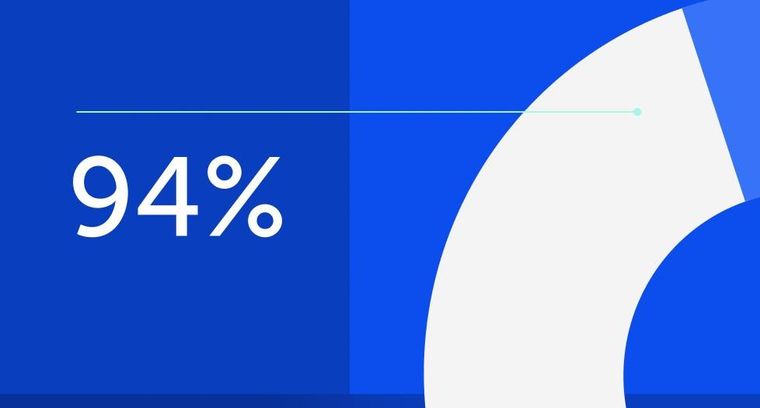
94% of researchers rate our articles as excellent or good
Learn more about the work of our research integrity team to safeguard the quality of each article we publish.
Find out more
ORIGINAL RESEARCH article
Front. For. Glob. Change, 04 May 2022
Sec. Tropical Forests
Volume 5 - 2022 | https://doi.org/10.3389/ffgc.2022.733820
This article is part of the Research TopicDrivers of Mangrove Forest Change and its Effects on Biodiversity and Ecosystem ServicesView all 13 articles
Mangrove vegetation is strongly dependent on the climate, the physicochemical variables of the sediment, and the hydrological dynamics. These drivers regulate the distribution of different mangrove ecotypes and their ecosystem services, so the net sediment accumulation rates in different mangrove ecotypes in Celestun Lagoon, a karstic zone in the NW Yucatan Peninsula, SE Mexico, were estimated. The measurements considering mangrove ecotypes and their spatial variability concerning the lagoon's salinity gradient (inner, middle, and outer lagoon zones) in three climate seasons (dry, rain, and “nortes”) were realized. We registered the structural variables of the forest, interstitial water physicochemical characteristics, and sediment variables that could influence the net sediment deposition. Fringe mangroves are exposed to low hydrodynamism and show the highest sedimentation rate (3.37 ± 0.49 kg m−2 year−1) compared to basin (1.68 ± 0.22 kg m−2 year−1), dwarf (1.27 ± 0.27 kg m−2 year−1), and “peten” (0.52 ± 0.12 kg m−2 year−1) mangroves. The highest sedimentation rate was recorded in the rainy season (0.24 ± 0.08 kg m−2 month−1), while spatially, the highest value was registered in the outer zone (0.44 ± 0.09 kg m−2 month−1). If the extension of each mangrove ecotype is considered, dwarf mangroves have the highest annual sediment accumulation (1,465 t year−1 in 14,706 ha). The structural, physicochemical, and sediment variables of the sites by mangrove ecotype show that dwarf mangroves represent a distinct group from those formed by fringe, basin, and peten mangroves. However, the sedimentation is high in fringe mangroves at the front of the lagoon and diminishes inland where peten mangroves exist. The differences are given by tree density, but salinity, as a proxy variable of the freshwater influence, significantly influences the sedimentation rate. These results indicate that mangroves in karstic environments can have critical roles in confronting climate change, considering water and sediment flows are the basis of sediment accumulation. According to their hydrogeomorphological drivers, conserving, managing, and restoring the mosaic of mangrove ecotypes improves ecosystem services, including mitigation and adaptation to climate change.
Mangroves grow in the sea–land confluence zone of tropical and subtropical regions. This ecosystem shows variability in its vegetation characteristics and adaptations like prop roots and succulent leaves (Naskar and Palit, 2015). Different forest types can be observed according to their vegetation structure, growing in diverse areas with hydrological, physicochemical, and sediment characteristics (Middelburg et al., 1996). The sediment accumulation in mangroves shows patterns (Adame et al., 2010) at spatial and temporal scales related to hydrodynamics (e.g., floods, water flows, precipitation, tides, surges, and storms), which, in turn, control organic and inorganic sediment supplies (Woodroffe et al., 2016), modulated by terrain slopes, topography, and geomorphological features (Twilley and Rivera-Monroy, 2009; Cannon et al., 2020; MacKenzie et al., 2021).
The interactions between these characteristics generate many ecosystem services (Getzner and Islam, 2020). Some of them are related to reducing mangroves and adjacent habitats' vulnerability to climate change impacts, including supporting ecosystem services originating from key ecological processes such as soil formation, nutrient cycling, and primary productivity. These processes are essential for other mangrove ecosystem services, such as wood and food provision (Mitsch et al., 2015).
The sediment capture is one of these critical ecological processes that contribute to a supporting ecosystem service, as the sedimentation in coastal ecosystems is associated with the regulation of tidal flow speed (Kobashi and Mazda, 2005) and the concentrations of suspended sediment and organic matter in the water flowing through mangroves (Kobashi and Mazda, 2005; Adame et al., 2015; Friess and McKee, 2021). There is a direct relationship between features such as tree density and dead trunks and water flow, thus determining sedimentation patterns in mangroves (Mazda et al., 1997; Montgomery et al., 2019).
In response to mesoscale processes related to hydrodynamics and sediment supply, the mangrove trees develop different associations and structures of vegetation that originate diverse mangrove ecological types (mangrove ecotypes): fringe, basin, dwarf, hammock, overwash, and riverine (Lugo and Snedaker, 1974), which show different functioning that may provide ecosystem services at different intensities (Agardy and Alder, 2005).
The mangrove capacity to capture sediments is reflected in the relatively high carbon accumulation observed (5 to 1,722 g C m−2 year−1; MacKenzie et al., 2021). This carbon accumulation in mangrove sediments contributes to other ecosystem services that mangroves provide, such as greenhouse gas regulation, removal of atmospheric CO2, coastal zone protection against sea storms, and sea-level rise through the vertical elevation of soil (Alongi, 2008; McKee, 2011). The accumulation of carbon in the sediment favors an increase in the soil level. This accumulation must be greater than the decomposition–respiration processes for the vertical balance of the soil to be positive. If the capture of C in the soil is not sufficient to overcome subsidence, the site sinks and is vulnerable to sea-level rise, causing flooding and putting the stability of the coastal zone at risk (Spalding et al., 2014). Although the rates of carbon sequestration in sediments are relatively high in the process of vertical soil accumulation, there are other factors involved, such as accretion, compaction, water flows, and the composition and formation of soil by living organisms, as well as deeper processes at the regional level (Lynch et al., 2015), that must also be considered.
The source of the sediments deposited in a determined zone is an essential factor influencing the sediment accumulation rate in different mangrove ecotypes. According to the geomorphological features of mangroves, their sediments can have different origins, so the mineral composition and the size of the particles that settle can be determined. The size of the particles is crucial because it is involved in the dispersion: small particles are usually transported greater distances and settle when the energy decreases; furthermore, the size of the sediment particles is related to the absorption of organic material and therefore to the carbon content at the site.
The use of stable isotopes, mainly carbon and nitrogen, is a valuable tool to determine the transference routes of materials (Adame and Fry, 2016), which is essential to identify the origin and transport routes of organic and inorganic material in sediments. This information allows us to deduce the role of an ecosystem as a sink, transformer, or source of organic material. In the places with relatively low hydrological dynamics (low energy level, small tidal ranges, no surface currents), mangroves are transformers and carbon sinks, which is reflected in high capture rates in the soil. The drivers that influence the accumulation and retention of sediments have been studied little (MacKenzie et al., 2021), but they respond to the influence of the local characteristics of the area. Knowing the sediment sources, such as where they come from, how the sediments support the processes, and functions of the mangrove forest is an essential tool for decision-making on management, considering the local dynamics.
The knowledge of the functions and processes of mangroves is the starting point for adequate management of the ecosystem, including decisions related to conservation and restoration based on these features that result in an essential tool for adaptation and mitigation to climate change. Knowing the factors associated with the processes; for example, what characteristic is responsible for the role of a sink, source, or transformer that a certain mangrove plays, as well as the source of sediments and carbon, helps to spatially and temporally delimit the implementation of actions that achieve conservation and restoration objectives.
