- 1Faculty of Science, Agriculture and Engineering, School of Natural and Environmental Sciences, Newcastle University, Newcastle upon Tyne, United Kingdom
- 2Faculty of Environment, School of Earth and Environment, University of Leeds, Leeds, United Kingdom
- 3Department of Environment and Geography, University of York, York, United Kingdom
- 4Tropical Forests and People Research Centre, University of the Sunshine Coast, Sippy Downs, QLD, Australia
- 5Department of Ecosystems and Conservation, Sokoine University of Agriculture, Morogoro, Tanzania
- 6Invertebrate Zoology Section, Zoology Department, National Museums of Kenya, Nairobi, Kenya
- 7The Biodiversity Consultancy, Cambridge, United Kingdom
- 8M.A.P Scientific Services, Pretoria, South Africa
- 9Department of Zoology and Entomology, University of Pretoria, Pretoria, South Africa
Global demand for agricultural products continues to grow. However, efforts to boost productivity exacerbate existing pressures on nature, both on farms and in the wider landscape. There is widespread appreciation of the critical need to achieve balance between biodiversity and human well-being in rural tropical crop production landscapes, that are essential for livelihoods and food security. There is limited empirical evidence of the interrelationships between natural capital, the benefits and costs of nature and its management, and food security in agricultural landscapes. Agroforestry practices are frequently framed as win-win solutions to reconcile the provision of ecosystem services important to farmers (i.e., maintaining soil quality, supporting pollinator, and pest control species) with nature conservation. Yet, underlying trade-offs (including ecosystem disservices linked to pest species or human-wildlife conflicts) and synergies (e.g., impact of ecosystem service provision on human well-being) are seldom analysed together at the landscape scale. Here, we propose a systems model framework to analyse the complex pathways, with which natural capital on and around farms interacts with human well-being, in a spatially explicit manner. To illustrate the potential application of the framework, we apply it to a biodiversity and well-being priority landscape in the Southern Agricultural Growth Corridor of Tanzania, a public-private partnership for increasing production of cash and food crops. Our framework integrates three main dimensions: biodiversity (using tree cover and wildlife as key indicators), food security through crop yield and crop health, and climate change adaptation through microclimate buffering of trees. The system model can be applied to analyse forest-agricultural landscapes as socio-ecological systems that retain the capacity to adapt in the face of change in ways that continue to support human well-being. It is based on metrics and pathways that can be quantified and parameterised, providing a tool for monitoring multiple outcomes from management of forest-agricultural landscapes. This bottom-up approach shifts emphasis from global prioritisation and optimisation modelling frameworks, based on biophysical properties, to local socio-economic contexts relevant in biodiversity-food production interactions across large parts of the rural tropics.
Introduction
Human land-use has expanded and intensified rapidly over the last 300 years alongside human population and consumption growth (Ramankutty et al., 2018). Global agricultural production more than tripled since the 1960s to cope with our rising demands for food (Food and Agriculture Organization [FAO], 2017). During the past 50 years, the area of land under cultivation increased from 76 to 120 M ha in Africa, 83 to 175 M ha in Latin America, and 115 to 161 M ha in Asia (Pellegrini and Fernández, 2018). Agricultural expansion has been accompanied by land intensification that has led to increases in irrigation, machinery and fertiliser use and the tripling of crop yields globally (Pellegrini and Fernández, 2018). Despite this, in Sub-Saharan Africa, it is estimated that agricultural output would still need to more than double to meet predicted demand by 2050 (Food and Agriculture Organization [FAO], 2017). Declining crop yield gains, soil quality and climate change are expected to further complicate that goal (Funk and Brown, 2009; Borrelli et al., 2020).
The environmental and social costs and benefits of agricultural expansion and intensification to close yield gaps (Box 1) are profound, encompassing dimensions of biodiversity (Chaudhary and Mooers, 2018), human well-being (Brown et al., 2018), and climate change resilience (Webb et al., 2017) of the socio-ecological systems being transformed. The loss of natural habitats, primarily forests and grasslands, at the expense of agricultural expansion in the rural tropics, has contributed to large-scale biodiversity declines (IPBES, 2019). The use of pesticides (including insecticides, molluscicides, herbicides, and fungicides) has caused substantial economic costs to human health (Trimmer et al., 2017), as well as ecosystem services (Pretty and Bharucha, 2015), with declines in species useful for pollination and natural pest control (Dudley et al., 2017). Croplands are also connected to global increases in soil erosion, subsequently leading to land degradation and fertility loss, eutrophication of waterways, enhanced flooding and changes in carbon cycling (Borrelli et al., 2017). These in turn are likely to incur “debts in natural capital,” with short-term gains in crop yield but potentially long-term losses in crop yield stability (Box 1).
