fuel (Kumar and Nair, 2004), and access education (Vasey, 1985; Iannotti et al., 2009; Maroyi, 2009). Concerning people's freedom of choice, crop production in TAFS can enhance farmers' self-sufficiency (Kumar and Nair, 2004), whilst women's involvement in farming and selling TAFS products generates personal income benefitting their independence (Marsh, 1998).
In the tropics, climate change will create warmer and drier environments, increasingly variable rainfall regimes and more frequent climate extremes (Serdeczny et al., 2017; Siyum, 2020). In contrast to cooler global regions, rises in temperature are expected to be stronger in the tropics (Gasparrini et al., 2017). Just a 2°C temperature increase risks substantial decreases in some crop yields grown by the 396 million of the approximately 900 million people involved in agriculture, which would severely impact livelihoods, food security and wellbeing unless adaptive measures are implemented (Zomer et al., 2016; Roy et al., 2018). Consequently, agroforestry has received growing interest as an adaptation to climate change measure for smallholder farmers (Palm et al., 2010; Lasco et al., 2014; Campbell et al., 2016; Ram et al., 2016; Altieri and Nicholls, 2017; Waldron et al., 2017). Most low-income countries (63 out of 88) now mention agroforestry as an adaptation solution in their climate change inventories, with the most interest in the tropics (Rosenstock et al., 2018).
Proponents contend that TAFS increase farmers' climate resilience through their regulating ecosystem services (Verchot et al., 2007; ICRAF, 2008; Thangata and Hildebrand, 2012; Nguyen et al., 2013; Garedew et al., 2017; Vargas Zeppetello et al., 2022). Tree canopies can lower irradiance and ambient air and soil temperature through shading effects (Mohri et al., 2013; van Noordwijk et al., 2021). Tree shading can lower ambient temperatures by 4°C (Liu et al., 2019) and soil temperatures by 6–10°C (Vandenbeldt and Williams, 1992; Belsky et al., 1993). More recent research shows that in tropical silvopastoral systems cooling effects are positively associated with increased woody carbon density (Vargas Zeppetello et al., 2022). TAFS can thereby regulate humidity, soil moisture evaporation and plant evapotranspiration (Lin et al., 2008) and, in turn, reduce water losses from crops and sustain production during heat stress (Rahn et al., 2018; Sida et al., 2018). Tree roots also aid soil water infiltration, reduce surface runoff, and reduce soil erosion during rainfall (Vaast et al., 2008; Siles et al., 2010). Tree biomass transfer increases soil organic matter, which improves soil aggregation stability, water retention capacity and infiltration preserving soil moisture (Phiri et al., 2003; Sileshi and Mafongoya, 2006; Wu et al., 2016). Tree canopies can also intercept precipitation and reduce throughfall (Dietz et al., 2006; Ilstedt et al., 2016), which mitigates soil erosion and crop damage caused by heavy rainfalls (Thorlakson and Neufeldt, 2012). Foliated trees can reduce rainwater reaching crops by 10–50% (Rao et al., 1998), and in homegardens rainwater interception can reach 54% (Otte et al., 2017).
Provisioning ecosystem services from agroforests can also increase smallholder farmers' climate resilience through the provision of crops and other products directly to households (Sileshi et al., 2007; Quandt et al., 2018). The replenishment of soil nutrients by TAFS can permit the sustainable cultivation of various crops (Neupane et al., 2002; Jose, 2009; Ajayi et al., 2011) grown year-round under agroforestry's micro-climate effects (Charles et al., 2014). By supplying high diversities of crops and products (Abebe et al., 2006; Hashini Galhena et al., 2013; Jemal et al., 2018) TAFS can alleviate the risks of crop failure under unpredictable and adverse climates (Thorlakson and Neufeldt, 2012; Nguyen et al., 2013), and increase food stability (Charles et al., 2014). The household income generated through selling these TAFS goods can also increase climate resilience and reduce food insecurity through increased purchasing power (Nguyen et al., 2013; Lasco et al., 2016; Quandt et al., 2018). In addition, TAFS can quicken household economic recovery rates post-climate impact by supplying products, such as timber and livestock, for selling when cash is needed, which shortens recovery times (Simelton et al., 2015).
The above benefits provided by TAFS imply that farmers using these systems may be generally more climate-resilient than farmers engaged in open farming systems (Pramova et al., 2012; Thorlakson and Neufeldt, 2012; Pandey et al., 2015; Lasco et al., 2016; Quandt et al., 2018). However, studies assessing agroforestry benefits (Brown et al., 2018; Kuyah et al., 2019) and climate resilience (Linger, 2014; Paul et al., 2017) often use open farming as a control group. Whilst these studies provide important insight into whether TAFS could increase farmers' climate resilience relative to open farming, these studies do not explicitly assess the impacts of climate change on TAFS. Instead, these studies examine relative differences in resilience under climate change to elucidate TAFS' benefits. Consequently, less is known about exactly how climate change will impact agroforestry systems themselves (Neufeldt et al., 2012; Coulibaly et al., 2014; Odeny et al., 2019).
Over the last decade, however, there has been a recognition that increasing temperatures, carbon dioxide levels and changes in humidity and rainfall could affect agroforestry systems (Luedeling et al., 2014; Mbow et al., 2014). Climate change may shift ecological zones in tropical mountains and affect tree species distributions (Lin et al., 2013; Ranjitkar et al., 2016). Tropical tree growth and survival may also be impacted through effects on photosynthesis, respiration, and nutrient cycles (Siyum, 2020), as evidenced by non-agroforestry tree-based studies (e.g., Yin et al., 2018). If observed on TAFS trees, such impacts would likely affect the ecosystem services supporting farmers' climate resilience. Furthermore, as tree-soil-crop interactions mediate crop production in TAFS, the possibility of resource competition between trees and crops has generated much debate on whether agroforestry benefits crop yields (Bayala et al., 2019). In any case, the impact of agroforestry on crop yields are likely to hinge upon external environmental factors, including temperature and precipitation (Tscharntke et al., 2011; Bardgett et al., 2013; Blaser et al., 2018), which implies that climate change could impact crop yields in TAFS.
Regarding long-term adaptation planning in TAFS for climate change, we need clear evidence on whether and how the changing weather conditions can affect the systems. We systematically reviewed academic research on the effects of climate change on TAFS returned from our search string to identify gaps in current knowledge and to guide future research directions. Specifically, we mapped how the climate change research in TAFS is distributed by geographic region, country, methodology (i.e., empirical or modeling), and approach (i.e., biophysical, livelihoods, or interdisciplinary). We assessed the reliability of evidence presented in all research and summarized the impacts of climate change on the trees, soils and crops in TAFS, as well as the effects on livelihoods under the systems. Lastly, we proposed a study approach and an associated conceptual model to guide research priorities for addressing the identified knowledge gaps and improving the reliability of evidence.
Methods
Systematic review
In July 2022, we conducted a search of academic literature using three academic databases (Web of Science's core collection, Scopus, and Google Scholar) following guidance from the ROSES systematic review protocol (Haddaway et al., 2018) (Figure 1). We tested various combinations of keywords combined with appropriate Boolean operators and wildcards that returned hits that covered a range of impacts that could be linked with climate change, for example, drought. The final search terms applied were (“climat* change” OR “global warming” OR “temperature ris*” OR “temperature increas*” OR “climat* vari*” OR “rainfall dec*” OR “rainfall increas*” OR “rainfall vari*” OR “drought” OR “flood*”) AND (“tropic*”) AND (“agroforest*” OR “farm tree*” OR “homegarden*” OR “home garden*” OR “taungya” OR “alley farm*” OR “alley crop*” OR “multipurpos* tree*” OR “woodlot*” OR “hedgerow*” OR “improv* fallow*”). The search returned 1,071 hits which were then reduced to 728 once hits were refined to peer-reviewed published articles and duplicates were removed. We refined our sample to include only published articles to ensure that the reviewed literature had been peer-reviewed as an initial quality control check.
For the inclusion criteria, we applied the criteria that studies should consider one or more aspects of climate change impacts on agroforestry systems, including farmers themselves, in the tropics. Additionally, articles should be based on primary research, which means that review papers were omitted. We also omitted studies that assessed the impacts of climate change on trees not associated with agroforestry in the article. These criteria were applied in a two-stage filtering process, first to the returned abstracts and next in a full paper screening. For the hits returned from the Google Scholar search, these were ordered by relevance (high to low), and only the first 100 hits were reviewed to check that no hits had been missed in the primary databases. Also, relevant papers known to the authors which meet these criteria not returned in the search were added before data extraction. For data extraction, we classified the primary methodology that studies used, for example, whether studies conducted modeling or empirical data collection and analysis, what type of approach was used to discern the impacts [either livelihoods, biophysical, or interdisciplinary (considering both the livelihoods and biophysical impacts)], the direction of impacts (either positive, negative or no effect), and the year and location of the study.
Assessment of evidence quality
To critically assess the evidence provided by published articles, we followed an adapted version of the evidence assessment tool proposed by Mupepele et al. (2016) for ecosystem services and conservation studies. The evidence assessment tool scores the individual quality of studies based on study design and its execution. Studies are then categorized according to an evidence hierarchy based on their individual score (Figure 2).
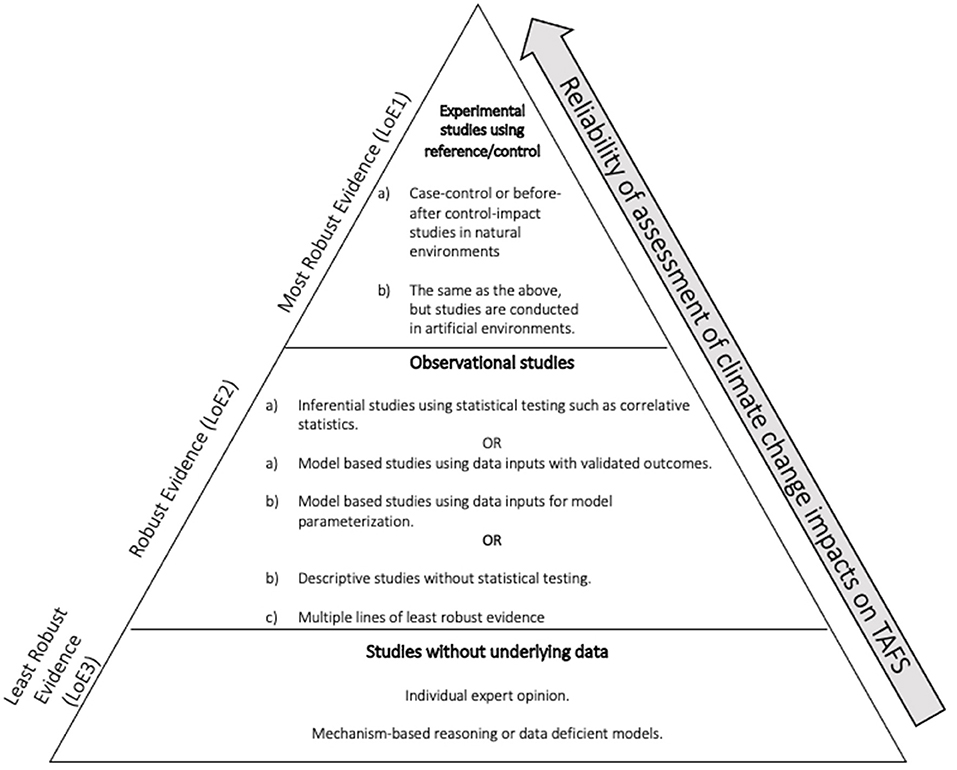
Figure 2. Hierarchy of evidence quality according to study design, with internally ranked sub-levels a, b and c. Adapted from Mupepele et al. (2016).
