- 1Department of Forest Resources Management, Faculty of Forestry, The University of British Columbia, Forest Sciences Centre, Vancouver, BC, Canada
- 2Canadian Wood Fibre Centre, Canadian Forest Service, Natural Resources Canada, Victoria, BC, Canada
- 3Forest Science, Planning and Practices Branch, British Columbia Ministry of Forests, Victoria, BC, Canada
The increasing frequency of severe drought and heat events under climate change is a major cause for concern for forest productivity and survival. One potential solution to improve forest resilience to drought may involve tree removal to reduce competition. To quantify potential benefits of partial cutting systems under drought, we use tree-ring data from a long-term partial cutting experiment in a dry ecosystem in western Canada, composed primarily of mature interior Douglas-fir (Pseudotsuga menziesii var. glauca). We ask: (1) How does tree removal change growth responses to drought and (2) how persistent are effects across multiple drought events? We compare growth responses in 83 trees up to 400 years in age from eight treatment units in a randomized experimental design representing a logging treatment with 25% basal area retention and a control (100% basal area retention). Retention harvesting was conducted in winter of 2002/2003 after a moderate drought in the summer of 2002. The site experienced a more severe drought in 2009 and a drought-heat event in 2015. In 2020, we obtained tree height and diameter data as well as tree increment cores from canopy dominant Douglas-fir, which were used to derive basal area increments. Based on these growth increments, we calculated four indices of resilience to drought. Growth increments of remaining individuals substantially increased after tree removal. Relative to the control, the low retention treatment expressed significantly higher post-drought growth increments compared to growth increments after the drought years of 2002 and 2009, i.e., higher drought recovery. Growth increments in the low retention treatment also had a significantly higher ability to regain pre-drought growth levels after the 2002 and 2009 droughts, i.e., higher drought resilience. However, the treatment did not show higher resilience under a heat-drought event in 2015. Although the treatment effects on drought response indices diminished over time, the absolute growth increments of residual trees remained higher than the control 17 years after harvest, despite repeated droughts. These results suggest that partial cutting on moisture-limited sites provides immediate and medium term benefits for remaining trees in terms of drought resilience, which may help forests adapt to climate change.
Introduction
Forests are increasingly becoming limited by extreme climatic events such as drought and heatwaves in moisture-limited regions (Peng et al., 2011; Hember et al., 2017; Girardin et al., 2021). Droughts can reduce forest health and productivity, and are often a pre-cursor for mortality (DeSoto et al., 2020; Hammond et al., 2022). Even when drought is not the main mortality agent, it can lower resistance to insects and disease, causing mortality indirectly (Hart et al., 2014; Johnstone et al., 2016; Seidl et al., 2017). Recent increases in drought-induced forest mortality (Allen et al., 2015; Choat et al., 2018) are associated with increased carbon release (Brown et al., 2012; Anderegg et al., 2015), loss of ecosystem function and services (Anderegg et al., 2013; Bennett et al., 2015; Clark et al., 2016), and contribute to fire hazards that affect public safety (Adams et al., 2012; Littell et al., 2016; Stephens et al., 2018). Altogether, forests and the services they provide are threatened by an increased frequency and severity of extreme weather and climate events.
To adapt forests to the pace of climate change and extreme events, several complementary silvicultural strategies have to be considered (Nagel et al., 2017; North et al., 2019). Options with potentially immediate and lasting benefits include reducing inter-tree competition by harvesting a proportion of standing trees on lands managed for timber production (Bolte et al., 2009; D’Amato et al., 2013; North et al., 2022). Tree removal approaches could involve variable retention harvesting, which retains a proportion of standing trees after harvest. Thinning also provides opportunities to remove lower-quality trees prior to final harvest or to support continuous-cover forestry (e.g., Tahvonen, 2016). Such partial cutting systems leave a proportion of merchantable trees for future harvests, while also providing a legacy of forest structure that emulates natural disturbances and improve wildlife habitat (Mitchell et al., 2007). Although removing trees reduces standing volume, growth and survival may be improved at the individual tree level (e.g., Bose et al., 2018) and potentially maintain forest health even under extreme droughts and heatwaves.
The benefits of partial cutting systems under drought have been well-documented in the literature. Such tree removal can also confer advantages under drought. Trees may benefit from higher precipitation throughfall (Donner and Running, 1986; Aussenac and Granier, 1988; Simonin et al., 2007) and can endure increased moisture limitation and/or aridity due to lower inter-tree competition for resources (Aussenac, 2000; D’Amato et al., 2013; Bradford and Bell, 2017). Using a network of forest inventory plots in the south-western United States, Bradford and Bell (2017) demonstrated positive correlations between tree mortality, aridity, and basal area for Douglas-fir (Pseudotsuga menziesii), ponderosa pine (Pinus ponderosa), and pinyon pine (Pinus edulis). Reductions in tree density were found to mitigate growth reductions during drought years and improved post-drought radial tree growth, pointing to better tree recovery and longer-term resilience (Laurent et al., 2003; D’Amato et al., 2013; Giuggiola et al., 2013; Sohn et al., 2016a,b; Diaconu et al., 2017). For conifers in particular, tree removal has been shown to improve growth responses and survival of Douglas-fir, ponderosa pine (Vernon et al., 2018), and limber pine (Pinus flexilis) (Millar et al., 2007), and of pine-dominated stands in the Sierra Nevada (Restaino et al., 2019). For Scots pine (Pinus silvestris) in Spain, Sánchez-Salguero et al. (2015) also found that lower competition increased performance under drought. Similarly, for trembling aspen (Populus tremuloides) and white spruce (Picea glauca) mixtures in Saskatchewan, Comeau (2021) showed that drought resilience increased with decreasing basal area per hectare. These results are consistent with a meta-analysis of 23 studies around the globe, indicating that tree removal generally led to positive effects on drought tolerance (Sohn et al., 2016b).
Although tree removal has generally been reported as being beneficial for remaining trees under drought, there is also evidence for adverse effects, pointing to more complex responses. For example, McDowell et al. (2006) found that while thinning improved performance under drought, it also led to stronger reductions in basal area increments in subsequent drought years. This increasing sensitivity to moisture deficits was also reported by Sánchez-Salguero et al. (2015). Tague et al. (2019) demonstrated that thinning-induced increases in tree size for trembling aspen and white spruce caused reductions in net primary productivity when the increased water demand of larger trees could not be met under drought. These authors conclude that the positive effects of thinning on drought tolerance depend on timing and climate conditions in subsequent years.
Quantifying how tree removal impacts growth under climatic extremes can be effectively studied using a hybrid research approach combining observational tree-ring methodologies with experimental manipulations in well-replicated, long-term field trials. Traditionally, these experiments often rely on tree-size metrics only, which provide a cumulative response variable to local environmental conditions over time. However, decades-old field trials can now be re-purposed to answer climate change questions using non-destructive sampling with tree-increment boring (e.g., D’Amato et al., 2013). Since a tree’s response to the environment are recorded in its tree rings, they can be retrospectively assessed to quantify resilience to drought and heatwaves (e.g., McLane et al., 2011). Combining the advantages of experimental designs with tree-ring methodologies can therefore be a powerful approach to quantify the extent to which tree removal improves drought recovery and resilience relative to untreated controls.
The objective of this study is to test the effectiveness of a partial cutting treatment to alleviate growth losses due to drought and heat events in the remaining trees. To meet this objective, we ask two main questions: To what extent does partial cutting mitigate impacts of drought and heat on productivity at the individual-tree level? How persistent are effects across subsequent drought and heat events? We additionally tested whether tree or topographic characteristics modulate growth responses to these events to provide additional guidance during the selective removal of trees. Understanding forest responses to tree removal will help improve predictions of responses to extreme climatic events. This knowledge can guide approaches to mitigate drought-induced forest mortality on 22 million hectares in British Columbia, helping to reduce carbon loss and to sustain ecosystem services for communities that rely on resilient forests.
Materials and methods
Experimental design
This study was overlaid on the Isobel Lake experimental site (Klenner and Arsenault, 2006), which was initially designed to test how varying levels of retention after harvest in dry Douglas-fir ecosystems alter conifer regeneration, understory vegetation composition and productivity, wildfire fuel loads, timber growth and wood quality. Here, we re-purpose this experiment to evaluate how a partial cutting treatment affects individual-tree growth responses to drought. As shown in Figure 1, the trial is located in southern British Columbia (BC), about 20 km north of Kamloops (Latitude: 50.84, Longitude: −120.44). The experiment is located at relatively high elevation (1,210 m above sea level) and the terrain is moderately mountainous. The soil in the area is moderately well drained, and classified as loamy sand with a moderate composition of coarse fragments, estimated at 44% (Young et al., 1992).