However, mangroves are differentially vulnerable to climate change impacts according to variables related to hydrodynamics (Cinco-Castro and Herrera-Silveira, 2020). This hydrodynamic in the coastal zone is modified according to predictions on changes in precipitation patterns and sea-level rise (IPCC, 2021) because the water dynamics at a site depend on what happens in the basin and how much water reaches the coastal area. The predictions related to more or less precipitation then influence the hydroperiod, salinity, redox potential, productivity, and decomposition processes of organic matter in mangroves, which translates into different sedimentation rates.
As sedimentation dynamics in mangroves contribute to conserving these ecosystems for a long time, maintaining and improving their hydrologic and topographic characteristics should be part of management policies (Chow, 2018). These characteristics guarantee ecosystem services at local, regional, and global scales. Based on the above, the objectives of this study were to determine differences in net sedimentation rates in different mangrove ecotypes distributed in a spatial gradient. The gradient is due to salinity in the lagoon and functions as the main stressor in mangrove ecosystems. The temporal variability takes into account distinct climatic seasons. Also, variables that influence sediment capture changes are identified, considering the sediment origin and composition.
The study area is in the mangrove forest in the north of Ria Celestun Biosphere Reserve (RCBR) in the NW Yucatan Peninsula (SE Mexico, Figure 1). The Yucatan Peninsula is geomorphologically formed by Tertiary limestone with high infiltration potential, which causes the absence of surface water but high groundwater flows (Batllori-Sampedro, 1995). The soils are shallow limestone, and topographically, the soil surface slope is less than 1% (SEMARNAT, 2000). These settings create an aquifer with a hydraulic gradient of 7–10 mm km−1 (Rey, 2012). The climate is predominantly dry, with an average annual precipitation of 759 mm but high interannual variability (395–1,239 mm year−1). However, there is a marked seasonal variation, with a dry period from March to May, heavy tropical rainfalls from June to October, and soft rains from November to February. This last season, called the “Nortes” season, represents a meteorological phenomenon that usually appears with rains, wind, and surges that change the hydrologic dynamics in the coastal zone.
Figure 1. Study sites in Celestun (Yucatan, México). Location by lagoon zones (distance among Inner, Middle, and Outer zones is 8 km on average) and by mangrove ecological type (distance among fringe, basin, dwarf and peten mangroves varies from 300 to 1,500 m).
Ria Celestun is a 22-km-long coastal lagoon with an average width of 1.25 km and an average depth of 1.2 m (Acosta-Lugo et al., 2010). This lagoon has a marked spatial salinity gradient that varies with the seasonal meteorological characteristics mentioned above (Herrera-Silveira, 1994) and the sea–lagoon flows, which establish minimum and maximum water residence times between 7 and 63 days, respectively, at the outer and inner parts of the lagoon (Herrera-Silveira and Comin, 1995). The groundwater springs are freshwater inputs with a maximum flow of 7 m3 s−1 during the rainy season and a minimum of 1.2 m3 s−1 in the dry season (SEMARNAT, 2000). The tides are 0.76 m in breadth (Torres-Mota et al., 2014) and they have a propagation efficiency in the aquifer of 39% (Rey, 2012).
Celestun has a great diversity of submerged aquatic vegetation, coastal dunes, mangroves, water bodies, floodplains, and lowland rainforests. The mangrove forests in the reserve polygon cover ~45,000 ha (CONABIO, 2021), and based on vegetation structural characteristics, the following mangrove ecotypes could be observed: fringe, basin, dwarf, and peten. On the Yucatan Peninsula, peten refers to a type of mangrove growing like a vegetation island associated with springs or “ojos de agua” that provide freshwater. These water inputs are characterized by low salinity and high nutrient concentrations, mainly nitrates, favoring less stress and resources available for tree growth. The Peten term is similar to the “hammock” mangrove ecotype described by Lugo and Snedaker (1974) in Florida.
Within the limits of the RCBR, the use of natural resources is made both for self-consumption and for commercial purposes through fishing, salt extraction, and ecotourism projects (SEMARNAT, 2000; Ramsar, 2004). Among the identified threats are mangrove and submerged aquatic vegetation losses, lagoon siltation, and eutrophication; however, altogether, mangroves in Celestun are considered in good condition from an ecological conservation point of view (Herrera-Silveira and Morales-Ojeda, 2010).
A satellite image analysis was carried out to obtain the mangrove extent in the study area. The mage from 1 March, 2020, from 21–45 scene (path and row), referred to Worldwide Reference System, from Landsat 8 OLI with 30 m resolution were downloaded from USGS Earth Explorer. During the preprocessing, an atmospheric correction was applied using the dark object subtraction (DOS) method, one of the most effective atmospheric correction methods (Wicaksono and Hafizt, 2018). The DOS directly transforms the digital values to reflectance, assuming reflectance from dark objects includes the atmospheric scattering (see Supplementary Annex A).
A preliminary visual analysis was carried out using a “false color” composition [Near-Infrared (NIR), red, and green]. For mangroves, five classes were observed according to their reflectance (fringe, basin, dwarf, disperse dwarf, and peten). Additionally, dune vegetation, without vegetation zones, and saline soil areas were identified. The polygonal “training sites” were defined using false-color composition and in situ verification for each class. A supervised classification was carried out using the maximum likelihood algorithm considering bands 2, 3, 4, and 5. The Kappa index (range 0 to 1) and error matrix were obtained to indicate classification accuracy based on classification and reference data differences.
For the monitoring of filed, 16 permanent plots (100 m2 each) representing different mangrove ecotypes, fringe (5), basin (7), dwarf (2), and peten (2), were selected to be studied according to the zone's knowledge. These plots were distributed in a spatial gradient given by salinity in three zones of the east shore of Celestun Lagoon: (a) the inner zone, characterized by freshwater inputs due to numerous springs (North); (b) the middle zone, represents the central point of the lagoon and is a mixing area where freshwater from the inner zone and marine water from outer zone converge; (c) the outer zone (South), located in the coastal lagoon inlet showing the marine influence and generally high salinity to the rest of the lagoon (Figure 1). The differences in permanent plots number among mangrove types are due to fringe and basin mangroves that exhibit a significant variability around the lagoon gradient compared with dwarf and peten mangroves that are usually more homogeneous. The forest structure vegetation was recorded in each plot once, and environmental variables (physical and chemical characterization) were sampled in all the plots in three seasons: (a) dry (March to May), (b) rains (June to October), and (c) “nortes” (November to February).
To determine the forest vegetation structure in each mangrove ecotype, species, height (H), and the diameter at breast height (DBH) in all trees with diameters greater than 2.5 cm were recorded in each plot according to the methods described by Rodríguez-Zúñiga et al. (2018). From these data, basal area (BA, m2 ha−1) and density (D, ind ha−1) were calculated, including seedling density at each site (ind m−2). Seedling density is an indicator of natural regeneration related to mangroves' resilience capabilities (Ellison, 2012).
Five sediment traps were located at the soil surface level in each permanent plot to measure the sedimentation rate in dry weight per area per time. Traps were created using filter paper on 8.2 cm diameter Petri boxes and metal mesh as protection. Wire fasteners were used to fix traps in soil (Supplementary Figure A). The traps were exposed in the field for 30 days in each climate season in 2019: a) dry (from 30 April to 30 May), b) rains (from 23 August to 22 September), and c) “nortes” (5 November to 5 December). Later, the traps were collected and carried to the laboratory. The materials such as leaves, trunks, and unidentified elements were separated, and sediments were dried using an oven at 70°C for 72 h to obtain the dry weight. The sedimentation rates were calculated using dry weight, filter area, and exposure days, according to the following equation:
The sedimentation rate was annually extrapolated by mangrove ecotype as kg m−2 year−1, considering the seasonal rates and the extension of the respective mangrove ecotypes in the RCBR polygon.
The environmental variables influence mangrove structure and provide information about the processes occurring in the ecosystem. These processes can be related to the sedimentation rate so that, in each plot, three samples of interstitial water at 30 cm depth were collected using a syringe and perforated acrylic tubes. The salinity was recorded using an Atago refractometer. The temperature, pH, and redox potential were recorded using an Ultrameter II™ 6PFCE device. Furthermore, in each plot, three aboveground flood levels were recorded, measuring 3 times the water height from the soil surface to the water surface using a 1 m ruler at the same sites as sediment, and environmental variables were recorded.
