- 1Faculty of Forestry and Environmental Management, University of New Brunswick, Fredericton, NB, Canada
- 2Department of Geography, Memorial University of Newfoundland, St. John’s, NL, Canada
Climate models predict an increase in the frequency and intensity of drought events in the Acadian Forest Region, with higher risk of tree growth decline and mortality. To accurately predict future species response, we need to better compare drought-coping traits between Acadian tree species, especially at early life stages as young trees tend to display increased sensitivity to small environmental changes than mature trees. Here, we compared the seasonal growth phenology and biomass allocation in seedlings of 13 Acadian tree species in a controlled environment to assess their ability to predict species drought tolerance rankings. We focused on two traits associated with drought tolerance, namely biomass allocation to root systems, which affects water uptake, and phenology of seasonal growth, where earlier growth can avoid the peak drought period in late summer. We find an earlier onset of height growth in drought-tolerant species (P < 0.05), supporting the late-summer drought avoidance hypothesis. The observed biomass allocation patterns did not support the hypothesis of a higher allocation to roots with drought tolerance. In fact, we report an initially higher shoot-to-root ratio in drought tolerant species (P < 0.05), which becomes non-significant as the season progresses. Our results highlight the complexity of drought response strategies, as the developmental traits reported here only account for a fraction of each species overall drought tolerance. Yet, the important differences in growth phenology observed here between species, and their linkage with drought tolerance indices, could help predict species response to future drought regime.
Introduction
Climate models predict an increase in frequency and intensity of drought events throughout large tracts of land across the globe, including North America (Dai, 2011; Xu et al., 2019). This climate shift could have severe impact on forest trees, as exemplified by the recent drought-driven widespread tree mortality reported in the southern Canadian boreal forest (Michaelian et al., 2011). Trees at the seedling stage are more vulnerable to differences in temperature and precipitation than mature trees, and demonstrate increased sensitivity to small environmental changes (Gerhardt, 1996; Bell et al., 2014; Mathys et al., 2018). Additionally, the limited root systems of seedlings mean they do not have access to water stores located in lower soil layers (Engelbrecht et al., 2005; Niinemets, 2010) compounding their increased sensitivity to drought events. Therefore, species-specific variation in seedling establishment patterns and survival rates may be a crucial predictor of species responses to climate change compared to mature trees, especially those at their distributional limits (Mathys et al., 2018).
Physiological and environmental conditions have created varying levels of tolerance to drought in tree species, where some species display increased tolerance to drought events relative to others (Niinemets and Valladares, 2006). Tree species drought-coping strategies are often categorized as drought avoidance and drought resistance (Kramer and Boyer, 1995; Polle et al., 2019). Drought avoiding species have adopted mechanisms to reduce water loss and maximize their uptake of water, including completing their seasonal growth prior to regular seasonal drought, increased leaf thickness, and deep root systems (Kramer and Boyer, 1995; Nguyen et al., 2017; Polle et al., 2019). Drought-resistant species like oaks have maximized traits like hydraulic resistance to embolisms that insure maintenance of superior growth during drought, providing a competitive advantage over more conservative species (McDowell et al., 2008). Knowledge of each species drought-coping traits, including their co-variations and trade-offs, are required to enable accurate prediction of their functional responses to drought events and competitive fitness under a warmer, drier climate.
Under water stress, plants often alter their morphology to maximize their belowground surface area to maximize water uptake and conservation (Brouwer, 1963; Markesteijn and Poorter, 2009). The most substantial morphological difference linked with drought tolerance is root morphology (Møller and Jennions, 2002; Niinemets and Valladares, 2006; Markesteijn and Poorter, 2009). There is a general understanding that the allocation of carbon to root production demonstrates an initial flush before aboveground production takes place, then peaks in late spring into the summer (Burke and Raynal, 1994; Steinaker et al., 2010; McCormack et al., 2014). Tree species that are adapted to drought generally have deeper root systems and increased root-to-shoot ratios than those with lower drought tolerance (Kozlowski and Pallardy, 2002; Hartmann, 2011), and this growth response is influenced by the severity of stress (Poorter et al., 2012; Brunner et al., 2015). Drought tolerant species often reduce their leaf biomass to minimize water loss, and increase carbon allocation to their root systems to allow for additional water acquisition (Niinemets and Valladares, 2006; Markesteijn and Poorter, 2009). While there is increasing evidence that the growth and structure of root systems are impacted by drought (Kozlowski and Pallardy, 2002), there is little information that allows comparison of the magnitude of their response to drought between species, as well as the role they may play in the overall species drought tolerance (McDowell et al., 2008; Hamanishi and Campbell, 2011; Brunner et al., 2015).
Climate model predictions suggest an earlier start to the spring growing season, leading to increasing drought during the later part of summer, which could cause substantial declines in forest productivity, as already observed in some North American forests (Richardson et al., 2010; Buermann et al., 2018). Species that are actively growing late in the growing season may thus be more vulnerable to these late summer droughts (Houle et al., 2012; Buermann et al., 2013, 2018). Similarly, recent studies have reported that sensitivity of tree growth to drought varied significantly over the growing season, depending on the phenology of growth and its synchrony with drought (Foster et al., 2014; D’Orangeville et al., 2018; Kannenberg et al., 2019b; Vanhellemont et al., 2019). The timing of drought may even have a greater impact on tree functionality than the drought intensity (D’Orangeville et al., 2018; Forner et al., 2018). Most of the current knowledge on species phenology is related to differences between wood structure types in deciduous species, where ring-porous species complete most of their annual growth earlier in the growing season and in a shorter amount of time relative to diffuse-porous species (Waring, 1951; Ladefoged, 1952; Zimmermann and Brown, 1971; Panchen et al., 2014). Historically, evidence of the onset and duration of shoot and diameter growth in coniferous species has been unclear (Ladefoged, 1952; Hunter and Lechowicz, 1992; Zweifel et al., 2006), however, recent studies have found that while the onset and duration of wood growth in conifers are not correlated (Cuny et al., 2014), the timing of other phenological patterns and weather signals display some control over xylem phenology (Rossi et al., 2013, 2016). Coniferous species, therefore, have high plasticity and are able to adapt their phenological patterns to local conditions (Rossi et al., 2013, 2016). Therefore, a better understanding of species growth phenology is needed to predict impacts of future drought events.
Assessing drought stress responses in mature trees is often done retroactively through dendrochronological approaches, while seedling performance are often evaluated in controlled environments, such as common gardens (McDowell et al., 2013; Csilléry et al., 2020). Common garden experiments have a long history of use in forest genetics to assess local adaptation of traits, as they provide a unique ability to control for phenotypic plasticity that cannot be controlled in situ (Sork et al., 2013; de Villemereuil et al., 2016; Housset et al., 2018). In these experiments, traits are often used as a proxy for other fundamental characteristics, and help inform species vulnerabilities, conservation practices, and adaptive management approaches (McKay et al., 2001; de Villemereuil et al., 2016; Housset et al., 2018). Drought stress affects many functional traits in seedlings, including phenology patterns (Nguyen et al., 2017; D’Orangeville et al., 2018), wood anatomical traits (Crous et al., 2012; D’Orangeville et al., 2021), and biomass allocation (Møller and Jennions, 2002; Niinemets and Valladares, 2006; Markesteijn and Poorter, 2009), however, it is still unclear which traits are most important for understanding and predicting species drought tolerances (Moran et al., 2017).
