- 1Hémera Centro de Observación de la Tierra, Escuela de Ingeniería Forestal, Facultad de Ciencias, Universidad Mayor, Santiago, Chile
- 2Forest Management Program, Mamiraua Institute for Sustainable Development, Tefé, Brazil
- 3Laboratorio Ecotono, Universidad Nacional del Comahue, Instituto de Investigaciones en Biodiversidad y Medioambiente (INIBIOMA)-CONICET, Bariloche, Argentina
- 4Unidad Mixta Pasteur INIA (UMPI), Institut Pasteur de Montevideo, Montevideo, Uruguay
Extreme drought events have increased in frequency during the 20th century triggered by global change. Thus, understanding tree-growth resilience across different terrestrial biomes has become a key goal in forest ecology. Here, we evaluate the tree-growth resilience to severe drought in the only Mediterranean-type Ecosystems of South America, using five isolated populations of Nothofagus macrocarpa. For each tree, in each sampling site, we obtained wood cores and fresh leaves for dendrochronological and population genetic analysis, respectively. An evaluation was conducted on growth resilience components in response to the most extreme drought of the 20th century in central Chile (i.e., 1968, with ∼80% of rainfall deficit), and the influence of genetic variability, biogeography, and tree size. We hypothesize that even though current remnant populations of N. macrocarpa are small and isolated, they have locally withstood changes in climate, and that they will be genetically diverse and have a high resilience to extreme droughts. We used nuclear microsatellite markers to estimate tree genetic variability in N. macrocarpa and investigate its correlation with phenotypic traits. We found a higher resistance in the two southernmost populations (mesic sites) than in the three northern populations (xeric sites), however those three xeric populations showed a higher recovery. In addition, a significant clear positive linear correlation between precipitation and resistance, and a negative recovery and relative resilience of tree growth to the extreme drought event of 1968 can be seen. High diversity for simple sequence repeats (SSR) markers was observed, although no population structure was inferred. Southern populations had a higher number of private alleles, which may be an indication of their long-lasting persistence under mesic conditions. Therefore, differences in resilience components are mainly explained by tree size and sites influences, but not genetic diversity. We concluded that observed differences in tree-growth resilience among sites can be explained by a great deal of phenotypic plasticity, fostered by genetically diverse gene pools. We advocate for a genome-wide analysis (i.e., SNP) so as to identify genomic regions correlated with phenotypic traits in order to improve the understanding of the evolutionary processes that shaped this forest resilience over time.
Introduction
Recent global warming has favored droughts (i.e., below-average precipitations and increased atmospheric evaporation) and desertification in most Mediterranean and temperate biome (Ahmed et al., 2013; Tsakiris, 2017; McDowell et al., 2020), but precipitation has increased in other regions, such as central Asia (Chen et al., 2021). Understanding how it influences tree growth and forest productivity across different terrestrial biomes has become a major aim of forest ecology research (Anderegg et al., 2015; Gazol et al., 2017a). A potential effect of drought and warming is the increase of climate-driven mortality of trees and forest decline (Allen et al., 2015; McDowell and Allen, 2015). Dendrochronology (tree-ring analysis) can help to reveal past forest growth conditions at annual resolution, and contribute with some important clues on trees adaptability (Schweingruber, 1996). In spite of the long tradition of tree-ring analysis, the relationship between genetic diversity and fitness in tree species is still poor (Matías et al., 2016; Evans et al., 2018). Broadly speaking, it is assumed that trees populations with greater genetic diversity have higher growth rates due to growth consistency and resilience to climatic disturbances (Reed and Frankham, 2003). However, there is still no agreement on the relationship between individual genetic diversity and tree growth, with positive relationships (e.g., Rodríguez-Quilón et al., 2015; Neophytou et al., 2016; González-Díaz et al., 2020) or no associations (Babushkina et al., 2016; Johnson et al., 2017).
The drought effects on tree growth may differ among forest type and species (Camarero et al., 2015). Some studies suggested that species from xeric habitats are better adapted to drought stress than species from mesic habitats (Linares and Tíscar, 2010), but others indicate the xeric habitats are highly affected by drought severity (Pompa-García et al., 2017). Therefore, for a better understanding of drought sensitivity it is necessary to analyze forests across a climatic gradient (Camarero et al., 2013). Moreover, the ecophysiological processes influencing tree growth are expected to change with individual age and size (Piovesan and Biondi, 2021). However, the genetic composition of tree populations is not explicitly considered in the traditional dendrochronological studies, and observed changes are assumed to be driven primarily by physiological responses to climatic change, without a significant genetic contribution (King et al., 2013). Heer et al. (2017) showed that phenotypic time series archived in tree rings are a powerful resource in genetic association studies. Recently González-Díaz et al. (2020) found that the levels of genetic diversity and its positive correlation with growth might have implications in the local tree response to increased drought conditions.
Mediterranean-type environments, like the central region of Chile, with winter rainfalls and summer droughts (Quintana and Aceituno, 2012), are one of the world’s “biodiversity hotspots” (Arroyo et al., 2006). They are among the most threatened forest ecosystems by climate change worldwide (Allen et al., 2010), and under significant habitat loss due to more than 300 years of intensive human activities (Hernández et al., 2016). The geology and geography of this Chilean region, characterized by a narrow valley between two great mountain cordilleras, Coastal and Andes range, and historical climate changes during Tertiary have generated high levels of endemism and biodiversity (Villagrán, 1995; Luebert and Pliscoff, 2006; Scherson et al., 2014). Specifically, the Mediterranean deciduous forest of Chile (MDFC) has suffered repeated cycles of flora expansion and isolation due to quaternary glaciations, which have contributed to shape the distribution of the current vegetation and floristic discontinuities between both mountains (Villagrán and Armesto, 2005).
These forests are relics of wider distributed floras from the past, that currently have been restricted as consequence of Quaternary’s climatic oscillations and anthropic influences, evidencing it is great ecological and evolutionary relevance (Mathiasen et al., 2020). Similarly, human activities have significant effects on genetic factors of tree taxa, whose consequences will depend on their life history characteristics (Mathiasen et al., 2007). Nothofagus macrocarpa [(DC.) Vásquez et Rodr.] distribution is considered a palaeoendemism, as it inhabits these Mediterranean-type climates at the genus northernmost range in South America, being considered the most important forest within MDFC (Gajardo, 2001; Donoso et al., 2010). It is currently distributed in small, isolated, disjoint populations remnants of its widespread past, where it is the dominant of tree species (Amigo and Rodriguez-Guitian, 2011). Because it is mainly restricted to the Metropolitan Region, near Santiago de Chile city, it has been subjected to intense exploitation pressures due to its wood quality (Donoso et al., 2010), mainly the populations near roads (Venegas-González et al., 2019). Recently it has been shown that N. macrocarpa forests growth rates are declining at a high rate due to increasing desertification and global climate change (Venegas-González et al., 2018a,b).
Nothofagus genus holds high genetic variability and it is widely distributed in South America, from Mediterranean to polar climates, colonizing diverse habitats (Premoli et al., 2012). In addition, Nothofagus species easily hybridize in contact zones, resulting in the introgression of new genetic variation through the development of fertile hybrids (Donoso, 1987; Acosta and Premoli, 2010; Premoli et al., 2012; Soliani et al., 2015; El Mujtar et al., 2017). Recently, based on nuclear microsatellites and chloroplast DNA, it was shown that N. macrocarpa is highly diverse and three geographically structured groups of populations were revealed through Coastal and Andes mountain ranges, and the Central depression (Mathiasen et al., 2020), supporting the high polymorphism expected along a latitudinal gradient in geographical patchy distributed species (Donoso, 1987).
Growth response to extreme drought and resilience vary across the distribution range of tree species (Gazol et al., 2017a), which could be related to individual size, site conditions or genetic aspects (Housset et al., 2021). In this study, we evaluated the growth resilience of N. macrocarpa populations, across the latitudinal gradient of its natural distribution, to severe drought in central Chile and the influence of genetic variability, bioclimatic envelope, and tree size. Considering that N. macrocarpa forests are relics that survived the last glacial period (Villagrán, 1995), we hypothesize that despite the isolation of current populations, all are highly resilient to extreme droughts. Long lasting local persistence of N. macrocarpa populations was also reinforced by phylogeographic analysis through chloroplast DNA (Mathiasen et al., 2020). Here, we assess components of forest growth resilience to 1968 drought through the estimate of resilience (Rs), relative resilience (RRs), resistance (Rt) and recovery (Rc) in central Chile during the 20th century, considering different age classes. We estimated the genetic variability of N. macrocarpa trees based on nuclear microsatellite markers and investigated their correlations with phenotypic traits expressed under extreme drought conditions. The main aims are: (i) to determine the resilience, resistance, and recovery to a severe drought in each population; (ii) analyze the genetic diversity within and among populations; and (iii) examine the contribution of genetic traits and tree size on growth resilience of N. macrocarpa individuals to drought.
Materials and Methods
Study Area and Nothofagus macrocarpa Populations
The study area is located in central Chile (32°57′ to 34°51′S, and 71°07′ to 70°40′W), both in Coastal and Andes mountain ranges. Five isolated populations of N. macrocarpa, structured in three genetic groups (Mathiasen et al., 2020), were sampled in central Chile mountains reaching the entire species distribution. Coastal sites are located in PLC (National Park La Campana), SER (Nature Sanctuary “El Roble”), SAC (Nature Sanctuary “Altos de Cantillana”), and RNL (National Reserve “Robleria Loncha”); while Andes site are located in SAH (Nature Sanctuary “Alto Huemul”) (Figure 1A and Supplementary Figure 1). All sampling sites are currently protected to different degrees (Park, Reserve, or Sanctuary) as national priority areas for flora and fauna conservation. However, the protection status was recently established, and most forests were under human disturbance activities until recently (for details see Venegas-González et al., 2019).
