- 1Coastal and Marine Lab, Florida State University, St. Teresa, FL, United States
- 2Department of Geological Sciences, University of Florida, Gainesville, FL, United States
Globally, mangrove range limits are expanding, often at the cost of adjacent coastal ecosystems including saltmarshes, potentially leading to a change in ecosystem services such as organic carbon (OC) sequestration. Studies in the southeastern US have focused almost exclusively on Avicennia germinans range expansion, the most cold-tolerant mangroves in North America. The Apalachicola Bay region of north Florida represents the northern range limit of mangroves in the Gulf of Mexico, and uniquely also includes Rhizophora mangle. The objective of this research was to quantify soil OC density beneath both mangrove species and compare results to the soils beneath two contiguous native tidal saltmarsh species: Juncus roemerianus and Spartina alterniflora in a barrier island setting. Dominant plant taxa were not a significant predictor of soil OC density, highlighting the relative importance of site-specific environmental attributes as controls on soil properties. Soil profile δ13C compositions included a range of values reflective of C3 and C4 plant inputs, suggesting that shifts in plant taxa, both from marsh to mangroves and between marsh species, have been occurring at all sites in this study. These findings support much of the literature on mangrove encroachment, which indicates mangrove soil OC concentrations, densities, or stocks are less than or equal to that of co-located tidal marsh habitats. Through a systematic review, the potential of several proposed explanatory variables (climate, environmental setting, plant physiology and productivity, and duration of encroachment) were identified to evaluate how soil OC density in mangrove habitats might increase over time, which is critical to forecasting how continued mangrove expansion might affect blue C storage as these habitats evolve.
Introduction
Ecosystems worldwide are experiencing changes in their spatial distribution and composition because of shifting abiotic factors associated with global climate change (Koch and Mooney, 1996; Vitousek et al., 1997; Monaghan et al., 2016). Increases in temperature, decreases in the frequency and intensity of freeze events, and rising sea level facilitate the latitudinal expansion of mangrove forests in tropical and sub-tropical regions (McMillan and Sherrod, 1986; Grindrod et al., 1999; Hashimoto et al., 2006; Osland et al., 2013; Smith, 2013; Cavanaugh et al., 2014; Saintilan et al., 2014; Giri and Long, 2016). Both poleward widening of mangrove range limits, facilitated by tropicalization, and lateral “within range” expansion, hereafter called “expansion,” generally occur at the expense of graminoid or succulent tidal marshes (Osland et al., 2021); recent evidence indicates that intertidal oyster reefs are a secondary front in mangrove expansion (McClenachan et al., 2021). The replacement of tidal saltmarshes by mangroves can affect coastal wetland function and ecosystem services which in part include habitat/nursery provision, shoreline stabilization, storm surge protection, and source/sink dynamics of organic carbon (OC; Saintilan et al., 2014; Kelleway et al., 2016).
Mangrove encroachment into saltmarshes has sparked considerable interest about potential changes to “blue carbon” (blue C), the OC stored in above- and belowground biomass, and soils of vegetated coastal ecosystems (e.g., Macreadie et al., 2019). Although these coastal blue C ecosystems represent a small global area, they are able to sequester disproportionately large amounts of C (Mcleod et al., 2011). Saltmarshes are estimated to occupy an average global area of 54,950 km2 (Mcowen et al., 2017) with an average OC burial rate of 244.7 g m–2 y–1 (Ouyang and Lee, 2014). Mangroves have a slightly lower range in global OC burial rates (163–180 g m–2 y–1; Breithaupt et al., 2012; Alongi, 2020) than saltmarshes but occupy a greater area (83,495 km2, Hamilton and Casey, 2016). Mangrove and saltmarsh ecosystems typically have higher OC sequestration rates than any other upland or wetland vegetated ecosystems (Mcleod et al., 2011). Mangrove encroachment of saltmarshes alters the areal extent of both wetland types and has the potential to disrupt soil OC storage through changes in the abundance, density, quality, and function (i.e., rhizosphere oxidation or turnover times) of roots and surface litter (Kelleway et al., 2017).
Global-scale differences in estimates of OC burial rates, stocks, and soil densities between mangroves and saltmarshes have been well documented (e.g., Chmura et al., 2003; Ouyang and Lee, 2020). However, these global comparisons commonly overlook small-scale spatial drivers of mangrove encroachment within different regions that can obscure comparisons of C sequestration and OC burial efficiency. For example, mangrove encroachment of saltmarshes often occurs at the latitudinal range limits of mangroves, where they are subjected to suboptimal growth conditions that may limit expected differences between saltmarsh and mangrove productivity (Mckee and Rooth, 2008; Soares et al., 2012). Previous work on mangrove encroachment of saltmarshes has shown conflicting results on resultant changes in OC storage in aboveground and belowground biomass, and in soils (Kelleway et al., 2017). However, the role of different mangrove taxa as a variable in OC burial and saltmarsh encroachment has been largely unexamined. Previous studies have focused almost exclusively on Avicennia germinans, colloquially known as the “black mangrove,” the most cold-tolerant of mangroves in North America and hence the most prevalent mangrove taxa at the northern range limits in the Gulf of Mexico (Figure 1C and references therein). Moreover, previous studies have often generalized multiple herbaceous or succulent species occupying saltmarshes into one collective category, often simply called “saltmarsh” or “tidal marsh,” ignoring potential species differences in soil OC storage (Steinmuller et al., 2020).
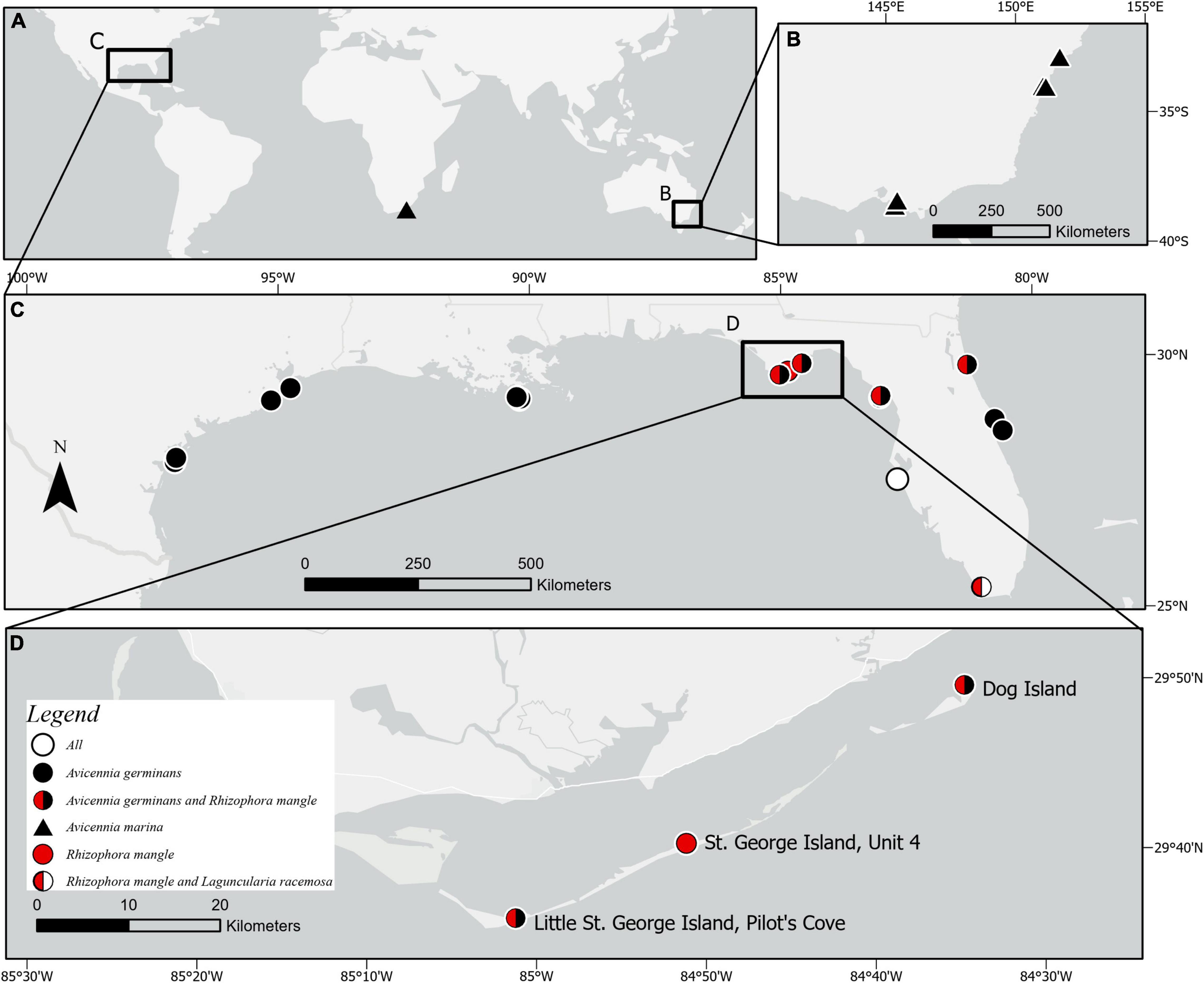
Figure 1. (A) Global extent of sites experiencing mangrove encroachment into tidal marsh with measurements of soil organic carbon (References in Table 4) with insets of (B) Southeast Australia, (C) North America, and (D) Study area of Apalachicola Bay region, including Dog Island, St. George Island, and Little St. George Island. The single study documenting mangrove encroachment into tidal marsh with a measurement of soil organic C in S. Africa is shown on the global map.
The central focus of this study was to address the hypothesis that mangrove encroachment into saltmarsh will increase OC storage. Our first objective was to examine OC density (a metric for OC storage) in surficial (0–20 cm) soils dominated by specific taxa of encroaching mangroves (A. germinans and Rhizophora mangle) and native saltmarsh (Spartina alterniflora and Juncus roemerianus) in a barrier island setting in Apalachicola Bay, FL, United States. In order to understand whether soil OC is derived from present day aboveground vegetation, the second objective of this study was to characterize the types of vegetation shifts that are reflected in soils throughout the Apalachicola Bay region (e.g., mangrove encroaching on marsh or low marsh encroaching on high marsh) by using soil C:N and δ13C depth profiles. Finally, the third objective of this study was to place our results in the context of other studies that have compared the density, concentration, or belowground stocks of soil OC and/or OM between co-located mangrove and saltmarshes along global mangrove range expansion fronts.