A surface sediment sample (0–15 cm deep) was collected using a metal nucleator 5.25 cm in diameter in each permanent plot. The samples were characterized and dried at 70°C for 72 h. The measures bulk density (BD), organic matter content, total phosphorous, nitrogen, and carbon content in sediments were measured to determine sediment variables and register how they change spatially and temporally. The BD was obtained from dry weight and sample volume quotient. The organic matter content was determined using the weight difference after sediment calcination at 550°C for 4 h (Chen and Twilley, 1999). The phosphorous content was determined using Strickland and Parsons's (1972) methodology. In sediments, the total nitrogen and carbon (%) were measured in an elemental autoanalyzer model Flash-EA-1112 using 20–30 mg of previously ground and homogenized samples. The organic carbon content was determined from the difference between the total and inorganic carbon determined after the ignition method (Heiri et al., 2001).
Cores of 5.25 cm in diameter and 5-cm deep surface sediment were taken for organic matter isotopic composition analysis to establish the sediment origin in each mangrove type. The isotopic signatures (δ13C, δ15N) were determined after grounding the samples with an agate mortar and were analyzed with an isotope ratio mass spectrometer and an elemental autoanalyzer. The calibration was based on acetanilide. The procedure was performed in duplicate, with 0.2% accuracy in both cases. δ13C and δ15N were calculated using the 13C:12C and 15N:14N relations of the sample (Rx) and standard (Rs) according to the following formula:
A mixed model diagram was used to determine the possible sediment sources, considering δ13C and N:C, and to compare our data in sediments with mangrove leaf values, senescent mangrove leaves, mangrove sediments, C3 plants, and mangrove-associated herbs (Batis maritima and Salicornia virginica). A rearranging isotopic mixing equation was applied (Shultz and Calder, 1976) to determine the autochthonous carbon fraction, comparing terrestrial source (mangrove leaves) and marine (phytoplankton) values with values in our sediment samples:
A modified Shapiro–Wilks normality test determined the sedimentation rate differences between mangrove types, seasons, and lagoon zones. A multifactorial analysis of variance (ANOVA) was run to fulfill this assumption. The same procedure was carried out for structural, interstitial water physicochemical, and sediment variables, including isotopic signatures.
The Similarity Percentages analysis (SIMPER) was carried out to determine the dissimilarity between mangrove ecotypes. The Pearson correlation coefficients were considered to identify correlated variables. A linear discriminate analysis (LDA) was carried out to determine the relationships of structural, physical–chemical, and the sediment characteristics with sedimentation rate, considering only variables that do not correlate between them. All data analyses were carried out using R 3.6.3 and InfoStat 2019.
The study area is a polygon in the north of the RCBR whose terrestrial and marine environments extend 42,739 ha. The image classification accuracy is 91.2% and 0.9058 for the Kappa coefficient. All classes were classified with accuracy more than 80% (Supplementary Figure B). Based on this, mangroves cover 26,133 ha, and the spatial distribution is related to the zone's characteristics, such as hydrology and topography. Fringe mangroves cover 2,735 ha bordering the lagoon and in the coastal zones where mangroves are strongly influenced by the ebb and flow of the tide. Basin mangroves have an extension of 811 ha and are located behind the fringe mangroves, in zones where the topographic profile is usually lower, favoring water accumulation during the ebb and increasing water and soils salinities due to high evaporation. The peten mangroves are identified as the highest vegetation (>15 m) due to freshwater discharges to which they are associated. This mangrove type has an extension of 4,011 ha. Finally, dwarf mangroves are smaller trees (see Results section) because they grow in areas with nutrient limitations. Despite this, dwarf mangroves cover 14,706 ha. However, in Celestun, dwarf mangroves can be associated with other types of wetlands inland (Figure 1).
Mangroves in the Celestun area have structural characteristics of the vegetation that define the ecotype. The data show that the peten mangroves (n = 55) have higher DBH and height (29.8 ± 2.2 cm and 14.8 ± 2.0 m, respectively) than fringe (n = 150, 20.2 ± 1.4 cm, and 11.7 ± 1.3 m) and basin mangroves (n = 327, 16.1 ± 1.2 cm, and 11.1 ± 1.0 m, respectively). The dwarf mangroves (n = 119) are, on average, the smallest (1.8 ± 2.4 cm, 1.67 ± 2.2 m), but they show high tree density (26,444 ± 1,393 ind ha−1), which makes them significantly different from the other types (p < 0.05). Seedling densities vary from 6 to 12 ind m−2; however, the mangrove types are not significantly different when we consider this variable.
According to the lagoon zones, DBH and height were significantly higher in the inner zone (n = 129, 23.80 ± 1.4 cm, and 18.26 ± 0.5 m, respectively) than in the middle (n = 369, DBH: 15.66 ± 1.3 cm, H: 8.59 ± 0.6 m) and outer zones (n = 153, DBH: 13.63 ± 1.4 cm, H: 7.09 ± 0.6 m) (p < 0.0001). However, the density and the basal area were higher in the middle zone (D: 5,481 ± 929 ind ha−1, BA: 73.91 ± 5.8 m2 ha−1) than in the other zones of the lagoon (p < 0.05). The seedling density is higher in the middle zone of the lagoon (12 ind m−2) than in the outer (9 ind m−2) and in the inner zones (7 ind m−2).
The highest sedimentation rate was registered in fringe mangroves (3.37 ± 0.49 kg m−2 year−1), followed by basin and dwarf mangroves (1.68 ± 0.22 kg m−2 year−1 and 1.27 ± 0.27 kg m−2 year−1, respectively). The lowest value was observed in peten mangroves (0.52 ± 0.12 kg m−2 year−1). Therefore, a decreasing pattern in sedimentation rate is related to the spatial distribution of mangrove types, from fringe mangroves at the lagoon edge to peten mangroves located further inland in the study area. In general, the mean sedimentation rate for the mangroves in Celestun is 1.71 ± 0.60 kg m−2 year−1.
In fringe and basin mangroves, the highest sedimentation rate was registered during the rainy season (0.24 ± 0.08 kg m−2 month−1); it decreased during the nortes period (0.19 ± 0.03 kg m−2 month−1), and the lowest value was registered during the dry season (0.04 ± 0.007 kg m−2 month−1) (Table 1). Then, the sedimentation rate shows a decreasing trend as precipitation decreases in these two mangrove ecotypes. However, this pattern is not observed in dwarf and peten mangroves, with the highest sedimentation rates recorded during the nortes season.
Table 1. Sedimentation rate (kg m−2 month−1) in four mangroves ecotypes during three climate seasons.
According to the location of mangroves along the coastal lagoon, mangroves in the inner zone have a sedimentation rate of 0.05 ± 0.007 kg m−2 month−1, followed by middle zone mangroves with 0.06±0.01 kg m−2 month−1. The highest value was registered in the outer zone (0.44 ± 0.09 kg m−2 month−1).
The statistical analysis shows that the most significant effect on the sedimentation rate is given by the position concerning the lagoon (F2,2 3= 14.05, p = 0.0001), and to a lesser extent, by the season (F2,23 = 4.01, p = 0.03) (Figure 2). There exists little interaction between these factors (F4,23 = 3.04, p = 0.04) that influences the sedimentation rate in Celestun mangroves.
Figure 2. Sedimentation rate (kg m−2 month−1) during three seasons (rains, nortes, dry) according to lagoon zones (Outer, Middle, Inner) in Celestun mangroves.
The dwarf mangroves have a more extension (14,706 ha) than other mangrove ecotypes in the study area, thus capturing approximately 1,465 t year−1 of sediment under a conservative approach. However, the fringe mangroves have an extension of 2,735 ha and show the second-highest total sedimentation (923 t year−1), followed in magnitude by peten mangroves (211 t year−1 in 4,011 ha) and basin mangroves, which have 811 ha in extension with a total sedimentation rate of 136 t year−1. The total sediment capture in the mangroves north of the RCBR is estimated at 3,483 t year−1.