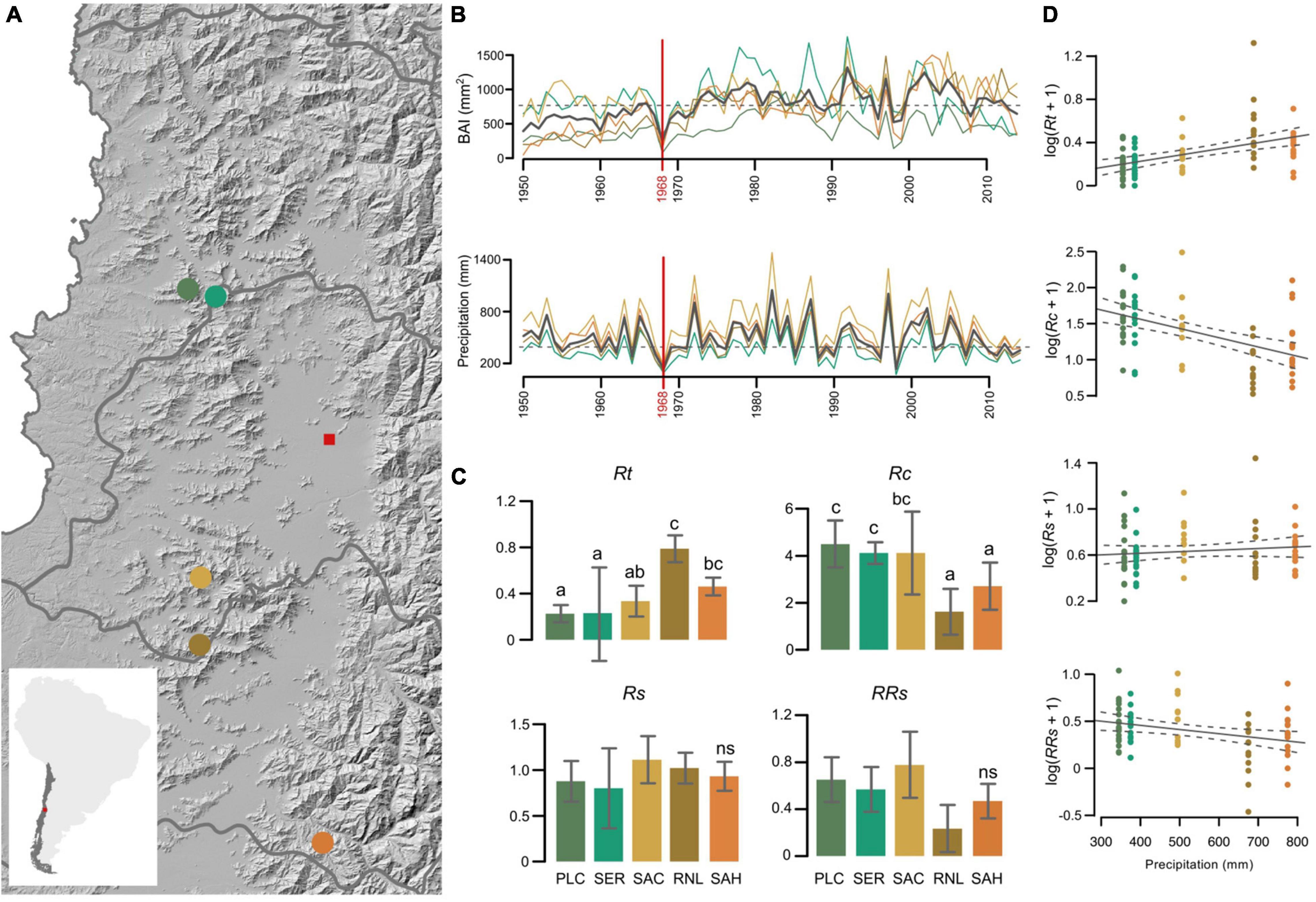
Figure 1. (A) Study area of five studied N. macrocarpa population (red is Santiago city). (B) BAI series of N. macrocarpa and annual total precipitation series by five sites for period 1950–2014. (C) Mean (bars) and SEM (segments) for the resilience components at each study site; Rt: Resistance, Rc: recovery, Rs: resilience, RRs: relative resilience. Different letters indicate significant difference between sites at 95% confidence level, while “ns” is not significative. (D) Relationship between precipitation and resilient components of N. macrocarpa five sites.
This Mediterranean ecoregion is characterized by a rainy winter (June to July) and a long dry summer (December to March), and the Coastal is drier than the Andes mountain range. The inter-annual rainfall in this region is highly variable and very influenced by the ENSO, with warm (cold) events in the central equatorial Pacific associated with wet (dry) conditions in central Chile (Montecinos and Aceituno, 2003). The five populations are found in a climatic gradient, being the northern populations those with the most xeric conditions (PLC and SER, annual precipitation <300 mm), while those of southern populations are more humid (RNL and SAH, annual precipitation >500 mm), and SAC is an intermediate site (Supplementary Figure 2). The mean temperature range for the study sites is 13–16°C. Throughout the Andean range, soils are developed from volcanic or granitic rocks and glacial sediments, and classified in the large group of forest brown soils, with a medium depth in slopes and deep in the high plains (Donoso, 1982). While through the Coastal range, soils are formed from granitic rocks and are poorly developed, usually residual on rocky outcrops (Villagrán and Armesto, 2005).
The geographical distribution of MDFC in the Andes mountain range has been shaped by the high elevations in these latitudes, which hampered the migrational dynamics of species (Armesto et al., 1995). This region has shown evidence of dynamical interactions between geomorphologic and climatic phenomena in the post-glacial period, with frequent cataclysm successions, volcanic eruptions and alluviums, which would also be associated with flora distribution patterns (Donoso, 1982), while the Coastal range is characterized by vegetation islands on hill tops, with high-elevation vegetation (Villagrán and Armesto, 2005). These Coastal plant assemblages would have been separated from the Andes vegetation due to the climatic changes during the last glacial-interglacial cycles in central Chile (Villagrán, 1995). This hypothesis is supported by the phytogeographic features of the hills at El Roble, La Campana, and Altos de Cantillana (Gajardo, 2001).
The selected tree species is N. macrocarpa, commonly called “Roble de Santiago,” considered a variety of Nothofagus obliqua for a long time, which is distributed further south (Donoso, 1987; Amigo and Rodriguez-Guitian, 2011). The taxonomic status as separate species was based on morphological traits (Vazquez and Rodríguez, 1999), cytogenetic evidence (Acosta and Premoli, 2018), and recently using nuclear DNA analysis (Mathiasen et al., 2020). These trees have deciduous leaves, reaching 25 m of height and >60 cm of diameter at breast height (DBH). The structure of N. macrocarpa formations usually includes a high arboreal stratum (over 16 m) of scattered individuals in non-intervened areas, and also as remnant areas of previous structures that were probably perturbed by fire or logging (Donoso et al., 2010).
Wood Core and Leaves Samplings
We sampled cores and leaves from the same individuals, on an average of 30 trees by site (for more detalis about sampling, see Venegas-González et al., 2018a). Trees were randomly chosen, aiming the inclusion of different age and size individuals (Supplementary Figure 3; Nehrbass-Ahles et al., 2014). For the dendrochronological analysis, two-three cores were taken at 1.3 m from each tree using a Pressler increment borer. For the genetic approach, fresh leaves were collected and kept in portable coolers, then dried with silica gel in the laboratory and stored at room temperature in paper bags until DNA extraction with CTAB method (Doyle, 1991).
Tree-Ring Analysis
Cores were prepared and cross-dated using classic methods of dendrochronology (Stokes and Smiles, 1996). Cross-dating and measurement accuracy were checked with the program COFECHA, which calculates cross correlations between individual series of each core and a master chronology (Holmes et al., 1986). More information about these chronologies are available in Venegas-González et al. (2018a). Tree growth was converted to basal area increment (BAI) according to the equation: BAI = π(r2t-r2t-1), where rt and rt-1 correspond to tree radius at DBH calculated at the end and beginning of annual increment in years t, respectively (Biondi and Qeadan, 2008). BAI is recommended to represent individual and stand-level changes (Camarero et al., 2015; Girardin et al., 2016). Annual BAI for each tree were estimated from raw ring-width series using R package dplR (Bunn and Korpela, 2016). In the absence of pith in cores, we used a geometric correction to estimate the number of missed rings and adjust the inner-ring date, i.e., pith was estimated by fitting a template of concentric circles with known radii to the curve of the innermost rings (Duncan, 1989). In trees in which the central core section could not be estimated because the innermost rings did not curve (last rings are parallels), we estimated missing rings with an individual model based on DBH without the bark (Venegas-González et al., 2019). After calculating the missing rings to the pith, we estimated the cambial age (at 1.3 m).
A biased sampling of big or fast-growing trees could produce false tree growth rates (Bowman et al., 2013). Therefore, it is important to sample coexisting trees of diverse sizes, growth rates and ages (Nehrbass-Ahles et al., 2014). We expect that BAI trends of N. macrocarpa and their climatic response varies with stand age. Thus, trees were classified in three age classes, young trees (<80 years, which were established after 1935), mature trees (80–160 years, which were established between 1855 and 1935) and elder trees (>160 years, which were established before of 1855). The 13 trees without pith had been classified as elder because they had at least 160 years, or missing distance to pith higher than half the radius.
Resilience Components to Extreme Drought Year
Based on BAI, we considered four resilience components (“resistance”-Rt, “recovery”-Rc, “resilience”-Rs, and “relative resilience”-RRs) to assess the extent to which tree growth can return to their pre-disturbance equilibrium, i.e., engineering resilience (Nikinmaa et al., 2020). Resistance (Rt) is defined as the individual capacity for maintaining performance during disturbance, recovery (Rc) as the individual ability for recovering the impact experienced, resilience (Rs) is the capacity to reach pre-disturbance performance levels and is estimated as the ratio between the performance after and before disturbance, and relative resilience (RRs) is the resilience weighted by the damage experienced during disturbance (Lloret et al., 2011). We considered 1968 as the extreme drought year (∼80% of rainfall deficit, Figure 1B), which is recognized as one of the three largest droughts of the last 1,000 years in central Chile (Le Quesne et al., 2006; Garreaud et al., 2017). Following Lloret et al. (2011), we computed the four metrics at the individual level:
where Dr indicates the BAI in the drought year, PreDr and PostDr indicate the mean BAI before and after the drought. We considered three years before and after the drought event in agreement with Anderegg et al. (2015) and DeSoto et al. (2020). Calculations were executed using the R package “pointRes” (van der Maaten-Theunissen et al., 2015).