Materials and Methods
Study Sites
Sampling sites were located on the barrier islands of the Apalachicola Bay region of Florida in the northern Gulf of Mexico (Figure 1), where mangrove range expansion has occurred over the past three decades (Snyder et al., 2021). The landward margins of these barrier islands are characterized by a patchwork of vegetation communities, with no linear progression of mangrove encroachment of marshes. Within Apalachicola Bay, the encroachment of mangroves into adjacent marshes is occurring in a stochastic manner, resulting in high spatial variability of mangrove presence/absence and stem density at different sites and locations. Avicennia germinans have been present in this region since at least 1941, and R. mangle since at least 1955 (Snyder et al., 2021), albeit in considerably lower numbers. The four species targeted in this study are found growing in close proximity to one another in a patchwork mosaic of coastal wetlands in the Apalachicola Bay region of northern Florida (Figure 1D), and the two marsh species selected represent the most common herbaceous marsh vegetation types within the inland barrier island marshes (Snyder et al., 2021). Other vegetation consists of Salicornia perennis, Batis maritima, Borrichia frutescens, and Baccharis halimifolia (Snyder et al., 2021). Soils were sampled from the center of the most homogeneous and mature locations of each plant taxon, at the following three sites, where mangroves are most abundant in the region: Dog Island (DI) (84.576399°W, 29.824802°N, ∼ 120,000 m2), Unit 4 (U4) (84.852959°W, 29.670528°N, ∼ 11,000 m2), and Pilot’s Cove (PC) (85.010329°W 29.601422°N, ∼245,500 m2) (Figure 1D). The distance from Dog Island, the easternmost site, to Pilot’s Cove, the westernmost site, is approximately 50 km. All four target vegetation taxa (A. germinans, R. mangle, S. alterniflora, and J. roemerianus) occur in close proximity to one other at each site except for Unit 4, where there are no A. germinans.
Sampling Methods
A total of 33 soil cores were collected, 12 at both DI and PC from each of the four dominant plant taxa, and 9 at U4 from each of the three dominant plant taxa (n = 3), by hammering a 10.16 cm interior-diameter polyvinylchloride (PVC) pipe into the soil to either the sand layer, or a maximum depth of 50 cm. Soil samples taken from A. germinans were collected from within 1 m of the main stem of the mangrove itself, while soil samples taken from R. mangle were taken from within the space constrained by mangrove prop-roots. Juncus roemerianus and S. alterniflora soil cores were taken from within a homogenous section of the target taxon. To reduce the risk of spatial autocorrelation, each replicate was sampled from a different mangrove stand or swath of herbaceous vegetation. All cores were capped, transported to the laboratory on ice, and processed within 2 weeks of field collection. A two-cm section was collected from the middle of each 5-cm depth interval to the bottom of each core.
Soil Physicochemical Analyses
A total of 216 soil core intervals (Table 1) were weighed wet, dried at 60°C until a constant weight was achieved (generally 24–48 h), and then re-weighed to determine moisture content and dry bulk density. Dry bulk density was calculated as dry sample mass divided by initial wet core section volume. Dried samples were subsequently homogenized using a ceramic mortar and pestle and a SPEX 8000M Mixer-Mill (Metuchen, NJ, United States). Total organic C and its δ13C were measured with acid-fumigated samples (sensu Harris et al., 2001); total nitrogen and δ15N were measured using non-fumigated samples. Total nitrogen analyses were included to derive molar OC:N ratios. Analysis was conducted at the Light Stable Isotope Lab in the Department of Geological Sciences at University of Florida using a Thermo Electron DeltaV Advantage isotope ratio mass spectrometer running in continuous flow mode coupled with a ConFlo II interface linked to a Carlo Erba NA 1500 CNHS Elemental Analyzer. Samples were measured relative to laboratory reference N2 and CO2 gases with USGS40 standards. Carbon isotope results are expressed relative to VPDB, while nitrogen isotope results are expressed relative to AIR, both in standard delta notation.
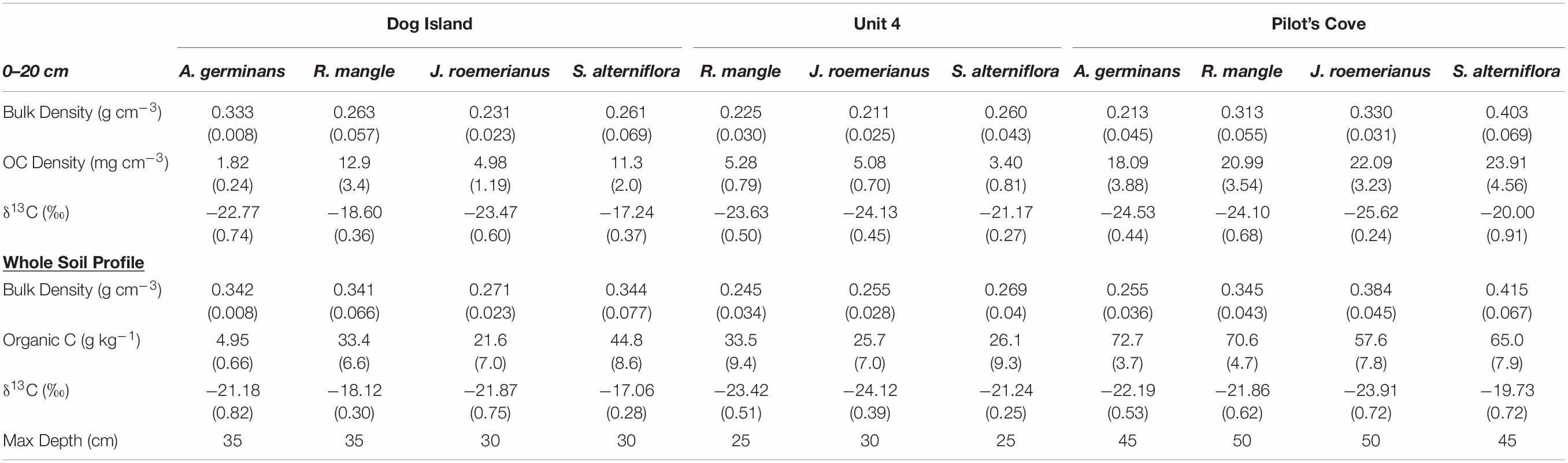
Table 1. Mean (±1 standard error) of each measured soil characteristic beneath dominant plant taxa at each site.
Data Analysis
Due to the sampling locations being on barrier islands, soil profiles began to transition to sand at depths below ca. 20 cm. The sand layer was present at DI at 20–25 cm and PC at 30–35 cm but was shallowest at U4 (Table 1). For the purposes of comparing OC density between plant taxa at each site, we restricted comparisons to a depth of 0–20 cm (n = 132). At depths greater than 20 cm, the prevalence of sand would skew bulk density toward higher values and shifting OC density toward values no longer representative of the overlying plant community. With the exception of OC density, all other values are reported to incorporate the entire soil-depth profile (n = 216). Maximum depths for each site and plant taxon are reported in Table 1, along with 0–20 cm dry bulk densities used to calculate OC density.
To address the second objective, soil δ13C values were compared to the expected isotopic signature of present-day vegetation; for this analysis, dominant plant endmembers consisted of S. alterniflora, a C4 plant, and J. roemerianus, A. germinans, and R. mangle, all C3 plants (S. alterniflora: −9 to −16 ‰; J. roemerianus, R. mangle, A. germinans: −20 – - 31‰). These comparisons qualitatively identify the following types of coastal plant vegetation shifts in the region: saltmarsh species replacement of other marsh species and mangrove taxa replacement of saltmarsh taxa, with the assumption that soil profiles represent chronological changes in plant communities (Figures 2, 3). We considered both the shallow soils (0 – 20 cm) used for our comparisons of OC density, and deeper soils (> 20 cm) separately to qualitatively identify the presence of antecedent vegetation. If values occurred between the C3 and C4 bounds (Lamb et al., 2006), then the soil was classified as “mixed” and attributed to a combination of S. alterniflora and one of the three C3 plant taxa. If the soil beneath a mangrove was C3-dominant, then the antecedent vegetation was classified as either that same mangrove type or J. roemerianus. When the soil beneath a mangrove was “mixed,” the expectation was that S. alterniflora was the primary driver of enriched δ13C values. When soil beneath S. alterniflora was “mixed,” antecedent vegetation was attributed to J. roemerianus because there has been no evidence of mangrove mortality at these sites in recent decades (personal observations).
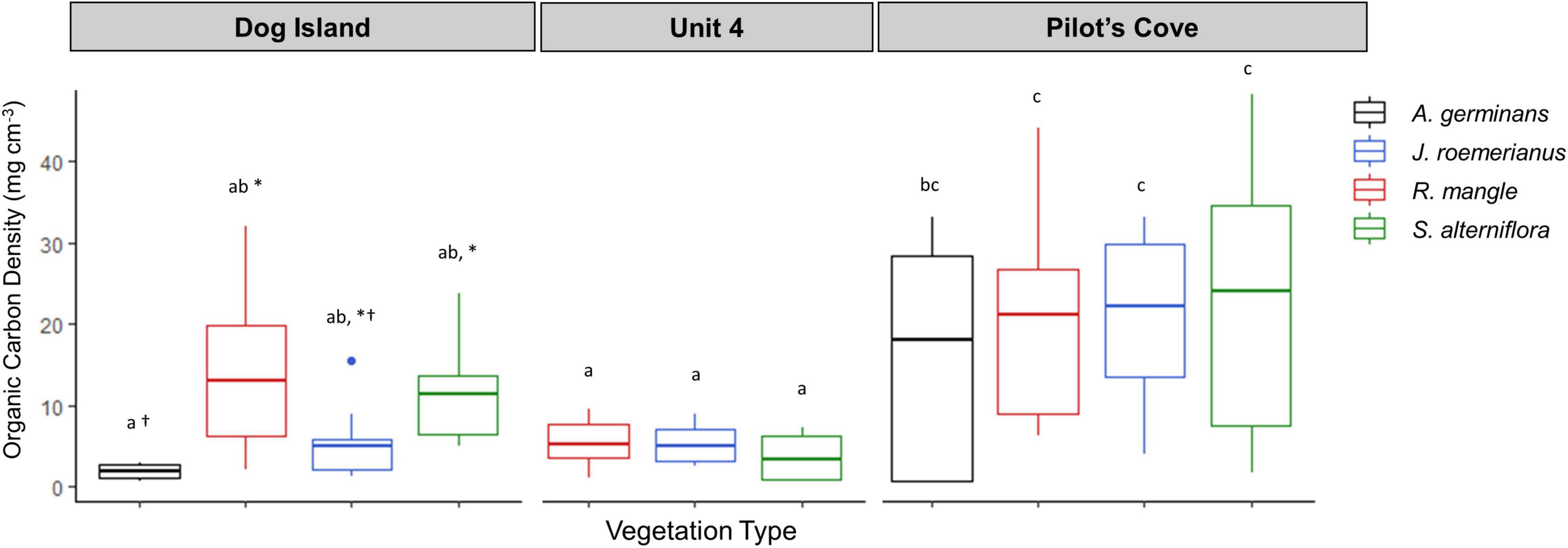
Figure 2. Boxplots of soil organic carbon density beneath each vegetation type as indicated by color, at each site. Horizontal lines in boxplots indicate mean. Lower and upper hinges indicate the first and third quartiles and upper and lower whiskers denote values within 1.5 the interquartile range. Lowercase letters indicate significant differences across sites, while asterisks and crosses indicate statistical differences within site.