The pore–water characteristics showed variability concerning mangrove ecotype, climate season, and lagoon zones. The highest salinity was recorded in the pore water from basin mangroves during the dry season (51.7 ± 17.7 psu), while the lowest salinity was measured in peten mangroves during the nortes season (21.2 ± 5.6 psu). However, there were no differences in pore–water salinity among mangrove ecotypes or seasons (Supplementary Table A). Regarding the location of the mangroves in the lagoon spatial gradient, salinity was significantly different in the inner, middle, and outer zones (F2, 34 = 28.45, p < 0.0001) (Supplementary Table B).
The temperature does not show differences among mangrove ecotypes; however, with values ranging from 26.7 ± 0.7°C in nortes to 30.4 ± 0.7°C in the dry season, the main effect is due to climate seasons (F2, 40 = 99.7, p < 0.0001). Spatially, the temperature does not show differences.
The pH values vary from 6.87 in fringe mangroves to 6.55 in peten mangroves. Therefore, pH decreases from the shore of the lagoon to inland. Considering the zones of the lagoon, the pH values ranged from 6.63 in the inner zone to 6.92 in the outer zone; with these values, the three zones were significantly different (p = 0.0091).
The redox potential remains with similar values among ecological types, varying from −184.39 mV in basin mangroves to −219.59 mV in peten mangroves. The seasonal gradient shows reduced electron conditions in the sediments considering the redox potential and negative values (Supplementary Table A). Concerning the spatial distribution, the redox potential varies from −234.82 in the inner zone to −156.42 in the outer zone (Supplementary Table B).
The flood levels change according to the climate seasons. During the dry season, it was significantly lower than that during the rainfall and nortes seasons (F2, 19 = 3.56, p = 0.0486) (Supplementary Table A). The significant differences are also observed between flood levels, which are higher in the inner than in the middle and outer zones of the lagoon (F2, 25 = 4.66, p <0.0201) (Supplementary Table B).
According to the ecotype, the mangrove surface sediments show differences in BD, organic matter, total phosphorous, total nitrogen, and total carbon content. Although fringe mangrove sediments are denser (0.20 ± 0.16 g cm−3), there are no differences among ecotypes. In most of the cases, more than half of the sediment content was organic matter (52.49 ± 16.55%); however, the highest proportion was found in peten mangroves (79.64 ± 3.53%), with significantly higher values than those of other mangrove ecotypes (F3, 86 = 10.27, p < 0.0001). The total carbon and nitrogen values showed similar differences, significantly higher in peten mangroves than in the other ecotypes (Table 2).
The sediment characteristics vary according to their location around the lagoon. The sediments have a higher BD and lower organic matter (OM) content in the outer zone than in the middle and inner zones, while the highest total nitrogen and carbon contents were recorded in the inner zone (Supplementary Table C).
Regarding the isotopic composition of sediments, δ13C varies from −28.37 to −22.61‰. The dwarf mangroves have significantly higher δ13C (−22.65 ± 0.41‰) than fringe, basin, and peten mangroves. δ15N is significantly higher in peten mangroves (7.85 ± 0.45‰) than in the other mangrove types. The N:C ratio did not present significant differences between mangrove types (Table 2).
The isotopic composition shows differences in δ13C, being higher in the sediment of the outer zone (−26.37 ± 0.09‰) than in the inner and middle zones. The δ15N and N:C ratios did not differ between zones (Supplementary Table C).
According to the δ13C isotopic composition and the mass balance, the carbon source in mangrove sediments is associated with 92% mangrove leaves; most of the sediment components are produced in situ. In fringe, basin, and dwarf mangroves, these proportions are 96, 97, and 90%, respectively, while in peten mangroves, they are more than 99%. Figure 3 shows the isotopic signature of different carbon sources and how mangrove sediment is distributed.
Figure 3. Isotopic signals (δ13C and N:C) in sediments of mangrove ecological types in Celestun. The values of possible sources of carbon and nitrogen are included: Reference data are from mangrove leaves (Wooller et al., 2003; Gonneea et al., 2004; Fry and Cormier, 2011), senescent mangrove leaves (Gonneea et al., 2004), mangrove sediments (Monacci et al., 2011), C3 plants (Adame and Fry, 2016), and mangrove associated herbs (Batis maritima and Salicornia virginica) (Grijalva, 2015).
The SIMPER analyses indicate that dissimilarity (59–99%) between mangrove ecotypes is due to tree density (ind ha−1). According to the Pearson correlation coefficients, the tree density correlates with mangrove structural vegetation characteristics (DBH, height, and basal area) and waterflood level. The physical–chemical variables and salinity have inverse relationships with DBH, height, basal area, and sediment carbon content concerning pore water. The sediment characteristics and OM percentage had an inverse relationship with BD and a direct relationship with total carbon and total nitrogen contents (p < 0.0001). Based on these relationships, the selected variables for LDA were tree density, pore-water salinity, flood level, and sediment OM content. According to these variables and sedimentation rates, the first axis in the discriminant space separates dwarf mangroves (CA1 = −7.65) associated with tree density. In contrast, the second axis shows a gradient of mangrove ecotypes associated with salinity, although with significant overlap between fringe, basin, and peten mangroves (CA2 = −1.11) (Figure 4).
Figure 4. An LDA. The distribution of sampling sites in four ecological types of mangroves (fringe, basin, dwarf, and peten), according to the density of trees, salinity, flood level, organic matter content, and sedimentation rate.
The net sedimentation rate is higher in fringe mangroves than in basin, dwarf, and peten mangroves (Figure 2). This pattern is similar to the values reported by Adame et al. (2010) in Australian mangroves, where they found that geomorphological features influence sedimentation patterns. The geomorphological influence is reflected in the low values of sedimentation rates obtained in our study (0.001–0.59 g cm−2 year−1), performed in the karstic environment of Yucatan Peninsula, and compared with those reported for mangroves growing in a geomorphologic scenario with rivers and tides up to 3 m, such as the Gulf of Papua (1.1–6.5 g cm−2 año−1) (Walsh and Nittrouer, 2004).
The lack of statistical significance among the sedimentation rates of mangrove ecotypes is related to their high seasonal and spatial variabilities observed in different lagoon zones in Celestun. This pattern in sedimentation is associated with flood level, which is minimum in peten mangrove and increases to fringe mangrove (Supplementary Table A). In this karstic ecosystem of the Yucatan Peninsula, groundwater discharges and surface water flow should also be considered because the contribution of groundwater is of such magnitude that it changes the structure and function of mangroves, such as in mangroves of the peten type, structured around a point source of groundwater that emanates and flows toward the coast, transporting nutrients and particles from production and decomposition processes. In the mangroves of Celestun, these particles settle according to the characteristics of slope, energy, tides, and waves; in other words, local hydrology affects the transport and production of particles and how they become part of the sediment (Walsh and Nittrouer, 2004).
Concerning the spatial gradient, mangroves in the inner zone of the lagoon are influenced by freshwater discharges, which is showing a high amount of sediment composed of small-sized particles giving a relatively low BD compared with mangroves in the middle and outer zones (Supplementary Table B). Remarkably, the outer zone has a higher sedimentation rate due to having direct contact with the sea, receiving sandy sediments that usually have higher BD. This zone is directly influenced by the tidal dynamics of the Gulf of Mexico, which has an average tidal range of 35 cm ( UNAM—Servicio Mareográfico Nacional,2016) but may range up to 76 cm on the coast of Celestun Lagoon (Torres-Mota et al., 2014). These characteristics are responsible for the differences in sedimentation rate among lagoon zones. In the outer zone, sandy sediment inputs are strongly related to the relatively intense hydrodynamics in this zone, which is close to the sea. In the middle and inner zones, the hydrodynamics is much lower (Herrera-Silveira and Comin, 1995) because the influence of sea tides does not reach these zones but has a significant freshwater influence from spring inputs. This influence is also reflected in the higher OM content observed in the inner zone (Supplementary Table B).