Species growing in the Acadian forest region are likely more susceptible to future changes in climate and environmental conditions than those found in other forest regions. The Acadian forest region is part of the ecological transition zone between northern boreal coniferous and southern temperate deciduous forests (Loo and Ives, 2003; Taylor et al., 2017), composed of species and communities living at the extreme edges of their range (Fisichelli et al., 2014; Taylor et al., 2017). As a result of ongoing rapid climate change, there will likely be a shift in the composition of the Acadian forest region toward more temperate species as conditions become less favourable for boreal conifers, reducing their ability to compete with temperate species (Bourque and Hassan, 2008; Taylor et al., 2017). In addition to this compositional shift, climate change throughout the 21st century will likely create a lagged growth effect; although temperate species are more competitive, still living mature boreal conifers block the ability of temperate species to colonize newly favorable environments, resulting in decreased overall growth of the Acadian forest (Zhu et al., 2012; Corlett and Westcott, 2013; Taylor et al., 2017). Previous studies on tree growth phenology and biomass allocation patterns are often limited to a small number of species mostly native to Europe (Panchen et al., 2014; Piper and Paula, 2020). Adequate knowledge comparing the drought-coping traits of the main Acadian tree species is critical to predict the species-specific impact of drought events in that ecosystem. In this study, we monitored the development of 13 Acadian tree species with different drought tolerances over one growing season to determine if their drought tolerance could be predicted from phenological and morphological growth traits. We focused on two traits associated with drought tolerance, namely biomass allocation and seasonal growth phenology, and tested the hypotheses that more drought-tolerant species would (i) complete their growth earlier in the season while conditions are more favorable, and (ii) allocate a higher fraction of their new biomass to their root system as the season progresses and risk of water stress increases.
Materials and Methods
With the exception of Norway spruce, seeds of thirteen coniferous and deciduous species characteristic of the Acadian forest were collected from local populations in Atlantic Canada and provided by the National Tree Seed Center, the Canadian repository of tree seeds. While Norway spruce is not indigenous to the Acadian forest, this species is widely present in the region due to historical and current plantations. Most seed sources were in regions of New Brunswick, while eastern white pine and Norway spruce seeds were collected from Nova Scotia and Maine, respectively (Table 1). All seeds were collected from within the Acadian forest region, suggesting similar forest dynamics between source locations, and grown under controlled conditions for one to 2 years. A total of 403 trees were released from dormancy on June 7, 2019, transplanted into 7.6 liter pots with standard tree nursery growing medium and grown outside in full sun with regular watering until the final measurements were recorded on October 3, 2019. The temperature that summer (18.2°C over JJA) was representative of the long-term average (18.1°C over 1981–2010). Sixty-five of these seedlings were monitored weekly as part of our phenology measurements, and the remaining 338 seedlings were used to assess biomass accumulation patterns.
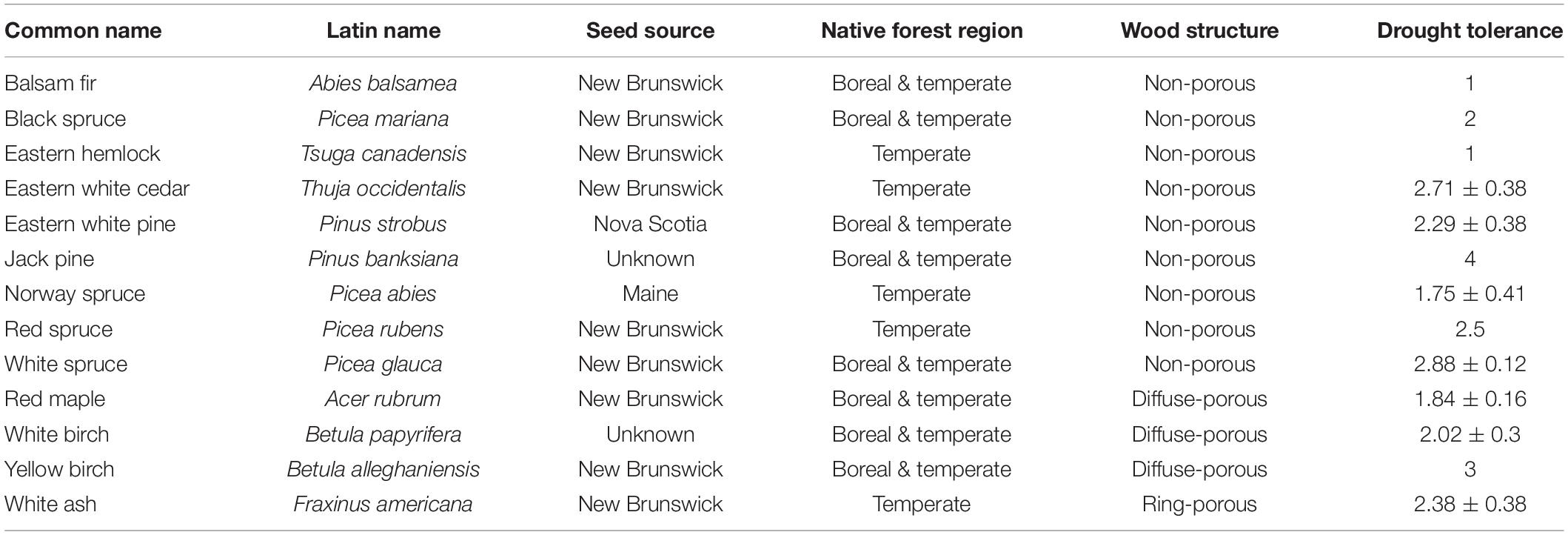
Table 1. Tree characteristics and seed provenance, including drought tolerance ranking from Niinemets and Valladares (2006) where 0 represents no tolerance and 5 represents maximal tolerance.
Height, diameter at root collar, and bud development stage were recorded for all individuals of each species when they were released from dormancy. Five seedlings of each species were randomly selected for repeated weekly measurements of height and root collar diameter.
Three additional seedlings of each species were randomly selected each week for destructive sampling and biomass measurements. These three seedlings were measured for height, root collar diameter, and bud development stage, and their oven-dried (70°C for 48 h) root, leaf, and aboveground wood biomass weight. The scaly foliage of eastern white cedar could not be weighed separately from wood.
Analysis of Data
Four individuals were excluded from the phenological analysis due to mortality or severe breakage during the experiment. We also excluded 12 individuals composed of balsam fir, white pine, jack pine, red maple, and red spruce species which displayed second flushes of growth as the data analysis was focused on a single flush of growth. Diameter growth was not determined for yellow birch as following the removal of trees experiencing mortality, only one sample tree of yellow birch remained.
A logistic growth model was fitted to the seasonal height and diameter growth of each tree separately:
where t is a given day of year during the growing season, G is the total height growth, Po is a scaling parameter for the Y axis, r is the growth rate, and ε is the residual error of the model. This approach is similar to that implemented by D’Orangeville et al. (2021), however, here the approach is applied to young trees to look at height as well as diameter growth, in a more controlled environment.
We derived three key variables associated with primary and secondary growth: the day of year (DOY) where each individual tree achieved 25, 50, and 75% of its cumulative annual growth of height and diameter. The period of rapid growth for each species was calculated from the difference between 25 and 75% DOY. Red maple and yellow birch were removed from diameter analysis because only two datapoints were available for red maple and one for yellow birch on a possibility of five.
Due to the increasing size and corresponding effort needed for biomass measurements, the number of individual samples per species was reduced from 3 to 2 from August 8, 2019 onward. The last two measurements of biomass data in September and October were excluded from the final analysis due to manipulation errors. Biomass ratios were then calculated for the foliage-to-belowground, wood-to-belowground, aboveground-to-belowground, and foliage-to-wood biomass allocation patterns of each species.
To test the hypothesis that measured traits reflected species drought tolerance, linear regression models were fitted to the resulting phenological indices as well as biomass values and ratios against species drought tolerance scores taken from Niinemets and Valladares (2006). Regression slope values and their significance were used to assess relationships between drought tolerance rankings and observed morphological and phenological species traits. All analyses were performed using R software (version 4.1.0, 2021).
Results
The seasonal growth models displayed a good overall fit for species height (normalized RMSE = 4.1%) and diameter growth (normalized RMSE = 5.4%), with the model demonstrating an insignificant fit (P > 0.05) for the height or diameter of less than 10% of sample trees. The onset of rapid height growth, defined here as the time when 25% of annual growth is completed, preceded the period of rapid diameter growth by 4–34 days across species (mean of 18 days; Figures 1A,B) apart from eastern hemlock, whose rapid diameter growth started 4 ± 3 days before rapid height growth.