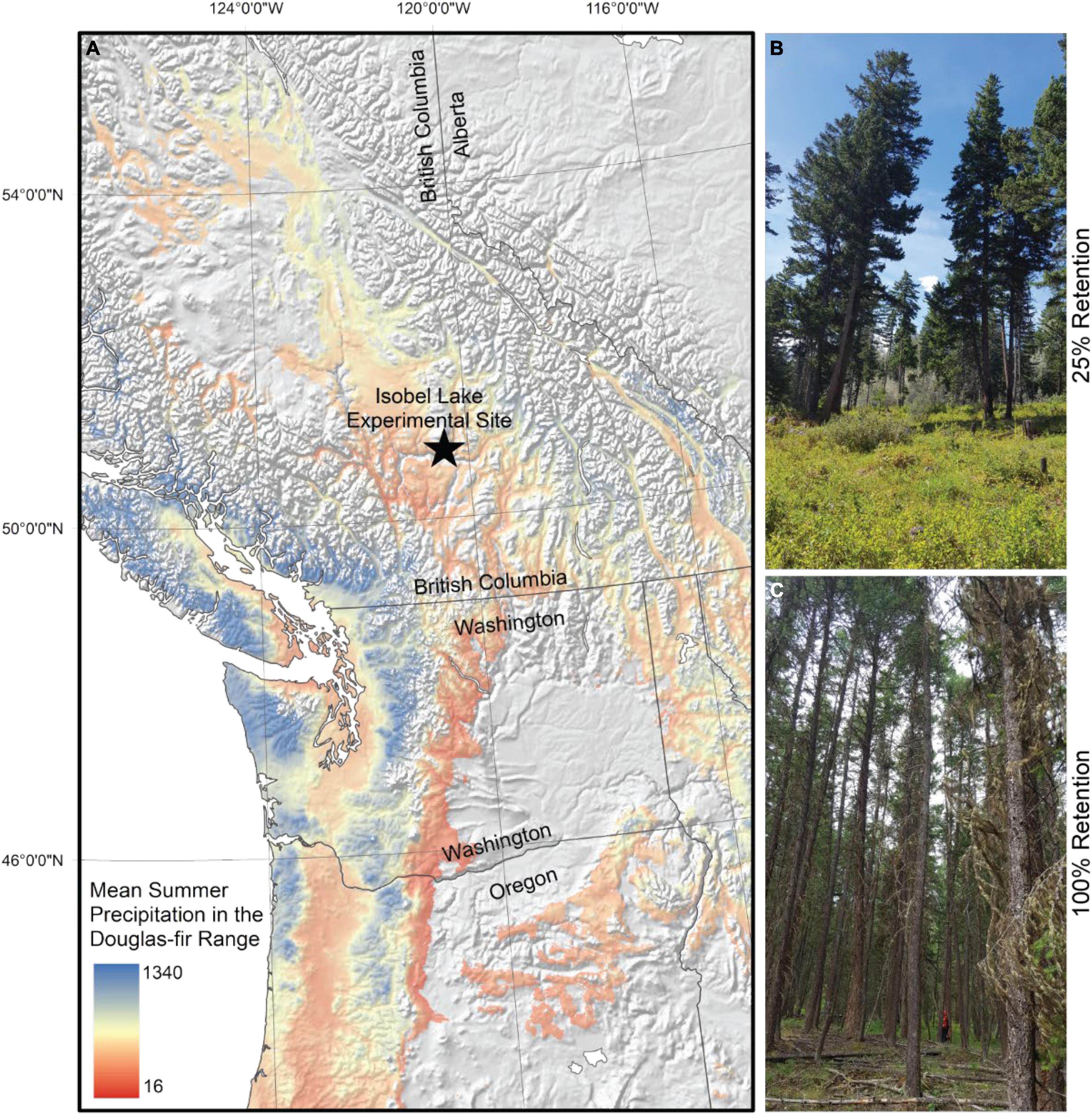
Figure 1. (A) Map of the distribution of Douglas-fir in the Pacific Northwest, shaded by mean annual temperature. The location of the experimental site is indicated by a star. The map was created by the authors using vector and raster data from www.naturalearthdata.com (Public Domain) and (Hamann et al., 2005). (B) Photo taken in treatment unit 9, showing stand conditions 17 years after harvest in the 25% retention treatment. (C) Photo taken in treatment unit 11, showing stand conditions of the no-treatment control 17 years after establishment of the experiment.
The experimental test site covers approximately 250 hectares. Prior to the partial cutting treatments, standing volumes ranged from 175 to 250 m3ha−1 (Klenner and Arsenault, 2006). The trial site was divided into 12 treatment units with an average size of 20 ha. Partial cutting treatments were applied in winter of 2002/2003, representing three levels of harvest retention based on basal area: 20–25% retention, 50% retention, and 100% retention. The partial cutting treatment focused on retaining trees in the dominant and co-dominant crown-classes, and can thus be classified as a strong thinning from below. Each treatment level was randomly assigned to 4 of the 12 treatment units (Klenner and Arsenault, 2006). For this study, we evaluated individual-tree growth responses to drought in the 20–25% retention treatment (R25% for brevity), representing the lowest density in the experiment, and responses in the high-density unharvested control representing 100% retention (R100%).
The ecology of the site can be best described through the province’s Biogeoclimatic Ecosystem Classification (BEC) system, which is a hierarchical system that organizes ecosystems at three levels of integration: local, regional, and chronological. At the broadest unit, BEC zones are defined by areas at the landscape scale characterized by uniform macroclimate. The study site is located in the Interior Douglas-fir (IDF) zone, which is typically dominated by interior Douglas-fir (Pseudotsuga menziesii var. glauca); at lower elevations drought tolerant ponderosa pine (Pinus ponderosa) may co-occur, while at higher elevations lodgepole pine (Pinus contorta) is more common; throughout the IDF pinegrass (Calamagrostis rubescens) is common in the understory (Lloyd et al., 1990). After the BEC zone, which is the most commonly-used BEC unit, subzones are used to further characterize a site by a particular climatic climax plant association on zonal sites (MacKinnon et al., 1992). The study site spans two BEC subzones: upland areas occur in the dry-cool (dk) IDF subzone (IDFdk) and, on lower slope positions, the very hot-dry (xh) IDF subzone (IDFxh). The IDFxh is the warmest and driest forested area within the IDF but both subzones are characterized by relatively long growing seasons where moisture deficits are common (Lloyd et al., 1990).
Site climate and drought years
The site is characterized by a continental climate, with a mean annual temperature of 4.2°C, and an average annual temperature difference of 24.4°C (Wang et al., 2016). The mean coldest month temperature is −8.9°C and the mean warmest month temperature is 15.5°C in summer. The annual precipitation sum of 449 mm is evenly distributed throughout the year: Precipitation is about 106 mm in winter, 95 mm in spring, 132 mm in summer and 116 mm in autumn.
Monthly temperature and precipitation data for the experimental site was extracted with the software ClimateWNA (Wang et al., 2016), covering the period from 1961 to 2018. To further infer drought conditions at the experimental site, we retrieved monthly values of the Standardized Precipitation Evapotranspiration Index (SPEI) from the Global SPEI Database (Vicente-Serrano et al., 2010). This index quantifies drought based on a water balance, and because it is standardized to an average of zero and a standard deviation of one, it enables relative comparisons across time and space. The SPEI can be determined for different time windows. For this study, we chose a 3-month integration window to capture short to mid-term changes in drought conditions.
This study examines three main drought events that occurred in 2002, 2009, and 2015 (Figure 2). The drought in 2002 was moderate according to the SPEI-index. Temperatures were above average in June and July, both representing 0.7 standard deviations above the long-term mean (1961–1990) for those months. Precipitation was lower than average in June, July, August, and September, representing −0.8, −0.9, −0.5, and −0.8 standard deviations, respectively. The drought of 2009 was more severe and prolonged, leading to negative SPEI-index values throughout the growing season (Figure 2). Temperatures in June, July, August, and September represented 0.5, 1.7, 0.9, and 1.4 standard deviations above the 58-year long-term climate average for those months. Precipitation in 2009 was low early in winter and spring, but lowest during the summer months of June, July, and August, with −1.1, −0.5, and −1.2 standard deviations below the mean, respectively.