On the other hand, the measurements performed in different seasons indicate that temporal variability is associated with hydrologic dynamics, with the surface flood water level as an indicator (Supplementary Table A). During the rainy season, the sedimentation rate is generally higher (Table 1) due to relatively higher water flows carrying sediments from inland to coastal zones due to the high freshwater supply and flood level (Zaldívar-Jiménez et al., 2010). However, there is a continuum of events from the rainy season to the nortes seasons. First, the fringe and basin mangroves increase their sedimentation rate, whereas dwarf and peten mangroves do so during the nortes season. This behavior could be related to these mangrove ecotypes' connectivity in the landscape and how the water flows from inland to mangrove fringes. This hydrological connectivity is essential because the water comes from the land within and from the effluents of the peten mangroves, and on a slope, moves through the shallow and basin mangroves until it reaches the fringe mangroves. In this displacement, the salinity and the availability of nutrients that sustain each ecological type and guarantee more environmental services per unit area are modified. For this reason, it is crucial to consider that the hydrological dynamics respond to the frequency and intensity of regional rainfall due to their connection with groundwater discharges of the springs and runoff. During rainy and nortes seasons, the water table rises, and springs in the area show the highest water discharges (Stalker et al., 2014), increasing the Wrunoff and sediments distributed in all ecological types of mangroves.
These water dynamics in the mangrove ecotypes throughout the lagoon areas and variability across the seasonal scale are reflected in the physicochemical characteristics of the pore water, such as salinity, pH, and redox potential (Supplementary Tables A,B), and with nutrient inputs. For example, relatively more acidic conditions occur in inland mangroves, such as dwarfs and petenes, while in the inner zone of the lagoon, the redox potential is more negative. More negative redox potential values mean that the flooding time is probably extensive, and OM degradation occurs in the absence of oxygen. These processes also affect organic carbon stocks registered in different mangrove ecotypes (Cinco-Castro, 2022). On the other hand, acidity is lower in fringe mangroves and the outer zone of the lagoon due to the influence of seawater on this variable. If we add the temporal variability, the results are that during the nortes season, the fringe and basin mangroves show higher pH, which should be related to local hydrology, as seawater inputs and turbulence favored by meteorological phenomena frequency along the coast during this period.
There is a relationship between forest vegetation structure and physicochemical variables of pore water. Salinity shows the same pattern, where it is relatively high in the outer zone due to marine influence and low in the inner zone due to water inputs from springs. Therefore, mangroves in the inner zone and peten show a better structure according to DBH and height (see Results section) than other ecotypes and other zones. This structure could be related to the low salinity in these zones (Supplementary Table A), where freshwater springs with high nitrates are dissolved, reducing vegetation stress and favoring biomass growth (Cintron, 1982; Herrera-Silveira, 1994). Then, the highest tree and seedling densities in the middle zone are due to the mixing characteristics reflecting an environment observed in estuarine conditions, which favors a significant interaction among species compared with the inner and outer zones. This interaction among mangrove species (Rhizophora mangle, Avicennia germinans, and Laguncularia racemosa) favors the observation of higher biomass production (Camacho-Rico, 2018; Bai et al., 2021).
All of the above factors are especially important due to directional relationships in different directions of the ecosystem, as observed among forest vegetation structure, hydrodynamics (flooding time, water sources), and sedimentation rates. These characteristics allow the evaluation of the condition and functioning of the ecosystem and how it could respond to sea-level rise. The areas with more significant sediment accumulation and exposed to constant changes in the tide, such as the outer zone, could be less vulnerable to these impacts from climate change if the sediment accumulation velocity is greater than sea-level rise. Knowing the relationship among ecological processes related to a specific ecosystem service is a pending issue in mangroves, and it must be approached with greater scientific rigor.
Much has been written about the interaction between structure, function, and ecosystem services (Fu et al., 2013); for example, the dynamics of water in the ecosystem is a process that translates into the provision of ecosystem services such as the provision of clean water and irrigation or that indirectly influences food production, the regulation of the microclimate or that favors aesthetics of the site. Something similar occurs with the carbon cycle as an ecological process from which services such as gas and temperature regulation, soil formation, and biodiversity conservation are derived. However, in mangroves, few examples (Lee et al., 2014) show this relationship between ecological processes and ecosystem services using direct measurements, remarking on the importance of this document.
Additionally, it is necessary to remember that sedimentation rates are related to hydrodynamics and that water sources and flows influence local hydrodynamics. However, precipitation, temperature, and cyclone frequency could also be considered sources of variation in hydrologic dynamics, and they explain the forest vegetation structure (Simard et al., 2019) and their variability at different spatial scales. Furthermore, climate change could affect mangrove ecosystems when current precipitation and sea-level rise patterns are modified (IPCC, 2021), modifying the hydrologic and sedimentary dynamics as sediment exportation and accumulation. If the climate and sea-level change, different scenarios of mangroves identified in this study could be changed, endangering their conservation, and consequently, the ecosystem services related to each one.
It is crucial to consider that the sediment accumulation rate measured in this study represents the net rate, and it is necessary to consider how water fluxes change spatially and temporally. The results integrate the exposition of the traps to the field conditions, sediment resuspension, and transport in each zone according to their specific hydrodynamic. This study represents a baseline for tracing sediment accumulation; however, it is necessary to implement monitoring programs that permit us to register the variability of sedimentation rates and their relationship with variables in a long-term context to determine changes that could be alarming and to implement management policies.
The sediment composition is related to the local hydrodynamics and the sources of the materials that form the sediments. In the inner zone of the Lagoon, the sediment is mostly lime, while in the outer zone, it is sandy. The inverse relationship between BD and organic matter (OM) content expresses the spatial heterogeneity of sediment composition in Celestun (Supplementary Tables B,C). The BD of the sediment in Celestun mangroves (0.12–0.20 g cm−3) is lower than that reported for other mangroves (0.7–1.42 g cm−3), but our OM values (45–76%) are higher than those reported from other sites (from 0.65% to 22.89%) (Hossain and Nuruddin, 2016). From this, two issues should be highlighted: (1) the inverse relationship between BD and OM content is evident, and (2) the differences between our data and global data reported in the literature are likely related to the variability of local characteristics. Similar differences are observed contrasting our data of mangroves in the Yucatan Peninsula with the sediment of other Mexican mangroves (BD: 0.9–0.22 g cm−3; OM: from 6.9% in deep layers to 85.8% in superficial layers) (Moreno et al., 2002).
The increasing BD and decreasing OM in the sediment of the mangroves from the inner to the outer zones of the lagoon are also related to the mangrove vegetation structure. The highest OM contribution occurs in the inner zone, where the freshwater inflows are high, and tree height shows the maximum values and structures near the soil level as proper roots and pneumatophores. These structures directly influence sediment accumulation, diminishing the water velocities. Additionally, OM is high in the middle zone due to estuarine water conditions, which favor overlapping different mangrove species (R. mangle, A. germinans, and L. racemosa) and favor litterfall production and OM input to the sediment (Camacho-Rico and Herrera-Silveira, 2015). In summary, the differences in sedimentation rates among the lagoon zones are explained by a smaller size of the sediment particles, and consequently, lower BD and higher OM in the inner zone than in the outer zone, where marine influence and sandy materials dominate.
The δ13C differences among mangrove ecotypes are related to their hydrology. The significantly higher mean value in dwarf mangroves can be related to the C4 plant association, such as Spartina sp. (from −10‰ to 0‰), and due to the accumulation of carbonates in freshwater (from −20‰ to 14‰), which is usually present in this mangrove ecotype (Table 2). Regarding spatial distribution, the inner zone shows low values (−27.52 ± 0.08‰) due to the strong influence of continental organic material as terrestrial plants. The outer zone has the highest values given by marine influence, probably by phytoplankton and seagrasses (Supplementary Table C).
Then, the isotope results show that carbon sources in mangroves, without river influence, are mainly autochthonous, and management strategies could be locally applied. Like the ones in this study that do not receive surface water flows from inland areas, mangroves have productivity mainly stored in the same place where they are produced, so they are functionally a sink of sediments, nutrients, and particulate organic carbon. Therefore, the management strategies must be focused on avoiding wastewater inputs to the mangrove forest because this would modify the nutrient dynamics by changing the ecosystem's structure due to variations in salinity and hydroperiod, for example. Additionally, logging and land-use change should be avoided because the carbon that accumulates and forms part of the sediments would no longer have its origin in the coastal ecosystem. Local management of autochthonous material is essential due to its relationship with blue carbon stocks (Saintilan et al., 2013).