Assessment of study design
We designated experimental studies employing group or before-after controls as the most robust sources of level of evidence (LoE1), i.e., studies where the TAFS is exposed to a climate parameter representing climate change. The impacts of climate change are assessed relative to a control TAFS or when an open farming system is used to highlight the impacts specifically on the TAFS. Within LoE1, experimental studies performed in natural environments provide the highest level of evidence (LoE1a), whilst experimental studies performed in artificial environments provide slightly less robust evidence, and thus were placed in LoE1b. Experimental studies conducted in artificial environments may not capture agroforestry's cross-scale effects' (van Noordwijk et al., 1996) and can neglect broader real-world interactions and effects that can influence study outcomes (Coe et al., 2014; Coulibaly et al., 2017). Nevertheless, such studies performed in artificial environments can more precisely assess the effect of climate factors on TAFS and can better associate the recorded impacts with climate change by simulating changes expected over more extended periods, i.e., more than 10 years.
The next level of evidence is “robust” (LoE2), which comprises observational study designs (Mupepele et al., 2016). Within this level of evidence, studies which draw inferences from correlative statistical testing are ranked LoE2a. For example, studies which statistically test the influence of a changing climate parameter on the quantity of a TAFS ecosystem service such as tree fruit production. Descriptive studies without statistical testing, such as studies presenting frequencies or data summaries, that provide weaker association between climate change and TAFS provide slightly less robust evidence, and were subsequently placed in LoE2b.
Modeling-based studies are commonly employed in climate change research to predict the impacts of anticipated future climate conditions. However, modeling the impacts of climate change on complex tree-soil-crop interactions in agroforests can produce uncertain predictions (Luedeling et al., 2014). Studies using modeling designs can therefore produce less robust evidence than empirical experimental studies, and thus were placed in LoE2. Within LoE2, model predictions made using field data for model parameterization and have corroborated predictions produce the most robust evidence (LoE2a), whilst model predictions not validated produce less robust evidence (LoE2b) (Mupepele et al., 2016). These standards are pertinent to TAFS modeling-based studies because to make inferences for the targeted TAFS, the data used should derive from the study site. Furthermore, given the levels of uncertainty in modeling approaches for projecting climate change impacts on TAFS, corroborating model predictions is key for proving evidence reliability. Predictions made from mechanistic models lacking field data produce the least robust evidence for discerning the effects of climate change on TAFS (LoE3), alongside expert opinion (Mupepele et al., 2016). However, multiple lines of LoE3 evidence can increase study reliability to robust levels of evidence (LoE2c).
Critical appraisal of study design implementation
Singularly considering study design as an indication of evidence quality is insufficient because the execution of the methodology may have been less rigorous (Rychetnik et al., 2002). Consequently, a critical appraisal of the implementation of the study design was also undertaken using the methodological quality checklist developed by Mupepele et al. (2016). This checklist was devised using information from 30 published quality checklists known for evaluating evidence-based practice. The aspects covered include setting the research aim, data collection, analysis, presentation of results and the conclusions drawn and more specific questions for some individual studies based on their design (e.g., control group studies have an additional five checklist questions). This seeks to highlight any bias and inferential error impacting the validity of the evidence. The checklist, however, does not provide specific detailed questions for all study approaches (for example, there are no specific questions for modeling-based studies) and so should not be considered entirely comprehensive (Mupepele et al., 2016). However, the aforementioned criteria were deemed sufficient to appraise all modeling and non-modeling studies included in our review.
We used the quality checklist to derive a percentage score for each study (each question answered “Yes” = 1 point; “No” = 0 points). Questions not applicable to a study given its methodology were not factored into the percentage score. Depending on the score, the study can be moved down a sub-category or category due to a less rigourous execution of study design (see Table 2). The amalgamation of the study design (Figure 2) and quality checklist score is the final step to determine the quality of evidence for supporting the study conclusions (Mupepele et al., 2016).
Results
Descriptive statistics of published articles
A total of 35 studies were retained following the search and two-stage inclusion criteria (Figure 1). Three known papers, which did not explicitly mention climate change-related terms and tropical agroforestry in the title or abstract, but assessed the impacts, were added, bringing the total to 38 studies. Regarding the methodology used, most studies undertook empirical data analysis (n = 33), with much fewer undertaking modeling (n = 5) which focused on simulating the future impacts of climate change (Figure 3A). By year there has been an increase in the number of published papers over the last decade, with the numbers of papers peaking in 2018 (n = 6) and 2019 (n = 5) (Figure 3B). Most studies undertook a biophysical approach to assess the impacts of climate change (n = 31), whilst fewer undertook an interdisciplinary (n = 6) or livelihoods approach (n = 1) (Figure 3C). In total, most studies found that the impacts of climate change were negative (n = 27), and only a minority of studies concluded either no effect (n = 9) or a positive impact (n = 2) (Figure 3D). Geographically, studies have been conducted in South and North America, Eastern and Western Africa, and South and Southeast Asia, with most studies conducted in India (n = 6) and Indonesia (n = 5) respectively (Figure 4).
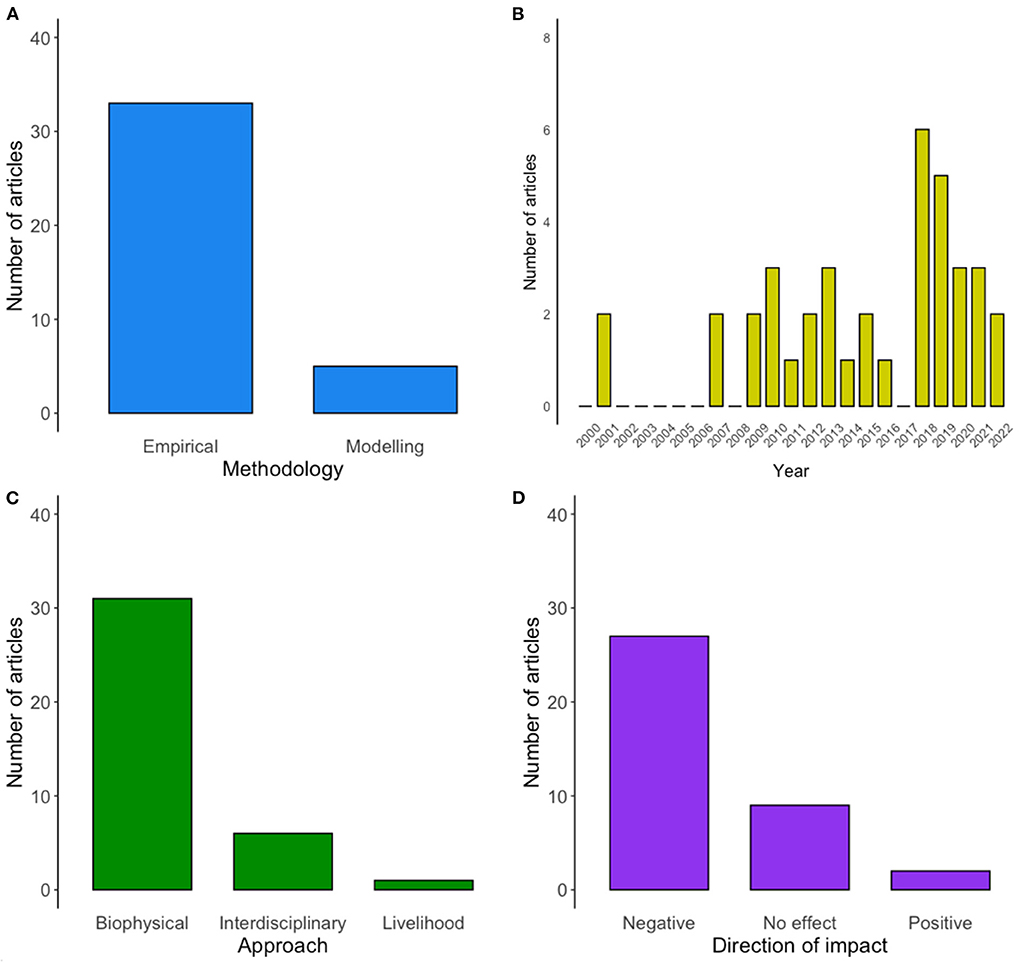
Figure 3. Number of published articles globally on the impacts of climate change by (A) methodology, (B) year starting from 2000, (C) approach, and (D) direction of impact recorded.
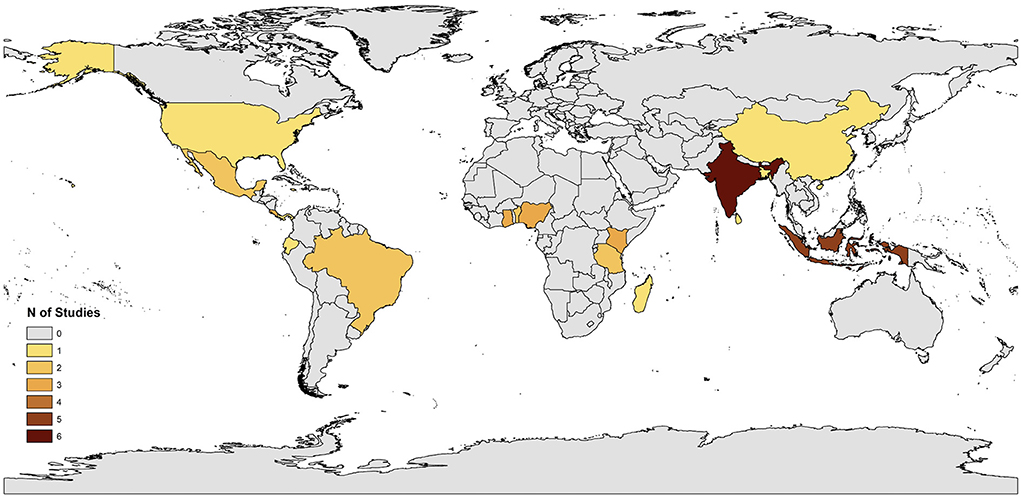
Figure 4. Global distribution of published articles on the impacts of climate change on TAFS per country.
Assessment of the evidence on the impacts of climate change on TAFS
Based purely on study design, studies providing LoE2a strength evidence are the most common (n = 13, 34.2%), which could be accredited to climate events, such as droughts, providing the conditions required to assess the effect of climate on TAFS. Next most common are studies providing LoE1b (n = 10, 26.3%) and LoE2b (n = 10, 26.3%) strength evidence (Figure 5A). No LoE3 study designs were found, and no modeling-based studies using field data corroborated their predicted impacts of climate change, which limited their evidence to LoE2b.