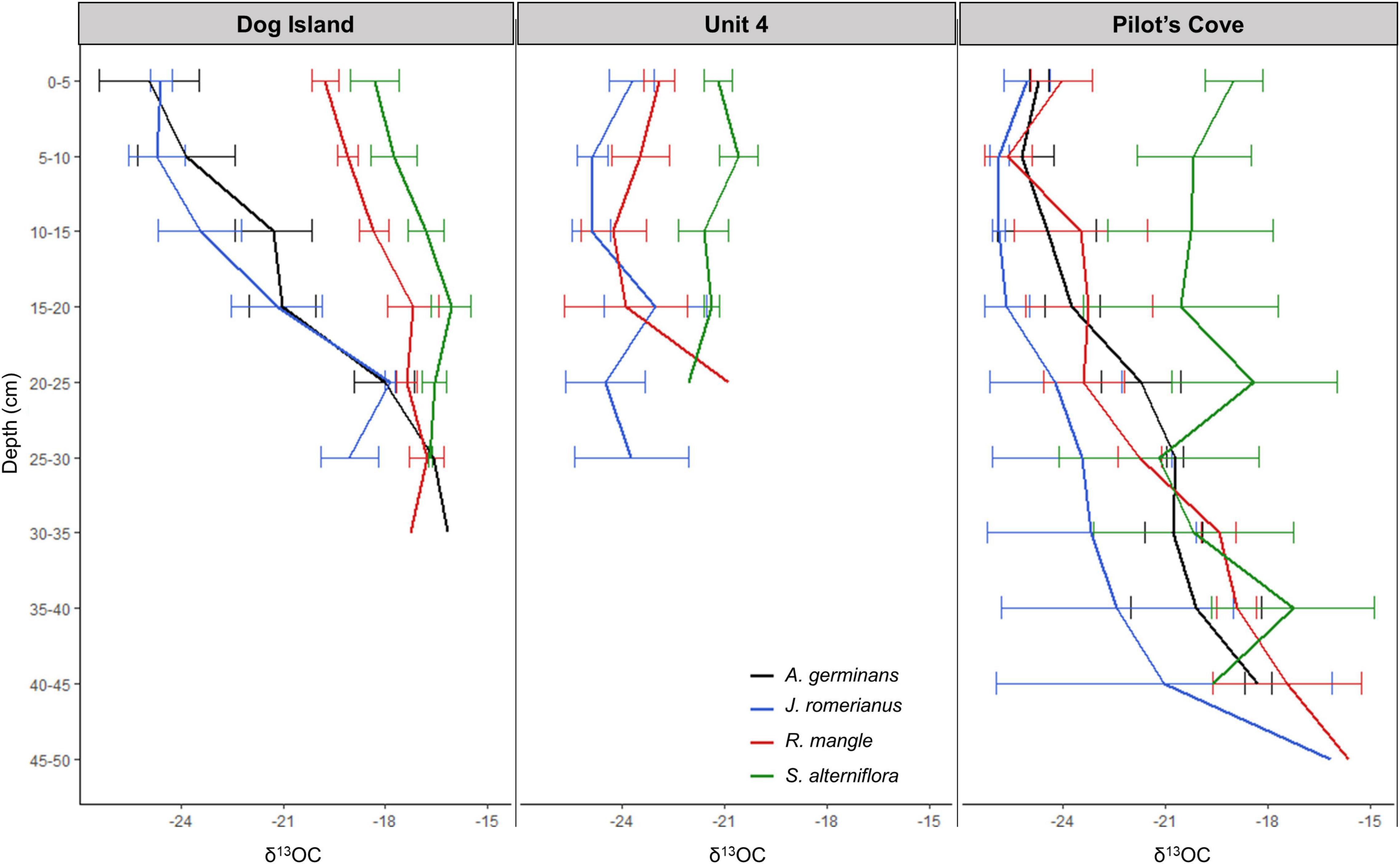
Figure 3. Means and standard error of δ13OC (‰) values, plotted by depth (in 5 cm intervals) and vegetation type (denoted by color) at each site.
All statistical analyses were performed in R (Version 4.1.0; R Institute for Statistical Computing, Vienna, Austria) with RStudio (Version 1.4.1717; RStudio Inc., Boston, MA, United States). Assumptions of normality and homogeneity of variance were verified using the Shapiro–Wilk test and Levene’s test, respectively. A linear model was used to test the effects of vegetation type, site, and the interaction between the two, within the 0–20 cm depths, for the following parameters: OC density, δ15N, and molar OC:N. For this analysis, depths were pooled over the 20 cm interval (n = 12). Soil δ13C was evaluated using a linear model to test the effects of vegetation type, site, depth (pooled 0–20 cm vs. pooled 20 + cm), and the interactions among them. Following determination of significant differences between means among predictor variables, post-hoc pairwise comparisons were made using package “emmeans” via the Tukey method. All values are reported as mean ± standard error.
Systematic Review
A Web of Science keyword search was conducted to identify literature reporting concentration, density, or belowground stocks of OC or OM within co-located mangroves and saltmarshes along global encroachment fronts. Keywords consisted of “mangrove,” “marsh,” “soil,” “organic carbon,” and “organic matter.” Results were further refined by examining each study to determine if mangrove and saltmarsh sites were co-located and represented an encroachment front. The relationship between mangrove and saltmarsh OC or OM concentration, density, or belowground stocks was either 1) determined through a direct statement by the authors concerning the statistical difference between the two vegetation types (mangrove or marsh, or 2) determined, in the absence of a direct statement of the statistical difference between the two vegetation types, by values extracted from the individual paper.
Results
Soil Organic Carbon Concentration and Density
Concentrations of OC were not significantly different among dominant plant taxa (p = 0.1) but were different between sites (p < 0.001), and with interactions between dominant plant taxa and site (p = 0.02). The only within site difference of OC concentration occurred at DI, where A. germinans was significantly lower than S. alterniflora (Table 1). Average OC concentrations at DI and U4 were significantly different from OC concentrations at PC; the highest OC concentrations were observed at PC and averaged 66.5 ± 3.12 g kg–1. Across all sites and vegetation types, soil OC ranged from 4.95 ± 0.66 g kg–1 in A. germinans at DI to 72.7 ± 3.7 g kg–1 in A. germinans at PC (Table 1).
Organic C density differed by site and the interaction between dominant plant taxa and site (Tables 1, 2). Though there were no differences in OC density among dominant plant taxa at PC and U4, at DI, soil OC density underlying A. germinans was significantly lower than soil OC density underlying both S. alterniflora (p = 0.03) and R. mangle (p = 0.0006; Figure 4 and Table 1).
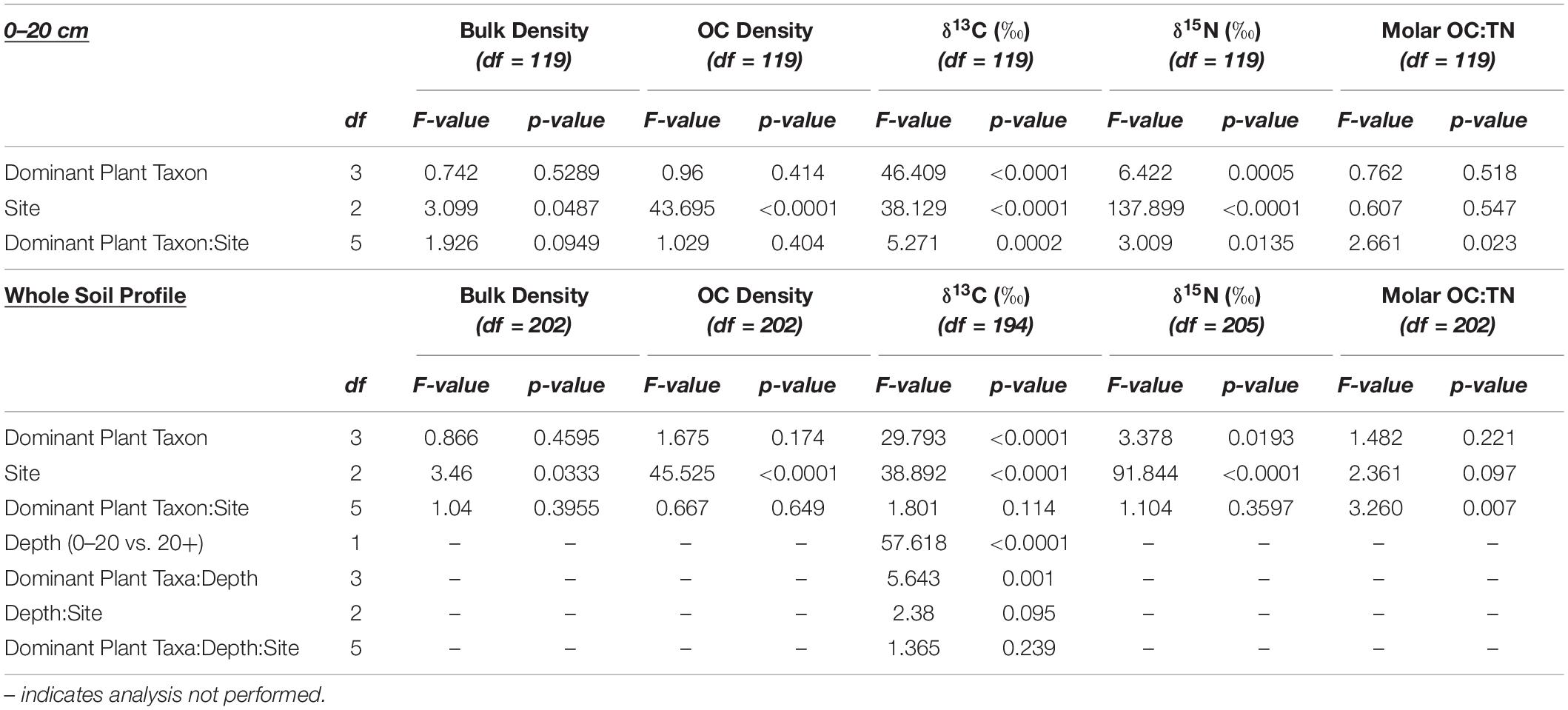
Table 2. Degrees of freedom (df), F-values, and p-values associated with the linear model, separated by parameter.