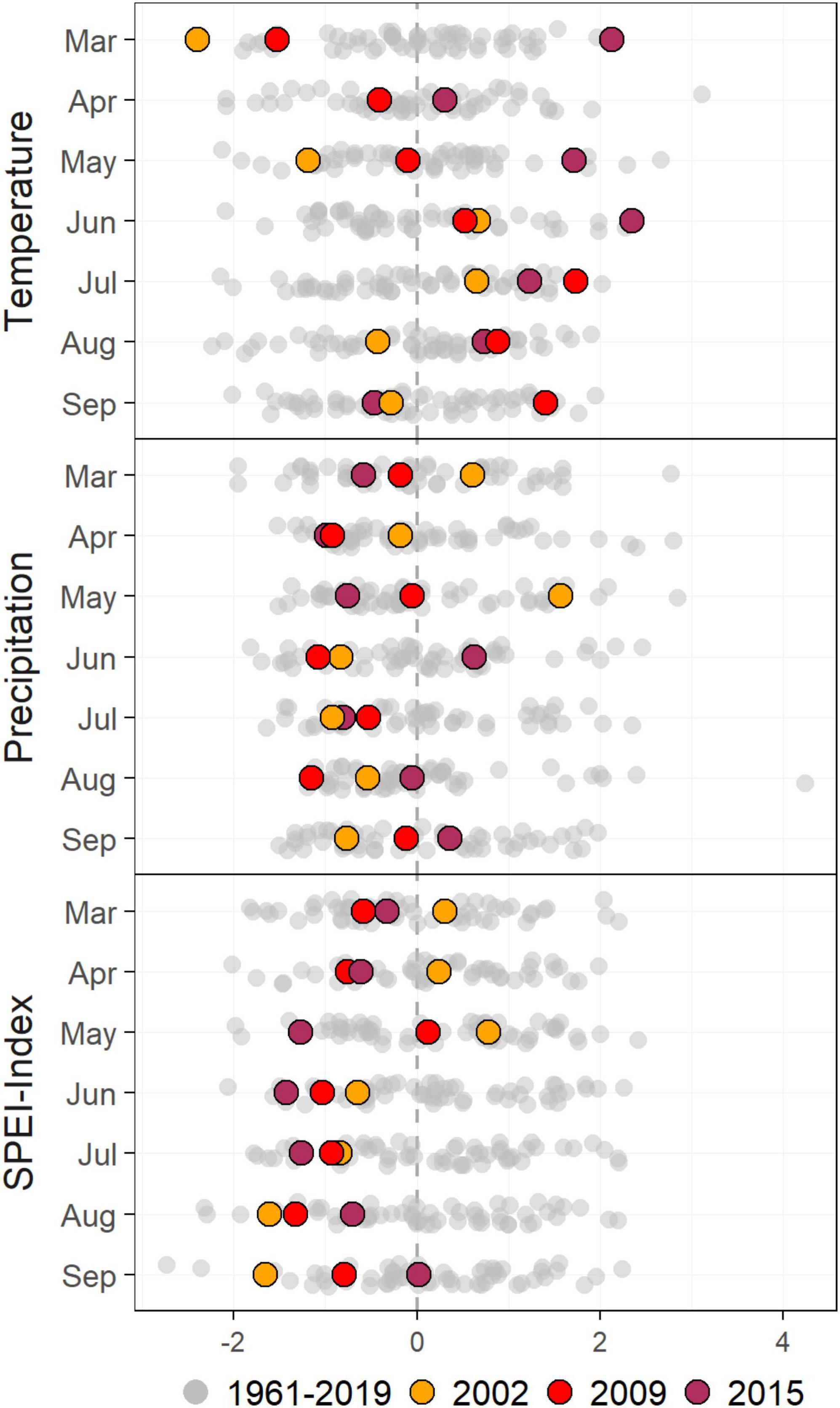
Figure 2. Timeseries for temperature, precipitation, and the Standardized Precipitation-Evaporation Index (SPEI). Each dot represents a value in standard deviations for a particular year from 1961–2019. The drought years shortly before (2002), and after the tree removal treatment (2009 and 2015) are indicated in orange, red, and maroon. All other years are shown in grey.
In contrast, 2015 was characterized by temperatures far above average throughout the year, starting in January and being sustained throughout the growing season: Average temperatures were 1.1, 1.6, 2.1, 0.3, 1.7, 2.4, 1.2, and 0.7 standard deviations above the mean for each month from January to August. The drought of 2015 also builds on warmer-than-average conditions throughout the growing season of 2014, where temperatures were 0.6, 0.2, 1.4, 1.1, 0.6, and 2.5 standard deviations above the mean for each month from May through to October, although precipitation was normal in 2014. In 2015, by contrast, after above-average precipitation in June, precipitation during the summer months were below average. This is reflected in the SPEI-index as well, with negative values throughout the growing season. Therefore, 2015 represents a drought that was exacerbated by an intense heat event.
Although higher-than-average temperature and lower-than-average precipitation was observed in the summer of 2017, an unusually high amount of precipitation as snow was recorded in the preceding months. This was reflected in the SPEI index values of 0.07, −0.59, and −0.77 for June, July, and August, respectively, that did not meet our drought criteria.
Field sampling and tree-ring measurements
In 2020, we obtained tree-size data and tree increment cores from 83 trees from four treatment units of both the control (R100%) and 25% retention treatment (R25%), for a total of eight treatment units. Measured trees were randomly selected from each plot’s dominant or co-dominant crown class. As potential covariates, slope and aspect for each tree was measured with a Haglöf Vertex Laser and a Vertex III hypsometer. Height measurements were obtained using the same instruments. Diameter at breast height (DBH, 1.3 m) was measured with a diameter tape. These tree size and micro-site conditions were relatively similar among treatments (Figure 3), and none differed significantly. GPS coordinates were recorded for each tree, and used to extract the BEC-subzone for each tree. Increment cores were obtained at DBH for each tree using increment borers. The cores were dried, mounted on fluted wooden boards and sanded with progressively fine sandpaper (120–1,800 grit). After polishing, cores were scanned at 2,400 dpi resolution (Canoscan 9000f MarkII, Canon, Tokyo). Tree-ring widths were subsequently measured and cross dated using the software suite Coorecorder and CDendro (version 9.5; Cybis Elektronik & Data AB), following methodology outlined in Maxwell and Larsson (2021).
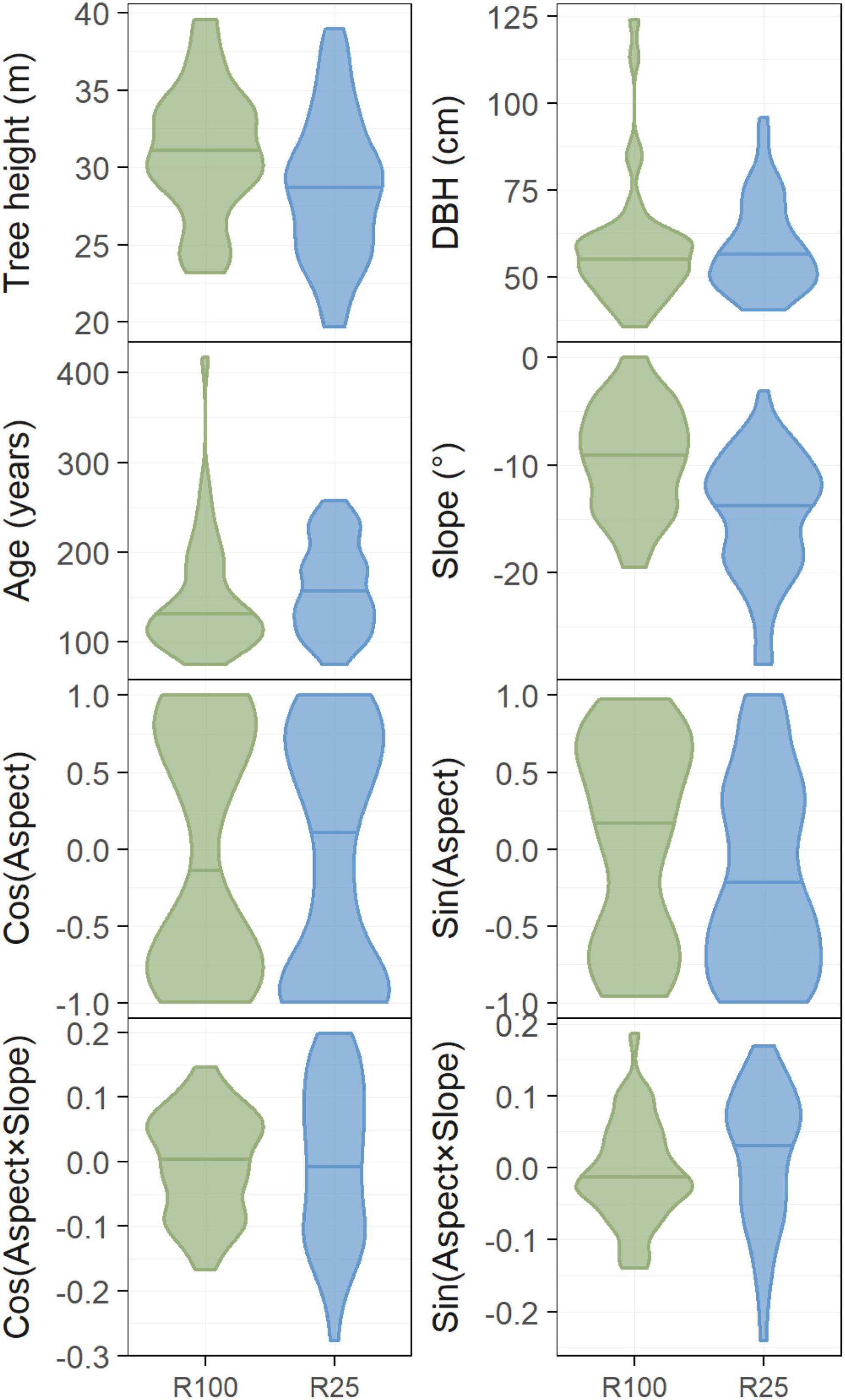
Figure 3. Comparison of differences between treatments in tree height, diameter at breast height (DBH, 1.3 m) and tree age (at 1.3 m, 17 years after treatment), slope (°), the cosine and sine of aspect, as well as the cosine and sine of aspect multiplied by the slope at each tree location. The distribution of values is indicated by the violin plots, and the median is indicated by horizontal lines. No significant differences were found for any of the tree or tree-location variables.
Drought indicators
For each of the three identified drought events, this study assesses drought tolerance using the framework proposed by Lloret et al. (2011), with four indicators: resistance, recovery, resilience, and relative resilience. Resistance describes the reduction in growth rate during a drought event, where a lower growth reduction in the drought year corresponds to higher resistance. Drought recovery represents growth rates after the drought event, relative to growth observed during the drought. Resilience is the ability to regain pre-drought growth levels after the drought, while relative resilience represents an integrated metric combining all three other variables, i.e., the ability to regain pre-drought growth performance weighted by the drop in growth during the drought event. Please see Lloret et al. (2011) for equations and conceptual illustrations of this approach. Indices were calculated on basal area increments (BAI), which were derived from tree-ring widths using the “bai.in” function of the dplR package in R (Bunn, 2008).