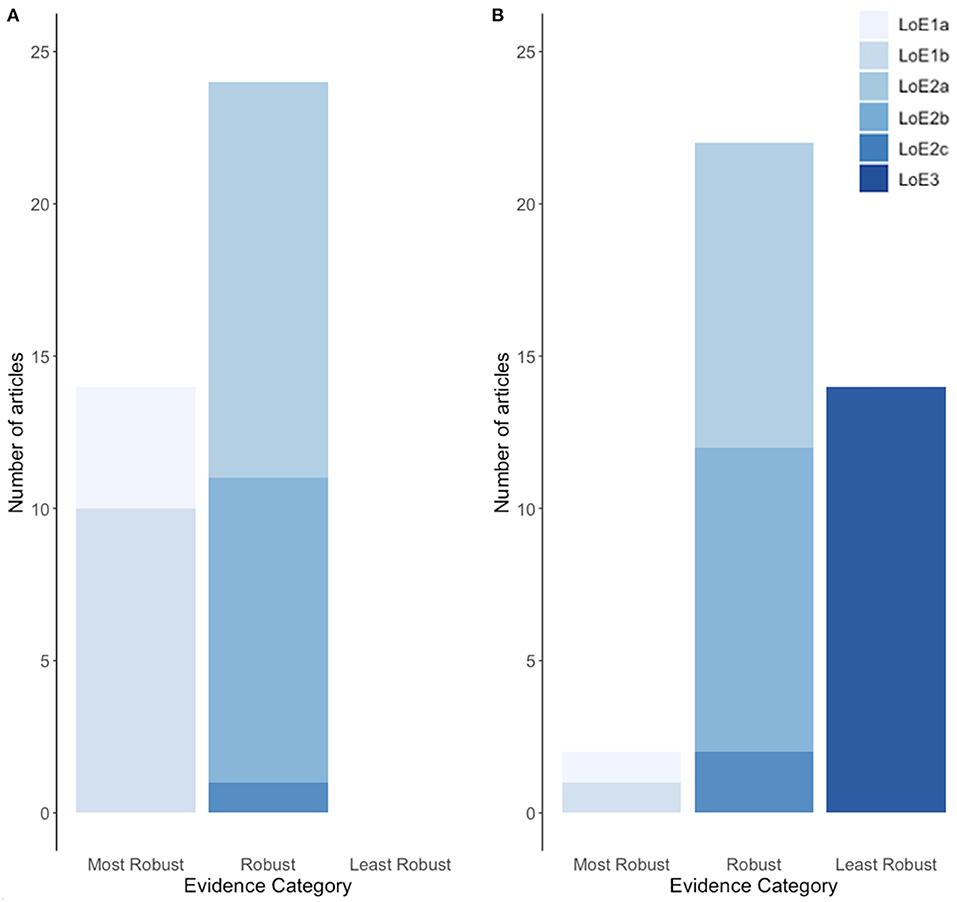
Figure 5. Evidence assessment scores for study design (A) and adjusted scores based on critical appraisal of study quality (B).
Following the appraisal of methodological quality, most studies were moved down in their LoE (n = 32, 84.2%) and 20 (62.5%) studies were moved down by a whole level or more (Figure 5B). This indicates that studies assessing the climate change impacts on TAFS commonly lack rigor in their execution, which could be due to their difficulty and costliness to set-up and implement in the field, and supports the notion that assessing study design alone is insufficient to determine evidence quality. Overall, studies performed well in data collection aspects such as defining the study population of interest and mitigating detection bias, as well as ensuring that their conclusions were well supported by the evidence. Studies performed less well in data collection aspects such as blind sampling, randomly allocating case-control groups, ensuring any surveys (if used) were piloted and secondary data (if used) were critically evaluated before use. By study design, modeling studies were implemented most reliably, scoring on average 83%, followed by experimental control group approaches (75%) and observation studies (66%). The lack of specific checklist criteria for modeling did not likely influence scores because experimental control group approaches scored on average 9% higher than observational studies despite having more criteria (5 compared to 1). Our synthesis of the literature presented in the following sections are mainly based on LoE3 strength evidence (n = 14) followed by LoE2a and LoE2b strength evidence (n = 10 each), with few cases of LoE1 strength evidence (n = 2) (Figure 5B).
The impact of climate change on TAFS
Studies using a biophysical approach to assess the impacts of climate change on TAFS
The impacts recorded in these studies are grouped under four themes that emerged during data synthesis: TAFS trees (including tree growth, distribution and mortality), tree fruit production, soils, and tree-soil-crop interactions. Some studies involved cross-cutting themes.
The impacts of climate change on TAFS trees
Our knowledge about the impacts of climate change on tree growth and distributions is based on LoE2a, LoE2b, and LoE3 strength evidence. LoE2a evidence from several studies indicate that reduced rainfall, water stress and increasing drought severity, which are expected under climate change, could limit the height and root growth of trees and plants in TAFS, including Amomum villosum (Feng and Li, 2007), Azadirachta indica (Puri and Swamy, 2001), Tectona grandis (Kumar et al., 2021), Guazuma ulmifolia, Albizia lebbeck, Leucaena leucocephala, Piscidia piscipula and Lysiloma latisiliquum (Tamayo-Chim et al., 2012). Although the consensus in these studies is that drought can negatively affect tree growth, some evidence revealed slight variation in responses. Legume tree species, for example, can exhibit greater resilience to mild drought than non-legumes (Tamayo-Chim et al., 2012). Compared to Tectona grandis, the growth rate of Acacia mangium and Eucalyptus camaldulensis was not significantly affected by reduced amounts of rainfall although their ability to sequester carbon declined (Kumar et al., 2021). In addition, LoE2b strength evidence revealed that Leucaena leucocephala seedling growth is negatively impacted by drought. However, these impacts are reduced in seedlings infected with Rhizobium tropici (root nodule bacteria) (Pereyra et al., 2015). On the contrary, we also have LoE2a strength evidence, which suggest that climate change, manifested through a combined effect of increased atmospheric temperatures and elevated CO2, could benefit trees species (Cedrela odorata and Gliricidia sepium) in terms of faster growth (Esmail and Oelbermann, 2011). However, the increased growth rate comes with a trade-off: the reduction of leaf nutrient value. Less robust evidence indicates that increased temperatures, drought, and decreased annual rainfall can impair the seed germination of Garcinia kola to the point that agroforestry farmers no longer plant the tree species to gain tree fruit (Agwu et al., 2018). Overall, the current evidence denotes that climate change is likely to negatively affect tree and plant growth in TAFS, albeit with some intra-species variation. Our evidence also suggests that legumes and trees infected with symbiotic bacteria could be more resilient to drought impacts.
According to LoE2b strength evidence, whether a future climate scenario entailing warmer temperatures and higher annual rainfall drives the upslope or downslope migration of tree species in montane environments and changes in their aboveground carbon can depend on emission scenarios and TAFS geographic location. For example, under RCP 4.5 projections in Tanzania, suitable climate conditions could shift upslope for Albizia gummifera and Persea americana and downslope for Mangifera indica, though under RCP 8.5 projections, suitable climate conditions for Persea americana could shift downslope (Odeny et al., 2019). Whether these tree species increase or decrease in their aboveground carbon may also vary depending on the different future climate scenarios they are exposed to; however, in Kenya, all these tree species except Albizia gummifera may decline in aboveground carbon irrespective of the different climate scenarios. In contrast, similarly robust evidence suggests that aboveground carbon in TAFS in India could remain unchanged (Russell and Kumar, 2019), whilst in Nigeria forecasted declines in rainfall amounts during the hottest months could reduce the land suitable for supporting Garcinia kola by up to 35.8% based on LoE3 evidence (Agwu et al., 2020). These findings indicate that the future impacts of climate change could be positive, negative or have no effect on tree distribution and size.
We found varied results concerning the impacts of climate change on the abundance and size of trees at the species level in TAFS. The abundance of Vitex doniana was unaffected by differences in climates comprising wet, intermediate, and dry climatic conditions (Hounkpèvi et al., 2018). However, the size of Vitex doniana significantly differed across different land cover types, but only those found in cropland significantly differed across climatic zones. This LoE2a strength evidence suggests that land cover type mainly effects the size of Vitex doniana, but climate change may impact Vitex doniana size if it is grown in croplands. In contrast, slightly less robust evidence (LoE2b) suggests that climate change through drought significantly and negatively affects tree size at the species level in TAFS (Vincent et al., 2009). The extent of decrease in size linked with drought varied according to the tree species and size. For example, Saurauia nudiflora, a large tree, declined by 80% in diameter at breast height compared to a 5% decrease in the smaller Archidendron bubalinum. The divergence in findings across these studies may be due to interspecies variation in responses to changes in climate conditions, as well as the harsher change in climate under drought.
The relationship between drought induced by climate change and tree mortality is equivocal. We found LoE2a strength evidence that severe drought can increase tree seedling mortality by 35–40% due to the inability to match transpiration demands (Puri and Swamy, 2001). However, equally robust evidence suggests that exposure to drought does not increase tree seedling mortality, despite impairments in their growth (Tamayo-Chim et al., 2012). We found similar discrepancies in studies reporting LoE2b strength evidence. For example, Gateau-Rey et al. (2018) found that drought could increase tree mortality for cacao trees and their shade trees in Brazil, implying that tree shading may not be effective against drought. Yet, Vincent et al. (2009) found no link between climate change-induced drought and tree mortality despite the extreme event clearly reducing tree size. Such discrepancies may be due to interspecies variation in responses to drought, for example, by reducing their size to adapt to drought and differences in drought severity. We also have 'least robust' evidence (LoE3), which shows that other climate extremes concerning flooding increase tree mortality in cacao TAFS. Tree mortality associated with flooding can be nuanced by age, however, with older TAFS potentially more vulnerable (Somarriba et al., 2001).
Impacts of climate change on tree fruit production in TAFS
A climate resilience benefit of TAFS is the ability to supply tree fruits and products to smallholder farmers when the climatic condition for crop production is not favorable. However, extreme weather induced by climate change can also negatively affect fruit production. This is supported by both robust (Schwendenmann et al., 2010; Peters and Carroll, 2012; Arnold et al., 2018; Gateau-Rey et al., 2018) and least robust evidence (Boissière et al., 2013; Tregidgo et al., 2020; Wagner et al., 2021).
Drought could decrease cacao yields by reducing the production of cacao pods per tree, often through outbreaks of disease (Gateau-Rey et al., 2018). However, LoE2a evidence indicates that declines in cacao yields in TAFS under drought are unlikely to be driven by disease (Schwendenmann et al., 2010). Instead, drought could reduce cacao disease outbreaks by creating less moist and humid environments, suggesting that other factors associated with climate may reduce cacao yields. We also have robust evidence demonstrating that both climate extremes (wet and dry) could negatively impact fruit production in TAFS by affecting pollinators. More intense and unpredictable rainfall can adversely impact coffee yields by increasing coffee flowering at times asynchronous with bee pollinators (Peters and Carroll, 2012). Drought can also hinder cacao yields in TAFS by depleting moisture needed for Ceratopogonidae (cacao midges and pollinators) larvae, reducing their abundance (Arnold et al., 2018). Our least robust evidence suggested that extreme hot weather could also reduce acai fruit production by preventing the trees from bearing fruits (Tregidgo et al., 2020), and severe storms could destroy fruit trees in homegardens and cause a direct loss of fruit production (Boissière et al., 2013). Equally weak evidence, however, suggests that it is unlikely that higher temperatures and increasing rainfall would increase the prevalence of coffee tree pests in TAFS (Jaramillo et al., 2013), though coffee fruit size and quality are still likely to decline from the associated accelerated ripening (Wagner et al., 2021).
Impacts of climate change on soils in TAFS
Our knowledge of the impacts of climate change on TAFS soils is based on most robust (LoE1a), robust (LoE2a to LoE2b) and least robust (LoE3) evidence. According to the most robust evidence, drought could negatively impact TAFS soils by reducing plant coverage and subsequently soil biota which are needed for soil nutrient cycles (Lakshmi et al., 2021). This is congruent with robust evidence which suggests that drought could reduce soil biota productivity in TAFS, as indicated by declining soil CO2 efflux, although only under severe drought (van Straaten et al., 2010). Similarly, model simulations suggested that increases in air temperature will drastically decrease soil C content, but only under a considerable temperature increase (+6°C) as opposed to a marginal increase (+2°C) (Russell and Kumar, 2019). In contrast, Andriamananjara et al. (2019) suggested that temperature increase (+10°C) will increase soil C and N content, possibly through increased soil biota activity and mineralization rates. However, this finding was based on an artificial experiment that maintained soil moisture at a constant level and may not properly reflect what occurs in TAFS soils when exposed to hotter climates, as well as less robust evidence (LoE3). Hence, increasing temperature is more likely to reduce soil C and biota activity productivity in TAFS based on the more reliable evidence. Concerning forecasted increases in rainfall amounts and intensity, the associated flooding and increased run-off could significantly increase soil nutrient loss and erosion in TAFS, as indicated by LoE2b (Choudhury et al., 2022) and LoE3 (Balasubramanian et al., 2015) strength evidence. Soil erosion in TAFS, however, may be less severe than in open farming systems (Choudhury et al., 2022). Overall, we cannot conclusively assert that climate change would negatively affect soils due to a limited number of soil properties assessed by only six studies.