Genetic Variability and Divergence Estimates
Simple sequence repeats (SSRs), i.e. nuclear microsatellite markers, of N. macrocarpa used for all the analyses were published in Mathiasen et al. (2020). Total genomic DNA was obtained as previously described in Mathiasen et al. (2020), using 40 mg of dried leaves that where grounded with a mechanic drill (Mixer-mill MM301, Restch, Inc., Germany) following the CTAB method (Doyle, 1991), with the modifications for other Nothofagus species described in Mathiasen and Premoli (2010). DNA amplification by PCR was performed using twelve microsatellite primer pairs previously developed for Nothofagus species (Jones et al., 2004; Marchelli and Gallo, 2006) that resulted in eight loci, which yielded reliable PCR products and consistent peak patterns (Mathiasen et al., 2020): NnBIO111, NnBIO72 and NnBIO37 developed for Nothofagus alpina by Marchelli et al. (2008), and ncutas04, ncutas08, ncutas09, ncutas12, and ncutas22 developed for Nothofagus cunninghamii by Jones et al. (2004). For the current genetic diversity analysis, we used eight nuclear microsatellite markers from 74 trees collected in the five sampling sites (PLC = 18, SER = 16, SAC = 12, RNL = 13, and SAH = 15) from the Central region of Chile. The genetic variability for each sampling location was estimated by the total number of alleles (AT), mean number of alleles (A), number of low-frequency alleles (A < 0.05), number of private alleles (AP), observed and expected heterozygosity (HO and HE, respectively), homozygosity by locus (HL) calculated using GENALEX v6.41 (Peakall and Smouse, 2006), and the allelic richness (AR) estimated in FSTAT v2.9.3.2 (Goudet, 1995). Divergence among sampling locations was calculated by pairwise RST statistics, accounting for the mutational distances among alleles, and the significance was evaluated by permutations based on 999 replicates. Two indexes were used to measure individual genetic heterozygosity, (i) the proportion of heterozygous loci in an individual (PHt) and (ii) the internal relatedness (IR) that estimates homozygosity accounting for allele frequency. PHt index weights the score for each locus by the average heterozygosity at that locus, whereas IR estimates heterozygosity giving a higher weight to homozygotes involving rare alleles more than the common alleles (Amos et al., 2001). A greater value of IR indicates decreased heterozygosity.
Statistical Analysis
To analyze trees sensitivity to drought, we performed linear mixed models (LMM) for each resilience component (Rt, Rs, Rc, and RRs), and for the basal area increase (BAI) at the individual level corresponding to 1968, using the “nlme” package (Pinheiro et al., 2017) from R statistical environment (R Core Team, 2018). Variables were log transformed [log(x + 1)] in order that the LMM residuals fit normality and were considered as response variables assuming a Gaussian error distribution with an identity link. We included as explanatory variables of fixed effects the trees size (DBH), the sampling sites (Site) and the genotype (private alleles and internal relatedness). These two genetic measures were included in the model to avoid collinearity because the internal relatedness (IR) is correlated with all other genetic variables tested, except private alleles (AP) (Figure 2A). In the same way, DBH and age are strongly correlated, so only DBH was used in the model (Supplementary Figure 4). As a random factor, we include the identity of the tree (Tree), to estimate variations in individual responses to environmental (Site) and genetic (Genotype) conditions. Finally, the model is as shown below:
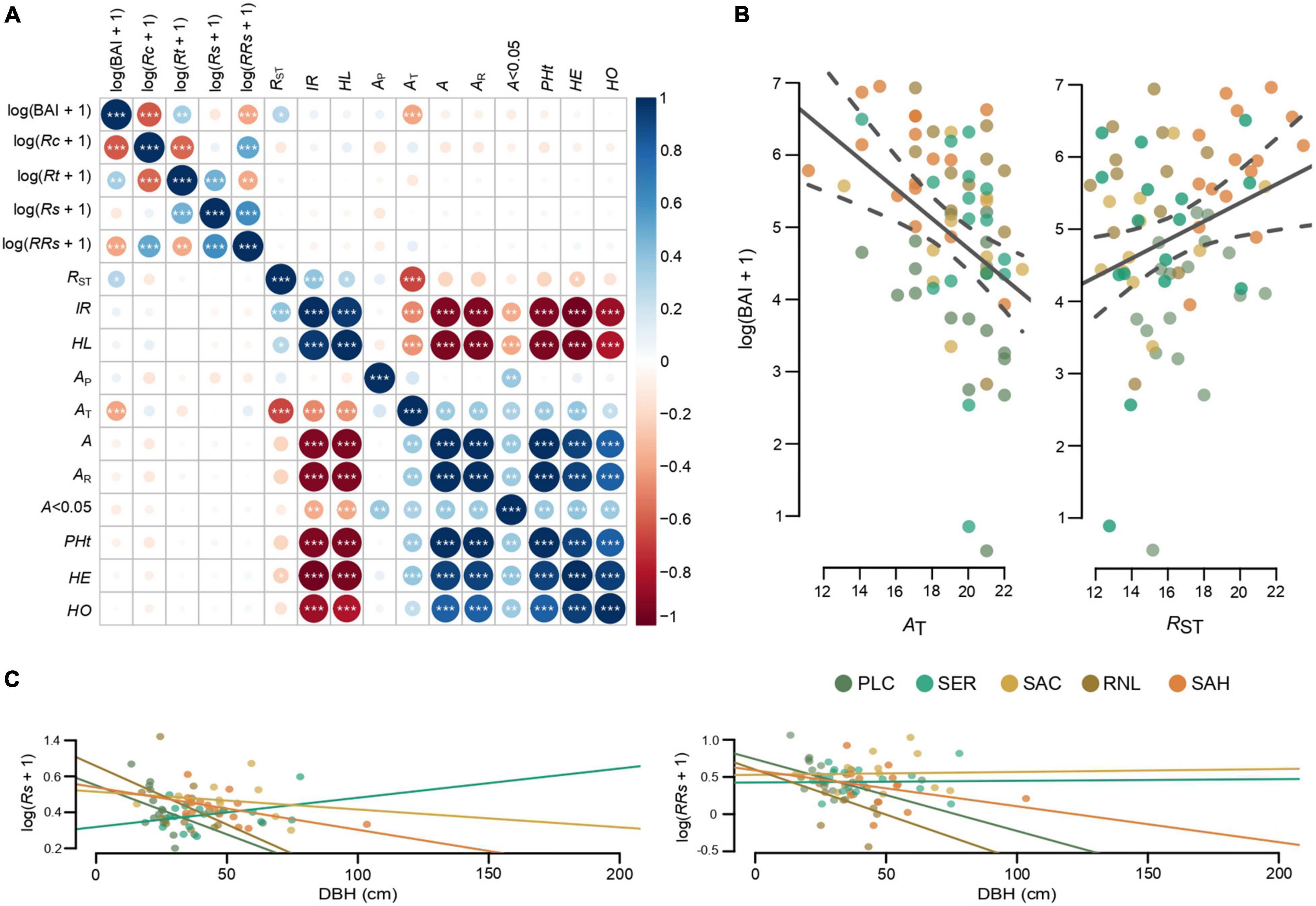
Figure 2. (A) Correlation matrix among tree growth variables and genetic variables. (B) Linear relation between tree growth variables and alleles numbers and distance genetic. These two genetics variables are the ones that have the highest correlations with the tree growth in 1968. (C) Linear relation between resilience indices and DBH.
where Y corresponds to the log transformed response variable and j represents growth and resilience components (BAI, Rt, Rc, Rs, and RRs) for 1968, DBH is a continuous variable that corresponds to the DBH at the individual level, Site is a five-level categorical variable (PLC, SER, SAC, RNL, and SAH) that represents the site conditions at the population level, IR is a continuous variable that represents the internal genetic relationship at the individual level, AP is a discrete variable corresponding to private alleles at the individual level, and trees corresponds to the random factor. The models were evaluated graphically (normality and homoscedasticity of the normalized residuals) and their adjustment was quantified with a pseudo-R2, both marginal and conditional [that is, the proportion of variance explained by fixed effects only and by random and fixed effects, respectively (Nakagawa and Schielzeth, 2013)].
Results
Tree-Ring Chronologies
The chronologies descriptive statistics were based only on trees with DNA analysis, and all trees are quite similar (Table 1). Rbar varied between 0.311 and 0.481, with EPS > 0.872, and time span (>5 trees) between 1826–2014 and 1929–2014. No differences were observed between trees used for each population chronologies (all trees) and those used for genetics, except for the age variable in SER site. The five populations showed different age structures: the youngest is PLC, in the north ranging, from 50 to 116 years, and the oldest are SAC and SAH, in the center and south respectively, ranging from 80 to ca. 300 years (Supplementary Figure 3). The lowest DBH values are found in PLC and RNL (northern and southern populations from the Coastal range), while the largest trees are found in the oldest populations. All chronologies showed negative point events in different years since 1950, but they coincide in the extreme drought event of 1968 (Supplementary Figure 5).
Growth Response to Extreme Drought
Our results show significant differences in two (Rt, Rc, p < 0.01) of the four resilience components to the extreme drought of 1968 in N. macrocarpa populations across Mediterranean forest in central Chile (Figure 1C). Specifically, we observed that the two southernmost populations (mesic sites) had 106% higher resistance (Rt) than the northernmost populations (xeric sites). However, the three xeric populations showed a marked recovery (125%) over mesic populations. A clearly linear relationship was obtained between precipitation and resistance (positive), as well as recovery and relative resilience (negative) of tree growth to the extreme event of 1968 (Figure 1D). We did not observe a significant difference among populations for the two resilience components (Rs and RRs), however there is a decreasing trend of the relative resilience (RRs) toward the south (Figure 1D).
Genetic Variation Among Sites
The genetic diversity for the 74 trees analyzed, a subsample of the reported populations in Mathiasen et al. (2020), was high in the five sampling sites for the eight nuclear microsatellite loci used. The number of alleles per locus ranged from five to 19, and the mean number of alleles per locus was 10.25. For the five localities, the multiloci expected (HE) and observed heterozygosity (HO) values ranged from 0.56 to 0.81 and 0.83 to 1.23, respectively, and mean HO = 0.394 and HE = 0.575 across all localities was moderate to high. All localities showed a high number of alleles, as evidenced by the genetic parameters AT, A, AE, AR as well as a high frequency of rare and private alleles (Table 2). Although it was not significant, it is observed that southern populations have a greater number of private alleles (Table 2). We also found a greater genetic distance from the southern populations (Andes), but they have a lower total number of alleles.