All four indicators were defined using a reference period covering a pre- and a post-drought period. The reference period outlined by Lloret et al. (2011) is 5 years (pre- and post-drought), and should capture years of normal growth. A standardized approach to setting the length of the reference periods is lacking (Schwarz et al., 2020). However, the key assumption relating to the length of the reference period is that it should be long enough to provide a reliable indication of growth performance pre- and post-drought. Here, a three-year window was chosen to capture the longest window possible while avoiding overlap among the drought periods. This was important because otherwise the pre- or post-drought periods for each event would have been artificially higher or lower depending on the growth response to previous and subsequent drought events. This would have created a bias in this study and would not have allowed comparison across drought events. For these reasons, a three year pre- and post-drought period was chosen, in agreement with e.g., Gazol et al. (2017), Gazol et al. (2018), Gazol et al. (2020), and Lucas-Borja et al. (2021).
Statistical analysis
The data was analyzed with linear mixed models, using treatment type (two levels) as a fixed effect, and treatment unit (eight levels) as a random effect to account for non-independence of trees within treatment units. The models were computed with the “lmer” function of the lmerTest package (Kuznetsova et al., 2017) in the R programming environment (R Core Team, 2021). To test for treatment differences in basal area increments over time, we specified the interaction between treatment type and year as fixed effects, and treatment unit as well as tree ID as random effects to additionally account for non-independence of years within individual trees. The resulting p-values were adjusted with the Benjamini and Hochberg method (Benjamini and Hochberg, 1995). Because responses of trees can depend on site conditions and tree size (Vernon et al., 2018; Rita et al., 2020), we tested whether including site- and tree-specific variables as covariates would improve model parsimony. To characterize north-south and east-west exposure, aspect was transformed with sine and cosine functions. To explore potential interaction effects, transformed aspect was multiplied by slope. The following covariates were tested using the “drop1” single term deletion function in R: tree height, DBH, age, slope, BEC-subzone, and aspect. However, including these covariates did not improve the model, as determined by AIC. Only the deletion of treatment type from the model resulted in a substantial increase of the AIC (86.50 compared to 82.19 for the full model). The deletion of all other terms resulted in only marginally higher (+0.19 for age) or lower AIC values. Therefore, we continued the analysis with a model including treatment type as sole fixed effect.
Results
Growth response post-treatment and 2002 drought
After the partial cutting treatment in winter of 2002/2003, the basal area increments of trees in the R25% treatment increased substantially (Figure 4). This response is relative to pre-treatment growth levels, where the stands that eventually became the R25% and the R100% treatment units had no significantly different growth rates. The recovery index was the only metric that could be calculated for the 2002 drought given the conflating effect of the actual treatment on growth rates. This increase in individual tree growth in the R25% treatment occurred despite potential legacy effects from the 2002 drought year and was likely heavily influenced by the strong basal area removal (of 75%) as evidenced by the growth release following treatment (Figure 4). In comparison, the basal area increments of trees in the R100% treatment decreased in 2003 after the 2002 drought year. The R25% treatment showed significantly higher recovery, over the three years following the 2002 drought event (Figure 5 and Table 1).
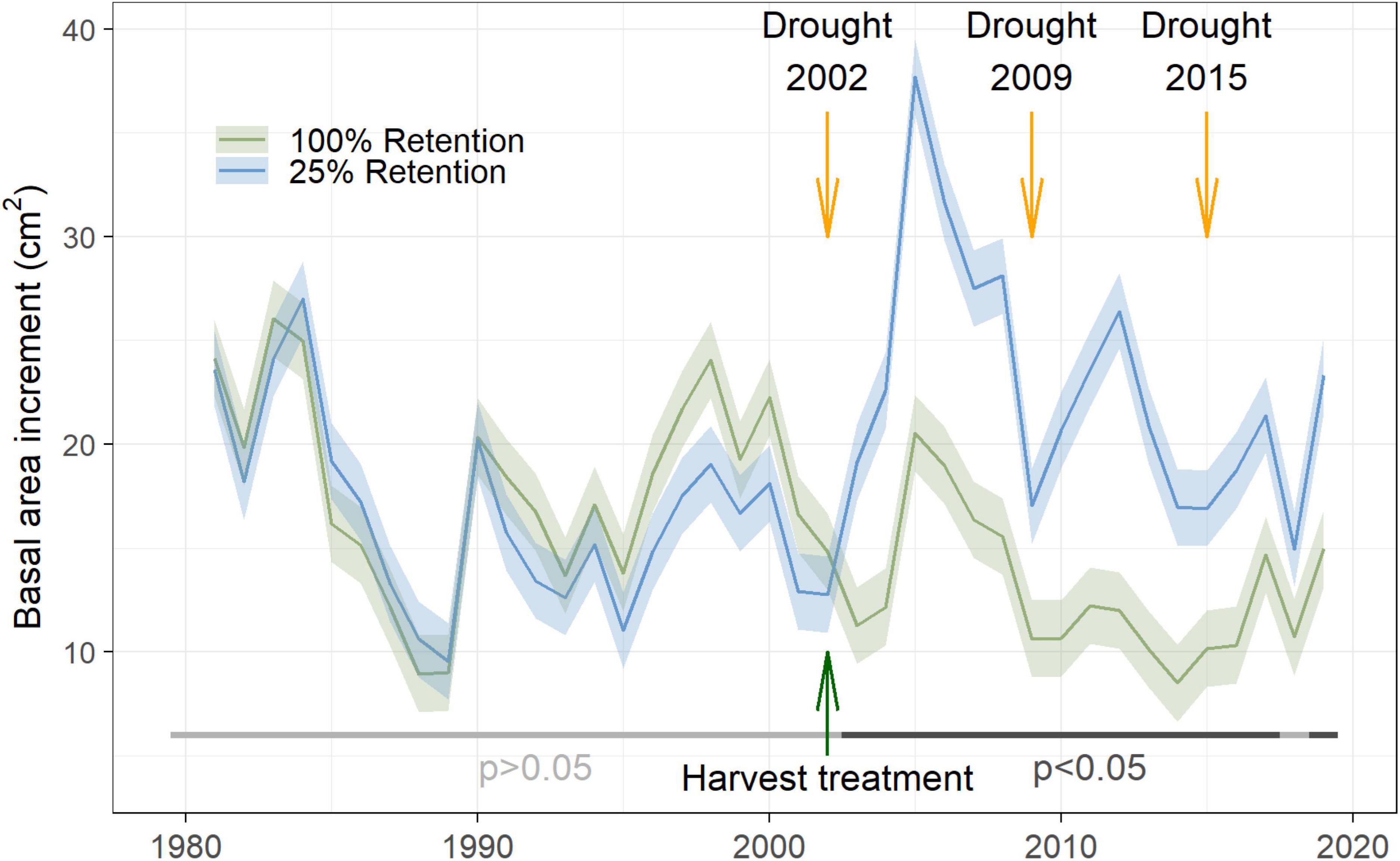
Figure 4. Timeseries of basal area increments for the no-harvest control (100% retention) and the harvest treatment (25% retention) from 1980 to 2019. The standard error of the mean is indicated with shaded ribbons. A green arrow indicates the harvest year in winter of 2002/2003. Three drought years shortly before (2002), and after the tree removal treatment (2009 and 2015) are indicated with orange arrows. Non-significance of differences in basal area increments is shown by a horizontal line in light grey, while a horizontal line shaded in dark grey indicates significant differences.