Impacts of climate change on tree-soil-crop interactions in TAFS
Whether climate change negatively affects tree-soil-crop interactions in TAFS can depend on the crop type, the tree species and the study design used to assess the impact. We found LoE1b strength evidence which demonstrated that drought can negatively influence tree-soil-crop interactions in cacao TAFS (Blaser et al., 2018). We also have LoE2a strength evidence supporting similar findings in cacao TAFS which encompassed Albizia ferruginea and Antiaris toxicaria shade trees (Abdulai et al., 2018). Under warmer and water-limited environmental conditions, competition between trees and cacao plants for scarce soil water resources intensified (Blaser et al., 2018). This can reduce soil moisture and cacao yields below levels in open-sun cacao farms. Drought conditons have also been shown to cause more significant water loss in cacao TAFS than in open-sun cacao farms as a result of the combined transpiration rates of both cacao plants and trees (Abdulai et al., 2018). This, in turn, intensifies water resource competition and can lead to cacao plant mortality. Similar resource competition for limited soil water has also been reported in maize and Grevillea robusta TAFS, which delays the flowering and development of maize and can lead to crop failure (Lott et al., 2009).
On the other hand, we also have LoE2a (Schwendenmann et al., 2010; Muñoz-Villers et al., 2020) and LoE2c (Köhler et al., 2010) strength evidence which implied that cacao and coffee in TAFS were not impacted by drought. Contrary to Blaser et al. (2018) and Abdulai et al. (2018), Schwendenmann et al. (2010) and Köhler et al. (2010) found that when cacao is cultivated with Gliricidia, water stress in cacao plants is low. This can be due to shading effects and complementary root structures, which involve crop roots occupying shallow soil layers whilst tree roots extract water from deeper soils. This helps preserve and more efficiently use limited water resources. Their evidence, however, was generated using artificial drought conditions created under roof plots, which can poorly resemble changes in temperature, radiation, and vapor pressure deficits that are also altered by drought (Schwendenmann et al., 2010). Hence, to develop knowledge of how climate change could affect tree-soil-crop interactions in TAFS, more research performed in natural environments using tree species and crops varying in root structures and depths is necessary.
Studies using a livelihood or interdisciplinary approach to assess the impacts of climate change on TAFS
Livelihood approach to assess climate change impacts
We found only one study using a livelihoods approach to assess the impacts of climate change on TAFS (Onyekuru and Marchant, 2016). This model-based assessment provided LoE2c strength evidence that there was no significant impact on household net revenues under the simulated future climate scenario of an increased temperature by 1°C and a 7% decreased annual rainfall in Nigeria. However, a low model R2 value (0.11) indicated that other important variables influencing net revenue were absent, and the model did not account for biophysical interactions between forests and climate change. Therefore, this assessment could potentially be underestimating the impacts.
Interdisciplinary approach to assess climate change impacts
All six studies using an interdisciplinary approach yielded LoE3 strength evidence. The negative impacts in these studies were mainly analyzed and presented through descriptive summarizes of survey data collected in Indonesia (Boissière et al., 2013), Nigeria (Agwu et al., 2018) and Ecuadorian highlands (Córdova et al., 2019). Biophysical impacts identified by these studies included adverse effects on crop yields, fruit production, fodder production, soil moisture, water quality and quantity and biodiversity, whilst the livelihood consequences involved negative effects on livestock, household income, cultural values, health, and the displacement of farmers from increasing temperatures, drought, and declines in annual rainfall. Conversely, a hypothetical study conducted in Hawaii found that the flammability of TAFS and groundwater recharge under this land use is likely not to be affected by increasing temperatures (+ 2°C), whilst farmers could expect higher economic returns for their produce under the warmer climate (Bremer et al., 2018). Similarly, farmers in Benin perceived that ecosystem services from TAFS, such as the supply of raw materials and food production, were unaffected by increases in drought, temperature and rainfall intensity (Belfrid et al., 2022). However, the exact details of how the biophysical and livelihood impacts intersected in these interdisciplinary studies were lacking. Therefore, it is unclear how climate change impacts the TAFS as a social-ecological system as a whole.
In contrast, an interdisciplinary assessment of the impacts of climate change on homegardens in Sri Lanka adopted an interdisciplinary conceptual framework to elucidate how climate change affected interactions and feedback between the homegardens biophysical and livelihood and farm management components (Landreth and Saito, 2014). Guided by their framework, their evidence implies that increasing rainfall variability and intensity enhances soil erosion and landslides and destroys farmers' food crops. In response, farmers then increased their food purchases and partially abandoned their homegardens. This, in turn, can increase wild animal incursions, further destroying crops and forcing farmers to simplify their crop production to inedible but drought-resistant cash crops. These consequences that negatively affected biodiversity (biophysical) but improved household income (livelihood) signal that climate change can create environmental and livelihood trade-offs in TAFS.
Discussion
The impacts of climate change on TAFS
Our review found that TAFS were mostly negatively affected by climate change. Climate change, manifested through increased temperature, changes in rainfall amounts and intensity and drought, may hinder agroforestry farmers' climate resilience in dealing with the decline in ecosystem services. These ecosystem services from TAFS are necessary to increase farmers' household resilience against climate change (Nguyen et al., 2013; Pandey et al., 2015; Quandt et al., 2017), and our results show that climate change mostly negatively impinges on their provision. To increase their resilience, farmers could plant leguminous trees and other tree species that our review found can be less sensitive to climate change, including Acacia mangium, Eucalyptus camaldulensis, Cedrela odorata, Gliricidia sepium and Vitex doniana, as well as tree species which support root nodule bacteria.
The negative impacts of climate change, particularly climate change-induced droughts, on tree mortality and growth in TAFS summarized in this review are mainly consistent with some tree-based climate studies in the tropics (e.g., Midgley and Thuiller, 2011; Yin et al., 2018), but contrasted with other studies (Koch and Kaplan, 2022). Concerning the impacts on TAFS fruits and crops, under some cacao-based TAFS droughts can cause lasting post-drought impacts on yields due to increased cacao plant mortality (Abdulai et al., 2018; Gateau-Rey et al., 2018), despite the initial general claim that TAFS quickens household economic recovery post-climate extreme (Simelton et al., 2015). Changes in rainfall amount and timing can also lower yields in TAFS by impeding the growth of fruit trees, crop development, flowering, and pollination (Peters and Carroll, 2012; Agwu et al., 2018; Wagner et al., 2021), with some crops producing much lower yields in TAFS than open farming systems (Lott et al., 2009). However, our review also found evidence suggesting that some TAFS crops may be unaffected by climate change (Köhler et al., 2010; Jaramillo et al., 2013), albeit supported by less robust evidence. Our limited evidence on the impacts to TAFS soils suggested that whether drought also negatively impacts TAFS soils could depend on drought severity. However, our knowledge regarding the impacts on soil is restricted by only a paucity of relevant studies. This also holds for our knowledge of the impacts of climate change on livelihoods in TAFS.
Concerning current debates on whether tree-soil-crop interactions in TAFS are complementary or competitive for natural resource use (Bayala et al., 2019), the findings from our review implied that under drought and water-limited environmental conditions, interactions can depend on tree-crop root systems. Robust evidence derived through field experiments reinforced some experts' opinions that climate change could impact ecological interactions in agroforests (Tscharntke et al., 2011; Luedeling et al., 2014). This could have implications for adaptation to climate change as positive climate buffering effects in TAFS could be outweighed by negative effects, including increased water loss and enhanced resource competition. This knowledge, however, was based on a limited number of studies. However, our review also found robust evidence supporting agroforestry proponents who maintain that complementary root structures in TAFS can protect crops against climate change (e.g., van Noordwijk et al., 2021). This evidence, however, was derived through studies mostly conducted in artificial conditions, which may not properly simulate actual drought conditions (e.g., Schwendenmann et al., 2010). The lack of experimental studies in the research field which examine the effects of climate change on tree-soil-crop interactions within TAFS encompassing more complementary root structures in natural environments limits what we are able to conclude from the available evidence.
Reliability of evidence
We found that studies primarily presented either robust (LoE2) or least robust (LoE3) evidence of climate change impacts. The shortage of “most robust” (LoE1) evidence could be due to the difficulty in devising a natural experimental control group approach encompassing different climate conditions. Most experimental controlled studies exploited drought events, which are only one aspect of climate change, or needed to artificially create climate conditions that can limit real-world applicability. However, we still acknowledge the value added by artificial experiment studies' to the field. No modeling-based studies corroborated their predictions, which restricted their predictions to LoE2b. We appreciate, however, that the long-term future climate scenarios targeted in modeling studies can make ground-truthing surveys challenging.
Few studies used a livelihood and interdisciplinary approach, and these studies only presented the least robust (LoE3) evidence. These studies provided limited insight as they only offered a “snapshot” of the impacts at a particular time and mainly displayed the recorded impacts through data frequencies. This restricted our understanding into how climate change was associated with their documented impacts. Agroforestry systems encompass close interactions between their biophysical and social elements (Elbakidze et al., 2021), where impacts on one element will impact the other through mutual relationships and feedbacks (Liu et al., 2007; Ibarra et al., 2021). Such descriptive and “snapshot” studies which overlook these relationships and feedbacks cannot sufficiently capture the multi-faceted nature of climate change impacts on TAFS. Interdisciplinary conceptual frameworks were shown to have the potential to capture these mechanisms and impacts (Landreth and Saito, 2014).
Limitations
Our review is presented with some limitations. Firstly, our review findings were based on a limited number of studies. We believe, however, that the small sample of relevant studies is an accurate reflection of the current literature on the review topic. Secondly, the findings presented in our review have also been established from English academic literature, which can bias results (Amano et al., 2016). Finally, we did not include gray literature in our review including reports, theses and dissertations, and as such, our findings are representative of published academic research.
Future research priorities
Overall, we found limited evidence on how climate change will impact the ecosystem services provided by TAFS. Particularly understudied areas include the impacts on the livelihoods of smallholders, and their wellbeing (i.e., cultural, material, food and health). Therefore, we are uncertain about the potential adverse impacts of climate change on people in TAFS. Discerning the potential impacts of climate change on crop production in TAFS and farmers' wellbeing is vital for long-term climate change adaptation planning. Trees are long-lived organisms, and their establishment in farmland requires resource investment (Luedeling et al., 2014). Hence, it is important to ascertain whether investing in TAFS can protect farmers in the long term against climate change. However, our review demonstrates that the current evidence from models targeting long-term periods lack corroboration, whilst insight from artificial experiments can omit meaningful broader interactions with the environment and people (Coe et al., 2014; Hounkpèvi et al., 2018) and may not be able to replicate all essential climate variables (Schwendenmann et al., 2010). To advance knowledge on climate change impacts on TAFS as a social ecological system, an ideal field study should consider collectively and in isolation the impacts on the biophysical system (trees, soils, water); social system (e.g., farmers' decisions); tree-soil-crop interactions; ecosystem services (crop provision) and farmers' wellbeing.
Our review also revealed that most studies focused on examining the impacts on one crop, which was often cacao. This is problematic for two reasons. Firstly, cacao is climate-sensitive (Wood and Lass, 1987), and our conclusions drawn from these publications may be skewed. Secondly, single crop-based systems poorly represent TAFS which are usually highly diverse (Pathak and Dagar, 2000; Jemal et al., 2018). To better represent TAFS in climate change studies, future research could study TAFS which support a diversity of crop types. For example, homegardens are known for their high crop, tree and tree fruit diversity (Kumar and Nair, 2004; Dagar and Tewari, 2017), offering an ideal system. Homegardens would therefore also provide more diverse root structures suitable for natural experimental studies on tree-soil-crop interactions. Such research could help address our identified knowledge gap regarding the dearth of studies investigating climate change impacts on ecological interactions in TAFS comprising deeper and more complementary tree roots.