Genetic Influence on Growth Resilience to Extreme Drought
The correlation matrix in Figure 2A shows that no relationship was found between the four resilience co-components and the 11 genetic variables. However, the number of alleles and the genetic distance yielded a significant correlation with the BAI of the year 1968 (p-value: < 0.001 and 0.01), with an inverse and direct relationship, respectively (Figure 2B).
The LMM results show that BAI and resilience components for the extreme drought year were mainly determined by the conditions at the site level (Site) and to a lesser extent by the tree size (DBH), but not by IR and AP (Table 3). Most of the total variation for each variable was explained by random effects, with high conditional R2 values (from 0.89 to 0.92) and lesser marginal R2 values (from 0.12 to 0.33), being the resilience with the lower value. Specifically, BAI, Rc and Rt were mainly explained by site conditions. While resilience Rs and relative resilience RRs directly depend on DBH (and site to RRs), with an inverse relationship in most populations (Figure 2C). Therefore, LMM do not show statistical significance of the genetic variables.
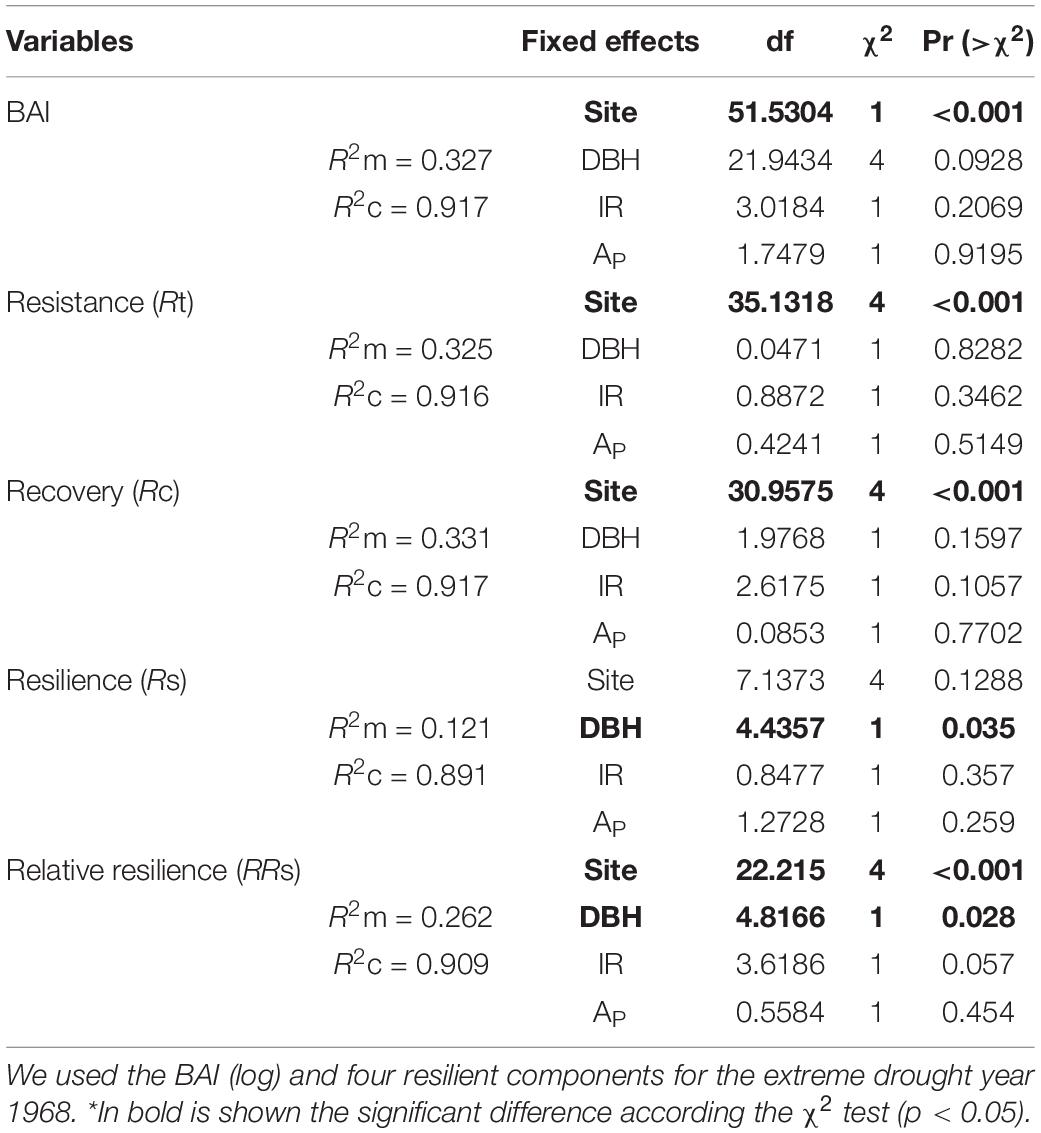
Table 3. Results of the log likelihood ratio test for the linear mixed model with site, internal relatedness (IR), private alleles (AP), and DBH as fixed factors and trees as random factors.
Discussion
We approached tree-growth resilience to an extreme drought occurred in 1968 in central Chile by dendrochronology and population genetics. On one hand, we focused on tree recovery (Rc) and resistance (Rt) to this extreme event in a 300-km transect along a latitudinal gradient (32°–34°S) of MDFC. On the other hand, we evaluated the contribution of genetic variability to tree-growth resilience. Drier sites (i.e., northern populations) had greater recovery (Rc) but less resistance (Rt), while the southern sites showed the inverse response. Despite the high genetic variability and private alleles in all populations, the differences in tree-growth resilience components (e.g., Rc and Rt) are mainly explained by tree size and precipitations.
Tree-Growth Resilience to Extreme Drought Year: Site Influence
All sites showed a significant decrease in radial growth in 1968, but the negative impact on tree-growth varies among them (Figure 1C). This can be explained by the foliar phenology trait in deciduous forests, since the forest productivity was affected at the same extreme drought year (Rita et al., 2020), possibly due to the reduction of the growing season (Decuyper et al., 2020). Our results reveal important seasonal differences in the resilience components in the five sites of N. macrocarpa. Humidity increases toward the south due to higher precipitation, and populations become more resistant to drought in both the Andes (SAH) and the Coastal (RNL) range, however a lower recovery trend was observed (Figure 1D). We hypothesize that despite the rainfall decrease in 1968 in southern sites, soil moisture was higher than at northern populations, due to geographical and climatic characteristics of the southern limit of the Andean-Mediterranean Central Forest.
Our findings are in agreement with the general trend found in the northern hemisphere, where tree-growth in populations inhabiting more humid climates were less sensitive to water deficit (i.e., higher Rt to drought), while populations in drier conditions recover faster (i.e., higher Rc to drought) (Gazol et al., 2017a, 2018). In southwestern Europe, the tree-growth resilience to extreme dry years was related to drought severity and forest composition, where Mediterranean species showed lower Rt to drought, but greater Rc than species from humid temperate forests (Gazol et al., 2018). In pine forests, similar results were found for 27 tree populations of Pinus halepensis, where Rc to drought was higher in drier sites, while Rt was higher in wetter sites (Gazol et al., 2017b). The same Rt–Rc relationship was found in Pinus pinaster forests from the Western Mediterranean Basin, where trees from drier sites were less resistant to drought, but recovered faster than in wetter sites (Sánchez-Salguero et al., 2018). These findings are in agreement with the only work published in Chile on resilience components for N. obliqua (Urrutia-Jalabert et al., 2021), where results showed that populations located at dry range edge were the most resilient to drought and have not been strongly affected by the current megadrought in the area.
A recent meta-analysis revealed that higher competition reduced Rt (p < 0.001) and improved Rc (p < 0.05), but did not consistently affect the resilience (Castagneri et al., 2021). The northern sites (PLC and SER) of N. macrocarpa populations have greater competition, as they consist of high-density forest stands, mainly composed by young trees derived of large-scale disturbance effects in the area (Venegas-González et al., 2018b). Although it showed higher Rc than southern populations (SAC, RNL, and SAH), it is necessary to consider that semi-arid forests presented a negative temporal trend in Rt to drought, which could limit the Rc potential if droughts become more frequent (Gazol et al., 2018). This result is especially relevant in the context of the historic megadrought that central Chile is experiencing, conditions that have increased during this century (Barría et al., 2021). Furthermore, legacy effects after drought have been reported to be more pervasive at dry than at wet sites (Anderegg et al., 2015). In addition, these forests in central Chile are the most degraded, and possibly have lost functional diversity and community composition, which would affect Rt and Rc to future extreme droughts (Granda et al., 2018). Moreover, trees that experienced more frequent long-term droughts become less resistant to extreme droughts (Bose et al., 2020), so it is expected that the southern populations showed lower Rt.
Tree-Growth Resilience to Extreme Drought Year: Diameter at Breast Height Influence
Tree size (DBH), and hence age (Supplementary Figure 2), negatively influenced tree-growth resilience, measured by resilience (Rs) and relative resilience (RRs), to the extreme drought of 1968 (i.e., larger trees are less able to reach pre-drought performance levels, Figure 2C). We previously verified that older trees of N. macrocarpa have decreased their growth in the last decades, at both northern and southern populations (Venegas-González et al., 2019). Similarly, larger trees of three abundant pine species (P. halepensis, Pinus nigra and Pinus sylvestris) in the northeast Iberian Peninsula were significantly less resistant and resilient to three extreme droughts recorded during the 1951–2010 period (Serra-Maluquer et al., 2018). These pine forests became less resistant, less resilient, and recovered worse during the last two droughts, suggesting an increased vulnerability after successive drought episodes. Another evaluation of P. halepensis populations (Granda et al., 2018) showed that growth Rs to drought was mostly dependent on tree age. In particular, younger trees were more resistant to drought but recovered less and were less resilient than elder trees (Ding et al., 2017). This evidence supports the hypothesis that larger trees are at higher risk of drought-induced hydraulic failure because of greater resistance in water transport upward (i.e., higher potential gradient and conduit resistance) (Bennett et al., 2015). However, the opposite trend was found in Mediterranean oak forests, where young trees are more sensitive and less resilient to drought than adult trees (Colangelo et al., 2017). How the ongoing climate change affects the resilience of Mediterranean-type forests of South America is a challenge that will help to identify local trends and contribute to the understanding of increasing droughts dynamics.