According to isotope results, δ15N is a good indicator of pollution. High δ15N (7.85 ± 0.45‰) in peten mangroves indicates anthropic nitrogen inputs (Bergamino et al., 2017) through sinkholes or springs associated with this mangrove ecotype. According to the spatial distribution, the inner and middle zones showed similar δ15N values, indicating that these sites were related to nitrogen inputs with anthropic origins, so the water that reaches the mangroves has had contact with or comes from human activities. This information highlights the influence of local management of ecosystems and the importance of management with a basin approach for conserving mangroves, which is challenging to implement in a karst environment where the sources and flows of water are underground, and they present connectivity at different spatial scales.
In another order of ideas, accretion in Celestun fringe mangroves is 2.91 mm year−1 (Cinco-Castro and Herrera-Silveira, 2020), while the sea-level rise in the Gulf of Mexico is 3.13 mm year−1 [National Oceanic and Atmospheric Administration (NOAA), 2016]. In this sense, the coastal area of the Yucatan Peninsula has changed since the Pleistocene. Among the main changes is the formation of ripples on the coastline that currently represent coastal wetlands with deposits of carbonate sediments from the quaternary (Bautista et al., 2005) that have continued to accumulate to the present day, conferring to coastal ecosystems their capacity to cope with sea-level rise (Kumara et al., 2010).
The results in this study support the hypothesis that net sedimentation rates in mangroves are higher in zones with high hydrodynamism than in quiet zones. Therefore, restoring hydrodynamics is a significant action to stimulate sediment accretion and recovery of mangrove ecotypes that are better adapted to the influence of high hydrodynamism as fringe mangroves (Teutli-Hernández et al., 2020). However, organic carbon accumulation is also related to the origin and accumulation of OM in the sediments. The OM is higher where hydrodynamics is lowest and markedly contributes to soil formation, such as in the inner zone. In addition, other ecological functions and ecosystem services can be provided differentially by different mangrove ecotypes in a territory (Himes-Cornell et al., 2018). Therefore, mangrove types must be considered in mangrove management as part of the mosaic with a landscape approach since this heterogeneity adds richness and stability to the ecosystem.
At the interecosystem scale (water body–mangrove), the seasonal differences between seawater and freshwater flow determine the role of different mangrove ecotypes. This information can be helpful to establish mitigation and adaptation strategies in front of climate change and particularly to understand how mangroves from karstic regions could adjust to sea-level rise. The dwarf and peten mangroves may be essential in mitigating climate change due to their high organic carbon accumulation. The fringe and basin mangroves have a more efficient role in buffering sea-level rise and sea storm effects because of their higher sedimentation rates. According to the above, the management strategy should be oriented toward conserving the mosaic of mangrove ecotypes that form a heterogeneous landscape that functions as a continuum under hydrogeomorphological drivers (Twilley and Rivera-Monroy, 2009). Actions that favor sediment supply, water flows, and healthy forest vegetation structure should focus on improving, recovering, and even maintaining vertical soil accretion as a proxy of soil formation as a key ecological process for providing ecosystem services.
Mangroves in karstic zones have a lower sedimentation rate than mangroves submitted to other conditions, reflecting the importance of hydrodynamism on sedimentation patterns. Spatial and seasonal hydrological dynamics define the changes in the sedimentation rate of mangroves around Celestun Lagoon. Mangroves close to the coastal zone submitted to high hydrodynamism accumulate sand material with low organic content at high rates, thus protecting against sea storms. Mangroves in the inner parts of the coastal zone show lower sediment accumulation rates but with high organic content, thus contributing to buffering climate change in the long term. The spatial differences observed in the sedimentation rates and the provision of related ecosystem services should be considered to manage a sustainable and desirable mosaic of ecological types of mangroves in karstic zones.
The raw data supporting the conclusions of this article will be made available by the authors, without undue reservation.
SC-C carried out field and laboratory work, analyzed data, prepared figures and/or tables, and authored and reviewed drafts of the article. JH-S and FC authored or reviewed drafts of the article and approved the final draft. All authors contributed to the article and approved the submitted version.
Fieldwork, materials, and laboratory analysis of this research were funded by the National Council for Science and Technology (CONACYT) through project CB-2015-I0017/254175 and by Primary Production Laboratory - CINVESTAV Merida Unit. The CONACYT awarded a Ph.D. scholarship to SC-C (CVU: 627399).
The authors declare that the research was conducted in the absence of any commercial or financial relationships that could be construed as a potential conflict of interest.
All claims expressed in this article are solely those of the authors and do not necessarily represent those of their affiliated organizations, or those of the publisher, the editors and the reviewers. Any product that may be evaluated in this article, or claim that may be made by its manufacturer, is not guaranteed or endorsed by the publisher.
Thanks to the National Council of Science and Technology (CONACYT) for the scholarship awarded (CVU: 627399) and for its contribution to the Project: I0017. Thanks to the National Commission of Natural Protected Areas (CONANP) awarded for fieldwork. The Primary Production Laboratory (LPP) staff of the Marine Resources Department at CINVESTAV-IPN (Merida Unit) is acknowledged for their fieldwork and sample processing support. Special thanks are given to Héctor Hernández Núñez of the Remote Sensing and Geographic Information Systems Laboratory in CINVESTAV-IPN for their support in spatial analysis.
The Supplementary Material for this article can be found online at: https://www.frontiersin.org/articles/10.3389/ffgc.2022.733820/full#supplementary-material
Acosta-Lugo, E., Alonzo-Parra, D., Andrade-Hernández, M., Castillo-Tzab, D., Chablé- Santo, J., Durán, R., et al. (2010). Plan de Conservación de la Eco-región Petenes-Celestún-Palmar. Yucatán: Universidad Autónoma de Campeche, Pronatura Península de Yucatán. A.C. p. 184.
Adame, M. F., and Fry, B. (2016). Source and stability of soil carbon in mangrove and freshwater wetlands of the Mexican Pacific coast. Wetlands Ecol. Manag. 24, 129–137. doi: 10.1007/s11273-015-9475-6
Adame, M. F., Hermoso, V., Perhans, K., Lovelock, C. E., and Herrera-Silveira, J. A. (2015). Selecting cost-effective areas for restoration of ecosystem services. Conserv. Biol. 29, 493–502. doi: 10.1111/cobi.12391
Adame, M. F., Neil, D., Wright, S. F., and Lovelock, C. E. (2010). Sedimentation within and among mangrove forests along a gradient of geomorphological settings. Estuar. Coast. Shelf Sci. 86, 1. doi: 10.1016/j.ecss.2009.10.013
Agardy, T., and Alder, J. (Ed.). (2005). “Coastal systems,” in: Ecosystems and Human Well-being: Current State and Trends, Volume 1. Findings of the Condition and Trends Working Group of the Millennium Ecosystem Assessment. Millennium Ecosystem Assessment Series, ed Hassan, R. et al. Available online at: http://www.vliz.be/imisdocs/publications/ocrd/224618.pdf (accessed April 13, 2021).
Alongi, D. (2008). Mangrove forest: Resilience, protection from tsunamis, and responses to global climate change. Estuar. Coast. Shelf Sci. 76, 1–13. doi: 10.1016/j.ecss.2007.08.024
Bai, J., Meng, Y., Gou, R., Jiacheng, L., Zheng, D., Xiaoping, D., et al. (2021). Mangrove diversity enhances plant biomass production and carbon storage in Hainan island, China. Funct. Ecol. 2021:1–13. doi: 10.1111/1365-2435.13753
Batllori-Sampedro, E. (1995). “Problemática ambiental generada por el desarrollo costero de Yucatán,” in: Procesos territoriales de Yucatán. Coord. G. M. Peraza. Mérida: Editorial Universidad Autónoma de Yucatán.
Bautista, F., Palacio-Apontee, G., Ortíz-Pérez, M., Batllori-Sampedro, E., and Castillo-González, M. (2005). “El origen y el manejo maya de las geoformas, suelos y aguas en la Península de Yucatán,” in Caracterización y Manejo de los Suelos de la Península de Yucatán: Implicaciones Agropecuarias, Forestales y Ambientales, ed F. Bautista and G. Palacio (Yucatan: Universidad Autónoma de Campeche, Universidad Autónoma de Yucatán, Instituto Nacional de Ecología), 1–12.