Box 1. Yield gaps in crop production systems.
Yield gap, i.e., the difference between potential and actual crop yield, is determined by abiotic and biotic variables, which are interrelated and include water and nutrient availability in soils, pest damage or pollination and natural pest control services (Bommarco et al., 2013; Dainese et al., 2019). Closing the yield gap, e.g., by boosting soil quality (Oldfield et al., 2019) or biodiversity regulated services (Carvalheiro et al., 2010; Dainese et al., 2019), can help farmers improve food security (Van Ittersum et al., 2016) and if linked to efficient access to markets may help alleviate poverty (Jayne et al., 2010). Yield gaps have reportedly been higher for low input forest-agricultural system compared to high input crop production systems (Kravchenko et al., 2017). We highlight that yield comparisons between low and high input systems so far are of limited use if not accounting for system properties changing over time, including soil quality, soil availability or water quality (through outflow of chemical fertilisers and pesticides), which can negatively affect crop yield stability in the long term (Schrama et al., 2018). Thus, reported yield gaps between these systems might be an artefact resulting from natural capital debt generated by unsustainable practices that in the long term will reduce crop yield stability (Schrama et al., 2018). Empirical evidence shows that additional plant diversity, either in crops or surrounding vegetation, can make agricultural systems more resilient with multiple benefits to farmers, including on yield stability, pollination or dependency on synthetic inputs (Isbell et al., 2017; Duriaux Chavarría et al., 2018; Williams et al., 2018; Dainese et al., 2019). Nevertheless, the contextual nature of yield metrics advise against one-size-fits-all approaches (Li et al., 2019).
Managing crop production systems to reconcile targets for biodiversity with targets for climate change and human well-being is a challenge that has attracted substantial controversy. Differences in proposed solutions may partly be explained by heterogeneity in the way crops can be produced and the socio-economic and political complexity of livelihoods within rural landscapes. Crop production systems differ in intensity of mechanic and chemical inputs and severity of habitat modification (Horlings and Marsden, 2011). They range from low input smallholder agroforestry systems – defined as the integration of trees and woody shrubs in crop and livestock production systems (Sinclair, 1999) – that support livelihoods of millions of people in the rural tropics (Mbow et al., 2014) to high input monoculture cropping systems that are favoured in agricultural transformation plans (Laurance et al., 2015). Low input agroforestry systems can improve biodiversity and ecosystem services (Tscharntke et al., 2011) and allow farmers to adapt to climate extremes (Lasco et al., 2014). Yet, they may require larger areas of land to meet yield demands (Balmford et al., 2018) and therefore reduce resources for wildlife that depend on large intact habitats (Pfeifer et al., 2017) and increase risk of human-wildlife conflict (Nyhus, 2016). The alternative, high yield farming on smaller areas of land, in turn can spare larger tracts of natural habitats reducing the biodiversity loss crisis (Balmford et al., 2018). However, it has been associated with high environmental (Tilman et al., 2002) and social costs (Rasmussen et al., 2018), while landscape-scale biodiversity benefits are inconclusive (Kremen, 2015).
Strategies for increasing crop production frequently promote high input systems as the way forward (Crist et al., 2017; Strassburg et al., 2020). This “land sparing” approach to farming overlooks evidence from the biodiversity literature, showing landscape variation in species’ responses to crop configurations and natural features (Kremen, 2015). Well-being (Box 2), a concept that integrates across interrelated issues of material wealth, food security and human health (Gough et al., 2007), has received limited attention by land sparing advocates in modelling and mapping approaches. That approach can underestimate the decline in pollination and pest control services to crop yields and health due to loss of species diversity within farms (Tscharntke et al., 2005; Bommarco et al., 2013). Smallholder farmers also face economic barriers that prevent them from achieving productivity gains based on external input intensification (Jayne et al., 2010). And there is little evidence to suggest that agricultural intensification can provide win-win outcomes for both the environment and human well-being (Rasmussen et al., 2018). Indeed, risks associated with climate change, projected to be especially high in tropical regions (Collins et al., 2019), can be exacerbated by increasing farmer vulnerability to climate extremes; reducing their options to adapt to climate change in the future (Lin et al., 2008; Paavola, 2008).