Tree-Growth Resilience to Extreme Drought Year: Genetic Variability Influence
The total number of alleles and the genetic distance that were negatively and positively associated to BAI of the 1968 extreme drought suggest that populations consisting of genotypes for particular alleles, and not necessarily the most genetically diverse ones, which in turn are more genetically distinct, may show greater growth. Nonetheless, the neutral markers used here do not allow to relate genetic variants to dendrophenotypes, such as BAI, and future genome wide association studies may greatly contribute to test this hypothesis. A negative association of heterozygosity and allelic richness was found with intra-population growth in northern marginal populations of Thuja occidentalis in the Canadian boreal forest (Housset et al., 2016). No genetic effect was detected on variation of BAI reported for another American species, Populus tremuloides (Latutrie et al., 2015). In the mountains of western Europe, the analysis of Pinus uncinata juvenile trees suggested that individuals with higher genetic diversity can grow more vigorously than others with less diversity, under favorable environmental conditions (González-Díaz et al., 2020). The same pattern was revealed for the European Spruce (Abies alba) and the authors concluded that higher genetic diversity favored adaptation to increased air pollution, also indicating that these forests are less affected by this phenomenon than their pairs of different origins and less diversity (Bosela et al., 2016). In the Norway Spruce (Picea abies), an economically important species that has been naturalized in many areas outside its native range, up to 44% of the phenotypic variation in the response to drought was explained by the genetic variation among alpine, central and southeastern European provenances (Trujillo-Moya et al., 2018).
Other studies have jointly analyzed genetic and dendroecological data (e.g., Babushkina et al., 2016; Johnson et al., 2017), and they have not found a significant correlation with growth traits. Despite the absence of a significant relationship, these authors hypothesized that genetic diversity could provide better fitness through increased growth when conditions are favorable. Heer et al. (2018) concluded that the absence of genetic signals could be attributed to stronger environmental effects compared to the genetic influence on growth processes, or to the lack of adequate genetic data.
Tree-growth and growth traits, fundamental components of forests adaptation, are highly influenced by physiology, genetic background, as well as biotic and abiotic drivers (Evans et al., 2018). Tree genetic diversity influences primary productivity and population recovery after disturbances (Hughes et al., 2008). Similarly, tree species responses to climate change are highly influenced by their genetic make-up, i.e., their evolutionary potential, and phenotypic plasticity (Housset et al., 2018). Therefore, tree-rings are an exceptional resource for understanding long-term growth response to environmental pressure, like extreme droughts. It can be potentially applied from individual-based genetic association studies in natural conditions (Heer et al., 2018) to the study of local adaptation to climate (Trujillo-Moya et al., 2018; Housset et al., 2021). Investigations of climate-driven responses based on tree-rings and population genetics are essential to assess tree species behavior to climate change.
The evidence presented here showed that greater among population divergence measured by RST was associated to higher BAI values measured under drought, suggesting that gene exchange can effectively affect their growth. Whether among population gene flow may promote tolerance to cope with environmental stress should be further tested given that it may enhance it as in Nothofagus dombeyi, that showed adaptive variation for water shortage (Diaz et al., 2021). Traditionally the difference in the tree-growth response to drought are explained by intrinsic differences in wood anatomy and physiology (Vitali et al., 2017), and the genetic architecture of trees may also contribute (González-Díaz et al., 2020; Fasanella et al., 2021). The genetic diversity of the N. macrocarpa 74 individuals analyzed here was high in the five sampling sites for the eight nuclear microsatellite loci used. However, the total number of alleles and genetic distance among sites were the only parameters that yielded significant differences among the populations (Table 2). Southern populations in the Andes range (i.e., RNL and SAH) have fewer total alleles, but showed the higher genetic distance against the remaining populations. Despite the fact that only five sites were analyzed, each one has its own genetic finger-print as shown by private alleles reported here and unique chloroplast DNA variants have been revealed (Mathiasen et al., 2020). Moreover, southern populations had a higher number of private alleles, which may be an indication of their long-lasting persistence under mesic conditions. Our results support that N. macrocarpa response to extreme drought would be mainly explained by the precipitation regime in each site and tree size, rather than by genetic variability (Table 3). Nonetheless, it was shown that genetic diversity of populations are a good proxy for phenotypic plasticity, population resilience, and evolutionary potential (Reusch et al., 2005; Noble et al., 2019; Pazzaglia et al., 2021). Recent genomic studies on the polymorphic N. dombeyi showed that single nucleotide polymorphisms (SNPs) could be applied to identify at the individual level distinct dendrophenotypes associated to drought tolerance (Fasanella et al., 2021). Thus, the high polymorphism of N. macrocarpa populations may have contributed to its long-lasting persistence and opens a new avenue to deeply investigate its response to future extreme climatic events.
Perspectives and Conclusion
An overall agreement exists that tree resilience to extreme droughts, under engineering resilience, depend of bioclimatic conditions of inhabiting sites (Nikinmaa et al., 2020). In central Chile, the ongoing rainfall decrease and accelerated temperature increase in winter and spring (Garreaud et al., 2020), could amplify drought stress and restrict population tree growth (Sánchez-Salguero et al., 2016). In addition, forest resilience to short-term extreme events such as heat waves is likely a survival key and must be accounted for a better prediction of its increasing impact on productivity and survival in future climates (Tatarinov et al., 2016). Recently, it has been proposed that tree radial growth will decrease at a regional level along 21st century influenced by the projected climate change (Matskovsky et al., 2021), however, it remains to be seen exactly what its impact will be, which will likely vary from site to site. Our work showed that N. macrocarpa resilience to the extreme drought of 1968 in central Chile is better explained by regional bioclimatic conditions and tree size than by genetic diversity levels. However, the use of genome-wide SNPs to assess genetically based tree vulnerability to past extreme droughts has allowed to identify adaptive patterns at the individual level (Fasanella et al., 2021). Therefore, we advocate a whole genome analysis (i.e., SNP) to identify genomic regions correlated with phenotypic traits (i.e., tree-growth), in order to improve the understanding of the evolutionary processes that shaped the resilience of this forest over time.
Moreover, deciduous forests have greater resistance to summer drought than evergreen forests, due to the high plasticity of cambial activity and the improvement of the carbon-structural carbohydrates status to growth (Pérez-de-Lis et al., 2017). A comparative analysis of phenotypic traits under common gardens and ecophysiological responses to experimentally induced drought on seedlings of the sister evergreen N. dombeyi and deciduous Nothofagus pumilio showed a greater vulnerability of the former to shortages in water supply (Diaz et al., 2021). In this sense, though broadleaved oak and beech are sensitive to late frosts that strongly reduce current year growth, they show high resistance and resilience to spring droughts and seem, therefore, better adapted to future climate projections (Vitasse et al., 2019). Here we infer that N. macrocarpa populations would be more resilient to future drought conditions than lower altitude sympatric communities (i.e., sclerophyllous forest), suggesting a different adaptational process and/or physiological plasticity of N. macrocarpa in the face of extreme drought events.
Climate predictions indicate drying trends and higher frequency of drought events in many regions worldwide, mainly in central Chile (Seager et al., 2019; Zappa et al., 2020). Currently the region is going through a historic megadrought since 2010, with a reduction in rainfall between 25 and 30% (Garreaud et al., 2020). In addition, the year 2019 and the present 2021 have been hyper-dry years, with a decrease in rainfall by 80% (Alvarez-Garreton et al., 2021; Garreaud, 2021). This scenario will strongly affect biogeochemical and eco-physiological processes of central Chile ecosystems. Therefore, the resilience of the only Mediterranean forests in South America will be further tested.
Data Availability Statement
The datasets generated for this study are available on request to the corresponding author/s.
Author Contributions
AV-G and PF conceived the manuscript idea. AV-G, PF, and CA-J sampled and compiled the data from the field. PM and AP conducted the genetic analysis. AV-G, SG-C, and PF made the statistical analysis and produced figures and tables. All authors revised and edited the manuscript.
Funding
This work was supported by Fondecyt 11180992, Rufford Small Grants for Nature Conservation 16501-2, 29211-1, PICT2019-149, and ClimatAmsud CLI2020009.
Conflict of Interest
The authors declare that the research was conducted in the absence of any commercial or financial relationships that could be construed as a potential conflict of interest.
The reviewer PM declared a shared parent affiliation, with no collaboration, with the authors PM and AP to the handling editor at the time of the review.
Publisher’s Note
All claims expressed in this article are solely those of the authors and do not necessarily represent those of their affiliated organizations, or those of the publisher, the editors and the reviewers. Any product that may be evaluated in this article, or claim that may be made by its manufacturer, is not guaranteed or endorsed by the publisher.
Acknowledgments
We thank the Chilean National Forest Corporation (CONAF), Christian Díaz, Julio Vergara, Fernanda Romero, Corporation Altos de Cantillana, Nature Sanctuary “Cerro El Roble,” Nature Sanctuary “Alto Huemul,” and Daniel de Pablo for the authorization of the fieldwork. In addition, AV-G thanks to his father, who helped in all the fieldwork in 2015 and was a motivator in the development of this project.
Supplementary Material
The Supplementary Material for this article can be found online at: https://www.frontiersin.org/articles/10.3389/ffgc.2022.762347/full#supplementary-material
Supplementary Figure 1 | Natural distribution range of Nothofagus macrocarpa according to the National Forestry Corporation of Chile (CONAF) Vegetation Record, but corrected by the study of Amigo and Rodriguez-Guitian (2011).
Supplementary Figure 2 | Ombrothermic diagram of five studied sites.
Supplementary Figure 3 | Classification of the structure of N. macrocarpa tree populations based on DBH and age.
Supplementary Figure 4 | Relation age vs. DBH, demonstrating a strong correlation between the variables (R2 = 0.40, p < 0.001).
Supplementary Figure 5 | Negative point years in the five sites of N. macrocarpa studied. These represent ≥75% trees of population considering all trees (≥65% in RNL). Arrows indicate the extreme drought year 1968.