To improve on the robustness of evidence in this research field, we recommend that future research explore the method of climate analog analysis. This involves using various locations where current climates are akin to predicted climates for a site of interest (Veloz et al., 2012). The approach is advocated as an alternative to modeling for projecting future climate impacts on agroforestry systems (Luedeling and Neufeldt, 2012; Luedeling et al., 2014). Mountains in the tropics can be valuable sites for climate analog analysis due to their steep change in climate with altitude. Therefore, experimental studies can use mountain elevation gradients as 'natural laboratories' instead of depending on creating artificial environments (Tito et al., 2020). Downslope locations are typically warmer and dryer, which can resemble drought conditions or future climate scenarios, and can remove the need to create these conditions artifically. The impacts of the change in climate conditions on ecological interactions in agroecosystems in these downslope locations can be more clear than when studied in artificial environments (Tito et al., 2020). Such an approach has been effectively applied to predict the impacts of future climates on crop yields (Tito et al., 2018), soil nutrient cycles (Becker et al., 2015), plant responses (Cardinaux et al., 2018) and soil decomposition processes (Nottingham et al., 2015) in tropical montane forests. Using downslope locations as a climate change treatment can allow studies to assess the impacts of drought, water-limited conditions, and future climate scenarios on TAFS in natural environments more robustly (LoE1a), including on tree-soil-crop interactions, without requiring artificial experiments (LoE1b) or observational study designs (LoE2). Exploiting the change in climate across mountain gradients can allow modeling-based studies focusing on warmer and dryer climates to validate their predictions and improve from LoE2b to LoE2a. Important non-climate and socio-economic factors, however, need to be sufficiently similar across study sites (Feller et al., 2015).
Finally, we recommend following a conceptual model to support the implementation of studies employing a climate analog analysis methodology to predict the response of montane TAFS to climate change (Figure 6). This conceptual model has been devised using the findings from our review which are based on reliable evidence and where the direction of impact was most consistent. Consistent with our recommendation for interdisciplinary research, our model considers the interactions of both TAFS biophysical and livelihood components in predicting the impacts of climate change. The model considers that provisioning ecosystem services from TAFS and the wellbeing of smallholders are mainly influenced by the tree-soil-crop interactions, which in turn are mediated by (i) the state of the trees, soils, and water in the biophysical system, and (ii) the decisions made in the livelihoods and farm management system (e.g., which trees and crops are planted). We expect the provisioning of ecosystem services and the wellbeing of farmers, including their cultural values, health, and income, to decline under climate change due to impaired tree size and growth (Feng and Li, 2007; Vincent et al., 2009; Tamayo-Chim et al., 2012), declining soil productivity and nutrient cycling (van Straaten et al., 2010; Russell and Kumar, 2019; Lakshmi et al., 2021), reduced water availability (Blaser et al., 2018), lower crop pollination (Arnold et al., 2018) and farmer's decision to change their farm management, which can influence biodiversity (Landreth and Saito, 2014). These biophysical and social impacts would influence tree-soil-crop interactions that underpin smallholder farmers' crop production and wellbeing. Through this dynamic process, and under warmer and dryer climate conditions downslope of mountains, limited soil water could be depleted more rapidly due to resource competition between trees and crops involving less complementary root systems (Lott et al., 2009; Abdulai et al., 2018); and biodiversity could potentially be reduced due to increased plant mortality (Abdulai et al., 2018) and crop failure (Lott et al., 2009). We expect the provision of crops to decrease under increasing climate change impacts (Blaser et al., 2018), and this would then feedback into the biophysical and farm management systems through potential changes in the management of biodiversity and natural resources, or through farmers' responses, such as changing crop types (Landreth and Saito, 2014). Although not included in our review papers, we acknowledge that other socio-economic factors, such as changes in crop market prices, can influence farmers' wellbeing outcomes and crop management choices. Furthermore, as our interdisciplinary papers did not sufficiently study the relationship between farmers' crops and their wellbeing outcomes, the impact on farmers' wellbeing with change in crop provisioning ecosystem service in our conceptual model is informed by the wellbeing-TAFS literature reviewed in our introduction.
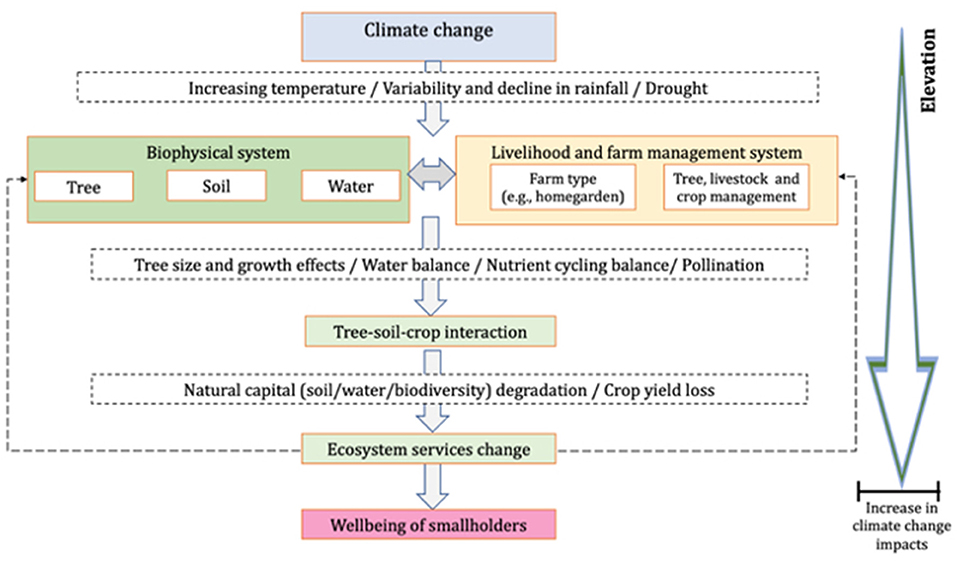
Figure 6. Conceptual model depicting the components, relationships, and feedbacks under climate change in TAFS and how these change with elevation. This model depicts the main processes (solid boxes) and underlying mechanisms (dashed boxes) affecting the wellbeing of smallholder farmers in TAFS. Solid arrows denote the main causal direction, whilst dashed arrows denote feedbacks.
Conclusion
Our review has provided a synthesis of the academic literature on the impacts of climate change on TAFS and assessed the quality of their evidence. The key findings are that climate change impacts on TAFS are under-studied, particularly using a livelihood and interdisciplinary approach, and that our current knowledge is based on robust but also “least robust” evidence. Overall, climate change was found to negatively impact TAFS, which implies that TAFS could still succumb to the adverse effects of climate change if used as a long-term adaptation measure. To ascertain this with more reliable evidence, we recommend that future studies follow an interdisciplinary approach and assess the potential impacts of future climate conditions on TAFS performance using climate analog analysis along mountain elevation gradients.
Data availability statement
The original contributions presented in the study are included in the article/Supplementary material, further inquiries can be directed to the corresponding authors.
Author contributions
MW and KP conceived the systematic review. MW and EM conceived the evidence assessment protocol. MW performed the literature search, data extraction, and wrote the manuscript. KP edited the manuscript. All authors discussed results, contributed to reviewing the manuscript, and approved the submitted version.
Funding
The research for this article was funded by the Economic and Social Research Council South Coast Doctoral Training Partnership (Grant Number ES/P000673/1).
Acknowledgments
We thank Marije Schaafsma and Emma Tompkins for their early guidance and discussions on this research topic.
Conflict of interest
The authors declare that the research was conducted in the absence of any commercial or financial relationships that could be construed as a potential conflict of interest.
Publisher's note
All claims expressed in this article are solely those of the authors and do not necessarily represent those of their affiliated organizations, or those of the publisher, the editors and the reviewers. Any product that may be evaluated in this article, or claim that may be made by its manufacturer, is not guaranteed or endorsed by the publisher.
Supplementary material
The Supplementary Material for this article can be found online at: https://www.frontiersin.org/articles/10.3389/ffgc.2022.880621/full#supplementary-material
References
Abdulai, I., Vaast, P., Hoffmann, M. P., Asare, R., Jassogne, L., van Asten, P., et al. (2018). Cocoa agroforestry is less resilient to sub-optimal and extreme climate than cocoa in full sun. Global Change Biol. 24, 273–286. doi: 10.1111/gcb.13885
Abebe, T., Wiersum, K. F., Bongers, F., and Sterck, F. (2006). “Diversity and dynamics in homegardens of southern Ethiopia,” in Tropical Homegardens. Dordrecht, Springer. 123–142. doi: 10.1007/978-1-4020-4948-4_8
Agwu, O. P., Bakayoko, A., Jimoh, S. O., and Stefan, P. (2018). Farmers' perceptions on cultivation and the impacts of climate change on goods and services provided by Garcinia kola in Nigeria. Ecol. Process. 7, 1–10. doi: 10.1186/s13717-018-0147-3
Agwu, O. P., Bakayokoa, A., Jimoh, S. O., Dimobe, K., and Porembski, S. (2020). Impact of climate on ecology and suitable habitat of Garcinia kola heckel in Nigeria. Trees, Forests People 1, 100006. doi: 10.1016/j.tfp.2020.100006
Ajayi, O. C., Place, F., Akinnifesi, F. K., and Sileshi, G. W. (2011). Agricultural success from Africa: the case of fertilizer tree systems in southern Africa (Malawi, Tanzania, Mozambique, Zambia and Zimbabwe). Int. J. Agric. Sustain. 9, 129–136. doi: 10.3763/ijas.2010.0554
Altieri, M. A., and Nicholls, C. I. (2017). The adaptation and mitigation potential of traditional agriculture in a changing climate. Clim. Change 140, 33–45. doi: 10.1007/s10584-013-0909-y
Amano, T., Gonzá Lez-Varo, J. P., and Sutherland, W. J. (2016). Languages Are Still a Major Barrier to Global Science. PLoS Biol. 14, e2000933. doi: 10.1371/journal.pbio.2000933
Andriamananjara, A., Chevallier, T., Masse, D., Razakamanarivo, H., and Razafimbelo, T. (2019). Land management modifies the temperature sensitivity of soil organic carbon, nitrogen and phosphorus dynamics in a Ferralsol. Appl. Soil Ecol. 138, 112–122. doi: 10.1016/j.apsoil.2019.02.023
Arnold, S. E. J., Bridgemohan, P., Perry, G. B., Spinelli, G. R., Pierre, B., Murray, F., et al. (2018). The significance of climate in the pollinator dynamics of a tropical agroforestry system. Agric. Ecosyst. Environ. 254, 1–9. doi: 10.1016/j.agee.2017.11.013
Atangana, A., Khasa, D., Chang, S., and Degrande, A. (2014). Tropical Agroforestry. Dordrecht, Netherland: Springer Netherlands. 1–380. doi: 10.1007/978-94-007-7723-1
Balasubramanian, D., Arunachalam, K., and Arunachalam, A. (2015). Soil microbial and biochemical properties as affected by floods in different landuse systems of Burachapori Wildlife Sanctuary, northeast India. Trop. Ecol. 56, 289–302.