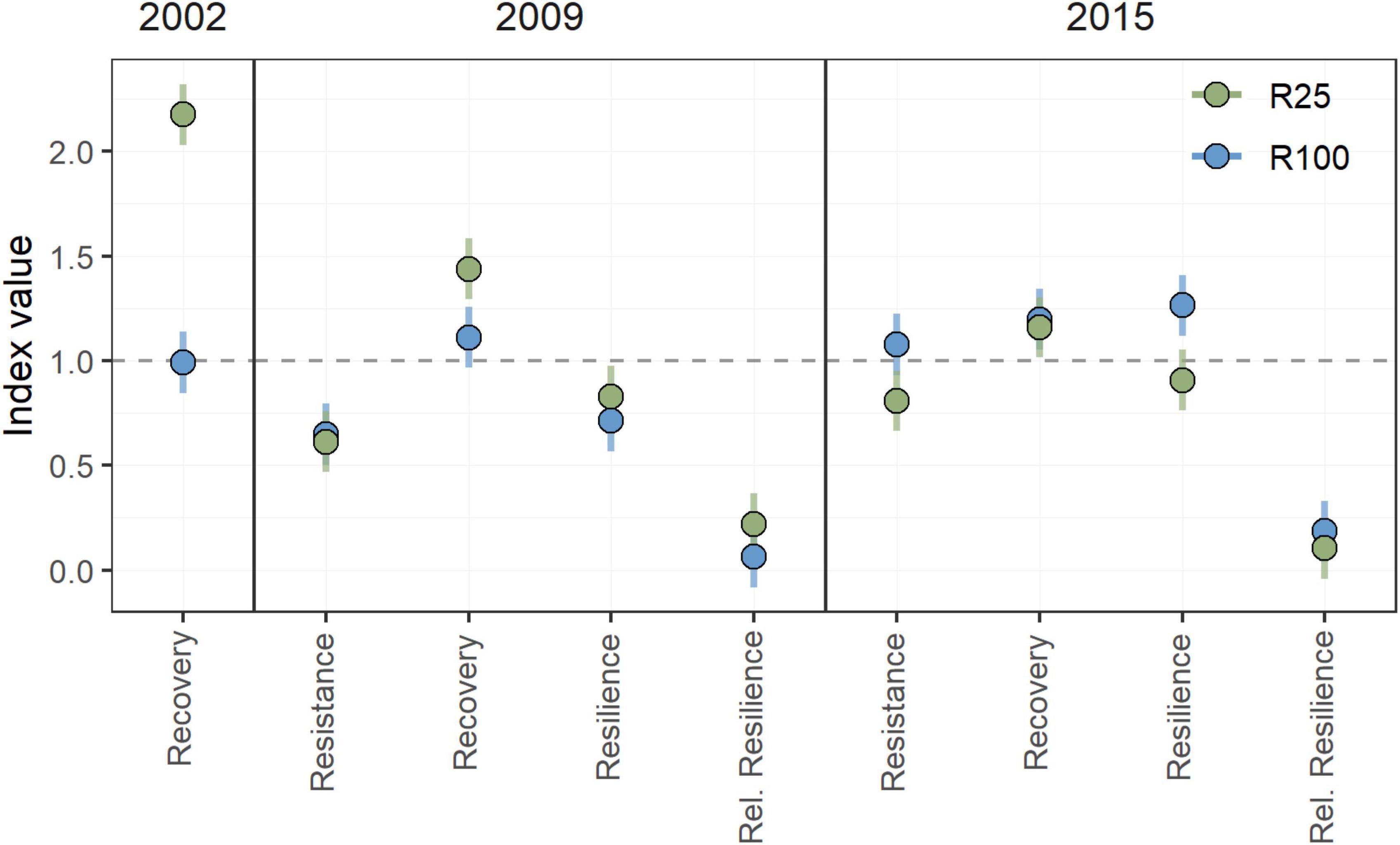
Figure 5. Comparison of drought indicators for the 25% retention treatment (R25) and the no-treatment control (100% retention or R100) for three drought years: 2002, 2009, and 2015. The partial cutting treatment occurred in the winter of 2002/2003. Therefore, only recovery is included for 2002. Error bars indicate the 95th confidence interval.
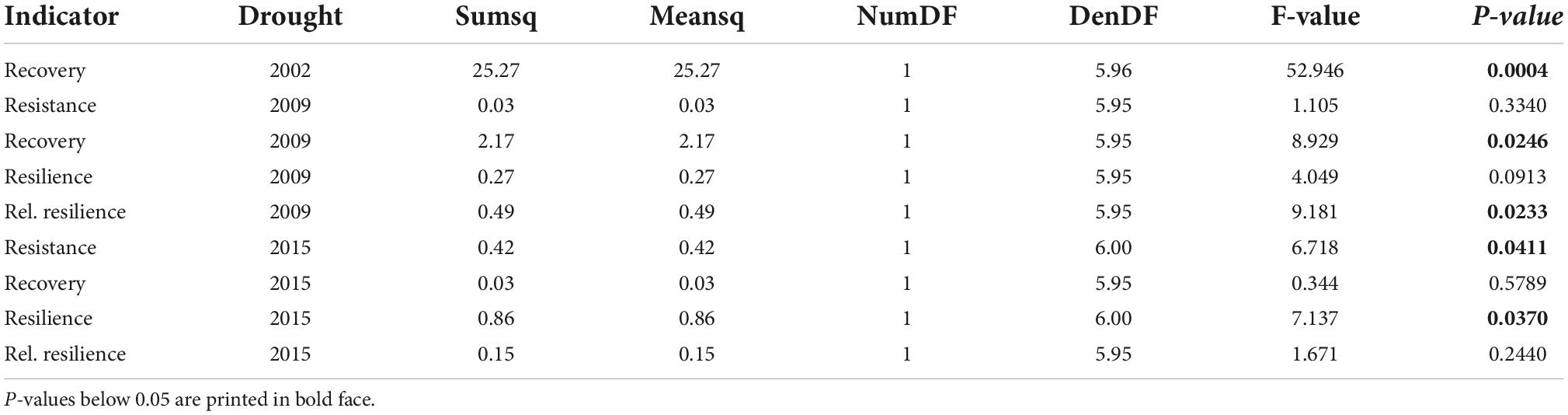
Table 1. Anova table for treatment effects after a mixed model analysis of drought indicator indices. These were calculated as relative changes in basal area increments before, during, and after drought events in 2009, and 2015. Because the partial cutting treatment occurred after the 2002 growing season, only the basal area increments during and after the drought of 2002 were used to calculate the recovery index. Tested groups are no-harvest control, and 25% retention of basal area per ha.
Treatment effect under subsequent droughts
The drought of 2009 provided the first opportunity to fully quantify treatment effects on pre- and post-drought effects. During this drought, trees in the R25% treatment expressed a sharp decline in growth rates but growth rebounded strongly in the subsequent year. In contrast, trees in the R100% treatment declined in 2009 but did not rebound in the next year. In terms of the drought resilience metrics, both treatment types showed low resistance and no significant differences were found (Figure 5 and Table 1). In terms of recovery and relative resilience, however, the R25% treatment significantly outperformed the R100% control. Resilience of trees in the R25% treatment was also higher than the R100% group, but this difference was not significant.
Partial cutting treatment under subsequent heat event
During the heat-drought event of 2015, which followed warmer than average conditions throughout 2014, the growth of trees in both treatments declined relative to the pre-drought levels. For this event, the R100% control outperformed the R25% treatment in all four drought tolerance metrics, although only resistance and resilience were significantly different (Table 1). These results appear to be linked to a decline in growth observed in 2014, which more strongly affected the R100% treatment, having the effect of lowering the pre-drought mean of growth increments. With a lower pre-drought average, the drop in 2015 does not appear as deep, which may have led to a larger resistance value. A lower pre-drought average also improves resilience scores. Therefore, we also tested the impact 2014 had on the results by analyzing 2014/2015 as a two-year drought and by shifting the pre-drought reference period to 2011–2013.
However, analyzing 2014 and 2015 as a combined heat-drought event did not result in changes: The trend remained the same with the R25% treatment showing lower tolerance values for all four drought metrics relative to the R100% control. Significance levels changed for resistance, recovery, and relative resilience, but not for resilience, which remained significantly different. The change was relatively small for resistance, shifting p-values from 0.04 for the 2015-only heat event to 0.08 for the 2014/2015 heat-drought event. The p-values for recovery shifted from 0.57 to 0.1, while relative resilience p-values shifted from 0.23 to 0.06.
Despite lower drought indicator values for 2015, trees in the low retention treatment maintained larger basal area increments than trees in the control, even in low growth years (Figures 4, 5).
Discussion
The combination of increasing temperature and moisture limitation, and their resulting indirect effects (e.g., bark beetle outbreaks, wildfire) have altered the health, dynamics, and occurrence of tree species at local to global scales (Van Mantgem et al., 2009; Allen et al., 2010; Clark et al., 2016; Hammond et al., 2022). Taken individually or collectively, these changes are significantly affecting ecological and social resilience and a myriad of goods and services that forests provide (Parmesan et al., 2022). Forest management practices that manipulate stand composition and structure (e.g., species, size, and density) may do so to increase resource values (e.g., increase productivity for timber), however, management interventions can also be used to mitigate the effects of drought at tree and stand levels (Clark et al., 2016).
The strong growth response and better recovery after the drought in 2002, as well as higher resilience to the drought of 2009, indicate that partial cutting can be a viable option to improve drought tolerance in the short-term to mid-term on moisture-limited sites. The environment at this site appear to have been limiting at the individual tree level given the strong post-harvest increase in basal area increments. Drought recovery is expected to be faster in water-limited systems where soil water storage capacity is low, and where trees have been released from strong sub-surface root competition for moisture (Tague et al., 2019). This is consistent with the higher recovery of released trees after 2002 and 2009 found in this study, and indicates that insufficient moisture has been an important limitation of the site.
The strong increase of basal area increments following the partial cutting treatment found in this study is consistent with the published research on growth release after canopy disturbance (Heath and Alfaro, 1990; e.g., Berg et al., 2006; Diaconu et al., 2015; Montoro Girona et al., 2016). For example, in dominant European Beech trees, Diaconu et al. (2015) found a post-thinning increase of ∼44% in basal area increments after removal of about 50% of the basal area. In this study, the post-harvest growth release coincided with a drought in 2002. Therefore, the higher recovery of the R25% treatment must be interpreted with caution as this experiment does not allow for disentangling these effects.