References
Acosta, M. C., and Premoli, A. C. (2010). Evidence of chloroplast capture in South American Nothofagus (subgenus Nothofagus, Nothofagaceae). Mol. Phylogenet. Evol. 54, 235–242. doi: 10.1016/j.ympev.2009.08.008
Acosta, M. C., and Premoli, A. C. (2018). Understanding the extensive hybridization in South American Nothofagus through karyotype analysis. Bot. J. Linn. Soc. 188, 74–86. doi: 10.1093/botlinnean/boy043
Ahmed, M., Anchukaitis, K. J., Asrat, A., Borgaonkar, H. P., Braida, M., Buckley, B. M., et al. (2013). Continental-scale temperature variability during the past two millennia. Nat. Geosci. 6, 339–346. doi: 10.1038/ngeo1797
Allen, C. D., Breshears, D. D., and McDowell, N. G. (2015). On underestimation of global vulnerability to tree mortality and forest die-off from hotter drought in the Anthropocene. Ecosphere 6, 1–55.
Allen, C. D., Macalady, A. K., Chenchouni, H., Bachelet, D., McDowell, N., Vennetier, M., et al. (2010). A global overview of drought and heat-induced tree mortality reveals emerging climate change risks for forests. For. Ecol. Manage. 259, 660–684.
Alvarez-Garreton, C., Boisier, J. P., Garreaud, R., Seibert, J., and Vis, M. (2021). Progressive water deficits during multiyear droughts in basins with long hydrological memory in Chile. Hydrol. Earth Syst. Sci. 25, 429–446. doi: 10.5194/hess-25-429-2021
Amigo, J., and Rodriguez-Guitian, M. (2011). Bioclimatic and phytosociological diagnosis of the species of the Nothofagus genus (Nothofagaceae) in South America. Int. J. Geobot. Res. 1, 1–20. doi: 10.5616/ijgr110001
Amos, C. I., De Andrade, M., and Zhu, D. K. (2001). Comparison of multivariate tests for genetic linkage. Hum. Hered. 51, 133–144. doi: 10.1159/000053334
Anderegg, W. R. L., Schwalm, C., Biondi, F., Camarero, J. J., Koch, G., Litvak, M., et al. (2015). Pervasive drought legacies in forest ecosystems and their implications for carbon cycle models. Forest Ecol. 349, 528–532. doi: 10.1126/science.aab1833
Armesto, J., Villagrán, C., and Arroyo, M. K. eds (1995). Ecología De Los Bosques Nativos. Santiago: Editorial Universitaria.
Arroyo, M. T. K., Marquet, P. A., Marticorena, C., Cavieres, L. A., Squeo, F. A., Simonetti Zambelli, J. A., et al. (2006). El Hotspot Chileno, Prioridad Mundial Para La Conservación. Diversidad De Ecosistemas, Ecosistemas Terrestres. Sandiago: Repositorio Academico.
Babushkina, E. A., Vaganov, E. A., Grachev, A. M., Oreshkova, N. V., Belokopytova, L. V., Kostyakova, T. V., et al. (2016). The effect of individual genetic heterozygosity on general homeostasis, heterosis and resilience in Siberian larch (Larix sibirica Ledeb.) using dendrochronology and microsatellite loci genotyping. Dendrochronol 38, 26–37.
Barría, P., Sandoval, I. B., Guzman, C., Chadwick, C., Alvarez-Garreton, C., Díaz-Vasconcellos, R., et al. (2021). Water allocation under climate changeA diagnosis of the Chilean system. Elem. Sci. Anthr. 9:00131
Bennett, A. C., McDowell, N. G., Allen, C. D., and Anderson-Teixeira, K. J. (2015). Larger trees suffer most during drought in forests worldwide. Nat. Plants 1, 1–5. doi: 10.1038/nplants.2015.139
Biondi, F., and Qeadan, F. (2008). A theory-driven approach to tree-ring standardization: defining the biological trend from expected basal area increment. Tree Ring Res. 64, 81–96.
Bose, A. K., Gessler, A., Bolte, A., Bottero, A., Buras, A., Cailleret, M., et al. (2020). Growth and resilience responses of Scots pine to extreme droughts across Europe depend on predrought growth conditions. Glob. Chang. Biol. 26, 4521–4537. doi: 10.1111/gcb.15153
Bosela, M., Popa, I., Gömöry, D., Longauer, R., Tobin, B., Kyncl, J., et al. (2016). Effects of post-glacial phylogeny and genetic diversity on the growth variability and climate sensitivity of European silver fir. J. Ecol. 104, 716–724. doi: 10.1111/1365-2745.12561
Bowman, D. M. J. S., Brienen, R. J. W., Gloor, E., Phillips, O. L., and Prior, L. D. (2013). Detecting trends in tree growth: not so simple. Trends Plant Sci. 18, 11–17. doi: 10.1016/j.tplants.2012.08.005
Camarero, J. J., Gazol, A., Sangüesa-Barreda, G., Oliva, J., and Vicente-Serrano, S. M. (2015). To die or not to die: early warnings of tree dieback in response to a severe drought. J. Ecol. 103, 44–57.
Camarero, J. J., Manzanedo, R. D., Sanchez-Salguero, R., and Navarro-Cerrillo, R. M. (2013). Growth response to climate and drought change along an aridity gradient in the southernmost Pinus nigra relict forests. Ann. For. Sci. 70, 769–780. doi: 10.1007/s13595-013-0321-9
Castagneri, D., Vacchiano, G., Hacket-Pain, A., DeRose, R. J., Klein, T., and Bottero, A. (2021). Meta-analysis reveals different competition effects on tree growth resistance and resilience to drought. Ecosystems, 13, 1–14.
Chen, F., Yu, S., Shang, H., Zhang, R., Zhang, T., Zhang, H., et al. (2021). The Productivity of Low-Elevation Juniper Forests in Central Asia Increased Under Moderate Warming Scenarios. J. Geophys. Res. Biogeosci. 12:e2021JG006269.
Colangelo, M., Camarero, J. J., Battipaglia, G., Borghetti, M., De Micco, V., Gentilesca, T., et al. (2017). A multi-proxy assessment of dieback causes in a Mediterranean oak species. Tree Physiol. 37, 617–631. doi: 10.1093/treephys/tpx002
Decuyper, M., Chávez, R. O., Čufar, K., Estay, S. A., Clevers, J. G. P. W., Prislan, P., et al. (2020). Spatio-temporal assessment of beech growth in relation to climate extremes in Slovenia–An integrated approach using remote sensing and tree-ring data. Agric. Meteorol. 287:107925. doi: 10.1016/j.agrformet.2020.107925
DeSoto, L., Cailleret, M., Sterck, F., Jansen, S., Kramer, K., Robert, E. M. R., et al. (2020). Low growth resilience to drought is related to future mortality risk in trees. Nat. Commun. 11, 1–9. doi: 10.1038/s41467-020-14300-5
Diaz, D. G., Ignazi, G., Mathiasen, P., and Premoli, A. C. (2021). Climate-driven adaptive responses to drought of dominant tree species from Patagonia. New For. 2007, 2411–2502.
Ding, H., Pretzsch, H., Schütze, G., and Rötzer, T. (2017). Size-dependence of tree growth response to drought for Norway spruce and European beech individuals in monospecific and mixed-species stands. Plant Biol. 19, 709–719. doi: 10.1111/plb.12596
Donoso, C. (1987). Variación natural en especies de Nothofagus en Chile. Bosque 8, 85–97. doi: 10.4206/bosque.1987.v8n2-03
Donoso, S. R., Pena-Rojas, K., Delgado-Flores, C., Riquelme, A., and Paratori, M. (2010). Above-ground biomass accumulation and growth in a marginal Nothofagus macrocarpa forest in central Chile. Interciencia 35, 65–69.
Doyle, J. (1991). “DNA protocols for plants,” in Molecular Techniques in Taxonomy eds M. H. Godfray, A. W. B. Johnston, and J. P. W. Young (Berling Springer), 283–293. doi: 10.1007/978-3-642-83962-7_18
Duncan, R. P. (1989). An evaluation of errors in tree age estimates based on increment cores in kahikatea (Dacrycarpus dacrydioides). New Zeal. Nat. Sci. 16, 1–37.
El Mujtar, V., Sola, G., Aparicio, A., and Gallo, L. (2017). Pattern of natural introgression in a Nothofagus hybrid zone from south American temperate forests. Tree Genet. Genomes 13, 1–13.
Evans, M. E. K., Gugger, P. F., Lynch, A. M., Guiterman, C. H., Fowler, J. C., Klesse, S., et al. (2018). Dendroecology meets genomics in the common garden: new insights into climate adaptation. New Phytol. 218, 401–403. doi: 10.1111/nph.15094
Fasanella, M., Suarez, M. L., Hasbun, R., and Premoli, A. C. (2021). Individual-based dendrogenomic analysis of forest dieback driven by extreme droughts. Can. J. For. Res. 51, 420–432.
Gajardo, R. (2001). Antecedentes sobre el “roble de Santiago” o “roble blanco”(Nothofagus macrocarpa) y sus problemas de conservación. Rev. Bosque Nativ. 28, 3–7.
Garreaud, R. (2021). Con El Estanque (Casi) Vacío y Cuesta Arriba. CR2. Available online at: https://www.cr2.cl/analisis-cr2-con-el-estanque-casi-vacio-y-cuesta-arriba/ (accessed August 10, 2021).
Garreaud, R., Alvarez-Garreton, C., Barichivich, J., Boisier, J. P., Christie, D., Galleguillos, M., et al. (2017). The 2010-2015 mega drought in Central Chile: Impacts on regional hydroclimate and vegetation. Hydrol. Earth Syst. Sci. 21, 6307–6327.