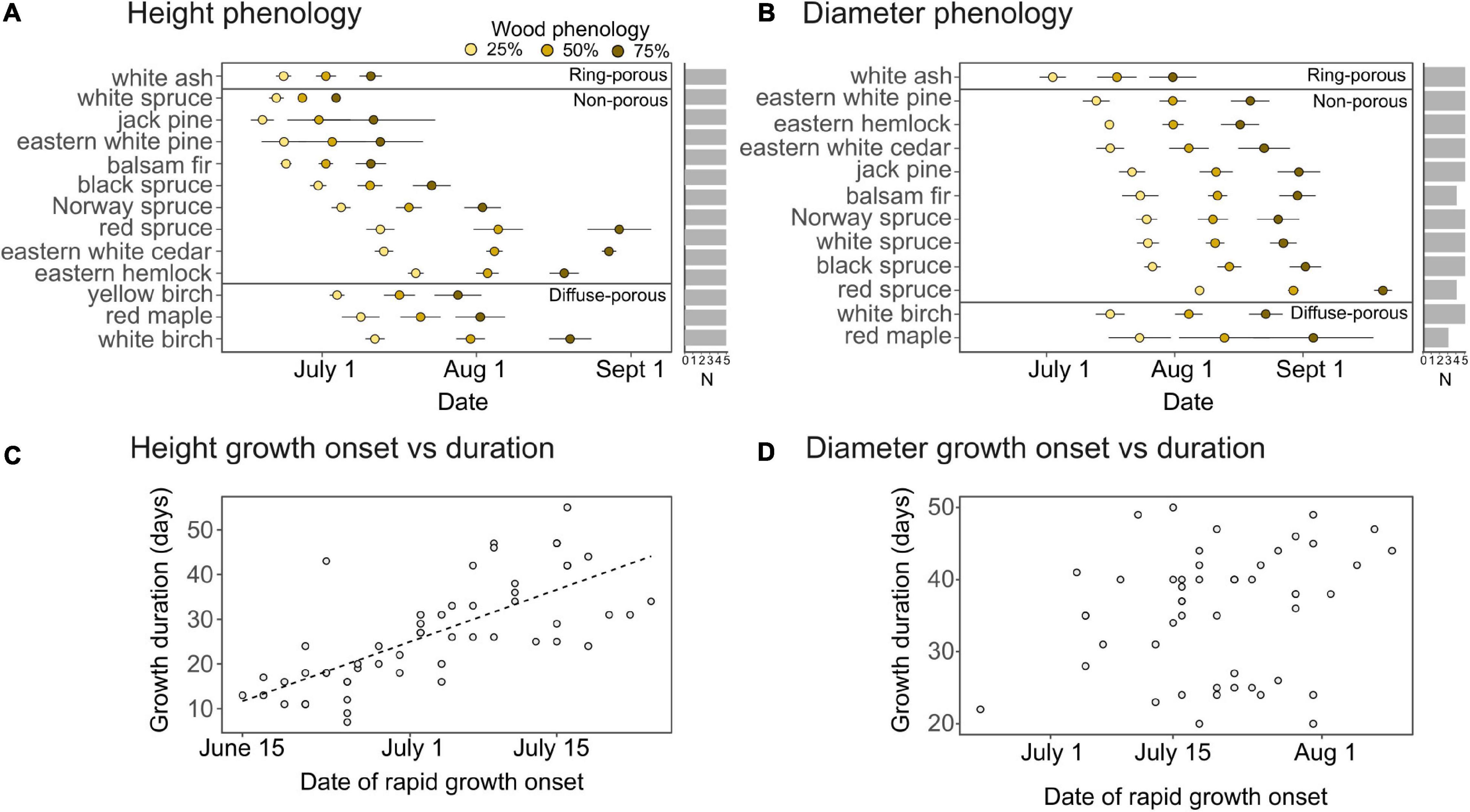
Figure 1. Average height and diameter growth phenology per species, expressed as the date when 25, 50, and 75% of annual height (A) and diameter (B) growth are achieved, as well as the relationship between growth duration and the onset of rapid height growth (C) and diameter growth (D). Duration is expressed as the number of days between 25 and 75% of growth. Error bars indicate standard error of the mean, while horizontal bars indicate sample replication per species. A dashed line indicates a significant (P < 0.001) relationship tested with a linear regression model.
While there was no clear trend of height and diameter growth phenology between coniferous and deciduous species (Figures 1A,B), within the conifers, temperate forest species displayed a later initiation of height growth which then extended further into the growing season. Among broadleaf species, the growth of ring-porous white ash was precocious relative to diffuse-porous species. White ash initiated its peak diameter growth period 21 ± 8 days earlier than white birch, and its peak height growth period at least 10 ± 3 days earlier than the three diffuse-porous species (Figures 1A,B). Among conifer species, the timing of rapid height growth onset was highly variable, ranging from June 17 ± 4 days for jack pine to July 18 ± 3 days for eastern hemlock (Figure 1A). With the exception of red spruce, which initiated rapid diameter growth in early August, the onset of the period of rapid diameter growth for the thirteen coniferous species occurred over a 2 week period in mid-July.
Onset and duration of height and diameter growth of seedlings varied according to wood structure types (ring-porous, diffuse porous, and non-porous). With the notable exception of eastern hemlock and eastern white cedar, coniferous species demonstrated the largest delay in diameter growth onset relative to height growth onset (Figures 1A,B). Ring-porous white ash had an earlier (by up to 19 days) and shorter (by 11 days on average) period of rapid height growth than the diffuse-porous species (Figures 1A,B). While the time at which ring-porous species and diffuse-porous species were actively growing was different, there was no obvious difference in the length of time between the onsets of height and diameter growth. Species initiating height growth later in the season, including white birch, eastern white cedar, and red spruce, tended to display lower growth rates (Supplementary Figure 1), leading to an extended height growth period (Figures 1A,C). This relationship between onset and duration of height growth was not significant for diameter growth (Figure 1D).
Biomass Allocation Patterns
With the exception of jack pine and white spruce, all species displayed an initial shoot-to-root ratio (SRR) of approximately 1:1, and reached an asymptote by the first week of August where the ratio remained the same or declined (Figure 2), suggesting more investment in belowground biomass later in the growing season. Throughout the growing season, the SRR remained lowest in balsam fir suggesting balsam fir allocates biomass to both above and belowground stores, while species with higher SRR, such as black spruce, demonstrate a strong preferential allocation pattern to aboveground biomass. Diffuse-porous species allocated more growth to aboveground biomass than ring-porous species, with SRR for ring-porous white ash consistently lower than in diffuse-porous species throughout the growing season. Both white and yellow birch consistently favoured aboveground biomass allocation during their growth (Figure 2). We did not report any differences in biomass allocation patterns between boreal and temperate species.