For the 2015 drought, resilience indices were lower in the treatment relative to the control. This could lead to an interpretation that the R25% treatment is less resilient to drought in the long-term, however, a more nuanced interpretation is required. The drought of 2015 was characterized by exceptionally high temperatures. Under a heat event, low canopy cover might have been a disadvantage because of evapotranspiration from ground vegetation (Nilsen et al., 2001), or direct evaporation from the soil surface (Simonin et al., 2007; Sun et al., 2016). For example, pinegrass is abundant in dry southern interior forests in BC, and typically dominates the understory in the IDF zone (Haeussler et al., 1990). The growth characteristics of pinegrass, such as early spring growth initiation, rhizomatous rooting, vigorous regrowth after disturbance, and its substantial soil water use during midsummer (Lopushinsky and Klock, 1990), suggest that this understory plant is a serious competitor for soil moisture in dry to xeric forests. Varying levels of overstory tree density and canopy cover could alter evaporative demand and/or understory plant abundance which in turn could mediate how tree growth responds to drought, heat coinciding with drought, or heatwaves. A reduction in growth benefits under subsequent droughts might also be due to changes occurring over time at the tree level after the partial cutting treatment. For example, trees respond plastically to changing environmental conditions by producing new xylem with properties linked to hydraulic safety or efficiency (Bryukhanova and Fonti, 2013; Montwé et al., 2014; Isaac-Renton et al., 2018). Trees in the partial cutting treatment may therefore have shifted their water conducting xylem structure toward more efficiency at the expense of hydraulic safety. In turn, this may have contributed to the observed lower resilience in the R25% treatment in 2015.
While the four drought indicators imply a decline in treatment efficacy under the 2015 heat-drought event, it is important to note that average basal area increment values of the 25% retention treatment remained higher than the control 17 years later. Most notably, the basal area increments in subsequent drought years (e.g., 2009, 2015) remained higher than the control, indicating overall that trees in the 25% retention treatment are growing more each year compared to control trees. We also note that this study evaluated two binary conditions: a relatively dense uneven-aged forest with no-harvest, versus a partial cutting treatment at 25% basal area retention that focused on leaving large canopy dominants and co-dominants. In the dry interior Douglas-fir zone, such strong thinning treatment are applied to emulate the natural disturbance regime of frequent, stand maintaining fires (Heyerdahl et al., 2012), and to improve wildlife habitat structure (Leclerc et al., 2021). There may be intermediate levels of tree removal that effectively manage for resilience to drought and heat through survival on moisture-limited sites, while also balancing the trade-off with productivity (e.g., volume per hectare). Intermediate levels of retention may provide sufficient release from competition for moisture to improve tolerance to increasing aridity, while also maintaining sufficient canopy cover to provide thermal protection under severe heat events. Thus, there may be an optimal blend of retention levels in moisture limited Douglas-fir forests, and more broadly in dry temperate forest ecosystems.
This study points to areas that would benefit from further research. Broadly, one important area would be identifying treatment levels that increase access to resources without reducing canopy cover to the extent that new stresses emerge. Research in California found that removal of 34% of forest basal area was sufficient to improve drought resilience in dry Douglas-fir stands (Vernon et al., 2018), which may translate well to the dry forests of southern BC. The Isobel Lake Experiment included a third treatment of 50% basal area retention, which was not included in the present study, but would be worthwhile to revisit given the present findings. Evaluating this treatment level in the future may help gauge whether intermediate retention levels suitably buffer against both extreme drought and heat. Applying the same tree-ring approach to all three treatment levels (R25, R50, and R100%) would be particularly valuable due to the record-breaking heatwave and drought that occurred in western North America in early summer of 2021. In addition, using stand mensuration data, neighborhood data of sampled trees, and microsite soil and climate include soil moisture would provide more detailed pictures of the growth dynamics at this site and may lead to predictive models that can be applied in operational partial cutting systems. Ideally this could be complemented with analyses of similar trials in other locations as well. This type of research focus would provide an opportunity to validate and expand on the results reported in this study, furthering our understanding of the direction (increase or decrease) and duration of growth responses to forest management interventions and extreme climate and weather events.
Leveraging long-term silviculture experiments to answer new climate-adaptation questions in forestry is important and furthers our understanding on how forest management activities may facilitate forest resilience to climate change. However, there is also a need to install new trials where testing climate change adaptation and ecosystem resilience are set as primary objectives. Effectively managing forest lands under warmer and more variable climates is becoming increasingly challenging. To guide the management decisions, which will have both immediate and longer-lasting impacts, reliable data from statistically-sound, replicated experimental designs are needed. These could be specifically designed to test forest resilience under climate change, and other disturbances such as wildfire, using varying levels of retention or thinning intensity under different spatial arrangements. Installing new trials to specifically evaluate these treatment scenarios would have the advantage of also assessing the efficacy of silvicultural planning and harvesting and evaluating economic outcomes.
Conclusion
Strong positive growth responses of trees in a partial cutting treatment under drought indicate that tree removal may be an effective short-term approach to improve forest resilience to extreme events. There may be climatic or temporal limitations to such benefits, though, since relative benefits diminished after a heat and drought event 13 years post-harvest. In the context of absolute values, however, growth rates of individual trees in the low retention partial cutting treatment were consistently higher than the control in poor years, and residual trees were also able to benefit more in better years. While it would be worthwhile to further explore the effects of thinning and partial cutting on drought resilience of interior Douglas-fir, this study indicates that partial cutting can provide long-term advantages under climate change.
Data availability statement
The raw data supporting the conclusions of this article will be made available by the authors, without undue reservation.
Author contributions
DM and JA conceptualized the study. DM, MI-R, and JA completed field data and sample collection. DM and AS prepared, measured, and cross-dated tree-ring samples. DM led statistical analyses. DM, MI-R, JA, and AS wrote the manuscript. All authors contributed to the article and approved the submitted version.
Funding
DM acknowledges funding from The University of British Columbia’s Work-Learn Program. MI-R acknowledges funding from the Canadian Wood Fibre Centre and the Canadian Forest Service’s Fibre Solutions program.
Acknowledgments
We thank Walt Klenner for an introduction to the site and to Peter Sprague for assistance in the field. We also thank internal reviewers in our respective organizations as well as two referees for their constructive feedback.
Conflict of interest
The authors declare that the research was conducted in the absence of any commercial or financial relationships that could be construed as a potential conflict of interest.
Publisher’s note
All claims expressed in this article are solely those of the authors and do not necessarily represent those of their affiliated organizations, or those of the publisher, the editors and the reviewers. Any product that may be evaluated in this article, or claim that may be made by its manufacturer, is not guaranteed or endorsed by the publisher.
References
Adams, H. D., Luce, C. H., Breshears, D. D., Allen, C. D., Weiler, M., Hale, V. C., et al. (2012). Ecohydrological consequences of drought- and infestation- triggered tree die-off: Insights and hypotheses. Ecohydrology 5, 145–159. doi: 10.1002/eco.233
Allen, C. D., Breshears, D. D., and McDowell, N. G. (2015). On underestimation of global vulnerability to tree mortality and forest die-off from hotter drought in the Anthropocene. Ecosphere 6:art129. doi: 10.1890/ES15-00203.1
Allen, C. D., Macalady, A. K., Chenchouni, H., Bachelet, D., McDowell, N., Vennetier, M., et al. (2010). A global overview of drought and heat-induced tree mortality reveals emerging climate change risks for forests. For. Ecol. Manag. 259, 660–684. doi: 10.1016/j.foreco.2009.09.001
Anderegg, W. R. L., Hicke, J. A., Fisher, R. A., Allen, C. D., Aukema, J., Bentz, B., et al. (2015). Tree mortality from drought, insects, and their interactions in a changing climate. New Phytol. 208, 674–683. doi: 10.1111/nph.13477
Anderegg, W. R. L., Kane, J. M., and Anderegg, L. D. L. (2013). Consequences of widespread tree mortality triggered by drought and temperature stress. Nat. Clim. Change 3, 30–36. doi: 10.1038/nclimate1635
Aussenac, G. (2000). Interactions between forest stands and microclimate: Ecophysiological aspects and consequences for silviculture. Ann. For. Sci. 57, 287–301. doi: 10.1051/forest:2000119
Aussenac, G., and Granier, A. (1988). Effects of thinning on water stress and growth in Douglas-fir. Can. J. For. Res. 18, 100–105. doi: 10.1139/x88-015
Benjamini, Y., and Hochberg, Y. (1995). Controlling the false discovery rate: A practical and powerful approach to multiple testing. J. Royal Stat. Soc. Series B (Methodological) 57, 289–300. doi: 10.3102/10769986025001060
Bennett, A. C., McDowell, N. G., Allen, C. D., and Anderson-Teixeira, K. J. (2015). Larger trees suffer most during drought in forests worldwide. Nat. Plants 1, 1–5.
Berg, E. E., Henry, J. D., Fastie, C. L., De Volder, A. D., and Matsuoka, S. M. (2006). Spruce beetle outbreaks on the Kenai Peninsula, Alaska, and Kluane National Park and Reserve, Yukon Territory: Relationship to summer temperatures and regional differences in disturbance regimes. For. Ecol. Manag. 227, 219–232. doi: 10.1016/j.foreco.2006.02.038
Bolte, A., Ammer, C., Löf, M., Madsen, P., Nabuurs, G. J., Schall, P., et al. (2009). Adaptive forest management in central Europe: Climate change impacts, strategies and integrative concept. Scand. J. Forest Res. 24, 473–482. doi: 10.1080/02827580903418224
Bose, A. K., Weiskittel, A., Kuehne, C., Wagner, R. G., Turnblom, E., and Burkhart, H. E. (2018). Tree-level growth and survival following commercial thinning of four major softwood species in North America. For. Ecol. Manag. 427, 355–364. doi: 10.1016/j.foreco.2018.06.019
Bradford, J. B., and Bell, D. M. (2017). A window of opportunity for climate-change adaptation: Easing tree mortality by reducing forest basal area. Front. Ecol. Environ. 15, 11–17. doi: 10.1002/fee.1445
Brown, M. G., Black, T. A., Nesic, Z., Fredeen, A. L., Foord, V. N., Spittlehouse, D. L., et al. (2012). The carbon balance of two lodgepole pine stands recovering from mountain pine beetle attack in British Columbia. Agr. For. Meteorol. 153, 82–93. doi: 10.1016/j.agrformet.2011.07.010
Bryukhanova, M., and Fonti, P. (2013). Xylem plasticity allows rapid hydraulic adjustment to annual climatic variability. Trees 27, 485–496. doi: 10.3929/ethz-b-000067358
Bunn, A. G. (2008). A dendrochronology program library in R (dplR). Dendrochronologia 26, 115–124. doi: 10.1016/j.dendro.2008.01.002
Choat, B., Brodribb, T. J., Brodersen, C. R., Duursma, R. A., López, R., and Medlyn, B. E. (2018). Triggers of tree mortality under drought. Nature 558, 531–539.