Box 2. Assessing human well-being in forest-agricultural landscapes.
In our framework we adopt the definition of well-being as “a state of being with others, which arises where human needs are met, where one can act meaningfully to pursue one’s goals and where one can enjoy a satisfactory quality of life” (Gough et al., 2007). It encompasses a holistic notion of social progress. Human well-being is broken down into universally relevant domains and context specific indicators (McGregor, 2018). We take an interdisciplinary approach and adopt the domains put forward by the Millennium Ecosystems Assessment (Millennium Ecosystem Assessment [MEA], 2003), although others have been proposed (King et al., 2014; McGregor, 2018). The five domains include: (1) Basic material for a good life – hereafter referred to as material well-being, (2) Health, (3) Social relations, (4) Security, (5) Freedom of choice and action. We translate these domains of well-being to the specific context of our focal landscape using the Well-being Indicator Selection Protocol (Loveridge et al., 2020) that integrates universal theory with place-based knowledge. Through community consultation 111 candidate well-being indicators were identified and subsequently simplified by redundancy analysis, statistical modelling and cross-validation with locally stated priorities to determine a final list of 19 local indicators representative of the five well-being domains (Loveridge et al., 2020). Approaches that use well-being metrics to evaluate the impact of land management interventions tailored to specific contexts are increasing, albeit significant knowledge gaps persist (McKinnon et al., 2016; Rasmussen et al., 2018). Smith et al. (2019), for example, tailored a multidimensional well-being index using 15 indicators across three dimensions to explore the impacts of land use intensification in Mozambique and found well-being improvements linked to smallholder commercial and subsistence agriculture.
While there is a rich literature on the positive links between agroforestry practices and several ecosystem services, such as soil fertility, drought resistance or biological pest control (e.g., Tscharntke et al., 2011), empirical evidence attempting to connect agroforestry practices directly to well-being (e.g., Ojedokun et al., 2020) is scarce. The link between crop yield and material well-being may be intuitive, yet other aspects of well-being that cover health, security or freedom of choice, are under-researched (McKinnon et al., 2016). Furthermore, the benefits and disadvantages of natural areas do not necessarily impact on all the dimensions of well-being equally (Rendón et al., 2019). Win-win outcomes may be less common than trade-offs (McShane et al., 2011; Howe et al., 2014).
Human well-being cannot be separated from other Sustainable Development Goals such as food security and poverty reduction. As such, crop production benefits from resilient agricultural landscapes as socio-ecological systems that have the capacity to adapt or transform in the face of change in ways that continue to support human well-being and ecological processes (Folke et al., 2016). The “resilience” component is crucial in crop production landscapes (Robards et al., 2011) and there is growing consensus that more diverse natural systems support more resilient crop production, ultimately providing greater well-being through increased security in terms of food and livelihoods (Gunderson et al., 2012). Resilience can be strengthened by high landscape connectivity (Grass et al., 2019), promoted for example by retaining or restoring trees and other natural habitats in and around farmlands. This is practiced in agroforestry systems globally, and compatible with sustainable livelihoods in rural communities (Chazdon, 2008). The Intergovernmental Panel on Biodiversity and Ecosystem Services is explicit about the value of natural habitats and their interlinkages with key Sustainable Development Goals for crop production landscapes in the tropics (IPBES, 2018).
However, a key challenge has been a fundamental lack of data on the crop production potential of diverse resilient forest-agricultural landscapes, caused by a lack of a unifying framework and associated method approaches (Woodhouse et al., 2015). In this article, we propose a novel unifying framework to conceptualise pathways to well-being and biodiversity outcomes in forest-agricultural landscapes (Figure 1). This framework allows us to measure the impact of landscape and regional level decisions on three main outcomes, i.e., crop yield, human well-being, and biodiversity (Figure 2). Our focus is on tropical landscapes dominated by smallholder farming that retain natural features on and around farms, and in which crop yield outputs are well correlated with food security and well-being (IFAD and UNEP, 2013).