Bergamino, L., Tudurí, A., Bueno, C., Brugnoli, E., Valenzuela, L. O., Martínez, A., et al. (2017). Aplicación de isótopos estables como indicadores de flujos de energía en ambientes costeros de Uruguay. Rev. Lab. Tecnol. Uruguay 13:09–18. doi: 10.26461/13.01
Camacho-Rico, A. (2018). Dinamica de intercambio de carbono y nutrientes entre el manglar y la Laguna costera de Celestun, Yucatan. Thesis, Cinvestav-Merida
Camacho-Rico, A., and Herrera-Silveira, J. A. (2015). “Dinámica de hojarasca y variación espacio temporal de carbono en un escenario cárstico como laguna de Celestún, Yucatán,” in Estado Actual del Conocimiento del Ciclo del Carbono y sus Interacciones en México: Síntesis a 2014. Serie Síntesis Nacionales, eds F. Paz and J. Wong. Texcoco, Estado de México: Programa Mexicano del Carbono en colaboración con el Centro de Investigación y Estudios Avanzados del Instituto Politécnico Nacional, Unidad Mérida y el Centro de Investigación y Asistencia en Tecnología y Diseño del Estado de Jalisco.
Cannon, D., Roddenberry, A., Kibler, K, Donnelly, M., McClenachan, G., Walters, L., et al. (2020). Hydrodynamic habitat thresholds for mangrove vegetation on the shorelines of a microtidal estuarine lagoon. Ecol. Eng. 158, 106070. doi: 10.1016/j.ecoleng.2020.106070
Chen, R., and Twilley, R. R. (1999). Pattern of mangrove forest structure and soil nutrient dynamics along the Shark River Estuary, Florida. Estuaries 22, 955–970. doi: 10.2307/1353075
Chow, J. (2018). Mangrove management for climate change adaptation and sustainable development in coastal zones. J. Sustain. For. 37, 139–156, doi: 10.1080/10549811.2017.1339615
Cinco-Castro, S. (2022). Servicios ecosistémicos de los manglares en la Península de Yucatán. Tesis doctoral. Centro de Investigación y de Estudios Avanzados del IPN (CINVESTAV-Mérida), Yuc., Méx.
Cinco-Castro, S., and Herrera-Silveira, J. A. (2020). Vulnerability of mangrove ecosystems to climate change effects: the case of the Yucatan Peninsula. Ocean Coast. Manag. 192, 105196. doi: 10.1016/j.ocecoaman.2020.105196
Cintron, G. (1982). Mangrove Forests: Ecology and Response to Natural and Man-Induced Stressors. Coral reefs, seagrass beds, and mangroves: Their interaction in the coastal zones of the Caribbean. Workshop. Working paper UNESCO/W.I.L.F.D.U./IOCARIBE.
CONABIO (2021). Distribución de los manglares en México en 2020, escala 1:50,000. México: Comisión Nacional para el Conocimiento y Uso de la Biodiversidad. Available online at: https://www.biodiversidad.gob.mx/monitoreo/smmm/extensionDist (accessed 23 June, 2021).
Ellison, J. C. (2012). Climate Change Vulnerability Assessment and Adaptation Planning for Mangrove Systems. Washington, DC: World Wildlife Fund (WWF). Available online at: https://www.worldwildlife.org/publications/climate-change-vulnerability-assessment-and-adaptation-planning-for-mangrove-systems
Friess, D. A., and McKee, K. L. (2021). “The history of surface-elevation paradigms in mangrove biogeomorphology,” in Dynamic Sedimentary Environments of Mangrove Coasts (Amsterdam: Elseiver), 179–198.
Fry, B., and Cormier, N. (2011). Chemical ecology of red mangroves, Rhizophora mangle, in the Hawaiian Islands. Pacific Sci. 65, 219–234. doi: 10.2984/65.2.219
Fu, B., Wang, S., Su, C., and Forsius, M. (2013). Linking ecosystem processes and ecosystem services. Curr. Opin. Environ. Sustain. 5, 4–10. doi: 10.1016/j.cosust.2012.12.002
Getzner, M., and Islam, M. S. (2020). Ecosystem services of mangrove forests: results of a meta-analysis of economic values. Int. J. Environ. Res. Public Health 17, 5830. doi: 10.3390/ijerph17165830
Gonneea, M. E., Paytan, A., and Herrera-Silveira, J. A. (2004). Tracing organic matter sources and carbon burial in mangrove sediments over the past 160 years. Estuarine Coast. Shelf Sci. 61, 211–227. doi: 10.1016/j.ecss.2004.04.015
Grijalva, L. S. (2015). Efectos del cambio global en la estructura trófica de los humedales a través del uso de isótopos estables (Doctoral dissertation, Universidad Autónoma de Madrid). Available online at: http://hdl.handle.net/10261/127832
Heiri, O., Lotter, A. F., and Lemcke, G. (2001). Loss on ignition as a method for estimating organic and carbonate content in sediments: reproducibility and comparability of results. Journal of Paleolimnology 25:101–110. doi: 10.1023/A:1008119611481
Herrera-Silveira, J. A. (1994). Spatial heterogeneity and seasonal patterns in a tropical coastal lagoon. J. Coast. Res. 10, 738–746.
Herrera-Silveira, J. A., and Comin, F. A. (1995). Nutrient fluxes in a tropical coastal lagoon. Ophelia 42, 127–146. doi: 10.1080/00785326.1995.10431500
Herrera-Silveira, J. A., and Morales-Ojeda, S. M. (2010). “Subtropical Karstic Coastal Lagoon Assessment, SE Mexico. The Yucatan Peninsula Case,” in Coastal Lagoons: Critical Habitats of Environmental Change (Boca Raton: CRC Press), 309–336
Himes-Cornell, A., Grose, S. O., and Pendleton, L. (2018). Mangrove ecosystem service values and methodological approaches to valuation: where do we stand? Front. Mar. Sci. 5, 376. doi: 10.3389/fmars.2018.00376
Hossain, M. D., and Nuruddin, A. A. (2016). Soil and mangrove: a review. J. Environ. Sci. Technol. 9, 198–207. doi: 10.3923/jest.2016.198.207
IPCC (2021). “Climate Change 2021: The Physical Science Basis,” in Contribution of Working Group I to the Sixth Assessment Report of the Intergovernmental Panel on Climate Change, eds V. Masson-Delmotte, P. Zhai, A. Pirani, S. L. Connors, C. Péan, S. Berger, N. Caud, et al. (Oxford: Cambridge University Press).
Kobashi, D., and Mazda, Y. (2005). Tidal ?ow in riverine-type mangroves. Wetlands Ecol. Manag. 13, 615–619. doi: 10.1007/s11273-004-3481-4
Kumara, M. P., Jayatissa, L. P., Krauss, K. W., Phillips, D. H., and Huxham, M. (2010). High mangrove density enhances surface accretion, surface elevation change, and tree survival in coastal areas susceptible to sea-level rise. Oecologia 164, 545. doi: 10.1007/s00442-010-1705-2
Lee, S. Y., Primavera, J. H., Dahdouh-Guebas, F., McKee, K., Bosire, J. O., Cannicci, S., et al. (2014). Ecological role and services of tropical mangrove ecosystems: a reassessment. Glob. Ecol. Biogeogr. 23, 726–743. doi: 10.1111/geb.12155
Lugo, A. E., and Snedaker, S. C. (1974). The ecology of mangroves. Annu. Rev. Ecol. Syst. 5, 39–64. doi: 10.1146/annurev.es.05.110174.000351
Lynch, J. C., Hensel, P., and Cahoon, D. R. (2015). The Surface Elevation Table and Marker Horizon Technique: A Protocol for Monitoring Wetland Elevation Dynamics. Natural Resource Report NPS/NCBN/NRR-−2015/1078. National Park Service, Fort Collins, Colorado.
MacKenzie, R., Sharma, S., and Royai, A. R. (2021). “Chapter 12—Environmental drivers of blue carbon burial and soil carbon stocks in mangrove forests,” in Dynamic Sedimentary Environments of Mangrove Coasts,eds F. Sidik and D. A. Friess (Amsterdam: Elsevier), 275–294.