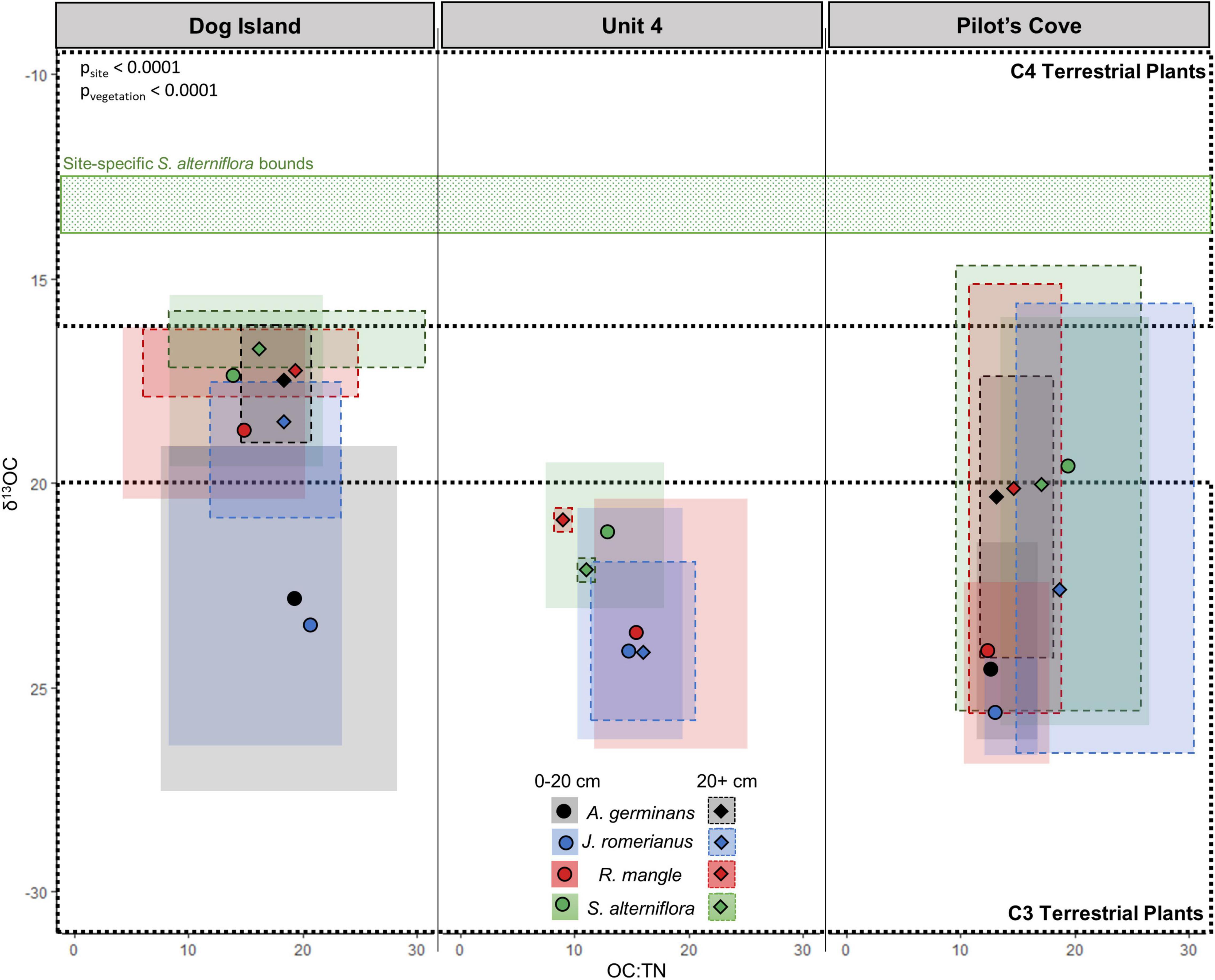
Figure 4. Ratio of organic carbon to total nitrogen, plotted with δ13OC by site (entire soil profile). Points represent mean and the size of the box represents the spread of the data. Dashed boxes and diamonds denote depths greater than 20 cm while unbounded boxes and circles denote depths less than 20 cm. Bounds of C3 and C4 terrestrial plants from Lamb et al. (2006) are indicated by bold dashed boxes. Site-specific S. alterniflora bounds are from Chanton and Lewis (2002).
δ13C, δ15N, and OC:N
Soil δ13C values differed among sites and dominant plant taxa, but not the interaction between the two (Tables 1, 2). Soil δ13C values in J. roemerianus habitats averaged −23.34 ± 0.40 ‰ and were significantly different from R. mangle soils, which averaged −21.05 ± 0.41‰, and S. alterniflora soils, which averaged −19.21 ± 0.43‰ (Figures 2, 3). In terms of site, δ13C at DI were significantly different from U4 and PC, which did not differ from each other. The most enriched values were observed at DI and averaged −19.5 ± 0.37‰. δ15N values also differed among sites (p < 0.0001) and dominant plant taxa (p = 0.02), but not the interaction between them (p = 0.4). δ15N values were lowest at DI, averaging 1.37 ± 0.12‰. Pilot’s Cove and U4 δ15N values did not differ from each other. Across all sites and dominant plant taxa, δ15N values ranged from 1.12 ± 0.26‰ in R. mangle soils at DI to 4.45 ± 0.15‰ in R. mangle soils at U4. The interaction between dominant plant taxa and site significantly affected soil molar OC:TN (Figure 3 and Table 2).
Systematic Review of Co-located Mangrove and Marsh Soil Organic Matter And/Or Organic Carbon Literature
Twenty studies were identified as investigating the differences in the density, concentration, or belowground stocks of OC or OM within co-located mangrove and saltmarsh soils along encroachment fronts. These twenty studies represented 33 individual sites or zones located in North America, South Africa, and Southeast Australia (Figure 1, Table 3, and Supplementary Table 2). Only 12% of these 33 individual sites or zones documented higher OC density/concentrations in mangrove soils, 27% documented higher density/concentrations in saltmarsh soils, and 61% showed no differences between the two vegetation types (Table 3).
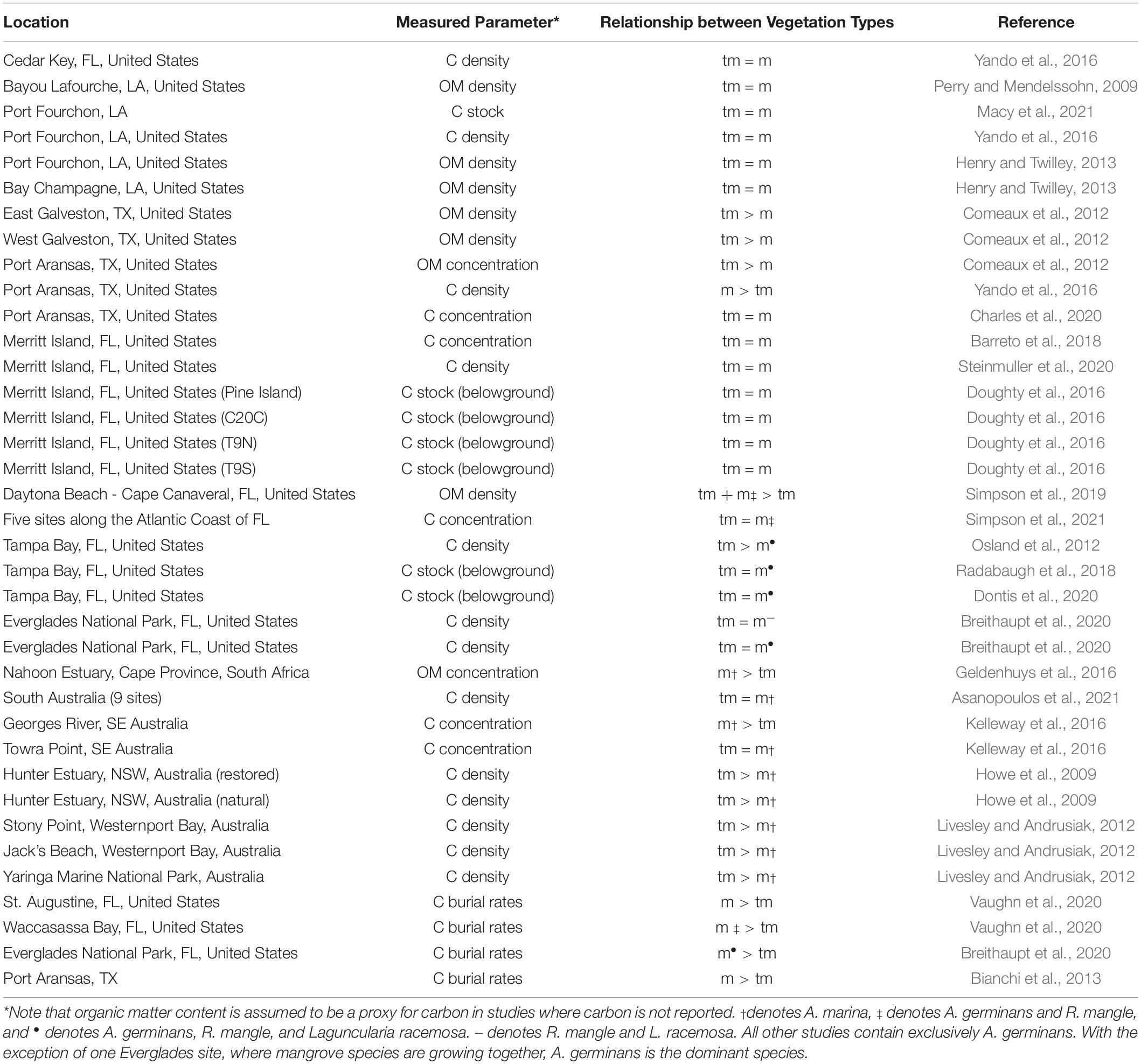
Table 3. Relationship of measured soil carbon or organic matter characteristics [density (g cm–3), belowground C stocks (Mg ha–1), concentration (g kg–1), and burial rates (g C m–2 y–1)] between vegetation types (mangrove = m; tidal marsh = tm) at locations along encroachment fronts in the northern Gulf of Mexico, southeastern Australia, and South Africa (ordered by latitude).