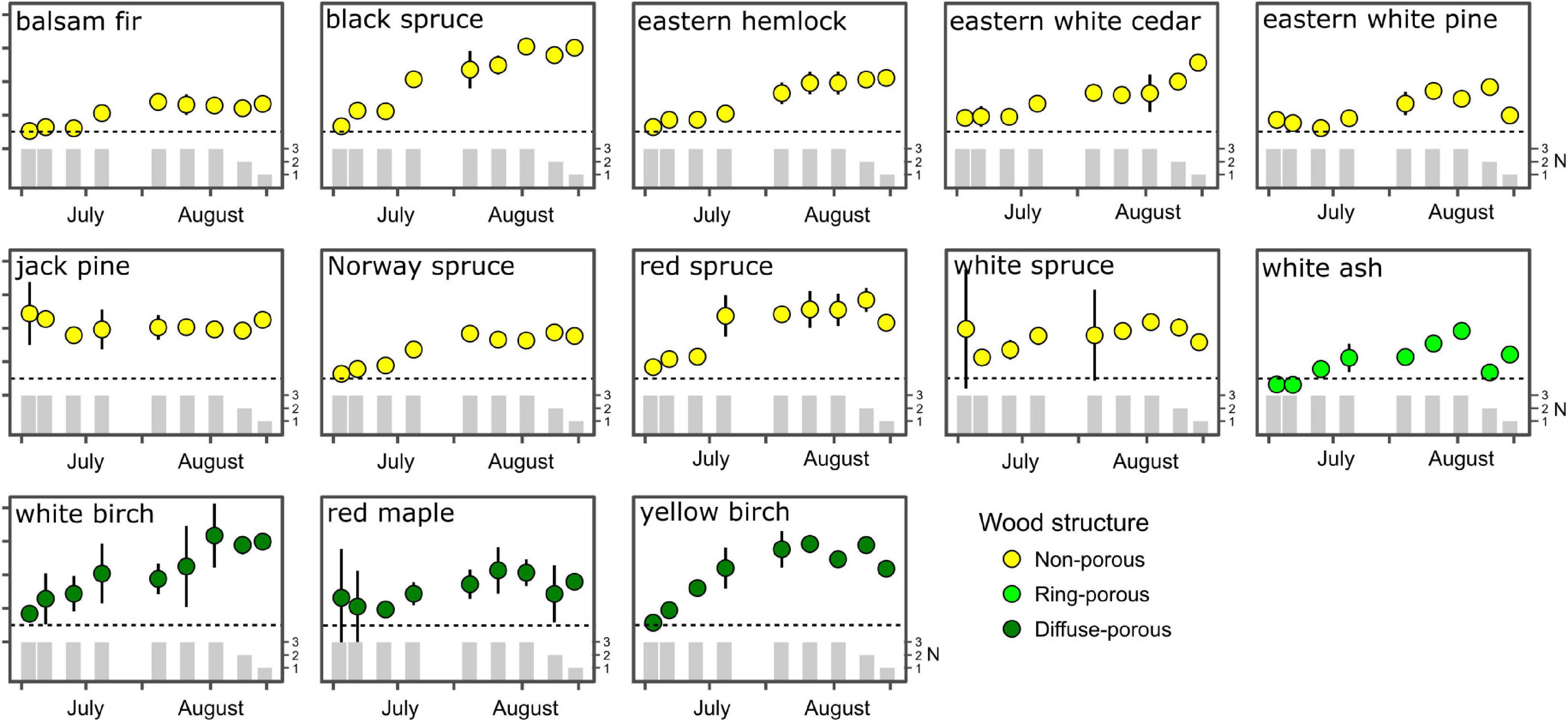
Figure 2. Seasonal variation in shoot-to-root ratio averaged per species. Error bars indicate standard error of the mean, while vertical bars indicate sample replication per observation.
Growth Trade-Offs With Drought Tolerance
In support with hypothesis 1, we find that drought tolerant species tend to initiate their period of rapid height growth earlier than drought intolerant species (P < 0.01; Figure 3A), this result remains significant (P < 0.01) without eastern white pine which has the highest drought tolerance. This trend weakens as the season progresses, with a near-significant relationship at 50% height growth (P = 0.06) and non-significant at 75% height growth (P > 0.1). Drought tolerance displayed no significant relationship with the phenology of diameter growth or the duration of growth (Figures 3B–D).
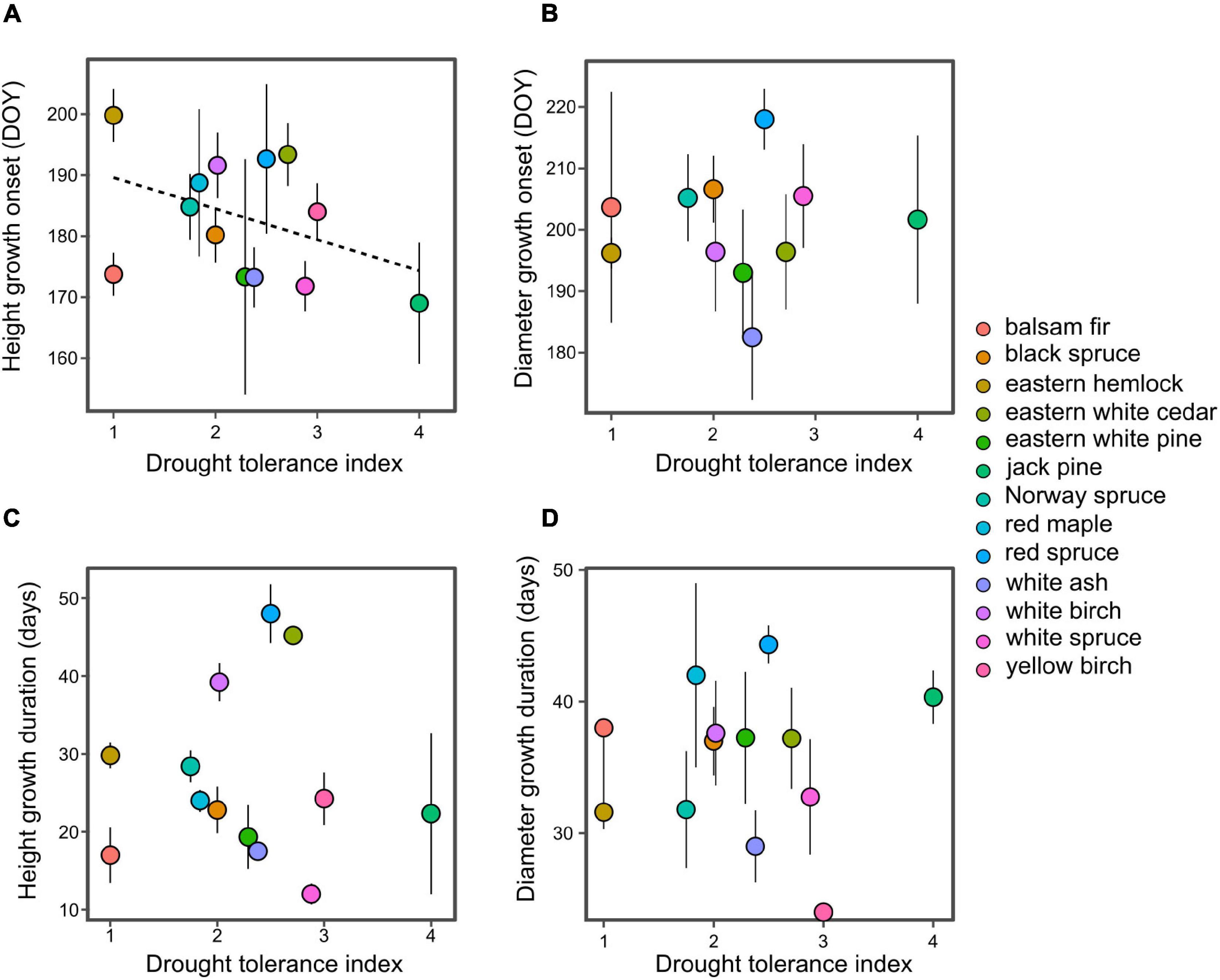
Figure 3. Relationship between species drought tolerance index and the onset of rapid height growth (A), rapid diameter growth (B), and the duration of height (C) and diameter growth (D). Onset represents the time when 25% of annual growth is reached, while duration represents the period between 25 and 75% of growth. Dashed lines indicate significant linear regression models at P < 0.01.
Contrary to our second hypothesis, drought tolerance was positively correlated with SRR during three out of four periods of the growing season (P < 0.05; Figure 4). A strong relationship was observed early in the season, which gradually declined as season progressed until no significant relationship was observed for the last period of growth in August. The gradual loss of significance was mostly due to the steady increase in SRR from drought intolerant species during the growing season, while SRR of drought tolerant species like jack pine remained stable, indicating even allocation of biomass to aboveground and belowground organs (Figure 4).