Clark, J. S., Iverson, L., Woodall, C. W., Allen, C. D., Bell, D. M., Bragg, D. C., et al. (2016). The impacts of increasing drought on forest dynamics, structure, and biodiversity in the United States. Glob. Change Biol. 22, 2329–2352. doi: 10.1111/gcb.13160
Comeau, P. G. (2021). Effects of thinning on dynamics and drought resistance of aspen-white spruce mixtures: Results from two study sites in Saskatchewan. Front. For. Glob. Change 3:621752. doi: 10.3389/ffgc.2020.621752
D’Amato, A. W., Bradford, J. B., Fraver, S., and Palik, B. J. (2013). Effects of thinning on drought vulnerability and climate response in north temperate forest ecosystems. Ecol. Appl. 23, 1735–1742. doi: 10.1890/13-0677.1
DeSoto, L., Cailleret, M., Sterck, F., Jansen, S., Kramer, K., Robert, E. M. R., et al. (2020). Low growth resilience to drought is related to future mortality risk in trees. Nat. Commun. 11:545. doi: 10.1038/s41467-020-14300-5
Diaconu, D., Kahle, H. P., and Spiecker, H. (2017). Thinning increases drought tolerance of European beech: A case study on two forested slopes on opposite sides of a valley. Eur. J. For. Res. 136, 319–328. doi: 10.1007/s10342-017-1033-8
Diaconu, D., Kahle, H.-P., and Spiecker, H. (2015). Tree-and stand-level thinning effects on growth of European beech (Fagus sylvatica L.) on a northeast-and a southwest-facing slope in southwest Germany. Forests 6, 3256–3277. doi: 10.3390/f6093256
Donner, B. L., and Running, S. W. (1986). Water stress response after thinning pinus contorta stands in Montana. For. Sci. 32, 614–625. doi: 10.1093/forestscience/32.3.614
Gazol, A., Camarero, J. J., Sánchez-Salguero, R., Vicente-Serrano, S. M., Serra-Maluquer, X., Gutiérrez, E., et al. (2020). Drought legacies are short, prevail in dry conifer forests and depend on growth variability. J. Ecol. 108, 2473–2484. doi: 10.1111/1365-2745.13435
Gazol, A., Camarero, J. J., Vicente-Serrano, S. M., Sánchez-Salguero, R., Gutiérrez, E., de Luis, M., et al. (2018). Forest resilience to drought varies across biomes. Glob. Change Biol. 24, 2143–2158.
Gazol, A., Camarero, J., Anderegg, W., and Vicente-Serrano, S. (2017). Impacts of droughts on the growth resilience of Northern Hemisphere forests. Glob. Ecol. Biogeogr. 26, 166–176. doi: 10.1111/geb.12526
Girardin, M. P., Guo, X. J., Metsaranta, J., Gervais, D., Campbell, E., Arsenault, A., et al. (2021). A national tree-ring data repository for canadian forests (CFS-TRenD): Structure, synthesis, and applications. Environ. Rev. 29, 225–241. doi: 10.1139/er-2020-0099
Giuggiola, A., Bugmann, H., Zingg, A., Dobbertin, M., and Rigling, A. (2013). Forest Ecology and Management Reduction of stand density increases drought resistance in xeric Scots pine forests. For. Ecol. Manag. 310, 827–835. doi: 10.1016/j.foreco.2013.09.030
Haeussler, S., Coates, D., and Mather, J., British Columbia. Ministry of Forests, Canada-British Columbia Forest Resource, Canada/Bc Economic & Regional Development Agreement Development Agreement, et al. (1990). Autecology of common plants in British Columbia: A literature review. FRDA Research Program, Research Branch, BC Ministry of Forests; Lands. Canada: Forestry Canada, 1990.
Hamann, A., Smets, P., Yanchuk, A. D., and Aitken, S. N. (2005). An ecogeographic framework for in situ conservation of forest trees in British Columbia. Can. J. For. Res. 35, 2553–2561. doi: 10.1139/x05-181
Hammond, W. M., Williams, A. P., Abatzoglou, J. T., Adams, H. D., Klein, T., López, R., et al. (2022). Global field observations of tree die-off reveal hotter-drought fingerprint for Earth’s forests. Nat. Commun. 13, 1–11. doi: 10.1038/s41467-022-29289-2
Hart, S. J., Veblen, T. T., Eisenhart, K. S., Jarvis, D., and Kulakowski, D. (2014). Drought induces spruce beetle (Dendroctonus rufipennis) outbreaks across northwestern Colorado. Ecology 95, 930–939. doi: 10.1890/13-0230.1
Heath, R., and Alfaro, R. I. (1990). Growth response in a Douglas-fir/lodgepole pine stand after thinning of lodgepole pine by the mountain pine beetle: A case study. J. Entomol. Soc. Br. Columbia 87, 16–21.
Hember, R. A., Kurz, W. A., and Coops, N. C. (2017). Increasing net ecosystem biomass production of Canada’s boreal and temperate forests despite decline in dry climates. Glob. Biogeochem. Cy. 31, 134–158. doi: 10.1002/2016GB005459
Heyerdahl, E. K., Lertzman, K., and Wong, C. M. (2012). Mixed-severity fire regimes in dry forests of southern interior British Columbia, Canada. Can. J. Forest Res. 42, 88–98. doi: 10.1139/x11-160
Isaac-Renton, M., Montwé, D., Hamann, A., Spiecker, H., Cherubini, P., and Treydte, K. (2018). Northern forest tree populations are physiologically maladapted to drought. Nat. Commun. 9:5254. doi: 10.1038/s41467-018-07701-0
Johnstone, J. F., Allen, C. D., Franklin, J. F., Frelich, L. E., Harvey, B. J., Higuera, P. E., et al. (2016). Changing disturbance regimes, ecological memory, and forest resilience. Front. Ecol. Environ. 14, 369–378. doi: 10.1002/fee.1311
Klenner, W., and Arsenault, A. (2006). Forest management options for interior dry forest ecosystems: The Opax Mt. and Isobel Research Trials. Extension Note–RSI, 5(10). Kamloops: Southern Interior Forest Region.
Kuznetsova, A., Brockhoff, P. B., and Christensen, R. H. B. (2017). lmerTest Package: Tests in linear mixed effects models. J. Stat. Softw. 82, 1–26. doi: 10.18637/jss.v082.i13
Laurent, M., Antoine, N., and Joël, G. (2003). Effects of different thinning intensities on drought response in norway spruce (Picea abies (L.) Karst.). For. Ecol. Manag. 183, 47–60.
Leclerc, M.-A. F., Daniels, L. D., and Carroll, A. L. (2021). Managing wildlife habitat: Complex interactions with biotic and abiotic disturbances. Front. Ecol. Evol. 9:613371. doi: 10.3389/fevo.2021.613371
Littell, J. S., Peterson, D. L., Riley, K. L., Liu, Y., and Luce, C. H. (2016). A review of the relationships between drought and forest fire in the United States. Glob. Change Biol. 22, 2353–2369. doi: 10.1111/gcb.13275
Lloret, F., Keeling, E. G., and Sala, A. (2011). Components of tree resilience: Effects of successive low-growth episodes in old ponderosa pine forests. Oikos 120, 1909–1920. doi: 10.1111/j.1600-0706.2011.19372.x
Lloyd, D., Angove, K., Hope, G., and Thompson, C. (1990). “A guide to site identification and interpretation for the Kamloops Forest Region,” in Land management handbook-ministry of forests, (British Columbia: British Columbia Forest Service).
Lopushinsky, W., and Klock, G. O. (1990). Soil water use by Ceanothus Velutinus and two grasses, Vol. 496. Portland, OR: Pacific Northwest Research Station, 1990.