Discussion
Site-Specific Controls on Soil Organic Carbon
Differences in overlying dominant plant taxa had no effect on soil OC density (Figure 4) in the top 20 cm, except at the DI site where R. mangle and S. alterniflora had higher OC densities than A. germinans. Most differences reported herein are related to site rather than dominant plant taxa, highlighting the relative importance of site-specific attributes that may include elevation, sediment supply, nutrient availability, hydrodynamics, geomorphic setting etc., (Livesley and Andrusiak, 2012; Henry and Twilley, 2013; Rovai et al., 2018; van Ardenne et al., 2018; Steinmuller et al., 2020) as controls on soil properties. For example, the observed increase in δ15N values from around 0 – 3‰ at DI to 2 – 6‰ at PC and U4 likely suggest the presence of a greater percent of organic nitrogen (Peterson and Fry, 1987), potentially because of detrital outfalls from the Apalachicola River floodplain (PC) and/or limited tidal exchange (U4). Environmental characteristics that might be responsible for controlling C storage within wetland soils, though not quantified within this study, merit further scrutiny to begin identifying and defining key characteristics responsible for controlling C storage of wetland soils. These findings further illustrate that using plant cover (saltmarsh vs. mangrove) as the sole predictor for estimating landscape-scale soil OC storage along encroachment fronts can be misleading; environmental setting can have more influence on soil OC storage than dominant plant taxa.
Evolving Shifts in Plant Taxa
It is important to consider the role of time in the context of mangrove establishment in this region where rapid mangrove expansion appears to be a recent phenomenon (Snyder et al., 2021). Consequently, care should be taken when attributing soil OC to the present type of surface vegetation. The δ13C composition of the soils at these sites include a range of values that indicate contributions from both C3 and C4-pathway plants (Lamb et al., 2006) and suggest that shifts in aboveground dominant plant taxa are not uncommon at these sites (Figures 2, 3). All three sites demonstrate locations of unambiguous marsh – marsh shifts within the shallow soils (0–20 cm) where present-day S. alterniflora occupies locations previously occupied by J. roemerianus (e.g., Ember et al., 1987; Figure 3), evidenced by the dilution of S. alterniflora signal in the soil with C3 J. roemerianus indications. These shifts are likely to have occurred recently because the majority, if not all, of the data points have δ13C values that are more negative (indicative of J. roemerianus) than the expected bounds for S. alterniflora soils (Figure 3). Though we cannot assign an exact timescale to these shifts, we can use regional record of relative sea level rise rates of 2.7 ± 0.61 mm y–1 between 1967 and 2020 (NOAA Station 8728690, Apalachicola FL), as a rough proxy for the rate of vertical development of these wetland soils (Breithaupt et al., 2017). Consequently, these “recent” shifts, observed in the top 20 cm of the soil have likely occurred within the past 60–95 years. The prevalence of areas that were previously dominated by high elevation marsh (J. roemerianus) which are now occupied by low elevation marsh (S. alterniflora) suggests that these locations are more inundated than they were in the past (Silberhorn, 1999) and indicates regional influence of sea-level rise. Similarly, sea-level rise is contributing to isolation of patches of S. alterniflora (Figure 5E). In shallow soils, locations that are presently J. roemerianus appear to have continuously been J. roemerianus throughout the past, however, a more mixed signal in the deeper soils suggests that S. alterniflora once occupied these soils (Figure 2).
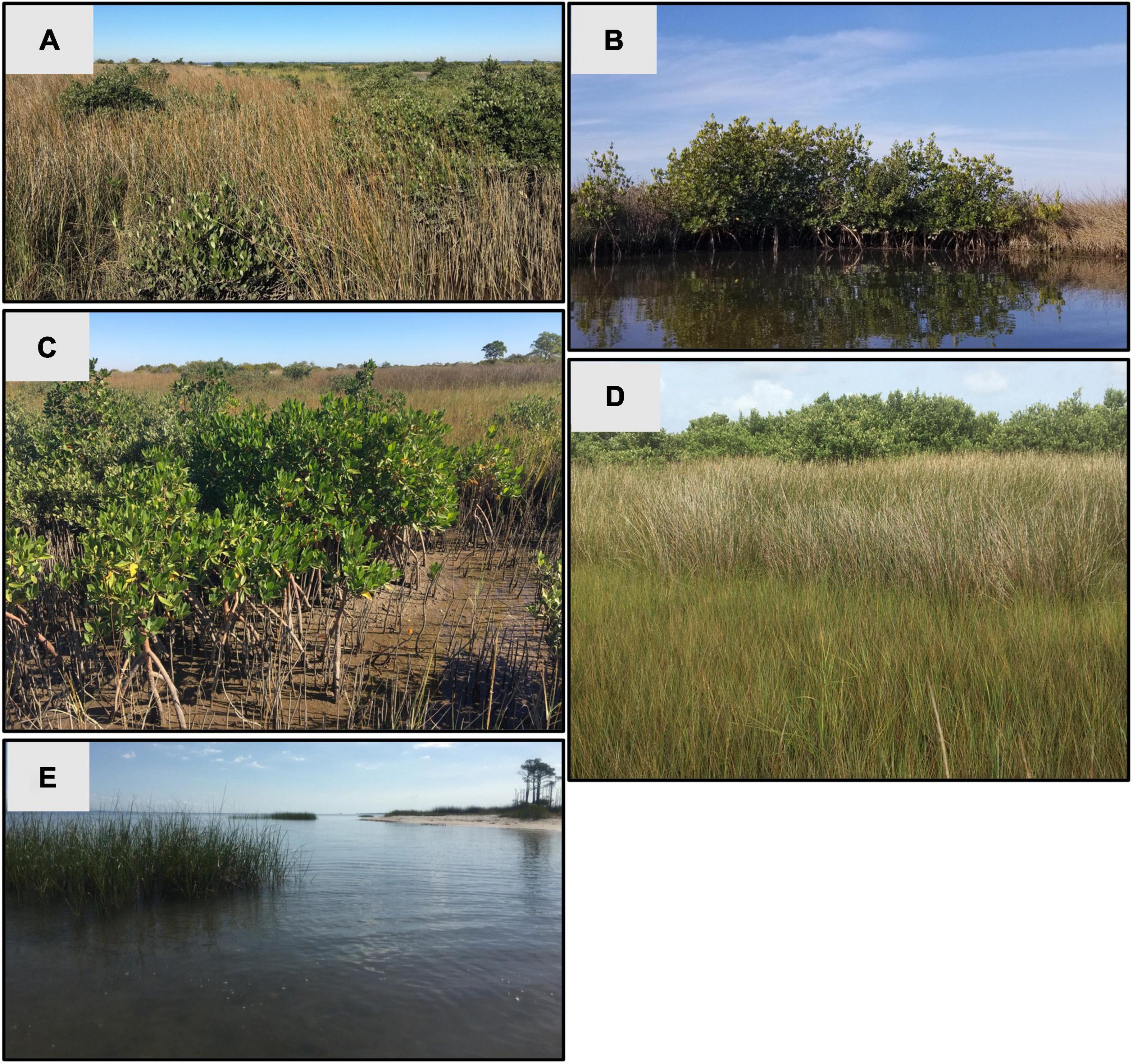
Figure 5. Regional photos of neighboring vegetation types. (A) Shows A. germinans replacement of J. roemerianus on Dog Island; (B) shows R. mangle replacement of J. roemerianus at Pilot’s Cove. (C) Shows A. germinans and R. mangle adjacent to S. alterniflora on Dog Island. (D) Shows S. alterniflora in the foreground, J. roemerianus in the middle, and A. germinans in the background at Pilot’s Cove. (E) Shows patches of S. alterniflora being inundated and isolated by sea-level rise at Unit 4.
Dog Island provides evidence of an unambiguous marsh - mangrove shift from S. alterniflora to R. mangle (Figures 2, 3) within the shallow soils, a signal that persists in the deeper soils. This type of transition is further supported by observations from the field where R. mangle commonly borders S. alterniflora (Figure 5C). This shift is in an early transitional stage, based on δ13C values which reflect a mixture of both C3 and C4 plants (Figure 3). Interestingly, this likely indicates an incomplete substitution of previous S. alterniflora by the present R. mangle community. The δ13C of shallow soils underlying R. mangle at U4 and PC sites are ambiguous about whether R. mangle has been present throughout the entire time represented by the soil profile, or whether a shift from J. roemerianus occurred at some point. This too is supported by field observations of R. mangle commonly identified growing near J. roemerianus at these two sites (Figure 5B). Additionally, the deep soils beneath R. mangle at PC and U4 are substantially more enriched than those of the shallow soils, indicating that S. alterniflora was the original species present when wetlands first occupied the sandy soil at the bottom of these profiles (Figures 2, 3). Additionally, at DI and PC, the δ13C of soils underlying A. germinans could represent continual occupation by A. germinans or a shift from J. roemerianus and S. alterniflora in the deeper soils (Figures 5A,D).
Inconsistencies With Literature-Based Predictions
Our data agree with the literature on mangrove encroachment, which shows that mangrove soil OC concentrations, densities, and/or stocks are less than or equal to antecedent tidal marsh soils (Table 3). However, as Table 4 illustrates, even studies that report no such increase at present contend that carbon storage is likely to increase with continued mangrove expansion into marsh soils (Table 4). What factors contribute to the prevailing view that mangrove encroachment will increase soil OC if only 12% of the empirical data support this idea (Table 3)?