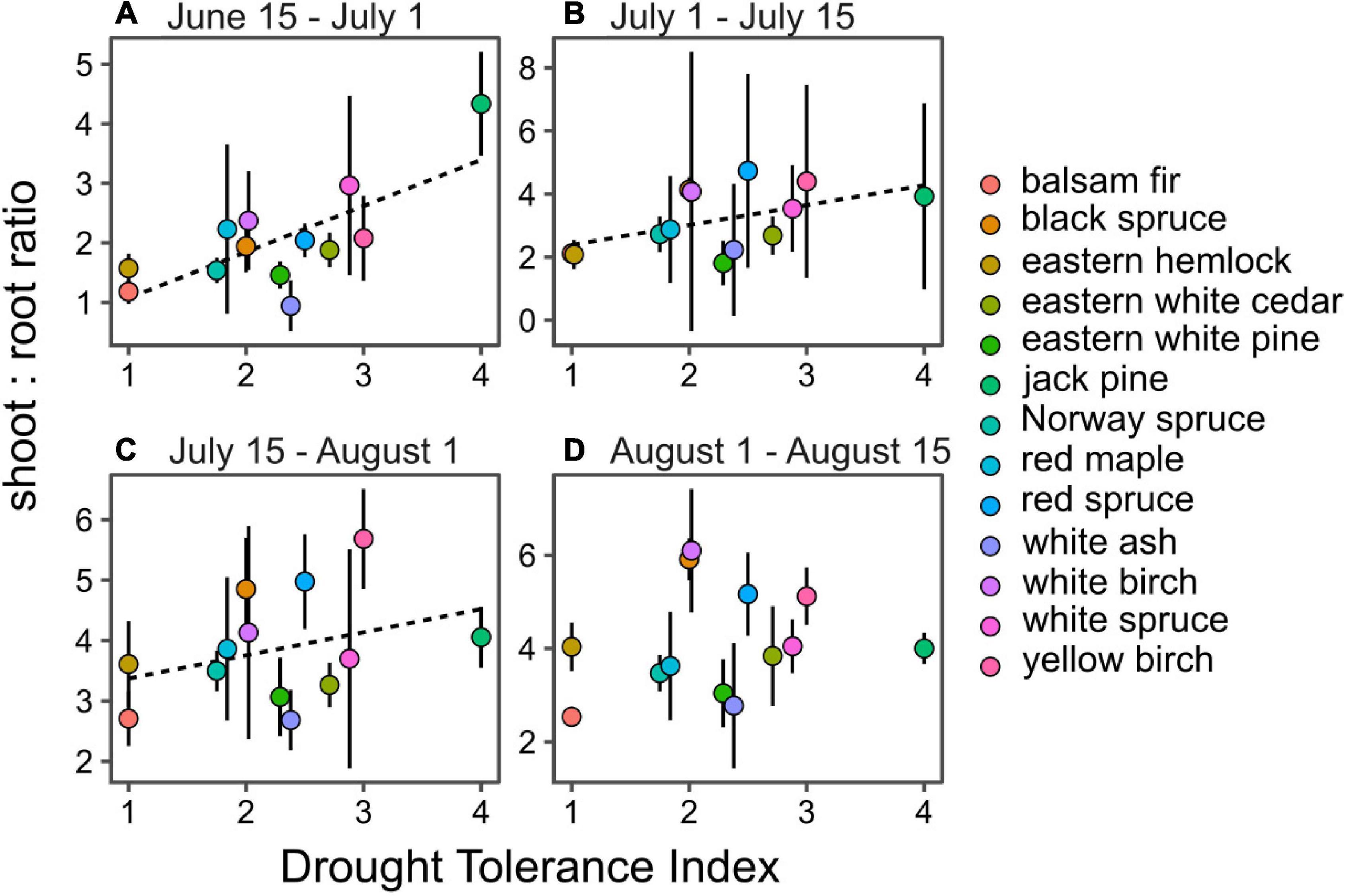
Figure 4. Seasonal correlation between species drought tolerance index and their shoot-to-root ratio from June 15 to July 1 (A), July 1 to July 15 (B), July 15 to August 1 (C) and August 1 to August 15 (D). Dashed lines indicate significant linear regression models at P < 0.05.
Discussion
The ability of tree seedlings to withstand drought conditions is dependent on the sum of their physiological, morphological, and phenological traits (Tyree et al., 2003; Poorter and Markesteijn, 2008). Though there are principles that apply to both gymnosperms and angiosperms, there are also significant fundamental differences in their physiology and morphology (Moran et al., 2017). The vessel elements in deciduous species make them less resistant to drought induced embolism than coniferous species, which only have tracheids, though deciduous species have much higher diversity in their anatomical responses to drought, allowing them to persist in harsh environments (Brodribb et al., 2012; Carnicer et al., 2013; Moran et al., 2017). Similarly, the differing climate and disturbance regimes between the northern, colder boreal forest and southern, warmer temperate forest create varying levels of drought tolerance (Bugmann, 1996; Carnicer et al., 2013), as those adapted to cooler environments are likely less capable of tolerating extreme heat, which was evidenced in the earlier onset of height growth in northern boreal forest conifers.
The extent of response to drought through biomass allocation is species specific (Zhang P. et al., 2020), and may also depend on individual drought coping strategies (Lloret et al., 2018; Zhang P. et al., 2020), where drought tolerant species demonstrate increased adaptation to drought through altered morphological patterns (Piper, 2011; Piper and Paula, 2020). Drought tolerant species, especially in drier forest regions, have been reported to display superior water uptake by increasing the biomass allocation to their root system, resulting in lower SRR (Poorter and Markesteijn, 2008; Wiley, 2020). Our results do not support these previous findings. Instead, we found that seedlings of Acadian tree species with higher drought tolerance maintained a steady, even allocation of growth between their roots and shoots throughout the season, while those with lower drought tolerance displayed a lower allocation to their root system. There is not yet a clear trend of SRR in relation to drought tolerance in seedlings (Sack et al., 2003; Poorter et al., 2012; Olmo et al., 2014; Puglielli et al., 2020); some tolerant species increase allocation to root systems, while others maintain more equal allocation patterns. Species additionally may be maladapted for drier conditions, or biomass allocation to root systems may not play a significant role in determining drought tolerance of seedlings in the Acadian forest. Maintaining equal allocation to roots throughout the season, as was found here, could be a conservative growth strategy by seedlings to ensure the maintenance of higher water uptake capacity.
The phenological traits of species are increasingly recognized to play an important role in their relative drought tolerance levels through the maximization or completion of annual growth prior to the regular drought season during late summer (Zhang P. et al., 2020; D’Orangeville et al., 2021). The timing of drought affects drought vulnerability in trees, since the process of cambial cell division at the heart of primary and secondary growth is highly sensitive to water availability (Gruber et al., 2010; Foster et al., 2014; Lempereur et al., 2015), though the productivity of coniferous forests may be less sensitive to changing phenology patterns than deciduous forests (Richardson et al., 2010; Carnicer et al., 2013). Drought impacts on the diameter growth of mature trees in temperate Eastern North America were reported to peak in June (D’Orangeville et al., 2018; Kannenberg et al., 2019a) coinciding with the period of maximal cambial activity (Rossi et al., 2006; Deslauriers et al., 2007). Shifts in phenological patterns have already been observed in many species (Alberto et al., 2013), and these adaptations can occur without the need for significant genetic evolution (Gienapp et al., 2008; Alberto et al., 2013). The significant observation of an earlier timing of height growth in seedlings with increasing drought tolerance provides additional evidence that height growth phenology could play an important role, along with many other physiological and morphological traits, in helping predict species drought tolerance. Impacts to height phenology patterns may additionally have increased importance in understanding vulnerabilities and survival of seedlings, given the role shoot extension plays in the competition for light resources. These findings are in line with previous works (Zapater et al., 2013; Vanhellemont et al., 2019; D’Orangeville et al., 2021), that demonstrate a drought avoidance strategy whereby species have altered growth phenology patterns, avoiding active growth during the drought season. In addition, the high level of interspecific variation in growth phenology reported here suggests that drought could have variable impacts.
While there is evidence that supports both the resilience of the Acadian forest (Zhang P. et al., 2020; Zhang Y.-L. et al., 2020), and the future demise of the region (Reyer et al., 2013; Taylor et al., 2017), lack of drought tolerance knowledge featuring a variety of species and geographic regions (Panchen et al., 2014; Piper and Paula, 2020) limit the accuracy of predictions. The complex drivers of species growth patterns (Pearse et al., 2017; Csilléry et al., 2020) additionally mean that the expected response to climate variables, such as drought, are often more complex than models are able to predict (Alberto et al., 2013). Photoperiod and temperature have significant control over phenology patterns (Körner and Basler, 2010), and these were consistent between species in this experiment, which likely resulted in a homogenizing effect of species’ phenology patterns (Csilléry et al., 2020). Similarly, the limited resources available to potted seedlings and relatively late release from dormancy compared to the natural start of the growing season mean species growth patterns may not reflect those in natural environments. Considerably more research into the drought tolerance and individual species adaptations is needed to better understand how the Acadian forest region will respond to the increased drought frequencies and intensities predicted for this region (Dai, 2013; Cook et al., 2015).