Garreaud, R. D., Boisier, J. P., Rondanelli, R., Montecinos, A., Sepúlveda, H. H., and Veloso-Aguila, D. (2020). The central Chile mega drought (2010–2018): a climate dynamics perspective. Int. J. Climatol. 40, 421–439. doi: 10.1002/joc.6219
Gazol, A., Camarero, J. J., Anderegg, W. R. L., and Vicente-Serrano, S. M. (2017a). Impacts of droughts on the growth resilience of Northern Hemisphere forests. Glob. Ecol. Biogeogr. 26, 166–176. doi: 10.1111/geb.12526
Gazol, A., Camarero, J. J., Vicente-Serrano, S. M., Sánchez-Salguero, R., Gutiérrez, E., de Luis, M., et al. (2018). Forest resilience to drought varies across biomes. Glob. Chang. Biol. 24, 2143–2158. doi: 10.1111/gcb.14082
Gazol, A., Ribas, M., Gutiérrez, E., and Camarero, J. J. (2017b). Aleppo pine forests from across Spain show drought-induced growth decline and partial recovery. Agric. For. Meteorol. 232, 186–194. doi: 10.1016/j.agrformet.2016.08.014
Girardin, M. P., Bouriaud, O., Hogg, E. H., Kurz, W., Zimmermann, N. E., Metsaranta, J. M., et al. (2016). No growth stimulation of Canada’s boreal forest under half-century of combined warming and CO2 fertilization. Proc. Natl. Acad. Sci. U. S. A. 113, E8406–E8414. doi: 10.1073/pnas.1610156113
González-Díaz, P., Gazol, A., Valbuena-Carabaña, M., Sangüesa-Barreda, G., Moreno-Urbano, A., Zavala, M. A., et al. (2020). Remaking a stand: links between genetic diversity and tree growth in expanding Mountain pine populations. For. Ecol. Manage. 472:118244. doi: 10.1016/j.foreco.2020.118244
Goudet, J. (1995). FSTAT A program for IBM PC compatibles to calculate Weir and Cockerham’s (1984) estimators of F-statistics (version 1.2). J. Hered. 86, 485–486. doi: 10.1093/oxfordjournals.jhered.a111627
Granda, E., Gazol, A., and Camarero, J. J. (2018). Functional diversity differently shapes growth resilience to drought for co-existing pine species. J. Veg. Sci. 29, 265–275. doi: 10.1111/jvs.12617
Heer, K., Behringer, D., Piermattei, A., Bässler, C., Brandl, R., Fady, B., et al. (2018). Linking dendroecology and association genetics in natural populations: stress responses archived in tree rings associate with SNP genotypes in silver fir (Abies alba Mill.). Mol. Ecol. 27, 1428–1438. doi: 10.1111/mec.14538
Heer, K., Behringer, D., Piermattei, A., Bassler, C., Fady, B., Jehl, H., et al. (2017). Linking dendroecology and association genetics: stress responses archived in tree rings associate with SNP genotypes in Abies alba (Mill.). bioRxiv, 27, 1428–1438.
Hernández, A., Miranda, M. D., Arellano, E. C., and Dobbs, C. (2016). Landscape trajectories and their effect on fragmentation for a Mediterranean semi-arid ecosystem in Central Chile. J. Arid Environ. 127, 74–81. doi: 10.1016/j.jaridenv.2015.10.004
Holmes, R. L., Adams, R. K., and Fritts, H. C., and Laboratory of Tree-Ring Research U. of A (1986). Tree-Ring Chronologies of Western North America: California, Eastern Oregon and Northern Great Basin with Procedures Used in the Chronology Development Work Including Users Manuals for Computer Programs COFECHA and ARSTAN. Tucson: The University of Arizona. doi: 10.150/304672.
Housset, J. M., Carcaillet, C., Girardin, M. P., Xu, H., Tremblay, F., and Bergeron, Y. (2016). In situ comparison of tree-ring responses to climate and population genetics: the need to control for local climate and site variables. Front. Ecol. Evol. 4:123. doi: 10.3389/fevo.2016.00123
Housset, J. M., Nadeau, S., Isabel, N., Depardieu, C., Duchesne, I., Lenz, P., et al. (2018). Tree rings provide a new class of phenotypes for genetic associations that foster insights into adaptation of conifers to climate change. New Phytol. 218, 630–645. doi: 10.1111/nph.14968
Housset, J. M., Tóth, E. G., Girardin, M. P., Tremblay, F., Motta, R., Bergeron, Y., et al. (2021). Tree-rings, genetics and the environment: complex interactions at the rear edge of species distribution range. Dendrochronologia 69:125863. doi: 10.1016/j.dendro.2021.125863
Hughes, A. R., Inouye, B. D., Johnson, M. T. J., Underwood, N., and Vellend, M. (2008). Ecological consequences of genetic diversity. Ecol. Lett. 11, 609–623. doi: 10.1111/j.1461-0248.2008.01179.x
Johnson, J. S., Chhetri, P. K., Krutovsky, K. V., and Cairns, D. M. (2017). Growth and its relationship to individual genetic diversity of mountain hemlock (Tsuga mertensiana) at alpine treeline in Alaska: combining dendrochronology and genomics. Forests 8:418. doi: 10.3390/f8110418
Jones, R. C., Vaillancourt, R. E., and Jordan, G. J. (2004). Microsatellites for use in Nothofagus cunninghamii (Nothofagaceae) and related species. Mol. Ecol. Notes 4, 14–16.
King, G. M., Gugerli, F., Fonti, P., and Frank, D. C. (2013). Tree growth response along an elevational gradient: climate or genetics? Oecologia 173, 1587–1600. doi: 10.1007/s00442-013-2696-6
Latutrie, M., Mérian, P., Picq, S., Bergeron, Y., and Tremblay, F. (2015). The effects of genetic diversity, climate and defoliation events on trembling aspen growth performance across Canada. Tree Genet. Genomes 11, 1–14.
Le Quesne, C., Stahle, D. W., Cleaveland, M. K., Therrell, M. D., Aravena, J. C., and Barichivich, J. (2006). Ancient Austrocedrus tree-ring chronologies used to reconstruct central Chile precipitation variability from AD 1200 to 2000. J. Clim. 19, 5731–5744.
Linares, J. C., and Tíscar, P. A. (2010). Climate change impacts and vulnerability of the southern populations of Pinus nigra subsp. salzmannii. Tree Physiol. 30, 795–806. doi: 10.1093/treephys/tpq052
Lloret, F., Keeling, E. G., and Sala, A. (2011). Components of tree resilience: effects of successive low-growth episodes in old ponderosa pine forests. Oikos 120, 1909–1920. doi: 10.1111/j.1600-0706.2011.19372.x
Luebert, F., and Pliscoff, P. (2006). Sinopsis Bioclimática y Vegetacional de Chile.2nd Edition, Santiago: Editorial Universitaria.
Marchelli, P., and Gallo, L. (2006). Multiple ice-age refugia in a southern beech of South America as evidenced by chloroplast DNA markers. Conserv. Genet. 7, 591–603. doi: 10.1007/s10592-005-9069-6
Marchelli, P., Caron, H., Azpilicueta, M. M., and Gallo, L. A. (2008). Primer note: a new set of highly polymorphic nuclear microsatellite markers for Nothofagus nervosa and related South American species. Silvae Genet. 57, 82–85. doi: 10.1515/sg-2008-0013
Mathiasen, P., and Premoli, A. C. (2010). Out in the cold: genetic variation of Nothofagus pumilio (Nothofagaceae) provides evidence for latitudinally distinct evolutionary histories in austral South America. Mol. Ecol. 19, 371–385. doi: 10.1111/j.1365-294X.2009.04456.x
Mathiasen, P., Rovere, A. E., and Premoli, A. C. (2007). Genetic structure and early effects of inbreeding in fragmented temperate forests of a self-incompatible tree, Embothrium coccineum. Conserv. Biol. 21, 232–240. doi: 10.1111/j.1523-1739.2006.00565.x
Mathiasen, P., Venegas-González, A., Fresia, P., and Premoli, A. C. (2020). A relic of the past: current genetic patterns of the palaeoendemic tree Nothofagus macrocarpa were shaped by climatic oscillations in central Chile. Ann. Bot. 126, 891–904. doi: 10.1093/aob/mcaa111
Matías, L., Gonzalez-Díaz, P., Quero, J. L., Camarero, J. J., Lloret, F., and Jump, A. S. (2016). Role of geographical provenance in the response of silver fir seedlings to experimental warming and drought. Tree Physiol. 36, 1236–1246. doi: 10.1093/treephys/tpw049
Matskovsky, V., Venegas-González, A., Garreaud, R., Roig, F. A., Gutiérrez, A. G., Muñoz, A. A., et al. (2021). Tree growth decline as a response to projected climate change in the 21st century in Mediterranean mountain forests of Chile. Glob. Planet. Change 198:103406. doi: 10.1016/j.gloplacha.2020.103406
McDowell, N. G., and Allen, C. D. (2015). Darcy’s law predicts widespread forest mortality under climate warming. Nat. Clim. Chang. 5:669.
McDowell, N. G., Allen, C. D., Anderson-Teixeira, K., Aukema, B. H., Bond-Lamberty, B., Chini, L., et al. (2020). Pervasive shifts in forest dynamics in a changing world. Science 8:368. doi: 10.1126/science.aaz9463
Montecinos, A., and Aceituno, P. (2003). Seasonality of the ENSO-related rainfall variability in central Chile and associated circulation anomalies. J. Clim. 16, 281–296. doi: 10.1175/1520-0442(2003)016<0281:soterr>2.0.co;2
Nakagawa, S., and Schielzeth, H. (2013). A general and simple method for obtaining R2 from generalized linear mixed-effects models. Methods Ecol. Evol. 4, 133–142. doi: 10.1093/sysbio/syy060
Nehrbass-Ahles, C., Babst, F., Klesse, S., Nötzli, M., Bouriaud, O., Neukom, R., et al. (2014). The influence of sampling design on tree-ring-based quantification of forest growth. Glob. Chang. Biol. 20, 2867–2885. doi: 10.1111/gcb.12599
Neophytou, C., Weisser, A.-M., Landwehr, D., Šeho, M., Kohnle, U., Ensminger, I., et al. (2016). Assessing the relationship between height growth and molecular genetic variation in Douglas-fir (Pseudotsuga menziesii) provenances. Eur. J. For. Res. 135, 465–481. doi: 10.1007/s10342-016-0946-y
Nikinmaa, L., Lindner, M., Cantarello, E., Jump, A. S., Seidl, R., Winkel, G., et al. (2020). Reviewing the use of resilience concepts in forest sciences. Curr. For. Reports 6, 61–80. doi: 10.1007/s40725-020-00110-x
Noble, D. W. A., Radersma, R., and Uller, T. (2019). Plastic responses to novel environments are biased towards phenotype dimensions with high additive genetic variation. Proc. Natl. Acad. Sci. U. S. A. 116, 13452–13461. doi: 10.1073/pnas.1821066116
Pazzaglia, J., Reusch, T. B. H., Terlizzi, A., Marín-Guirao, L., and Procaccini, G. (2021). Phenotypic plasticity under rapid global changes: the intrinsic force for future seagrasses survival. Evol. Appl. 14, 1181–1201. doi: 10.1111/eva.13212
Peakall, R., and Smouse, P. E. (2006). GENALEX 6: genetic analysis in Excel. Population genetic software for teaching and research. Mol. Ecol. Notes 6, 288–295. doi: 10.1111/j.1471-8286.2005.01155.x
Pérez-de-Lis, G., Olano, J. M., Rozas, V., Rossi, S., Vázquez-Ruiz, R. A., and García-González, I. (2017). Environmental conditions and vascular cambium regulate carbon allocation to xylem growth in deciduous oaks. Funct. Ecol. 31, 592–603. doi: 10.1111/1365-2435.12789
Pinheiro, J., Bates, D., DebRoy, S., and Sarkar, D. (2017). nlme: Linear and Nonlinear Mixed Effects Models Version 3.1-153.