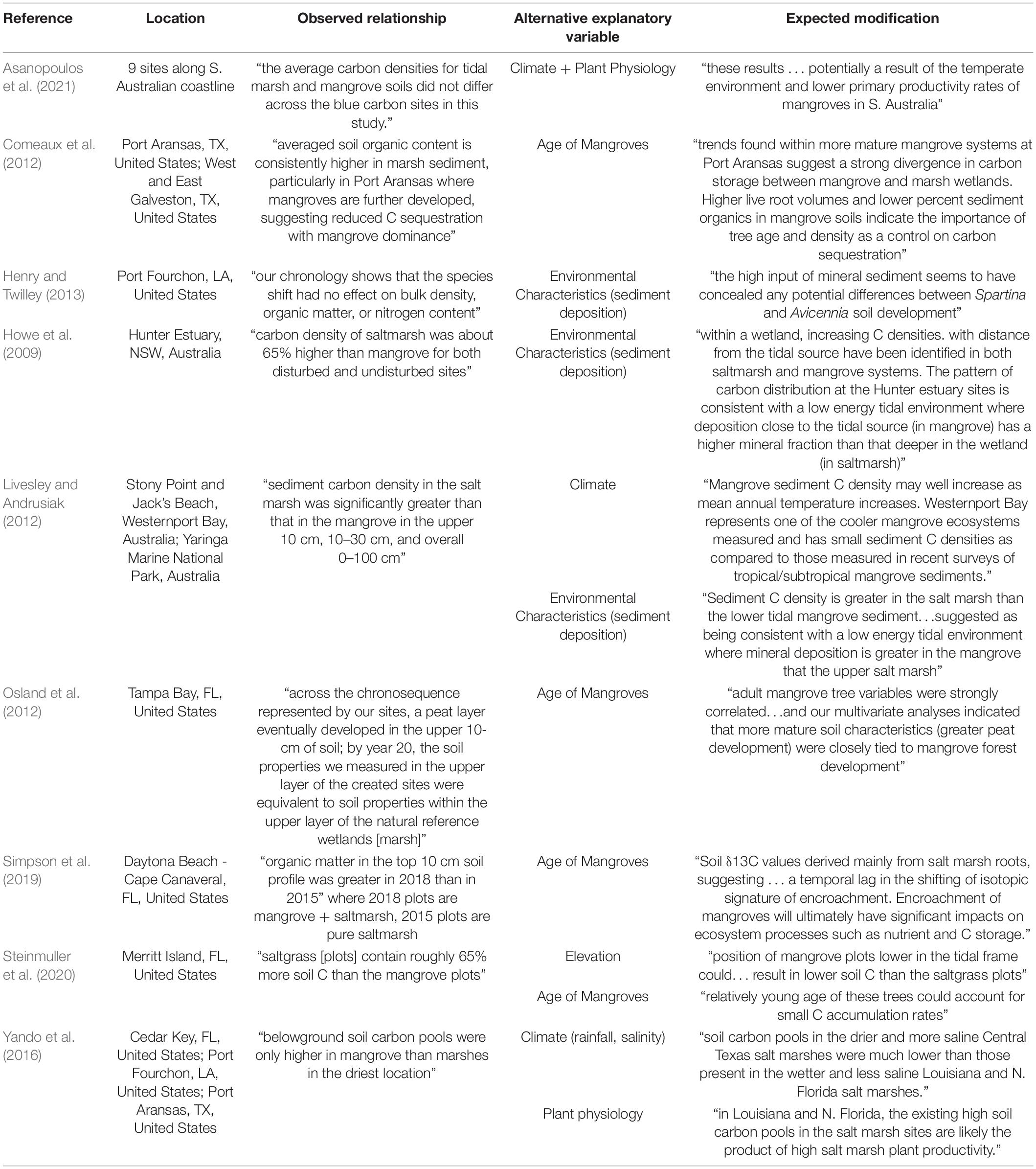
Table 4. Studies with published C density relationships between mangroves and tidal marshes along encroachment fronts with quotes that explain (A) the observations between the two dominant plant taxa, and (B) expectations of how alternative explanatory variables might modify that relationship.
We hypothesize that this dichotomy between observations and expectations is due to differences in scale between global estimates and local-scale comparisons of mangrove and saltmarsh soil characteristics. For example, in contrast to many of the comparisons at mangrove encroachment fronts where no differences have been found (Table 3), global mean values indicate that: “The average soil carbon density of mangrove swamps (0.055 ± 0.004 g cm–3) is significantly higher than the salt marsh average (0.039 ± 0.003 g cm–3)” (Chmura et al., 2003). However, these global numbers are collected and reported in a different analytical context. The data used for the global comparisons of C density did not come from sites where both mangroves and saltmarshes were co-located; rather, most mangrove data originated in the tropics and subtropics where their productivity is higher than at poleward expansion fronts. Similarly, the majority of the global saltmarsh data originated in temperate zones, which have lower productivity rates (Chmura et al., 2003) than saltmarshes occupying encroachment fronts. However, we also hypothesize that the regional-scale findings of no difference between vegetation type are not necessarily at odds with the global findings that there is a difference. Both “within site” lateral expansion and mangrove poleward range expansion are forecasted to continue (Chapman et al., 2021), facilitated by warming (Coldren et al., 2019; Sturchio et al., 2021), especially at their poleward range limit (Chapman et al., 2021), decreased frequency of damaging or mortality-inducing freezes (Cavanaugh et al., 2014), and the availability of optimal abiotic characteristics, often mediated by saltmarsh vegetation (i.e., Adgie and Chapman, 2021). Though the regional data do not yet support the claim that mangrove encroachment of saltmarshes will increase blue C storage (Table 3 and Supplementary Table 1), there is context to surmise that these comparisons will change as mangrove range expansion continues. Throughout the literature on mangrove range expansion, several explanatory variables have been proposed that can influence soil OC dynamics as a function of surface vegetation along mangrove encroachment fronts; these are discussed in the following paragraphs and include: climate, environmental variables, physiology and productivity, and duration of vegetation development.
Rainfall and temperature are key factors controlling differences in soil OC density between mangroves and saltmarshes over broad spatial scales (Livesley and Andrusiak, 2012; Yando et al., 2016; Asanopoulos et al., 2021). Additionally, environmental characteristics such as geomorphic setting (informing type and rate of sediment deposition), tidal frame position, salinity, and elevation have been cited as drivers of different soil OC density within and between coastal vegetation types along mangrove encroachment fronts on saltmarshes (Livesley and Andrusiak, 2012; Henry and Twilley, 2013; Rovai et al., 2018; van Ardenne et al., 2018; Steinmuller et al., 2020). Overall, these climate and environmental drivers may lead to differences over broad spatial scales, but they affect all vegetation types equally across this region and therefore are not expected to lead to changes between saltmarsh and mangrove soil OC storage in the future.
Alternatively, there is reason to expect that mangroves at range expansion fronts will increase in productivity given more time, continued warming, and infrequent freeze events (Coldren et al., 2019; Chapman et al., 2021). Worldwide, mangrove species have higher rates of gross primary productivity on average than saltmarsh species (Alongi, 2020). In contrast, greater primary productivity in saltmarshes (S. alterniflora) compared to mangroves (A. germinans) has been reported at an encroachment interface, attributed to suboptimal growth conditions for mangroves at the edge of their range limits (Mckee and Rooth, 2008). Differences in primary productivity can translate to differences in soil organic matter accumulation and soil OC densities and have been cited as a factor explaining higher soil OC pools in saltmarsh relative to mangrove sites (Yando et al., 2016). Though we made no assessments of above- or belowground productivity in this study, our observations of different mangrove heights, from less than 1 m at DI to greater than 3 m at PC, suggest that productivity may vary between sites and contribute to between-site difference in OC density (Figure 4).
The length of time that expanding mangroves have been encroaching on saltmarshes at mangrove range limits is another factor leading to the pervasive expectation (Table 4) that mangroves increase the soil carbon storage relative to that of the wetlands where they are expanding. From this perspective, the expectation is that mangroves have simply not been present at these range limits for long enough or with conditions suitable to stimulate their development to full maturity; young stand age of mangroves has been proposed to explain why they have equal or lower soil OC density/concentrations compared to regionally proximal marsh soils (Perry and Mendelssohn, 2009; Comeaux et al., 2012; Osland et al., 2012; Simpson et al., 2019; Steinmuller et al., 2020; Table 4). Soil OC concentrations are positively correlated with mangrove stand age; thus, young mangrove stands may have lower soil OC concentrations than the nearby established saltmarsh because of differences in biomass production (Lunstrum and Chen, 2014). Across a chronosequence of mangrove restoration in Tampa Bay, FL, Osland et al. (2012) found that after 20 years of mangrove growth and development, soil organic matter content within the top 10 cm were equivalent to soil organic matter content of nearby reference marshes. Kelleway et al. (2016) demonstrated similar results: along two 70-year chronosequences of mangrove growth in Southeast Australia, mangroves and saltmarsh exhibited equal C storage within the upper 15 cm at one site, and higher C concentrations in the top 50 cm of the soil profile in 70-year-old mangroves compared to saltmarsh at another site. This suggests that for timescales on the order of decades, newly encroaching mangrove soil OC densities increase to match, or become higher than those of saltmarsh. At our sites, freeze events have likely slowed or set back mangrove productivity several times since the middle of the last century (Snyder et al., 2021). The most severe freeze within Apalachicola Bay was in December 1989, where temperatures dipped to −10°C, low enough to cause mangrove mortality (Snyder et al., 2021).
Another aspect of time related to OC storage (or OC density) is that of OC burial rates. Measures of soil OC density have no time component; they are representative of both present-day conditions and the integration of an unknown amount of the time in the past. Organic carbon burial rates however specifically quantify the amount of OC that accumulates in the soil in a given period of time. Two sites with equal carbon densities can have different rates of OC burial. This is evidenced from sites where radiometric dates have been used to measure OC burial rates at sites of mangrove encroachment on saltmarshes (Bianchi et al., 2013; Breithaupt et al., 2020; Vaughn et al., 2020). In each of these three cases, burial rates in mangroves were higher than those in saltmarshes. In the Everglades where mangroves are encroaching on fresh and brackish marshes, the OC burial rates were substantially higher even though OC densities were not statistically different (Breithaupt et al., 2020). It can be expected that the continued higher rates of burial in mangrove soils will eventually result in mangrove soil OC densities surpassing those of saltmarshes, though it is critical to understand that at the present time, this is not the case.
Furthermore, these differences in burial rates by dominant plant taxa prompt a discussion of differences in organic carbon “quality.” Though the mean global burial rates of mangroves are slightly lower than associated mean burial rates of saltmarshes, mangrove soils accumulate more recalcitrant carbon than saltmarsh soils, like those occupied by S. alterniflora, which accumulate more labile OC (Cui et al., 2021). Bianchi et al. (2013) and Vaughn et al. (2020) both reported higher lignin inputs into mangrove soils than saltmarsh soils, suggesting more recalcitrant compounds within mangrove soils. Similarly, rates of mineralization of C and N have been demonstrated to be greater in saltmarsh soils compared to mangrove soils (Steinmuller et al., 2020; Geoghegan et al., 2021). Together, these conclusions suggest greater preservation potential and resilience to mineralization associated with relative sea level rise and other aspects of global climate change within mangrove soils, relative to saltmarsh soils.
Conclusion
It is critical to understand how mangrove encroachment of saltmarshes alters global C sequestration and burial in these vital coastal habitats. In Apalachicola Bay, FL, at sites along the interior barrier islands, where two taxa of mangroves are encroaching on two saltmarsh taxa, no significant relationship was found between soil OC density and overlying dominant plant taxa. Instead, soil OC densities differed by site, which suggests that other environmental differences appear to have more influence in determining soil OC storage than dominant plant taxa. Consequently, we posit that using mangrove and saltmarsh plant cover as the sole metric for changes in landscape-scale soil OC storage in mangrove encroachment on saltmarsh habitats is potentially misleading. Further research is required to identify and quantify the specific environmental characteristics that control soil OC storage within wetlands experiencing mangrove encroachment.