Bardgett, R. D., Manning, P., Morriën, E., and De Vries, F. T. (2013). Hierarchical responses of plant-soil interactions to climate change: Consequences for the global carbon cycle. J. Ecol. 101, 334–343. doi: 10.1111/1365-2745.12043
Bayala, J., Oborn, I., and Dupraz, C. (2019). “Belowground resource sharing in mixed tree-crop systems: methods to better understand belowground interactions,” in van Noordwijk, M., ed. Sustainable development through trees on farms: agroforestry in its fifth decade. Bogor: World Agroforestry (ICRAF). p. 93–109.
Becker, J., Pabst, H., Mnyonga, J., and Kuzyakov, Y. (2015). Annual litterfall dynamics and nutrient deposition depending on elevation and land use at Mt. Kilimanjaro. Biogeosciences 12, 5635–5646. doi: 10.5194/bg-12-5635-2015
Belfrid, D. M., Arcadius, D., N'tcha Yekanbessoun, M., Mariano, S., Fidèle, S., Jean, A., et al. (2022). Inventory of agroecosystem services and perceptions potential implications due to climate change: A case study from Benin in West Africa. Environ. Impact Assess. Rev. 95, 106792. doi: 10.1016/j.eiar.2022.106792
Belsky, A. J., Mwonga, S. M., and Duxbury, J. M. (1993). Effects of widely spaced trees and livestock grazing on understory environments in tropical savannas. Agroforestry Syst. 24, 1–20. doi: 10.1007/BF00705265
Blanckaert, I., Swennen, R. L., Paredes Flores, M., Lopez, R., and Saade, R. L. (2004). Floristic composition, plant uses and management practices in homegardens of San Rafael Coxcatlan, Valley of Tehuacan-Cuicatlan, Mexico. J. Arid Environ. 57, 39–62. doi: 10.1016/S0140-1963(03)00100-9
Blaser, W. J., Oppong, J., Hart, S. P., Landolt, J., Yeboah, E., and Six, J. (2018). Climate-smart sustainable agriculture in low-to-intermediate shade agroforests. Nat. Sustain. 1, 234–239. doi: 10.1038/s41893-018-0062-8
Boissière, M., Locatelli, B., Sheil, D., Padmanaba, M., and Sadjudin, E. (2013). Local perceptions of climate variability and change in tropical forests of Papua, Indonesia. Ecol. Soc. 18, 13. doi: 10.5751/ES-05822-180413
Bremer, L. L., Mandle, L., Trauernicht, C., Pascua, P., McMillen, H. L., Burnett, K., et al. (2018). Bringing multiple values to the table: Assessing future land-use and climate change in North Kona, Hawai‘i. Ecol. Soc. 23, 33. doi: 10.5751/ES-09936-230133
Brown, S. E., Miller, D. C., Ordonez, P. J., and Baylis, K. (2018). Evidence for the impacts of agroforestry on agricultural productivity, ecosystem services, and human well-being in high-income countries: a systematic map protocol. Environ. Evid. 7, 24. doi: 10.1186/s13750-018-0136-0
Campbell, B. M., Vermeulen, S. J., Aggarwal, P. K., Corner-Dolloff, C., Girvetz, E., Loboguerrero, A. M., et al. (2016). Reducing risks to food security from climate change. Global Food Secur. 11, 34–43. doi: 10.1016/j.gfs.2016.06.002
Cardinaux, A., Hart, S. P., and Alexander, J. M. (2018). Do soil biota influence the outcome of novel interactions between plant competitors? J. Ecol. 106, 1853–1863. doi: 10.1111/1365-2745.13029
Charles, R., Munishi, P., and Nzunda, E. (2014). Agroforestry as a resilient strategy in mitigating climate change in Mwanga District, Kilimanjaro, Tanzania. Global J. Biol., Agric. Health Sci. 3, 11–17.
Choudhury, B. U., Nengzouzam, G., and Islam, A. (2022). Runoff and soil erosion in the integrated farming systems based on micro-watersheds under projected climate change scenarios and adaptation strategies in the eastern Himalayan mountain ecosystem (India). J. Environ. Manage. 309, 114667. doi: 10.1016/j.jenvman.2022.114667
Coe, R., Sinclair, F., and Barrios, E. (2014). Scaling up agroforestry requires research “in” rather than “for” development. Curr. Opin. Environ. Sustain. 6, 73–77. doi: 10.1016/j.cosust.2013.10.013
Córdova, R., Hogarth, N. J., and Kanninen, M. (2019). Mountain farming systems' exposure and sensitivity to climate change and variability: Agroforestry and conventional agriculture systems compared in Ecuador's Indigenous Territory of Kayambi people. Sustainability 11, 1–30. doi: 10.3390/su11092623
Coulibaly, J. Y., Chiputwa, B., Nakelse, T., and Kundhlande, G. (2017). Adoption of agroforestry and the impact on household food security among farmers in Malawi. Agricultural Systems. 155, 52–69. doi: 10.1016/j.agsy.2017.03.017
Coulibaly, Y. N., Mulia, R., Sanou, J., Zombré, G., Bayala, J., Kalinganire, A., et al. (2014). Crop production under different rainfall and management conditions in agroforestry parkland systems in Burkina Faso: observations and simulation with WaNuLCAS model. Agroforest Syst. 88, 13–28. doi: 10.1007/s10457-013-9651-8
Dagar, J. C., and Tewari, V. P. (2017). “Evolution of agroforestry as a modern science,” in Agroforestry. Singapore: Springer Singapore. p. 13–90. doi: 10.1007/978-981-10-7650-3_2
de la Cerda, H. E. C., and Mukul, R. R. G. (2008). Homegarden production and productivity in a Mayan community of Yucatan. Human Ecol. 36, 423–433. doi: 10.1007/s10745-008-9166-5
Dietz, J., Hölscher, D., Leuschner, C., and Hendrayanto. (2006). Rainfall partitioning in relation to forest structure in differently managed montane forest stands in Central Sulawesi, Indonesia. Forest Ecol. Manag. 237, 170–178. doi: 10.1016/j.foreco.2006.09.044
Elbakidze, M., Surová, D., Muñoz-Rojas, J., Persson, J. O., Dawson, L., Plieninger, T., et al. (2021). Perceived benefits from agroforestry landscapes across North-Eastern Europe: What matters and for whom? Landscape Urban Plan. 209, 104044. doi: 10.1016/j.landurbplan.2021.104044
Esmail, S., and Oelbermann, M. (2011). The impact of climate change on the growth of tropical agroforestry tree seedlings. Agroforestry Syst. 83, 235–244. doi: 10.1007/s10457-011-9424-1
Feller, U., Cristina Lomba, Â., Luedeling, E. M., Bos, S. P., Pagella, T., et al. (2015). Climate analogs for agricultural impact projection and adaptation-a reliability test. Front. Environ. Sci. 3, 65. doi: 10.3389/fenvs.2015.00065
Feng, Y. L., and Li, X. (2007). The combined effects of soil moisture and irradiance on growth, biomass allocation, morphology and photosynthesis in Amomum villosum. Agroforestry Syst. 71, 89–98. doi: 10.1007/s10457-007-9076-3
Garedew, W., Hailu, B. T., Lemessa, F., Pellikka, P., and Pinard, F. (2017). Coffee Shade Tree Management: An Adaptation Option for Climate Change Impact for Small Scale Coffee Growers in South-West Ethiopia. Cham: Springer. p. 647–659. Available online at: http://link.springer.com/10.1007/978-3-319-49520-0_40 (accessed December 5, 2018). doi: 10.1007/978-3-319-49520-0_40
Gasparrini, A., Guo, Y., Sera, F., Vicedo-Cabrera, A. M., Huber, V., Tong, S., et al. (2017). Projections of temperature-related excess mortality under climate change scenarios. Lancet Planetary Health 1, e360–e367. doi: 10.1016/S2542-5196(17)30156-0
Gateau-Rey, L. J., Tanner, E. V., Rapidel, B., Marelli, J.-P., and Royaert, S. (2018). Climate change could threaten cocoa production: Effects of 2015-16 El Niño-related drought on cocoa agroforests in Bahia, Brazil. PLOS ONE 13, e0200454. doi: 10.1371/journal.pone.0200454
Haddaway, N. R., Macura, B., Whaley, P., and Pullin, A. S. (2018). ROSES RepOrting standards for Systematic Evidence Syntheses: pro forma, flow-diagram and descriptive summary of the plan and conduct of environmental systematic reviews and systematic maps. Environ. Evid. 7, 7. doi: 10.1186/s13750-018-0121-7
Hashini Galhena, D., Freed, R., and Maredia, K. M. (2013). Home gardens: a promising approach to enhance household food security and wellbeing. Agric. Food Secur. 2, 1–13. doi: 10.1186/2048-7010-2-8
Hounkpèvi, A., Kouassi, E. K., and Kakaï, R. G. (2018). Effects of climatic variability and local environment patterns on the ecology and population structure of the multipurpose plant species, Vitex doniana Sweet (Lamiaceae) in Benin. Trop. Ecol. 59, 129–143.
Iannotti, L., Cunningham, K., and Marie, R. (2009). Improving Diet Quality and Micronutrient Nutrition Homestead Food Production in Bangladesh. Washing DC. Available online at: www.ifpri.org/millionsfed (accessed August 13, 2020).