Pompa-García, M., González-Cásares, M., Acosta-Hernández, A. C., Camarero, J. J., and Rodríguez-Catón, M. (2017). Drought Influence over Radial Growth of Mexican Conifers Inhabiting Mesic and Xeric Sites. Forests 8:175. doi: 10.3390/f8050175
Premoli, A. C., Acosta, M. C., Mathiasen, P., and Donoso, C. (2012). Variación genética en Nothofagus (subgénero Nothofagus). Bosque 33, 115–125.
Quintana, J. M., and Aceituno, P. (2012). Changes in the rainfall regime along the extratropical west coast of South America (Chile): 30-43o S. Atmósfera 25, 1–22.
R Core Team (2018). R: A Language and Environment for Statistical Computing. Vienna, Austria: R Foundation for Statistical Computing. Available online at: https://www.r-project.org/
Reed, D. H., and Frankham, R. (2003). Correlation between fitness and genetic diversity. Conserv. Biol. 17, 230–237. doi: 10.1046/j.1523-1739.2003.01236.x
Reusch, T. B. H., Ehlers, A., Hämmerli, A., and Worm, B. (2005). Ecosystem recovery after climatic extremes enhanced by genotypic diversity. Proc. Natl. Acad. Sci. U S A. 102, 2826–2831. doi: 10.1073/pnas.0500008102
Rita, A., Camarero, J. J., Nolè, A., Borghetti, M., Brunetti, M., Pergola, N., et al. (2020). The impact of drought spells on forests depends on site conditions: the case of 2017 summer heat wave in southern Europe. Glob. Chang. Biol. 26, 851–863. doi: 10.1111/gcb.14825
Rodríguez-Quilón, I., Santos-del-Blanco, L., Grivet, D., Jaramillo-Correa, J. P., Majada, J., Vendramin, G. G., et al. (2015). Local effects drive heterozygosity–fitness correlations in an outcrossing long-lived tree. Proc. R. Soc. B. Biol. Sci. 282:20152230. doi: 10.1098/rspb.2015.2230
Sánchez-Salguero, R., Camarero, J. J., Gutiérrez, E., Rouco, F G., Gazol, A., Sangüesa-Barreda, G., et al. (2016). Assessing forest vulnerability to climate warming using a process-based model of tree growth: bad prospects for rear-edges. Glob. Chang. Biol. 23, 2705–2719. doi: 10.1111/gcb.13541
Sánchez-Salguero, R., Camarero, J. J., Rozas, V., Génova, M., Olano, J. M., Arzac, A., et al. (2018). Resist, recover or both? Growth plasticity in response to drought is geographically structured and linked to intraspecific variability in Pinus pinaster. J. Biogeogr. 45, 1126–1139. doi: 10.1111/jbi.13202
Scherson, R. A., Albornoz, A. A., Moreira-Muñoz, A. S., and Urbina-Casanova, R. (2014). Endemicity and evolutionary value: a study of Chilean endemic vascular plant genera. Ecol. Evol. 4, 806–816. doi: 10.1002/ece3.960
Schweingruber, F. H. (1996). Tree rings and environment: dendroecology. Paul Haupt. AG Bern. 138, 743–750 doi: 10.1046/j.1469-8137.1998.00149-8.x
Seager, R., Osborn, T. J., Kushnir, Y., Simpson, I. R., Nakamura, J., and Liu, H. (2019). Climate variability and change of Mediterranean-type climates. J. Clim. 32, 2887–2915. doi: 10.1175/jcli-d-18-0472.1
Serra-Maluquer, X., Mencuccini, M., and Martínez-Vilalta, J. (2018). Changes in tree resistance, recovery and resilience across three successive extreme droughts in the northeast Iberian Peninsula. Oecologia 187, 343–354. doi: 10.1007/s00442-018-4118-2
Soliani, C., Tsuda, Y., Bagnoli, F., Gallo, L. A., Vendramin, G. G., and Marchelli, P. (2015). Halfway encounters: meeting points of colonization routes among the southern beeches Nothofagus pumilio and N. antarctica. Mol. Phylogenet. Evol. 85, 197–207. doi: 10.1016/j.ympev.2015.01.006
Stokes, M. A., and Smiles, T. (1996). An Introduction to Tree-Ring Dating. Tucson: University of Arizona Press.
Tatarinov, F., Rotenberg, E., Maseyk, K., Ogée, J., Klein, T., and Yakir, D. (2016). Resilience to seasonal heat wave episodes in a Mediterranean pine forest. New Phytol. 210, 485–496. doi: 10.1111/nph.13791
Trujillo-Moya, C., George, J.-P., Fluch, S., Geburek, T., Grabner, M., Karanitsch-Ackerl, S., et al. (2018). Drought sensitivity of Norway spruce at the species’ warmest fringe: quantitative and molecular analysis reveals high genetic variation among and within provenances. G3 8, 1225–1245. doi: 10.1534/g3.117.300524
Urrutia-Jalabert, R., Barichivich, J., Rozas, V., Lara, A., Rojas, Y., Bahamondez, C., et al. (2021). Climate response and drought resilience of Nothofagus obliqua secondary forests across a latitudinal gradient in south-central Chile. For. Ecol. Manage. 485:118962. doi: 10.1016/j.foreco.2021.118962
van der Maaten-Theunissen, M., van der Maaten, E., and Bouriaud, O. (2015). pointRes: an R package to analyze pointer years and components of resilience. Dendrochronologia 35, 34–38. doi: 10.1016/j.dendro.2015.05.006
Vazquez, F. M., and Rodríguez, R. A. (1999). A new subspecies and two new combinations of Nothofagus Blume (Nothofagaceae) from Chile. Bot. J. Linn. Soc. 129, 75–83. doi: 10.1111/j.1095-8339.1999.tb00491.x
Venegas-González, A., Roig, F. A., Gutiérrez, A. G., and Tomazello Filho, M. (2018a). Recent radial growth decline in response to increased drought conditions in the northernmost Nothofagus populations from South America. For. Ecol. Manage. 409, 94–104. doi: 10.1016/j.foreco.2017.11.006
Venegas-González, A., Roig, F. A., Peña-Rojas, K., Hadad, M. A., Aguilera-Betti, I., and Muñoz, A. A. (2019). Recent Consequences of Climate Change Have Affected Tree Growth in Distinct Nothofagus Macrocarpa (DC.) FM Vaz & Rodr Age Classes in Central Chile. Forests 10:653. doi: 10.3390/f10080653
Venegas-González, A., Roig, F., Gutiérrez, A. G., Peña-Rojas, K., and Tomazello Filho, M. (2018b). Efecto de la variabilidad climática sobre los patrones de crecimiento y establecimiento de Nothofagus macrocarpa en Chile central. Bosque 39, 81–93. doi: 10.4067/s0717-92002018000100081
Villagrán, C., and Armesto, J. J. (2005). Fitogeografía histórica de la Cordillera de la Costa de Chile. Hist. Biodivers 2005, 99–116.
Villagrán, C. M. (1995). “Quaternary history of the Mediterranean vegetation of Chile,” in Ecology and Biogeography of Mediterranean Ecosystems in Chile, California, and Australia eds M. T. Kalin Arroyo, P. H. Zedler and M.D. Fox (New York, NY: Springer), 3–20. doi: 10.7717/peerj.9517
Vitali, V., Büntgen, U., and Bauhus, J. (2017). Silver fir and Douglas fir are more tolerant to extreme droughts than Norway spruce in south-western Germany. Glob. Chang. Biol. 23, 5108–5119. doi: 10.1111/gcb.13774
Vitasse, Y., Bottero, A., Cailleret, M., Bigler, C., Fonti, P., Gessler, A., et al. (2019). Contrasting resistance and resilience to extreme drought and late spring frost in five major European tree species. Glob. Chang. Biol. 25, 3781–3792. doi: 10.1111/gcb.14803
Keywords: dendroecology, genetic diversity, geographic isolated forests, phenotypic plasticity tree-growth resilience, global change, Chilean forests, Mediterranean-type ecosystems
Citation: Venegas-González A, Gibson-Capintero S, Anholetto-Junior C, Mathiasen P, Premoli AC and Fresia P (2022) Tree-Ring Analysis and Genetic Associations Help to Understand Drought Sensitivity in the Chilean Endemic Forest of Nothofagus macrocarpa. Front. For. Glob. Change 5:762347. doi: 10.3389/ffgc.2022.762347
Received: 21 August 2021; Accepted: 04 January 2022;
Published: 28 February 2022.
Edited by:
Veronica De Micco, University of Naples Federico II, ItalyReviewed by:
Paula Marchelli, CONICET Instituto de Investigaciones Forestales y Agropecuarias Bariloche (IFAB), ArgentinaFeng Chen, Yunnan University, China
Copyright © 2022 Venegas-González, Gibson-Capintero, Anholetto-Junior, Mathiasen, Premoli and Fresia. This is an open-access article distributed under the terms of the Creative Commons Attribution License (CC BY). The use, distribution or reproduction in other forums is permitted, provided the original author(s) and the copyright owner(s) are credited and that the original publication in this journal is cited, in accordance with accepted academic practice. No use, distribution or reproduction is permitted which does not comply with these terms.
*Correspondence: Alejandro Venegas-González, YWxlamFuZHJvLnZlbmVnYXNAdW1heW9yLmNs; Pablo Fresia, cGZyZXNpYUBwYXN0ZXVyLmVkdS51eQ==