These results are shown, to some extent, on a global scale, where greater than 60% of studies that examined soil OC/OM storage metrics within co-located tidal marshes and mangroves along encroachment fronts demonstrated equal soil OC/OM storage between mangroves and marshes (Table 3). Despite these results, the idea persists within the literature that mangrove OC storage surpasses saltmarsh OC storage (Table 4); multiple factors have been suggested as potential reasons for this increased mangrove OC storage relative to saltmarsh OC storage, including climate, environmental characteristics, differences between saltmarsh and mangrove physiology and productivity, and the duration associated with development of vegetation (Table 4). While these factors might be responsible for changes to the relationship between mangrove and saltmarsh soil OC densities in the future, it is critical to understand that within the timeline associated with these studies, there is little evidence for a difference between mangrove and saltmarsh soil OC storage.
On a regional scale, our data indicates that the interior barrier island wetlands within Apalachicola Bay are experiencing vegetation shifts, including both mangrove encroachment and low saltmarsh occupying previously high saltmarsh zones. “Recent” shifts, within the past 60–95 years, between high and low marsh species at all three sites indicate that these locations are experiencing changes associated with relative sea level rise, specifically increased inundation or the isolation of patches of low marsh species (S. alterniflora).
Data Availability Statement
The raw data supporting the conclusions of this article are available in the Supplementary Material.
Author Contributions
HS: conceptualization, methodology, investigation, formal analysis, writing – original draft, writing – review and editing, and visualization. JB: conceptualization, methodology, investigation, writing – review and editing, visualization, supervision, resources, and project administration. KE: methodology, investigation, writing – review and editing, and visualization. PA: investigation and writing – review and editing. TB: conceptualization, resources, and writing – review and editing. All authors contributed to the article and approved the submitted version.
Funding
Funding for HS was provided by a post-doctoral fellowship for the Coastal and Marine Laboratory supported by the Office of the Vice President for Research at Florida State University. JB and KE were supported by the Triumph Gulf Coast Inc., Project #69: Apalachicola Bay System Initiative. TB was in part supported by the Jon and Beverly Thompson Endowed Chair of Geological Sciences at University of Florida.
Conflict of Interest
The authors declare that the research was conducted in the absence of any commercial or financial relationships that could be construed as a potential conflict of interest.
Publisher’s Note
All claims expressed in this article are solely those of the authors and do not necessarily represent those of their affiliated organizations, or those of the publisher, the editors and the reviewers. Any product that may be evaluated in this article, or claim that may be made by its manufacturer, is not guaranteed or endorsed by the publisher.
Acknowledgments
We wish to thank Sydney Rodetsky, Kamri Colvin-Reece and Katarina Hennessy for assistance in the lab and field. We are grateful to the Apalachicola National Estuarine Research Reserve and The Nature Conservancy for facilitating access to the research sites.
Supplementary Material
The Supplementary Material for this article can be found online at: https://www.frontiersin.org/articles/10.3389/ffgc.2022.852910/full#supplementary-material
References
Adgie, T. E., and Chapman, S. K. (2021). Salt Marsh Plant Community Structure Influences Success of Avicennia germinans During Poleward Encroachment. Wetlands 41, 1–12. doi: 10.1007/s13157-021-01463-0
Alongi, D. M. (2020). Carbon Balance in Salt Marsh and Mangrove Ecosystems: a Global Synthesis. J. Mar. Sci. Eng. 8:767. doi: 10.3390/jmse8100767
Asanopoulos, C. H., Baldock, J. A., MacDonald, L. M., and Cavagnaro, T. R. (2021). Quantifying blue carbon and nitrogen stocks in surface soils of temperate coastal wetlands. Soil Res. 59, 619–629. doi: 10.1071/SR20040
Barreto, C. R., Morrissey, E. M., Wykoff, D. D., and Chapman, S. K. (2018). Co-occurring Mangroves and Salt Marshes Differ in Microbial Community Composition. Wetlands 38, 497–508. doi: 10.1007/s13157-018-0994-9
Bianchi, T. S., Allison, M. A., Zhao, J., Li, X., Comeaux, R. S., and Feagin, R. A. (2013). Historical reconstruction of mangrove expansion in the Gulf of Mexico: linking climate change with carbon sequestration in coastal wetlands. Estuar. Coast. Shelf Sci. 119, 7–16. doi: 10.1016/J.ECSS.2012.12.007
Breithaupt, J. L., Smoak, J. M., Bianchi, T. S., Vaughn, D. R., Sanders, C. J., Radabaugh, K. R., et al. (2020). Increasing rates of carbon burial in southwest Florida coastal wetlands. J. Geophys. Res. 125:e2019JG005349.
Breithaupt, J. L., Smoak, J. M., Rivera-Monroy, V. H., Castañeda-Moya, E., Moyer, R. P., Simard, M., et al. (2017). Partitioning the relative contributions of organic matter and mineral sediment to accretion rates in carbonate platform mangrove soils. Mar. Geol. 390, 170–180.
Breithaupt, J. L., Smoak, J. M., Smith, T. J., Sanders, C. J., and Hoare, A. (2012). Organic carbon burial rates in mangrove sediments: strengthening the global budget. Glob. Biogeochem. Cycles 26, 1–11. doi: 10.1029/2012GB004375
Cavanaugh, K. C., Kellner, J. R., Forde, A. J., Gruner, D. S., Parker, J. D., Rodriguez, W., et al. (2014). Poleward expansion of mangroves is a threshold response to decreased frequency of extreme cold events. Proc. Natl. Acad. Sci. 111, 723–727. doi: 10.1073/pnas.1315800111
Chanton, J., and Lewis, F. G. (2002). Examination of coupling between primary and secondary production in a river-dominated estuary: apalachicola Bay. Florida, USA. Limnol. Oceanogr. 47, 683–697.
Chapman, S. K., Feller, I. C., Canas, G., Hayes, M. A., Dix, N., Hester, M., et al. (2021). Mangrove growth response to experimental warming is greatest near the range limit in northeast Florida. Ecology 102:e03320. doi: 10.1002/ecy.3320
Charles, S. P., Kominoski, J. S., Armitage, A. R., Guo, H., Weaver, C. A., and Pennings, S. C. (2020). Quantifying how changing mangrove cover affects ecosystem carbon storage in coastal wetlands. Ecology 101:e02916. doi: 10.1002/ecy.2916
Chmura, G. L., Anisfeld, S. C., Cahoon, D. R., and Lynch, J. C. (2003). Global carbon sequestration in tidal, saline wetland soils. Glob. Biogeochem. Cycles 17, 1–11. doi: 10.1029/2002GB001917
Coldren, G. A., Langley, J. A., Feller, I. C., and Chapman, S. K. (2019). Warming accelerates mangrove expansion and surface elevation gain in a subtropical wetland. J. Ecol. 107, 79–90.
Comeaux, R. S., Allison, M. A., and Bianchi, T. S. (2012). Mangrove expansion in the Gulf of Mexico with climate change: implications for wetland health and resistance to rising sea levels. Estuar., Coast. Shelf Sci. 96, 81–95. doi: 10.1016/j.ecss.2011.10.003
Cui, L., Sun, H., Du, X., Feng, W., Wang, Y., Zhang, J., et al. (2021). Dynamics of labile soil organic carbon during the development of mangrove and salt marsh ecosystems. Ecol. Indicat. 129:107875.
Dontis, E. E., Radabaugh, K. R., Chappel, A. R., Russo, C. E., and Moyer, R. P. (2020). Carbon Storage Increases with Site Age as Created Salt Marshes Transition to Mangrove Forests in Tampa Bay. Florida (USA). Estuar. Coasts 43, 1470–1488. doi: 10.1007/s12237-020-00733-0
Doughty, C. L., Langley, J. A., Walker, W. S., Feller, I. C., Schaub, R., and Chapman, S. K. (2016). Mangrove Range Expansion Rapidly Increases Coastal Wetland Carbon Storage. Estuar. Coasts 39, 385–396. doi: 10.1007/s12237-015-9993-8
Ember, L. M., Williams, D. F., and Morris, J. T. (1987). Processes that influence carbon isotope variations in salt marsh sediments. Mar. Ecol. Progr. Ser. 36, 33–42.
Geldenhuys, C., Cotiyane, P., and Rajkaran, A. (2016). Understanding the creek dynamics and environmental characteristics that determine the distribution of mangrove and salt marsh communities at Nahoon Estuary. South Afr. J. Bot. 107, 137–147.
Geoghegan, E. K., Langley, J. A., and Chapman, S. K. (2021). A comparison of mangrove and marsh influences on soil respiration rates: a mesocosm study. Estuar. Coast. Shelf Sci. 248:106877.
Giri, C., and Long, J. (2016). Is the geographic range of mangrove forests in the conterminous United States really expanding? Sensors 16:2010. doi: 10.3390/s16122010
Grindrod, J., Moss, P., and Kaars, S., and van der. (1999). Late Quaternary cycles of mangrove development and decline on the north Australian continental shelf. J. Quater. Sci. 14, 465–470.
Hamilton, S. E., and Casey, D. (2016). Creation of a high spatio-temporal resolution global database of continuous mangrove forest cover for the 21st century (CGMFC-21). Glob. Ecol. Biogeogr. 25, 729–738.
Harris, D., Horwáth, W. R., and van Kessel, C. (2001). Acid fumigation of soils to remove bonates prior to total organic carbon or CARBON-13 isotopic analysis. Soil Sci. Soc. Am. J. 65, 1853–1866. doi: 10.2136/sssaj2001.1853
Hashimoto, T. R., Saintilan, N., and Haberle, S. G. (2006). Mid-Holocene development of mangrove communities featuring Rhizophoraceae and geomorphic change in the Richmond River Estuary. N. South Wales, Aus. Geogr. Res. 44, 63–76.
Henry, K. M., and Twilley, R. R. (2013). Soil Development in a Coastal Louisiana Wetland during a Climate-Induced Vegetation Shift from Salt Marsh to Mangrove. J. Coast. Res. 292, 1273–1283. doi: 10.2112/JCOASTRES-D-12-00184.1
Howe, A. J., Rodríguez, J. F., and Saco, P. M. (2009). Surface evolution and carbon sequestration in disturbed and undisturbed wetland soils of the Hunter estuary, southeast Australia. Estuar. Coast. Shelf Sci. 84, 75–83.