Ibarra, J. T., Caviedes, J., Altamirano, T. A., Urra, R., Barreau, A., and Santana, F. (2021). Social-ecological filters drive the functional diversity of beetles in homegardens of campesinos and migrants in the southern Andes. Sci. Rep. 11, 1–14. doi: 10.1038/s41598-021-91185-4
Ilstedt, U., Bargués Tobella, A., Bazi,é, H. R., Bayala, J., Verbeeten, E., Nyberg, G., et al. (2016). Intermediate tree cover can maximize groundwater recharge in the seasonally dry tropics. Nat. Sci. Rep. 6, 21930. doi: 10.1038/srep21930
Jaramillo, J., Setamou, M., Muchugu, E., Chabi-Olaye, A., and Jaramillo, A. (2013). Climate Change or Urbanization? Impacts on a Traditional Coffee Production System in East Africa over the Last 80 Years. PLoS ONE, 8, 51815. doi: 10.1371/journal.pone.0051815
Jemal, O., Callo-Concha, D., and van Noordwijk, M. (2018). Local agroforestry practices for food and nutrition security of smallholder farm households in southwestern ethiopia. Sustainability 10, 2722. doi: 10.3390/su10082722
Jose, S. (2009). Agroforestry for ecosystem services and environmental benefits: an overview. Agroforestry Syst. 76, 1–10. doi: 10.1007/s10457-009-9229-7
Koch, A., and Kaplan, J. O. (2022). Tropical forest restoration under future climate change. Nat. Clim. Change 12, 279–283. doi: 10.1038/s41558-022-01289-6
Köhler, M., Schwendenmann, L., and Hölscher, D. (2010). Throughfall reduction in a cacao agroforest: Tree water use and soil water budgeting. Agric. Forest Meteorol. 150, 1079–1089. doi: 10.1016/j.agrformet.2010.04.005
Kumar, B. M., and Nair, P. K. R. (2004). The enigma of tropical homegardens. Agroforest. Syst. 61, 135–152. doi: 10.1007/978-94-017-2424-1_10
Kumar, N., Qumruzzaman, H. M., David, C., Malcolm, F., Merchant, P. A., Halder, N. K., et al. (2021). Intra-specific patterns of d 13 C, growth and wood density variation at sites of contrasting precipitation with implications for modelling carbon sequestration of tropical tree species Trees at the low precipitation site showed lower. Agroforest. Syst. 95, 1429–1443. doi: 10.1007/s10457-021-00646-2
Kuyah, S., Whitney, C. W., Jonsson, M., Sileshi, G., Öborn, I., Muthuri, C. W., et al. (2019). Agroforestry delivers a win-win solution for ecosystem services in sub-Saharan Africa. A meta-analysis. Agronomy Sustain. Develop. 39, 47. doi: 10.1007/s13593-019-0589-8
Lakshmi, G., Beggi, F., Menta, C., Kumar, N. K., and Jayesh, P. (2021). Dynamics of soil microarthropod populations affected by a combination of extreme climatic events in tropical home gardens of Kerala, India. Pedobiologia 85, 150719. doi: 10.1016/j.pedobi.2021.150719
Landreth, N., and Saito, O. (2014). An Ecosystem Services Approach to Sustainable Livelihoods in the Homegardens of Kandy, Sri Lanka. Australian Geogr. 45, 355–373. doi: 10.1080/00049182.2014.930003
Lasco, R. D., Delfino, R. J. P., Catacutan, D. C., Simelton, E. S., and Wilson, D. M. (2014). Climate risk adaptation by smallholder farmers: the roles of trees and agroforestry. Curr. Opin. Environ. Sustain. 6, 83–88. doi: 10.1016/j.cosust.2013.11.013
Lasco, R. D., Espaldon, M. L. O., and Habito, C. M. D. (2016). Smallholder farmers' perceptions of climate change and the roles of trees and agroforestry in climate risk adaptation: evidence from Bohol, Philippines. Agroforest. Syst. 90, 521–540. doi: 10.1007/s10457-015-9874-y
Lin, B., Perfecto, I., and Vandermeer, J. (2008). Synergies between agricultural intensification and climate change could create surprising vulnerabilities for crops. Bioscience 58, 847–854. doi: 10.1641/B580911
Lin, Y., Liu, A., Ma, E., and Zhang, F. (2013). Impacts of future climate changes on shifting patterns of the agro-ecological zones in China. Adv. Meteorol. 2013, 1–9. doi: 10.1155/2013/163248
Linger, E. (2014). Agro-ecosystem and socio-economic role of homegarden agroforestry in Jabithenan District, North-Western Ethiopia: implication for climate change adaptation. Springer Plus 3, 154. doi: 10.1186/2193-1801-3-154
Liu, J., Dietz, T., Carpenter, S. R., Alberti, M., Folke, C., Moran, E., et al. (2007). Complexity of coupled human and natural systems. Science 317, 1513–1516. doi: 10.1126/science.1144004
Liu, Q., Peng, P.-H., Wang, Y.-K., Xu, P., and Guo, Y.-M. (2019). Microclimate regulation efficiency of the rural homegarden agroforestry system in the Western Sichuan Plain, China. J. Mountain Sci. 16, 516–528. doi: 10.1007/s11629-018-5112-1
Lott, J. E., Ong, C. K., and Black, C. R. (2009). Understorey microclimate and crop performance in a Grevillea robusta-based agroforestry system in semi-arid Kenya. Agric. Forest Meteorol. 149, 1140–1151. doi: 10.1016/j.agrformet.2009.02.002
Luedeling, E., Kindt, R., Huth, N. I., and Koenig, K. (2014). Agroforestry systems in a changing climate-challenges in projecting future performance. Curr. Opin. Environ. Sustain. 6, 1–7. doi: 10.1016/j.cosust.2013.07.013
Luedeling, E., and Neufeldt, H. (2012). Carbon sequestration potential of parkland agroforestry in the Sahel. Clim. Change 115, 443–461. doi: 10.1007/s10584-012-0438-0
Maroyi, A. (2009). Traditional homegardens and rural livelihoods in Nhema, Zimbabwe: a sustainable agroforestry system. Int. J. Sustain. Develop. World Ecol. 16, 1–8. doi: 10.1080/13504500902745895
Marsh, R. (1998). Building on traditional gardening to improve household food security. Food Nutr. Agric. 22, 4–14.
Mbow, C., Van Noordwijk, M., Prabhu, R., and Simons, T. (2014). Knowledge gaps and research needs concerning agroforestry's contribution to Sustainable Development Goals in Africa. Curr. Opin. Environ. Sustain. 6, 162–170. doi: 10.1016/j.cosust.2013.11.030
Midgley, G. F., and Thuiller, W. (2011). Potential responses of terrestrial biodiversity in Southern Africa to anthropogenic climate change. Reg. Environ. Change 11, 127–135. doi: 10.1007/s10113-010-0191-8
Mitchell, R., and Hanstad, T. (2004). “Small homegarden plots and sustainable livelihoods for the poor,” in FAO, LSP WP 11, Access to Natural Resources Sub-Programme. Available online at: http://landwise-production.s3.amazonaws.com/2022/03/Mitchell_Small_homegarden_plots_and_sustainable_livelihoods_for_the_poor_2004-1.pdf
Mohri, H., Lahoti, S., Saito, O., Mahalingam, A., Gunatilleke, N., Irham, H.oang, V. T., et al. (2013). Assessment of ecosystem services in homegarden systems in Indonesia, Sri Lanka, and Vietnam. Ecosyst. Serv. 5, 124–136. doi: 10.1016/j.ecoser.2013.07.006
Muñoz-Villers, L.yssette, E., Geris, J., Alvarado-Barrientos, María, S., Holwerda, F., and Dawson, T. (2020). Coffee and shade trees show complementary use of soil water in a traditional agroforestry ecosystem. Hydrol. Earth Syst. Sci. 24, 1649–1668. doi: 10.5194/hess-24-1649-2020
Mupepele, A. C., Walsh, J. C., Sutherland, W. J., and Dormann, C. F. (2016). An evidence assessment tool for ecosystem services and conservation studies. Ecol. Applic. 26, 1295–1301. doi: 10.1890/15-0595
Nair, P. K. R. (1993). An Introduction to Agroforestry. Dordrecht: Kluwer Academic Publishers. Available online at: https://www.worldagroforestry.org/Units/Library/Books/PDFs/32_An_introduction_to_agroforestry.pdf?n=161 (accessed November 7, 2018).
Neufeldt, H., Dawson, I. K., Luedeling, E., Ajayi, O. C., Beedy, T., Gebrekirstos, A., et al. (2012). “Climate change vulnerability of agroforestry,” Nairobi: ICRAF. No. 143. doi: 10.5716/WP12013.PDF
Neupane, R. P., Sharma, K. R., and Thapa, G. B. (2002). Adoption of agroforestry in the hills of Nepal: a logistic regression analysis. Agric. Syst. 72, 177–196. doi: 10.1016/S0308-521X(01)00066-X
Nguyen, Q., Hoang, M. H., Öborn, I., and van Noordwijk, M. (2013). Multipurpose agroforestry as a climate change resiliency option for farmers: An example of local adaptation in Vietnam. Clim. Change. 117, 241–257. doi: 10.1007/s10584-012-0550-1
Nottingham, A. T., Whitaker, J., Turner, B. L., Salinas, N., Zimmermann, M., Malhi, Y., et al. (2015). Special section on tropical forests climate warming and soil carbon in tropical forests: insights from an elevation gradient in the peruvian andes. Special Sect. Trop. Forest. 65, 906–921. doi: 10.1093/biosci/biv109
Odeny, D., Karan-Ja, F., Mwachala, G., Pellikka, P., and Marchant, R. (2019). Impact of climate change on species distribution and carbon storage of agroforestry trees on isolated east african mountains impact of climate change on species distribution and carbon storage of agroforestry trees on isolated east african mountains. Am. J. Clim. Change 8, 364–386. doi: 10.4236/ajcc.2019.83020
Okigbo, B. (1990). “Home gardens in tropical africa,” in Landauer, K., and Brazil, M., , eds. Tropical Home Gardens. Tokyo, Japan: United Nations University Press. p. 21–40.
Onyekuru, N. J. A., and Marchant, R. (2016). Assessing the economic impact of climate change on forest resource use in Nigeria: A Ricardian approach. Agric. Forest Meteorol. 220, 10–20. doi: 10.1016/j.agrformet.2016.01.001
Otte, I., Detsch, F., Gütlein, A., Scholl, M., Kiese, R., Appelhans, T., et al. (2017). Seasonality of stable isotope composition of atmospheric water input at the southern slopes of Mt. Kilimanjaro, Tanzania. Hydrol. Process. 31, 3932–3947. doi: 10.1002/hyp.11311
Palm, C. A., Smukler, S. M., Sullivan, C. C., Mutuo, P. K., Nyadzi, G. I., and Walsh, M. G. (2010). Identifying potential synergies and trade-offs for meeting food security and climate change objectives in sub-Saharan Africa. Proc. National Academy Sci. 107, 19661–19666. doi: 10.1073/pnas.0912248107
Pandey, R., Meena, D., Aretano, R., Satpathy, S., Semeraro, T., Kumar Gupta, A., et al. (2015). Socio-ecological vulnerability of smallholders due to climate change in mountains: agroforestry as an adaptation measure. Change Adapt. Socio Ecol. Syst. 2, 26–41. doi: 10.1515/cass-2015-0003
Pathak, P. S., and Dagar, J. C. (2000). “Traditional agroforestry systems: an overview,” in Landmarks of botmany in India. Suria International Pub, p. 26–49.
Paul, C., Weber, M., and Knoke, T. (2017). Agroforestry versus farm mosaic systems -Comparing land-use efficiency, economic returns and risks under climate change effects. Sci. Total Environ. 587, 22–35. doi: 10.1016/j.scitotenv.2017.02.037
Pereyra, G., Hartmann, H., Michalzik, B., Ziegler, W., and Trumbore, S. (2015). Influence of rhizobia inoculation on biomass gain and tissue nitrogen content of Leucaena leucocephala seedlings under drought. Forests 6, 3686–3703. doi: 10.3390/f6103686
Peters, V. E., and Carroll, C. R. (2012). Temporal variation in coffee flowering may influence the effects of bee species richness and abundance on coffee production. Agroforest. Syst. 85, 95–103. doi: 10.1007/s10457-011-9476-2
Phiri, E., Verplancke, H., Kwesiga, F., and Mafongoya, P. (2003). Water balance and maize yield following improved sesbania fallow in eastern Zambia. Agroforest. Syst. 59, 197–205. doi: 10.1023/B:AGFO.0000005220.67024.2c
Pramova, E., Locatelli, B., Djoudi, H., and Somorin, O. A. (2012). Forests and trees for social adaptation to climate variability and change. Climate Change 3, 581–596. doi: 10.1002/wcc.195
Puri, S., and Swamy, S. L. (2001). Growth and biomass production in Azadirachta indica seedlings in response to nutrients (N and P) and moisture stress. Agroforest. Syst. 51, 57–68. doi: 10.1023/A:1006402212225
Quandt, A., Neufeldt, H., and Mccabe, J. T. (2017). The role of agroforestry in building livelihood resilience to floods and drought in Kenya. Ecol. Soc. 22, 10. doi: 10.5751/ES-09461-220310
Quandt, A., Neufeldt, H., and Mccabe, J. T. (2018). Building livelihood resilience: what role does agroforestry play? Climate Develop. 11:485–500. doi: 10.1080/17565529.2018.1447903
Rahn, E., Vaaset, P., Laderach, P., van Asten, P., Jassogne, L., and Ghazoul, J. (2018). Exploring adaptation strategies of coffee production to climate change using a process-based model. Ecol. Modell. 371, 76–89. doi: 10.1016/j.ecolmodel.2018.01.009
Ram, N., Chaturvedi, O. P., and Handa, A. K. (2016). Recent development in agroforestry research and its role in climate change adaptation and mitigation. Indian J. Agroforestry 18, 1–9.
Ranjitkar, S., Sujakhu, N. M., Lu, Y., Wang, Q., Wang, M., He, J., et al. (2016). Climate modelling for agroforestry species selection in Yunnan Province, China. Environ. Model. Software 75, 263–272. doi: 10.1016/j.envsoft.2015.10.027
Rao, M. R., Nair, P. K. R., and Ong, C. K. (1998). Biophysical interactions in tropical agroforestry systems. Agroforest. Syst. 38, 3–50. doi: 10.1023/A:1005971525590
Rosenstock, T., Wilkes, A., Jallo, C., Namoi, N., Bulusu, M., Suber, M., et al. (2018). Making Trees Count. The Netherlands: Wageningnen.