Data Availability Statement
The raw data supporting the conclusions of this article will be made available by the authors, without undue reservation.
Author Contributions
LD’O contributed to conception and design of the study and to data collection. EP performed the statistical analysis and wrote the first draft of the manuscript with contributions from LD’O. Both authors contributed to the interpretation of the results and to the manuscript revision, read, and approved the submitted version.
Funding
This research was financially supported by the Natural Sciences and Engineering Research Council of Canada (Discovery Grant awarded to LD’O RGPIN-2019-04353) and New Brunswick Innovation Fund (Start-Up Grant RIF 2019-029).
Conflict of Interest
The authors declare that the research was conducted in the absence of any commercial or financial relationships that could be construed as a potential conflict of interest.
Publisher’s Note
All claims expressed in this article are solely those of the authors and do not necessarily represent those of their affiliated organizations, or those of the publisher, the editors and the reviewers. Any product that may be evaluated in this article, or claim that may be made by its manufacturer, is not guaranteed or endorsed by the publisher.
Acknowledgments
We would like to thank Megan Doyle for her assistance with data collection throughout the 2019 growing season, Cédric Albert for additional assistance with data analysis, as well as members of the Northern EDGE Lab for their critical reviews of earlier drafts of this manuscript.
Supplementary Material
The Supplementary Material for this article can be found online at: https://www.frontiersin.org/articles/10.3389/ffgc.2022.784382/full#supplementary-material
Supplementary Figure 1 | The relative growth rate of seedlings of thirteen species throughout one growing season.
References
Alberto, F. J., Aitken, S. N., Alía, R., González-Martínez, S. C., Hänninen, H., Kremer, A., et al. (2013). Potential for evolutionary responses to climate change – evidence from tree populations. Glob. Change Biol. 19, 1645–1661. doi: 10.1111/gcb.12181
Bell, D. M., Bradford, J. B., and Lauenroth, W. K. (2014). Early indicators of change: divergent climate envelopes between tree life stages imply range shifts in the western United States. Glob. Ecol. Biogeogr. 23, 168–180. doi: 10.1111/geb.12109
Bourque, C. P.-A., and Hassan, Q. K. (2008). Projected impacts of climate change on species distribution in the Acadian Forest region of eastern Nova Scotia. For. Chron. 84, 553–557. doi: 10.5558/tfc84553-4
Brodribb, T. J., Pittermann, J., and Coomes, D. A. (2012). Elegance versus Speed: examining the Competition between Conifer and Angiosperm Trees. Int. J. Plant Sci. 173, 673–694. doi: 10.1086/666005
Brouwer, R. (1963). Some aspects of the equilibrium between overground and underground plant parts. Jaarb. Van Het Inst. Voor Biol. En Scheikd. Onderz. Aan Landbouwgewassen 213, 31–39.
Brunner, I., Herzog, C., Dawes, M. A., Arend, M., and Sperisen, C. (2015). How tree roots respond to drought. Front. Plant Sci. 6:547. doi: 10.3389/fpls.2015.00547
Buermann, W., Bikash, P. R., Jung, M., Burn, D. H., and Reichstein, M. (2013). Earlier springs decrease peak summer productivity in North American boreal forests. Environ. Res. Lett. 8:024027. doi: 10.1088/1748-9326/8/2/024027
Buermann, W., Forkel, M., O’Sullivan, M., Sitch, S., Friedlingstein, P., Haverd, V., et al. (2018). Widespread seasonal compensation effects of spring warming on northern plant productivity. Nature 562, 110–114. doi: 10.1038/s41586-018-0555-7
Bugmann, H. (1996). Functional types of trees in temperate and boreal forests: classification and testing. J. Veg. Sci. 7, 359–370. doi: 10.2307/3236279
Burke, M. K., and Raynal, D. J. (1994). Fine root growth phenology, production, and turnover in a northern hardwood forest ecosystem. Plant Soil 162, 135–146. doi: 10.1007/BF01416099
Carnicer, J., Barbeta, A., Sperlich, D., Coll, M., and Penuelas, J. (2013). Contrasting trait syndromes in angiosperms and conifers are associated with different responses of tree growth to temperature on a large scale. Front. Plant Sci. 4:409. doi: 10.3389/fpls.2013.00409
Cook, B. I., Ault, T. R., and Smerdon, J. E. (2015). Unprecedented 21st century drought risk in the American Southwest and Central Plains. Sci. Adv. 1:e1400082. doi: 10.1126/sciadv.1400082
Corlett, R. T., and Westcott, D. A. (2013). Will plant movements keep up with climate change? Trends Ecol. Evol. 28, 482–488. doi: 10.1016/j.tree.2013.04.003
Crous, C. J., Jacobs, S. M., and Esler, K. J. (2012). Wood anatomical traits as a measure of plant responses to water availability: invasive Acacia mearnsii De Wild. compared with native tree species in fynbos riparian ecotones, South Africa. Trees 26, 1527–1536. doi: 10.1007/s00468-012-0726-3
Csilléry, K., Buchmann, N., and Fady, B. (2020). Adaptation to drought is coupled with slow growth, but independent from phenology in marginal silver fir (Abies alba Mill.) populations. Evol. Appl. 13, 2357–2376. doi: 10.1111/eva.13029
Cuny, H. E., Rathgeber, C. B. K., Frank, D., Fonti, P., and Fournier, M. (2014). Kinetics of tracheid development explain conifer tree-ring structure. New Phytol. 203, 1231–1241. doi: 10.1111/nph.12871
Dai, A. (2011). Drought under global warming: a review: drought under global warming. Wiley Interdiscip. Rev. Clim. Change 2, 45–65. doi: 10.1002/wcc.81
Dai, A. (2013). Increasing drought under global warming in observations and models. Nat. Clim. Change 3, 52–58. doi: 10.1038/nclimate1633
de Villemereuil, P., Gaggiotti, O. E., Mouterde, M., and Till-Bottraud, I. (2016). Common garden experiments in the genomic era: new perspectives and opportunities. Heredity 116, 249–254. doi: 10.1038/hdy.2015.93
Deslauriers, A., Rossi, S., and Anfodillo, T. (2007). Dendrometer and intra-annual tree growth: what kind of information can be inferred? Dendrochronologia 25, 113–124. doi: 10.1016/j.dendro.2007.05.003
D’Orangeville, L., Itter, M., Kneeshaw, D., Munger, J. W., Richardson, A. D., Dyer, J. M., et al. (2021). Peak radial growth of diffuse-porous species occurs during periods of lower water availability than for ring-porous and coniferous trees. Tree Physiol. tab101. doi: 10.1093/treephys/tpab101
D’Orangeville, L., Maxwell, J., Kneeshaw, D., Pederson, N., Duchesne, L., Logan, T., et al. (2018). Drought timing and local climate determine the sensitivity of eastern temperate forests to drought. Glob. Change Biol. 24, 2339–2351. doi: 10.1111/gcb.14096
Engelbrecht, B. M. J., Kursar, T. A., and Tyree, M. T. (2005). Drought effects on seedling survival in a tropical moist forest. Trees 19, 312–321. doi: 10.1007/s00468-004-0393-0