Lucas-Borja, M., Andivia, E., Candel-Pérez, D., Linares, J., and Camarero, J. (2021). Long term forest management drives drought resilience in Mediterranean black pine forest. Trees 35, 1651–1662. doi: 10.1007/s00468-021-02143-6
MacKinnon, A., Meidinger, D., and Klinka, K. (1992). Use of the biogeoclimatic ecosystem classification system in British Columbia. Forest. Chron. 68, 100–120. doi: 10.5558/tfc68100-1
Maxwell, R. S., and Larsson, L. A. (2021). Measuring tree-ring widths using the CooRecorder software application. Dendrochronologia 67:125841. doi: 10.1016/j.dendro.2021.125841
McDowell, N. G., Adams, H. D., Bailey, J. D., Hess, M., and Kolb, T. E. (2006). Homeostatic maintenance of ponderosa pine gas exchange. Ecol. Appl. 16, 1164–1182. doi: 10.1890/1051-0761(2006)016[1164:hmoppg]2.0.co;2
McLane, S. C., LeMay, V. M., and Aitken, S. N. (2011). Modeling lodgepole pine radial growth relative to climate and genetics using universal growth-trend response functions. Ecol. Appl. 21, 776–788. doi: 10.1890/10-0131.1
Millar, C. I., Westfall, R. D., and Delany, D. L. (2007). Response of high-elevation limber pine (Pinus flexilis) to multiyear droughts and 20th-century warming, Sierra Nevada, California, USA. Can. J. For. Res. 37, 2508–2520. doi: 10.1139/X07-097
Mitchell, A. K., Koppenaal, R., Goodmanson, G., Benton, R., and Bown, T. (2007). Regenerating montane conifers with variable retention systems in a coastal British Columbia forest: 10-Year results. For. Ecol. Manag. 246, 240–250. doi: 10.1016/j.foreco.2007.04.036
Montoro Girona, M., Morin, H., Lussier, J.-M., and Walsh, D. (2016). Radial growth response of black spruce stands ten years after experimental shelterwoods and seed-tree cuttings in boreal forest. Forests 7:240. doi: 10.3390/f7100240
Montwé, D., Spiecker, H., and Hamann, A. (2014). An experimentally controlled extreme drought in a Norway spruce forest reveals fast hydraulic response and subsequent recovery of growth rates. Trees 28, 891–900. doi: 10.1007/s00468-014-1002-5
Nagel, L. M., Palik, B. J., Battaglia, M. A., D’Amato, A. W., Guldin, J. M., Swanston, C. W., et al. (2017). Adaptive silviculture for climate change: A national experiment in manager-scientist partnerships to apply an adaptation framework. J. For. 115, 167–178. doi: 10.5849/jof.16-039
Nilsen, E. T., Clinton, B. D., Lei, T. T., Miller, O. K., Semones, S. W., and Walker, J. F. (2001). Does Rhododendron maximum L. (Ericaceae) reduce the availability of resources above and belowground for canopy tree seedlings? Am. Midl. Nat. 145, 325–343. doi: 10.1674/0003-00312001145[0325:DRMLER]2.0.CO;2
North, M. P., Stevens, J. T., Greene, D. F., Coppoletta, M., Knapp, E. E., Latimer, A. M., et al. (2019). Tamm Review: Reforestation for resilience in dry western U.S. forests. For. Ecol. Manag. 432, 209–224. doi: 10.1016/j.foreco.2018.09.007
North, M. P., Tompkins, R. E., Bernal, A. A., Collins, B. M., Stephens, S. L., and York, R. A. (2022). Operational resilience in western US frequent-fire forests. For. Ecol. Manag. 507:120004. doi: 10.1016/j.foreco.2021.120004
Parmesan, C., Morecroft, M., Trisurat, Y., Adrian, R., Anshari, G., Arneth, A., et al. (2022). “Terrestrial and freshwater ecosystems and their services,” in Climate Change 2022: Impacts, adaptation and vulnerability. contribution of working group II to the sixth assessment report of the intergovernmental panel on climate change, eds H.-O. Pörtner, D. C. Roberts, M. Tignor, E. S. Poloczanska, K. Mintenbeck, A. Alegría, et al. (Cambridge: Cambridge University Press), 197–377. doi: 10.1017/9781009325844.004
Peng, C., Ma, Z., Lei, X., Zhu, Q., Chen, H., Wang, W., et al. (2011). A drought-induced pervasive increase in tree mortality across Canada’s boreal forests. Nat. Clim. Change 1, 467–471. doi: 10.1038/nclimate1293
R Core Team (2021). R: A language and environment for statistical computing. Vienna: R Foundation for Statistical Computing.
Restaino, C., Young, D. J. N., Estes, B., Gross, S., Wuenschel, A., Meyer, M., et al. (2019). Forest structure and climate mediate drought-induced tree mortality in forests of the Sierra Nevada, USA. Ecol. Appl. 29, 1–14. doi: 10.1002/eap.1902
Rita, A., Camarero, J. J., Nolè, A., Borghetti, M., Brunetti, M., Pergola, N., et al. (2020). The impact of drought spells on forests depends on site conditions: The case of 2017 summer heat wave in southern Europe. Glob. Change Biol. 26, 851–863. doi: 10.1111/gcb.14825
Sánchez-Salguero, R., Linares, J. C., Camarero, J. J., Madrigal-González, J., Hevia, A., Sánchez-Miranda, Á, et al. (2015). Disentangling the effects of competition and climate on individual tree growth: A retrospective and dynamic approach in Scots pine. For. Ecol. Manag. 358, 12–25. doi: 10.1016/j.foreco.2015.08.034
Schwarz, J., Skiadaresis, G., Kohler, M., Kunz, J., Schnabel, F., Vitali, V., et al. (2020). Quantifying growth responses of trees to drought — A critique of commonly used resilience indices and recommendations for future studies. Curr. Forest. Rep. 6, 185–200. doi: 10.1007/s40725-020-00119-2
Seidl, R., Thom, D., Kautz, M., Martin-Benito, D., Peltoniemi, M., Vacchiano, G., et al. (2017). Forest disturbances under climate change. Nat. Clim. Change 7, 395–402. doi: 10.1038/nclimate3303
Simonin, K., Kolb, T. E., Montes-Helu, M., and Koch, G. W. (2007). The influence of thinning on components of stand water balance in a ponderosa pine forest stand during and after extreme drought. Agr. For. Meteorol. 143, 266–276. doi: 10.1016/j.agrformet.2007.01.003
Sohn, J. A., Hartig, F., Kohler, M., Huss, J., and Bauhus, J. (2016a). Heavy and frequent thinning promotes drought adaptation in Pinus sylvestris forests. Ecol. Appl. 26, 2190–2205. doi: 10.1002/eap.1373
Sohn, J. A., Saha, S., and Bauhus, J. (2016b). Potential of forest thinning to mitigate drought stress: A meta-analysis. For. Ecol. Manag. 380, 261–273. doi: 10.1016/j.foreco.2016.07.046
Stephens, S. L., Collins, B. M., Fettig, C. J., Finney, M. A., Hoffman, C. M., Knapp, E. E., et al. (2018). Drought, tree mortality, and wildfire in forests adapted to frequent fire. Bioscience 68, 77–88. doi: 10.1093/biosci/bix146
Sun, X., Onda, Y., Otsuki, K., Kato, H., and Gomi, T. (2016). The effect of strip thinning on forest floor evaporation in a Japanese cypress plantation. Agr. For. Meteorol. 216, 48–57. doi: 10.1016/j.agrformet.2015.10.006
Tague, C. L., Moritz, M., and Hanan, E. (2019). The changing water cycle: The eco-hydrologic impacts of forest density reduction in Mediterranean (seasonally dry) regions. Wiley Interdiscip. Rev. Water 6:e1350. doi: 10.1002/wat2.1350
Tahvonen, O. (2016). Economics of rotation and thinning revisited: The optimality of clearcuts versus continuous cover forestry. For. Policy Econ. 62, 88–94. doi: 10.1016/j.forpol.2015.08.013
Van Mantgem, P. J., Stephenson, N. L., Byrne, J. C., Daniels, L. D., Franklin, J. F., Fulé, P. Z., et al. (2009). Widespread increase of tree mortality rates in the western United States. Science 323, 521–524. doi: 10.1126/science.1165000
Vernon, M. J., Sherriff, R. L., Mantgem, P. v., and Kane, J. M. (2018). Thinning, tree-growth, and resistance to multi-year drought in a mixed-conifer forest of northern California. For. Ecol. Manag. 422, 190–198. doi: 10.1016/j.foreco.2018.03.043
Vicente-Serrano, S. M., Beguerìa, S., and López-Moreno, J. I. (2010). A multiscalar drought index sensitive to global warming: The standardized precipitation evapotranspiration index. J. Climate 23, 1696–1718. doi: 10.1175/2009JCLI2909.1
Wang, T., Hamann, A., Spittlehouse, D., and Carroll, C. (2016). Locally downscaled and spatially customizable climate data for historical and future periods for North America. PLoS One 11:e0156720. doi: 10.1371/journal.pone.0156720
Keywords: heat stress, silviculture, drought, retention harvest, tree rings, climate change adaptation
Citation: Montwé D, Isaac-Renton M, Standish A and Axelson J (2022) Partial cutting in a dry temperate forest ecosystem alleviates growth loss under drought. Front. For. Glob. Change 5:761458. doi: 10.3389/ffgc.2022.761458
Received: 19 August 2021; Accepted: 01 November 2022;
Published: 23 November 2022.
Edited by:
Barry Alan Gardiner, Institut Européen de la Forêt Cultivée (IEFC), FranceReviewed by:
Mark Kimsey, University of Idaho, United StatesDavid M. Bell, USDA Forest Service, United States
Copyright © 2022 Montwé, Isaac-Renton, Standish and Axelson. This is an open-access article distributed under the terms of the Creative Commons Attribution License (CC BY). The use, distribution or reproduction in other forums is permitted, provided the original author(s) and the copyright owner(s) are credited and that the original publication in this journal is cited, in accordance with accepted academic practice. No use, distribution or reproduction is permitted which does not comply with these terms.
*Correspondence: David Montwé, ZGF2aWQubW9udHdlQHViYy5jYQ==