Roy, J., Tschakert, P., Waisman, H., Halim, S. A., Antwi-Agyei, P., Dasgupta, P., et al. (2018). IPCC Global Warming of 1.5°C Report - Chapter 5: Sustainable Development, Poverty Eradication and Reducing Inequalities. Nenenteiti Teariki-Ruatu. Penny. Available from: https://www.ipcc.ch/site/assets/uploads/sites/2/2018/11/SR15_Chapter5_Low_Res-1.pdf (accessed Decembe 15, 2018).
Russell, A. E., and Kumar, B. M. (2019). Modeling experiments for evaluating the effects of trees, increasing temperature, and soil texture on carbon stocks in agroforestry systems in Kerala, India. Forests 10, 1–16. doi: 10.3390/f10090803
Rychetnik, L., Frommer, M., Hawe, P., and Shiell, A. (2002). Criteria for evaluating evidence on public health interventions. J. Epidemiol Commun. Health 56, 119–127. doi: 10.1136/jech.56.2.119
Schroth, G. B., da Fonseca, G. A., Harvey, C. A., Gascon, C., Vasconcelos, H. L., et al. (2004). Agroforestry and biodiversity conservation in tropical landscapes. Washington DC: Island Press. Available online at: http://library.uniteddiversity.coop/Permaculture/Agroforestry/Agroforestry_and_Biodiversity_Conservation_in_Tropical_Landscapes.pdf (accessed December 1, 2018).
Schwendenmann, L., Veldkamp, E., Moser, G., Hölscher, D., Köhler, M., Clough, Y., et al. (2010). Effects of an experimental drought on the functioning of a cacao agroforestry system, Sulawesi, Indonesia. Global Change Biol. 16, 1515–1530. doi: 10.1111/j.1365-2486.2009.02034.x
Serdeczny, O., Adams, S., Baarsch, F., Coumou, D, Robinson, A, Alexander, H., et al. (2017). Climate change impacts in Sub-Saharan Africa: from physical changes to their social repercussions. Regional Environ. Change 17, 1585–1600. doi: 10.1007/s10113-015-0910-2
Sida, T. S., Baudron, F., Kim, H., and Giller, K. E. (2018). Climate-smart agroforestry: Faidherbia albida trees buffer wheat against climatic extremes in the Central Rift Valley of Ethiopia. Agric. Forest Meteorol. 248, 339–347. doi: 10.1016/j.agrformet.2017.10.013
Siles, P., Jean-Michel, H., and Vaast, P. (2010). Effects of Inga densiflora on the microclimate of coffee (Coffea arabica L.) and overall biomass under optimal growing conditions in Costa Rica. Agroforest. Syst. 78, 269–286. doi: 10.1007/s10457-009-9241-y
Sileshi, G., Akinnifesi, F. K., Ajayi, O. C., Chakeredza, S., Kaonga, M., and Matakala, P. W. (2007). Contributions of agroforestry to ecosystem services in the miombo eco-region of eastern and southern Africa. African J. Environ. Sci. Technol. 1, 68–80.
Sileshi, G., and Mafongoya, P. L. (2006). Long-term effects of improved legume fallows on soil invertebrate and macrofauna and maize yield in eastern Zambia. Agric. Ecosyst. Environ. 115, 69–78. doi: 10.1016/j.agee.2005.12.010
Simelton, E., Viet Dam, B., and Catacutan, D. (2015). Trees and agroforestry for coping with extreme weather events: experiences from northern and central Viet Nam. Agroforest. Syst. 89, 1065–1082. doi: 10.1007/s10457-015-9835-5
Siyum, Z. G. (2020). Tropical dry forest dynamics in the context of climate change: syntheses of drivers, gaps, and management perspectives. Ecol. Process. 9, 25. doi: 10.1186/s13717-020-00229-6
Somarriba, E., Valdivieso, R., Vasquez, W., and Galloway, G. (2001). Survival, growth, timber productivity and site index of Cordia alliodora in forestry and agroforestry systems. Agroforest Syst. 51, 111–118. doi: 10.1023/A:1010699019745
Talukder, A., Kiess, L., Huq, N., De Pee, S., Darnton-Hill, I., and Bloem, M. W. (2000). Increasing the production and consumption of vitamin A-rich fruits and vegetables: Lessons learned in taking the Bangladesh homestead gardening programme to a national scale. Food Nutrition Bull. 21, 165–172. doi: 10.1177/156482650002100210
Tamayo-Chim, M., Reyes-García, C., and Orellana, R. (2012). A combination of forage species with different responses to drought can increase year-round productivity in seasonally dry silvopastoral systems. Agroforest. Syst. 84, 287–297. doi: 10.1007/s10457-011-9470-8
Thangata, P. H., and Hildebrand, P. E. (2012). Carbon stock and sequestration potential of agroforestry systems in smallholder agroecosystems of sub-Saharan Africa: Mechanisms for “reducing emissions from deforestation and forest degradation” (REDD+). Agric. Ecosyst. Environ. 158, 172–183. doi: 10.1016/j.agee.2012.06.007
Thorlakson, T., and Neufeldt, H. (2012). Reducing subsistence farmers' vulnerability to climate change: evaluating the potential contributions of agroforestry in western Kenya. Agric. Food Secur. 1, 1–13. doi: 10.1186/2048-7010-1-15
Tito, R., Vasconcelos, H. L., and Feeley, K. J. (2018). Global climate change increases risk of crop yield losses and food insecurity in the tropical Andes. Global Change Biol. 24, e592–e602. doi: 10.1111/gcb.13959
Tito, R., Vasconcelos, H. L., and Feeley, K. J. (2020). Mountain ecosystems as natural laboratories for climate change experiments. Front. Forests Global Change 3, 1–8. doi: 10.3389/ffgc.2020.00038
Tregidgo, D., Campbell, A. J., Rivero, S., Antonio, M., Freitas, B., and Almeida, O. (2020). Vulnerability of the Açaí palm to climate change. Human Ecol. 48, 505–514. doi: 10.1007/s10745-020-00172-2
Tscharntke, T., Clough, Y., Bhagwat, S. A., Buchori, D., Faust, H., Hertel, D., et al. (2011). Multifunctional shade-tree management in tropical agroforestry landscapes - A review. J. Appl. Ecol. 48, 619–629. doi: 10.1111/j.1365-2664.2010.01939.x
Vaast, P., van Kanten, R., Siles, P., Angrand, J., and Aguilar, A. (2008). “Biophysical interactions between timber trees and Arabica coffee in suboptimal conditions of central America,” in Toward Agroforestry Design: An Ecological Approach. 133–146. doi: 10.1007/978-1-4020-6572-9_9
van Noordwijk, M., Coe, R., Sinclair, F. L., Luedeling, E., Bayala, J., Muthuri, C. W., et al. (2021). “Climate change adaptation in and through agroforestry: four decades of research initiated by Peter Huxley,” in Mitigation and Adaptation Strategies for Global Change. Netherlands: Springer. doi: 10.1007/s11027-021-09954-5
van Noordwijk, M., Lawson, G., Hairiah, K., and Wilson, J. (1996). “Root distribution of trees and crops: competition and/or complementarity,” in Tree-crop Interactions: Agroforestry in a Changing Climate. 221–257. doi: 10.1079/9781780645117.0221
van Straaten, O., Veldkamp, E., Köhler, M., and Anas, I. (2010). Spatial and temporal effects of drought on soil CO2 efflux in a cacao agroforestry system in Sulawesi, Indonesia. Biogeosciences 7, 1223–1235. doi: 10.5194/bg-7-1223-2010
Vandenbeldt, R. J., and Williams, J. H. (1992). The effect of soil surface temperature on the growth of millet in relation to the effect of Faidherbia albida trees. Agric. Forest Meteorol. 60, 93–100. doi: 10.1016/0168-1923(92)90076-G
Vargas Zeppetello, L. R., Cook-Patton, S. C., Parsons, L. A., Wolff, N. H., Kroeger, T., Battisti, D. S., et al. (2022). Consistent cooling benefits of silvopasture in the tropics. Nat. Commun. 13, 708. doi: 10.1038/s41467-022-28388-4
Vasey, D. E. (1985). Household gardens and their niche in Port Moresby, Papua New Guinea. Food Nutr. Bull. 7, 37–43. doi: 10.1177/156482658500700312
Veloz, S., Williams, J. W., Lorenz, D., Notaro, M., Vavrus, S., and Vimont, D. J. (2012). Identifying climatic analogs for Wisconsin under 21st-century climate-change scenarios. Clim. Change 112, 1037–1058. doi: 10.1007/s10584-011-0261-z
Verchot, L., van Noordwijk, M., Kandji, S., Tomich, T., Ong, C., Albrecht, A., et al. (2007). Climate change: linking adaptation and mitigation through agroforestry. Mitigat. Adapt. Strategies Global Change 12, 901–918. doi: 10.1007/s11027-007-9105-6
Vincent, G., de Foresta, H., and Mulia, R. (2009). Co-occurring tree species show contrasting sensitivity to ENSO-related droughts in planted dipterocarp forests. Forest Ecol. Manage. 258, 1316–1322. doi: 10.1016/j.foreco.2009.06.035
Wagner, S., Jassogne, L., Price, E., Jones, M., and Preziosi, R. (2021). Impact of climate change on the production of coffea arabica at Mt. Kilimanjaro, Tanzania. Agriculture. 11, 1–15. doi: 10.3390/agriculture11010053
Waldron, A., Garrity, D., Malhi, Y., Girardin, C., Miller, D. C., and Seddon, N. (2017). Agroforestry can enhance food security while meeting other sustainable development goals. Tropical Conservat. Sci. 10, 1–6. doi: 10.1177/1940082917720667
Whitney, C. W., Luedeling, E., Tabuti, J. R. S., Nyamukuru, A., Hensel, O., Gebauer, J., et al. (2018). Crop diversity in homegardens of southwest Uganda and its importance for rural livelihoods. Agric. Human Values 35, 399–424. doi: 10.1007/s10460-017-9835-3
Wu, J., Liu, W., and Chen, C. (2016). Can intercropping with the world's three major beverage plants help improve the water use of rubber trees? J. Appl. Ecol. 53, 1787–1799. doi: 10.1111/1365-2664.12730
Yin, Y., Ma, D., and Wu, S. (2018). Climate change risk to forests in China associated with warming. Sci. Rep. 8, 493. doi: 10.1038/s41598-017-18798-6
Keywords: ecosystem services (ES), climate resilience, drought, global warming, homegardens
Citation: Watts M, Hutton C, Mata Guel EO, Suckall N and Peh KS-H (2022) Impacts of climate change on tropical agroforestry systems: A systematic review for identifying future research priorities. Front. For. Glob. Change 5:880621. doi: 10.3389/ffgc.2022.880621
Received: 21 February 2022; Accepted: 04 August 2022;
Published: 22 August 2022.
Edited by:
Manuel R. Guariguata, Center for International Forestry Research (CIFOR), IndonesiaReviewed by:
Susan Cook-Patton, The Nature Conservancy, United StatesMathew Mpanda, European Union External Action, Tanzania
Copyright © 2022 Watts, Hutton, Mata Guel, Suckall and Peh. This is an open-access article distributed under the terms of the Creative Commons Attribution License (CC BY). The use, distribution or reproduction in other forums is permitted, provided the original author(s) and the copyright owner(s) are credited and that the original publication in this journal is cited, in accordance with accepted academic practice. No use, distribution or reproduction is permitted which does not comply with these terms.
*Correspondence: Martin Watts, bWF3MnUxN0Bzb3Rvbi5hYy51aw==