Fisichelli, N. A., Frelich, L. E., and Reich, P. B. (2014). Temperate tree expansion into adjacent boreal forest patches facilitated by warmer temperatures. Ecography 37, 152–161. doi: 10.1111/j.1600-0587.2013.00197.x
Forner, A., Valladares, F., Bonal, D., Granier, A., Grossiord, C., and Aranda, I. (2018). Extreme droughts affecting Mediterranean tree species’ growth and water-use efficiency: the importance of timing. Tree Physiol. 38, 1127–1137. doi: 10.1093/treephys/tpy022
Foster, T. E., Schmalzer, P. A., and Fox, G. A. (2014). Timing matters: the seasonal effect of drought on tree growth 1. J. Torrey Bot. Soc. 141, 225–241. doi: 10.3159/TORREY-D-13-00060.1
Gerhardt, K. (1996). Effects of root competition and canopy openness on survival and growth of tree seedlings in a tropical seasonal dry forest. For. Ecol. Manag. 82, 33–48. doi: 10.1016/0378-1127(95)03700-4
Gienapp, P., Teplitsky, C., Alho, J. S., Mills, J. A., and Merilä, J. (2008). Climate change and evolution: disentangling environmental and genetic responses. Mol. Ecol. 17, 167–178. doi: 10.1111/j.1365-294X.2007.03413.x
Gruber, A., Strobl, S., Veit, B., and Oberhuber, W. (2010). Impact of drought on the temporal dynamics of wood formation in Pinus sylvestris. Tree Physiol. 30, 490–501. doi: 10.1093/treephys/tpq003
Hamanishi, E. T., and Campbell, M. M. (2011). Genome-wide responses to drought in forest trees. For. Int. J. For. Res. 84, 273–283. doi: 10.1093/forestry/cpr012
Hartmann, H. (2011). Will a 385 million year-struggle for light become a struggle for water and for carbon? – How trees may cope with more frequent climate change-type drought events. Glob. Change Biol. 17, 642–655. doi: 10.1111/j.1365-2486.2010.02248.x
Houle, D., Bouffard, A., Duchesne, L., Logan, T., and Harvey, R. (2012). Projections of Future Soil Temperature and Water Content for Three Southern Quebec Forested Sites. J. Clim. 25, 7690–7701. doi: 10.1175/JCLI-D-11-00440.1
Housset, J. M., Nadeau, S., Isabel, N., Depardieu, C., Duchesne, I., Lenz, P., et al. (2018). Tree rings provide a new class of phenotypes for genetic associations that foster insights into adaptation of conifers to climate change. New Phytol. 218, 630–645. doi: 10.1111/nph.14968
Hunter, A. F., and Lechowicz, M. J. (1992). Predicting the Timing of Budburst in Temperate Trees. J. Appl. Ecol. 29:597. doi: 10.2307/2404467
Kannenberg, S. A., Novick, K. A., Alexander, M. R., Maxwell, J. T., Moore, D. J. P., Phillips, R. P., et al. (2019b). Linking drought legacy effects across scales: from leaves to tree rings to ecosystems. Glob. Change Biol. 25, 2978–2992. doi: 10.1111/gcb.14710
Kannenberg, S. A., Maxwell, J. T., Pederson, N., D’Orangeville, L., Ficklin, D. L., and Phillips, R. P. (2019a). Drought legacies are dependent on water table depth, wood anatomy and drought timing across the eastern US. Ecol. Lett. 22, 119–127. doi: 10.1111/ele.13173
Körner, C., and Basler, D. (2010). Phenology Under Global Warming. Science 327, 1461–1462. doi: 10.1126/science.1186473
Kozlowski, T. T., and Pallardy, S. G. (2002). Acclimation and adaptive responses of woody plants to environmental stresses. Bot. Rev. 68, 270–334.
Kramer, P. J., and Boyer, J. S. (1995). Water relations of plants and soils. San Diego: Academic Press.
Ladefoged, K. (1952). The periodicity of wood formation. Biol. Skr. Kgl Dan. Vidensk. Selsk. Kobenhavn 7:98.
Lempereur, M., Martin-StPaul, N. K., Damesin, C., Joffre, R., Ourcival, J.-M., Rocheteau, A., et al. (2015). Growth duration is a better predictor of stem increment than carbon supply in a Mediterranean oak forest: implications for assessing forest productivity under climate change. New Phytol. 207, 579–590. doi: 10.1111/nph.13400
Lloret, F., Sapes, G., Rosas, T., Galiano, L., Saura-Mas, S., Sala, A., et al. (2018). Non-structural carbohydrate dynamics associated with drought-induced die-off in woody species of a shrubland community. Ann. Bot. 121, 1383–1396. doi: 10.1093/aob/mcy039
Loo, J., and Ives, N. (2003). The Acadian forest: historical condition and human impacts. For. Chron. 79, 462–474. doi: 10.5558/tfc79462-3
Markesteijn, L., and Poorter, L. (2009). Seedling root morphology and biomass allocation of 62 tropical tree species in relation to drought- and shade-tolerance. J. Ecol. 97, 311–325. doi: 10.1111/j.1365-2745.2008.01466.x
Mathys, A. S., Coops, N. C., Simard, S. W., Waring, R. H., and Aitken, S. N. (2018). Diverging distribution of seedlings and mature trees reflects recent climate change in British Columbia. Ecol. Model. 384, 145–153. doi: 10.1016/j.ecolmodel.2018.06.008
McCormack, M. L., Adams, T. S., Smithwick, E. A. H., and Eissenstat, D. M. (2014). Variability in root production, phenology, and turnover rate among 12 temperate tree species. Ecology 95, 2224–2235. doi: 10.1890/13-1942.1
McDowell, N., Pockman, W. T., Allen, C. D., Breshears, D. D., Cobb, N., Kolb, T., et al. (2008). Mechanisms of plant survival and mortality during drought: why do some plants survive while others succumb to drought? New Phytol. 178, 719–739. doi: 10.1111/j.1469-8137.2008.02436.x
McDowell, N. G., Ryan, M. G., Zeppel, M. J. B., and Tissue, D. T. (2013). Feature: improving our knowledge of drought-induced forest mortality through experiments, observations, and modeling. New Phytol. 200, 289–293. doi: 10.1111/nph.12502
McKay, J. K., Bishop, J. G., Lin, J.-Z., Richards, J. H., Sala, A., and Mitchell-Olds, T. (2001). Local adaptation across a climatic gradient despite small effective population size in the rare sapphire rockcress. Proc. R. Soc. Lond. B Biol. Sci. 268, 1715–1721. doi: 10.1098/rspb.2001.1715
Michaelian, M., Hogg, E. H., Hall, R. J., and Arsenault, E. (2011). Massive mortality of aspen following severe drought along the southern edge of the Canadian boreal forest. Glob. Change Biol. 17, 2084–2094. doi: 10.1111/j.1365-2486.2010.02357.x
Møller, A., and Jennions, M. D. (2002). How much variance can be explained by ecologists and evolutionary biologists? Oecologia 132, 492–500. doi: 10.1007/s00442-002-0952-2
Moran, E., Lauder, J., Musser, C., Stathos, A., and Shu, M. (2017). The genetics of drought tolerance in conifers. New Phytol. 216, 1034–1048. doi: 10.1111/nph.14774
Nguyen, H. T., Meir, P., Sack, L., Evans, J. R., Oliveira, R. S., and Ball, M. C. (2017). Leaf water storage increases with salinity and aridity in the mangrove Avicennia marina: integration of leaf structure, osmotic adjustment and access to multiple water sources. Plant Cell Environ. 40, 1576–1591. doi: 10.1111/pce.12962
Niinemets, Ü (2010). Responses of forest trees to single and multiple environmental stresses from seedlings to mature plants: past stress history, stress interactions, tolerance and acclimation. For. Ecol. Manag. 260, 1623–1639. doi: 10.1016/j.foreco.2010.07.054
Niinemets, Ü, and Valladares, F. (2006). Tolerance to Shade, Drought, and Waterlogging of Temperate Northern Hemisphere Trees and Shrubs. Ecol. Monogr. 76, 521–547.