Kelleway, J. J., Cavanaugh, K., Rogers, K., Feller, I. C., Ens, E., Doughty, C., et al. (2017). Review of the ecosystem service implications of mangrove encroachment into salt marshes. Glob. Change Biol. 23, 3967–3983. doi: 10.1111/gcb.13727
Kelleway, J. J., Saintilan, N., Macreadie, P. I., Skilbeck, C. G., Zawadzki, A., and Ralph, P. J. (2016). Seventy years of continuous encroachment substantially increases “blue carbon” capacity as mangroves replace intertidal salt marshes. Glob. Change Biol. 22, 1097–1109. doi: 10.1111/gcb.13158
Koch, G. W., and Mooney, H. A. (1996). Response of terrestrial ecosystems to elevated CO2: A synthesis and summary. In Carbon Dioxide and Terrestrial Ecosystems. Eds. G W Koch and H A Mooney. pp 415–428. San Diego, USA: Academic Press
Lamb, A. L., Wilson, G. P., and Leng, M. J. (2006). A review of coastal palaeoclimate and relative sea-level reconstructions using δ13C and C/N ratios in organic material. Earth-Sci. Rev. 75, 29–57.
Livesley, S. J., and Andrusiak, S. M. (2012). Temperate mangrove and salt marsh sediments are a small methane and nitrous oxide source but important carbon store. Estuar. Coast. Shelf Sci. 97, 19–27. doi: 10.1016/j.ecss.2011.11.002
Lunstrum, A., and Chen, L. (2014). Soil carbon stocks and accumulation in young mangrove forests. Soil Biol. Biochem. 75, 223–232. doi: 10.1016/j.soilbio.2014.04.008
Macreadie, P. I., Anton, A., Raven, J. A., Beaumont, N., Connolly, R. M., Friess, D. A., et al. (2019). The future of Blue Carbon science. Nat. Commun. 10, 1–13.
Macy, A., Osland, M. J., Cherry, J. A., and Cebrian, J. (2021). Effects of chronic and acute stressors on transplanted black mangrove (Avicennia germinans) seedlings along an eroding Louisiana shoreline. Restor. Ecol. 29:e13373.
McClenachan, G., Witt, M., and Walters, L. J. (2021). Replacement of oyster reefs by mangroves: unexpected climate-driven ecosystem shifts. Glob. Change Biol. 27, 1226–1238. doi: 10.1111/gcb.15494
Mckee, K. L., and Rooth, J. E. (2008). Where temperate meets tropical: multi-factorial effects of elevated CO2, nitrogen enrichment, and competition on a mangrove-salt marsh community. Glob. Change Biol. 14, 971–984. doi: 10.1111/j.1365-2486.2008.01547.x
Mcleod, E., Chmura, G. L., Bouillon, S., Salm, R., Björk, M., Duarte, C. M., et al. (2011). A blueprint for blue carbon: toward an improved understanding of the role of vegetated coastal habitats in sequestering CO2. Front. Ecol. Environ. 9:552–560. doi: 10.1890/110004
McMillan, C., and Sherrod, C. L. (1986). The Chilling Tolerance of Black Mangrove, Avicennia Germinans, from the Gulf of Mexico coast of Texas, Louisiana and Florida.
Mcowen, C. J., Weatherdon, L. v, van Bochove, J.-W., Sullivan, E., Blyth, S., Zockler, C., et al. (2017). A global map of saltmarshes. Biodivers. Data J. 5:e11764. doi: 10.3897/BDJ.5.e11764
Monaghan, A. J., Morin, C. W., Steinhoff, D. F., Wilhelmi, O., Hayden, M., Quattrochi, D. A., et al. (2016). On the seasonal occurrence and abundance of the Zika virus vector mosquito Aedes aegypti in the contiguous United States. PLoS Curr. 8:currents.outbreaks.50dfc7f46798675fc63e7d7da563da76. doi: 10.1371/currents.outbreaks.50dfc7f46798675fc63e7d7da563da76
Osland, M. J., Enwright, N., Day, R. H., and Doyle, T. W. (2013). Winter climate change and coastal wetland foundation species: salt marshes vs. mangrove forests in the southeastern United States. Glob. Change Biol. 19, 1482–1494.
Osland, M. J., Spivak, A. C., Nestlerode, J. A., Lessmann, J. M., Almario, A. E., Heitmuller, P. T., et al. (2012). Ecosystem Development After Mangrove Wetland Creation: plant-Soil Change Across a 20-Year Chronosequence. Ecosystems 15, 848–866. doi: 10.1007/s10021-012-9551-1
Osland, M. J., Stevens, P. W., Lamont, M. M., Brusca, R. C., Hart, K. M., Waddle, J. H., et al. (2021). Tropicalization of temperate ecosystems in North America: the northward range expansion of tropical organisms in response to warming winter temperatures. Glob. Change Biol. 27, 3009–3034.
Ouyang, X., and Lee, S. Y. (2014). Updated estimates of carbon accumulation rates in coastal marsh sediments. Biogeosciences 11, 5057–5071. doi: 10.5194/bg-11-5057-2014
Ouyang, X., and Lee, S. Y. (2020). Improved estimates on global carbon stock and carbon pools in tidal wetlands. Nat. Commun. 11, 1–7. doi: 10.1038/s41467-019-14120-2
Perry, C. L., and Mendelssohn, I. A. (2009). Ecosystem effects of expanding populations of Avicennia germinans in a Louisiana salt marsh. Wetlands 29, 396–406. doi: 10.1672/08-100.1
Peterson, B. J., and Fry, B. (1987). Stable isotopes in ecosystem studies. Ann. Rev. Ecol. Syst. 18, 293–320.
Radabaugh, K. R., Moyer, R. P., Chappel, A. R., Powell, C. E., Bociu, I., Clark, B. C., et al. (2018). Coastal Blue Carbon Assessment of Mangroves, Salt Marshes, and Salt Barrens in Tampa Bay, Florida, USA. Estuar. Coast. 41, 1496–1510. doi: 10.1007/s12237-017-0362-7
Rovai, A. S., Twilley, R. R., Castañeda-Moya, E., Riul, P., Cifuentes-Jara, M., Manrow-Villalobos, M., et al. (2018). Global controls on carbon storage in mangrove soils. Nat. Clim. Change 8 6, 534–538. doi: 10.1038/s41558-018-0162-5
Saintilan, N., Wilson, N. C., Rogers, K., Rajkaran, A., and Krauss, K. W. (2014). Mangrove expansion and salt marsh decline at mangrove poleward limits. Glob. Change Biol. 20, 147–157. doi: 10.1111/gcb.12341
Silberhorn, G. M. (1999). Common Plants of the Mid-Atlantic Coast: a Field Guide. Baltimore: JHU Press.
Simpson, L. T., Cherry, J. A., Smith, R. S., and Feller, I. C. (2021). Mangrove encroachment alters decomposition rate in saltmarsh through changes in litter quality. Ecosystems 24, 840–854.
Simpson, L. T., Stein, C. M., Osborne, T. Z., and Feller, I. C. (2019). Mangroves dramatically increase carbon storage after 3 years of encroachment. Hydrobiologia 834, 13–26. doi: 10.1007/s10750-019-3905-z
Smith, J. A. M. (2013). The Role of Phragmites australis in Mediating Inland Salt Marsh Migration in a Mid-Atlantic Estuary. PLoS One 8:e65091. doi: 10.1371/journal.pone.0065091
Snyder, C. M., Feher, L. C., Osland, M. J., Miller, C. J., Hughes, A. R., and Cummins, K. L. (2021). The Distribution and Structure of Mangroves (Avicennia germinans and Rhizophora mangle) Near a Rapidly Changing Range Limit in the Northeastern Gulf of Mexico. Estuar. Coasts 45, 181–195.
Soares, M. L. G., Estrada, G. C. D., Fernandez, V., and Tognella, M. M. P. (2012). Southern limit of the Western South Atlantic mangroves: assessment of the potential effects of global warming from a biogeographical perspective. Estuar. Coast. Shelf Sci. 101, 44–53.
Steinmuller, H. E., Foster, T. E., Boudreau, P., Ross Hinkle, C., and Chambers, L. G. (2020). Tipping Points in the Mangrove March: characterization of Biogeochemical Cycling Along the Mangrove–Salt Marsh Ecotone. Ecosystems 23, 417–434. doi: 10.1007/s10021-019-00411-8
Sturchio, M. A., Chieppa, J., Chapman, S. K., Canas, G., and Aspinwall, M. J. (2021). Temperature acclimation of leaf respiration differs between marsh and mangrove vegetation in a coastal wetland ecotone. Glob. Change Biol. 28, 612–629. doi: 10.1111/gcb.15938
van Ardenne, L. B., Jolicouer, S., Bérubé, D., Burdick, D., and Chmura, G. L. (2018). The importance of geomorphic context for estimating the carbon stock of salt marshes. Geoderma 330, 264–275. doi: 10.1016/j.dib.2018.07.037
Vaughn, D. R., Bianchi, T. S., Shields, M. R., Kenney, W. F., and Osborne, T. Z. (2020). Increased Organic Carbon Burial in Northern Florida Mangrove-Salt Marsh Transition Zones. Glob. Biogeochem. Cycles 34:e2019GB006334. doi: 10.1029/2019GB006334
Vitousek, P. M., Mooney, H. A., Lubchenco, J., and Melillo, J. M. (1997). Human Domination of Earth’s Ecosystems. Science 277, 494–499.
Keywords: mangroves, tidal marsh, mangrove range expansion, blue carbon, Rhizophora mangle (red mangrove), Avicennia germinans (black Mangrove), Spartina alterniflora, Juncus roemerianus
Citation: Steinmuller HE, Breithaupt JL, Engelbert KM, Assavapanuvat P and Bianchi TS (2022) Coastal Wetland Soil Carbon Storage at Mangrove Range Limits in Apalachicola Bay, FL: Observations and Expectations. Front. For. Glob. Change 5:852910. doi: 10.3389/ffgc.2022.852910
Received: 11 January 2022; Accepted: 17 May 2022;
Published: 01 July 2022.
Edited by:
Raymond D. Ward, University of Brighton, United KingdomReviewed by:
Miguel Villoslada, Estonian University of Life Sciences, EstoniaScott Jones, United States Geological Survey (USGS), United States
Copyright © 2022 Steinmuller, Breithaupt, Engelbert, Assavapanuvat and Bianchi. This is an open-access article distributed under the terms of the Creative Commons Attribution License (CC BY). The use, distribution or reproduction in other forums is permitted, provided the original author(s) and the copyright owner(s) are credited and that the original publication in this journal is cited, in accordance with accepted academic practice. No use, distribution or reproduction is permitted which does not comply with these terms.
*Correspondence: Joshua L. Breithaupt, amJyZWl0aGF1cHRAZnN1LmVkdQ==