Mazda, Y., Wolanski, E., King, B., Sase, A., Ohtsuka, D., and Magi, M. (1997). Drag force due to vegetation in mangrove swamps. Mangrov. Salt Marsh. 1, 193–199. doi: 10.1023/A:1009949411068
McKee, K. L. (2011). Biophysical controls on accretion and elevation change in Caribbean mangrove ecosystems. Estuar. Coast. Shelf Sci. 91, 475–483. doi: 10.1016/j.ecss.2010.05.001
Middelburg, J. J., Nieuwenhuize, J., Slim, F. J., and Ohowa, B. (1996). Sediment biogeochemistry in an East African mangrove forest (Gazi Bay, Kenya). Biogeochemistry 34, 133–155. doi: 10.1007/BF00000899
Mitsch, W., Bernal, B., and Hernandez, M. E. (2015). Ecosystem services of wetlands. Int. J. Biodivers. Sci. Ecosyst. Serv. Manag. 11, 1–4, doi: 10.1080/21513732.2015.1006250
Monacci, N. M., Meier-Grünhagen, U., Finney, B. P., Behling, H., and Wooller, M. J. (2011). Paleoecology of mangroves along the Sibun River, Belize. Q. Res. 76, 220–228. doi: 10.1016/j.yqres.2011.06.001
Montgomery, J. M., Bryan, K. R., Mullarney, J. C., and Horstman, E. M. (2019). Attenuation of storm surges by coastal mangroves. Geophys. Res. Lett. 46, 2680–2689. doi: 10.1029/2018GL081636
Moreno, E., Guerrero, A., Gutiérrez, M. C., Ortiz, C. A., and Palma, D. (2002). Los manglares de Tabasco, una reserva natural de carbono. Madera y Bosques 8, 115–128. Available online at: https://www.redalyc.org/articulo.oa?id=61780107
Naskar, S., and Palit, P. K. (2015). Anatomical and physiological adaptations of mangroves. Wetlands Ecol. Manage 23, 357–370. doi: 10.1007/s11273-014-9385-z
National Oceanic Atmospheric Administration (NOAA) (2016). Available online at: http://www.star.nesdis.noaa.gov/sod/lsa/SeaLevelRise/ (accessed November 2016)
Ramsar (2004). Ficha informativa de los humedales de Ramsar, Reserva de la Biosfera Ría Celestún. Available online at: http://www.smaas.campeche.gob.mx/humedales/wp-content/uploads/2012/01/ria_celestun.pdf (accessed February 18, 2021).
Rey, W. (2012). Evaluación hidrodinámica y modelación numérica de la laguna La Carbonera, Yucatán. Tesis. Universidad Autónoma de Yucatán.
Rodríguez-Zúñiga, M. T, Villeda Chávez, E., Vázquez-Lule, A. D., Bejarano, M., Cruz López, M. I., Olguín, M., et al. (2018). Métodos para la caracterización de los manglares mexicanos: un enfoque espacial multiescala. Comisión Nacional para el Conocimiento y Uso de la Biodiversidad. Ciudad de México.
Saintilan, N., Rogers, K., Mazumder, D., and Woodroffe, C. (2013). Allochthonous and autochthonous contributions to carbon accumulation and carbon store in southeastern Australian coastal wetlands. Estuar. Coast. Shelf Sci. 128, 84–92. doi: 10.1016/j.ecss.2013.05.010
SEMARNAT (2000). Programa de manejo Reserva de la Biosfera Ría Celestún. Comisión Nacional de Áreas Naturales Protegidas—Secretaría de Medio Ambiente y Recursos Naturales. México, D.F. Available online at: https://www.conanp.gob.mx/que_hacemos/pdf/programas_manejo/celestun.pdf (accessed November, 2020).
Shultz, D., and Calder, J. A. (1976). Organic carbon 13C/12C variations in estuarine sediments. Geochim. Cosmochmuca Acta. 40, 381–385.
Simard, M., Fatoyinbo, L., Smetanka, C., Rivera-Monroy, V., Castañeda-Moya, E., Thomas, N., and Van der Stocken, T. (2019). Mangrove canopy height globally related to precipitation, temperature, and cyclone frequency. Nature Geosci. 12, 40–45 (2019). doi: 10.1038/s41561-018-0279-1
Spalding, M., Mcivor, A., Tonneijck, F., Tol, S., and van Eijk, P. (2014). Mangroves for Coastal Defence. Guidelines for Coastal Managers and Policy Makers. Available online at: https://www.nature.org/media/oceansandcoasts/mangroves-for-coastal-defence.pdf
Stalker, J. C., Price, R. M., Rivera-Monroy, V. H., Herrera-Silveira, J., Morales, S., Benítez, J., and Alonzo-Parra, D. (2014). Hydrologic dynamics of a subtropical estuary using geochemical tracers, Celestún, Yucatan, Mexico. Estuar. Coasts 37, 1376–1387. doi: 10.1007/s12237-014-9778-5
Strickland, J., and Parsons, T. (1972). A practical handbook of seawater analysis. Fish. Res. Board Canada Bull. 169, 1–310. doi: 10.1002/iroh.19700550118
Teutli-Hernández, C., Herrera-Silveira, J. A., Cisneros-de la Cruz, D. J., and Román-Cuesta, R. (2020). Mangrove ecological restoration guide: Lessons learned. Mainstreaming Wetlands into the Climate Agenda: a multilevel approach (SWAMP). CIFOR/CINVESTAV-IPN/UNAM-Sisal/PMC, 42p.
Torres-Mota, R., Salles-Alfonso-de-Almeida, P., and López-González, J. (2014). Effects of increased sea levels from climate change on the Celestún Estuary morphology. Tecnol. Ciencias Agua 5, 5–20. Available online at: http://www.scielo.org.mx/scielo.php?script=sci_arttextandpid=S2007-24222014000500001andlng=esandtlng=es (accessed February 08, 2021).
Twilley, R. R., and Rivera-Monroy, V. H. (2009). Ecogeomorphic models of nutrient biogeochemistry for mangrove wetlands. Coastal wetlands: an integrated ecosystem approach. Amsterdam: Elsevier, p. 641.
UNAM—Servicio Mareográfico Nacional (2016). Universidad Nacional Autónoma de México. Instituto de Geofísica, Servicio Mareográfico Nacional, México. Available online at: http://www.mareografico.unam.mx/portal/index.php?page=tiposMarea (accessed June 25, 2021).
Walsh, J. P., and Nittrouer, C. A. (2004). Mangrove-bank sedimentation in a mesotidal environment with large sediment supply, Gulf of Papua. Mar. Geol. 208, 225–248. doi: 10.1016/j.margeo.2004.04.010
Wicaksono, P., and Hafizt, M. (2018). Dark target effectiveness for dark-object subtraction atmospheric correction method on mangrove aboveground carbon stock mapping. IET Image Process. 12, 582–587. doi: 10.1049/iet-ipr.2017.0295
Woodroffe, C. D., Rogers, K., McKee, K. L., Lovelock, C. E., Mendelssohn, I. A., and Saintilan, N. (2016). Mangrove sedimentation and response to relative sea-level rise. Annu. Rev. Mar. Sci. 8, 243–66. doi: 10.1146/annurev-marine-122414-034025
Keywords: mangrove types, sedimentation rate, ecosystem services (ES), conservation, mitigation and adaptation, karstic area
Citation: Cinco-Castro S, Herrera-Silveira J and Comín F (2022) Sedimentation as a Support Ecosystem Service in Different Ecological Types of Mangroves. Front. For. Glob. Change 5:733820. doi: 10.3389/ffgc.2022.733820
Received: 30 June 2021; Accepted: 23 March 2022;
Published: 04 May 2022.
Edited by:
Mark Andrew Adams, Swinburne University of Technology, AustraliaReviewed by:
Matthew Brolly, University of Brighton, United KingdomCopyright © 2022 Cinco-Castro, Herrera-Silveira and Comín. This is an open-access article distributed under the terms of the Creative Commons Attribution License (CC BY). The use, distribution or reproduction in other forums is permitted, provided the original author(s) and the copyright owner(s) are credited and that the original publication in this journal is cited, in accordance with accepted academic practice. No use, distribution or reproduction is permitted which does not comply with these terms.
*Correspondence: Siuling Cinco-Castro, c2djaW5jb0Bob3RtYWlsLmNvbQ==
Disclaimer: All claims expressed in this article are solely those of the authors and do not necessarily represent those of their affiliated organizations, or those of the publisher, the editors and the reviewers. Any product that may be evaluated in this article or claim that may be made by its manufacturer is not guaranteed or endorsed by the publisher.
Research integrity at Frontiers
Learn more about the work of our research integrity team to safeguard the quality of each article we publish.