Olmo, M., Lopez-Iglesias, B., and Villar, R. (2014). Drought changes the structure and elemental composition of very fine roots in seedlings of ten woody tree species. Implications for a drier climate. Plant Soil 384, 113–129. doi: 10.1007/s11104-014-2178-6
Panchen, Z. A., Primack, R. B., Nordt, B., Ellwood, E. R., Stevens, A.-D., Renner, S. S., et al. (2014). Leaf out times of temperate woody plants are related to phylogeny, deciduousness, growth habit and wood anatomy. New Phytol. 203, 1208–1219. doi: 10.1111/nph.12892
Pearse, I. S., Aguilar, J., Schroder, J., and Strauss, S. Y. (2017). Macroevolutionary constraints to tolerance: trade-offs with drought tolerance and phenology, but not resistance. Ecology 98, 2758–2772. doi: 10.1002/ecy.1995
Piper, F. I. (2011). Drought induces opposite changes in the concentration of non-structural carbohydrates of two evergreen Nothofagus species of differential drought resistance. Ann. For. Sci. 68, 415–424. doi: 10.1007/s13595-011-0030-1
Piper, F. I., and Paula, S. (2020). The Role of Nonstructural Carbohydrates Storage in Forest Resilience under Climate Change. Curr. For. Rep. 6, 1–13. doi: 10.1007/s40725-019-00109-z
Polle, A., Chen, S. L., Eckert, C., and Harfouche, A. (2019). Engineering Drought Resistance in Forest Trees. Front. Plant Sci. 9:1875. doi: 10.3389/fpls.2018.01875
Poorter, H., Niklas, K. J., Reich, P. B., Oleksyn, J., Poot, P., and Mommer, L. (2012). Biomass allocation to leaves, stems and roots: meta-analyses of interspecific variation and environmental control. New Phytol. 193, 30–50. doi: 10.1111/j.1469-8137.2011.03952.x
Poorter, L., and Markesteijn, L. (2008). Seedling Traits Determine Drought Tolerance of Tropical Tree Species. Biotropica 40, 321–331. doi: 10.1111/j.1744-7429.2007.00380.x
Puglielli, G., Laanisto, L., Poorter, H., and Niinemets, Ü (2020). Global patterns of biomass allocation in woody species with different tolerance of shade and drought: evidence for multiple strategies. New Phytol. 229, 308–322. doi: 10.1111/nph.16879
Reyer, C. P. O., Leuzinger, S., Rammig, A., Wolf, A., Bartholomeus, R. P., Bonfante, A., et al. (2013). A plant’s perspective of extremes: terrestrial plant responses to changing climatic variability. Glob. Change Biol. 19, 75–89. doi: 10.1111/gcb.12023
Richardson, A. D., Andy Black, T., Ciais, P., Delbart, N., Friedl, M. A., Gobron, N., et al. (2010). Influence of spring and autumn phenological transitions on forest ecosystem productivity. Philos. Trans. R. Soc. B Biol. Sci. 365, 3227–3246. doi: 10.1098/rstb.2010.0102
Rossi, S., Anfodillo, T., Čufar, K., Cuny, H. E., Deslauriers, A., Fonti, P., et al. (2013). A meta-analysis of cambium phenology and growth: linear and non-linear patterns in conifers of the northern hemisphere. Ann. Bot. 112, 1911–1920. doi: 10.1093/aob/mct243
Rossi, S., Anfodillo, T., Čufar, K., Cuny, H. E., Deslauriers, A., Fonti, P., et al. (2016). Pattern of xylem phenology in conifers of cold ecosystems at the Northern Hemisphere. Glob. Change Biol. 22, 3804–3813. doi: 10.1111/gcb.13317
Rossi, S., Deslauriers, A., Anfodillo, T., Morin, H., Saracino, A., Motta, R., et al. (2006). Conifers in cold environments synchronize maximum growth rate of tree-ring formation with day length. New Phytol. 170, 301–310. doi: 10.1111/j.1469-8137.2006.01660.x
Sack, L., Grubb, P. J., and Marañón, T. (2003). The functional morphology of juvenile plants tolerant of strong summer drought in shaded forest understories in southern Spain. Plant Ecol. 168, 139–163.
Sork, V. L., Aitken, S. N., Dyer, R. J., Eckert, A. J., Legendre, P., and Neale, D. B. (2013). Putting the landscape into the genomics of trees: approaches for understanding local adaptation and population responses to changing climate. Tree Genet. Genomes 9, 901–911. doi: 10.1007/s11295-013-0596-x
Steinaker, D. F., Wilson, S. D., and Peltzer, D. A. (2010). Asynchronicity in root and shoot phenology in grasses and woody plants. Glob. Change Biol. 16, 2241–2251. doi: 10.1111/j.1365-2486.2009.02065.x
Taylor, A. R., Boulanger, Y., Price, D. T., Cyr, D., McGarrigle, E., Rammer, W., et al. (2017). Rapid 21st century climate change projected to shift composition and growth of Canada’s Acadian Forest Region. For. Ecol. Manag. 405, 284–294. doi: 10.1016/j.foreco.2017.07.033
Tyree, M. T., Engelbrecht, B. M. J., Vargas, G., and Kursar, T. A. (2003). Desiccation Tolerance of Five Tropical Seedlings in Panama. Relationship to a Field Assessment of Drought Performance. Plant Physiol. 132, 1439–1447. doi: 10.1104/pp.102.018937
Vanhellemont, M., Sousa-Silva, R., Maes, S. L., Van den Bulcke, J., Hertzog, L., De Groote, S. R. E., et al. (2019). Distinct growth responses to drought for oak and beech in temperate mixed forests. Sci. Total Environ. 650, 3017–3026. doi: 10.1016/j.scitotenv.2018.10.054
Waring, P. F. (1951). Growth Studies in Woody Species IV. The Initiation of Cambial Activity in Ring-Porous Species. Physiol. Plant. 4, 546–546. doi: 10.1111/j.1399-3054.1951.tb07692.x
Wiley, E. (2020). Do Carbon Reserves Increase Tree Survival during Stress and Following Disturbance? Curr. For. Rep. 6, 14–25. doi: 10.1007/s40725-019-00106-2
Xu, L., Chen, N., and Zhang, X. (2019). Global drought trends under 1.5 and 2 °C warming. Int. J. Climatol. 39, 2375–2385. doi: 10.1002/joc.5958
Zapater, M., Bréda, N., Bonal, D., Pardonnet, S., and Granier, A. (2013). Differential response to soil drought among co-occurring broad-leaved tree species growing in a 15- to 25-year-old mixed stand. Ann. For. Sci. 70, 31–39. doi: 10.1007/s13595-012-0233-0
Zhang, P., Zhou, X., Fu, Y., Shao, J., Zhou, L., Li, S., et al. (2020). Differential effects of drought on nonstructural carbohydrate storage in seedlings and mature trees of four species in a subtropical forest. For. Ecol. Manag. 469:118159. doi: 10.1016/j.foreco.2020.118159
Zhang, Y.-L., Moser, B., Li, M.-H., Wohlgemuth, T., Lei, J.-P., and Bachofen, C. (2020). Contrasting Leaf Trait Responses of Conifer and Broadleaved Seedlings to Altered Resource Availability Are Linked to Resource Strategies. Plants 9:621. doi: 10.3390/plants9050621
Zhu, K., Woodall, C. W., and Clark, J. S. (2012). Failure to migrate: lack of tree range expansion in response to climate change. Glob. Change Biol. 18, 1042–1052. doi: 10.1111/j.1365-2486.2011.02571.x
Zimmermann, M. H., and Brown, C. L. (1971). Trees: structure and function. 4. printing. Berlin: Springer.
Keywords: Acadian forest, biomass allocation, drought tolerance, phenology, shoot-to-root ratio, root mass fraction
Citation: Pearson E and D’Orangeville L (2022) Relating the Growth Phenology and Biomass Allocation in Seedlings of 13 Acadian Tree Species With Their Drought Tolerance. Front. For. Glob. Change 5:784382. doi: 10.3389/ffgc.2022.784382
Received: 27 September 2021; Accepted: 24 January 2022;
Published: 14 February 2022.
Edited by:
Nan Cong, Institute of Geographic Sciences and Natural Resources Research, Chinese Academy of Sciences (CAS), ChinaReviewed by:
Roberto Tognetti, University of Molise, ItalyKe Huang, University of Copenhagen, Denmark
Copyright © 2022 Pearson and D’Orangeville. This is an open-access article distributed under the terms of the Creative Commons Attribution License (CC BY). The use, distribution or reproduction in other forums is permitted, provided the original author(s) and the copyright owner(s) are credited and that the original publication in this journal is cited, in accordance with accepted academic practice. No use, distribution or reproduction is permitted which does not comply with these terms.
*Correspondence: Loïc D’Orangeville, loic.dorangeville@unb.ca