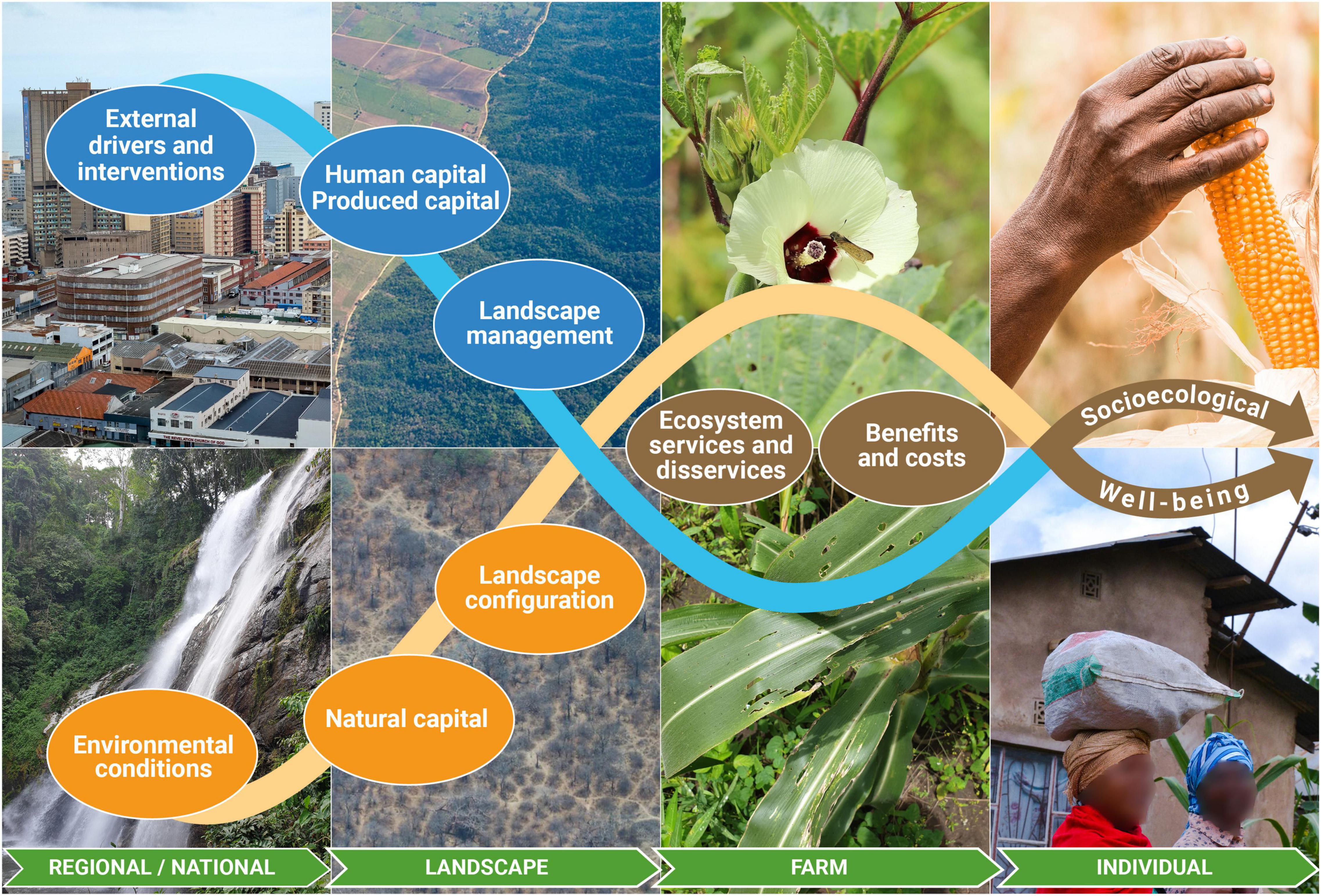
Figure 1. Pathways to socioecological well-being outcomes in forest-agricultural landscapes. The framework integrates across the social and ecological dimensions. It includes interventions and drivers external to the socioecological system, socioeconomic and environmental drivers of change operating at larger spatial scales. While variables can have higher ecological (orange stream) or social (blue stream) leanings, their interaction with natural and other types of capital at landscape level produces impacts on biodiversity and ecosystem service provision (interactions in brown, represented along the middle line), the latter including crop yield. The provision of those services, and trade-offs with disservices, then affects both biodiversity and human well-being outcomes. We use the term “socioecological well-being” to represent both the ecological processes and functions that define self-sustaining ecosystems and the social processes that influence the fulfilment of human needs, individual and communal goals, and a satisfactory quality of life (Brueckner-Irwin et al., 2019). The image of the two women comes from pexels.com with a license that covers its use in this publication.
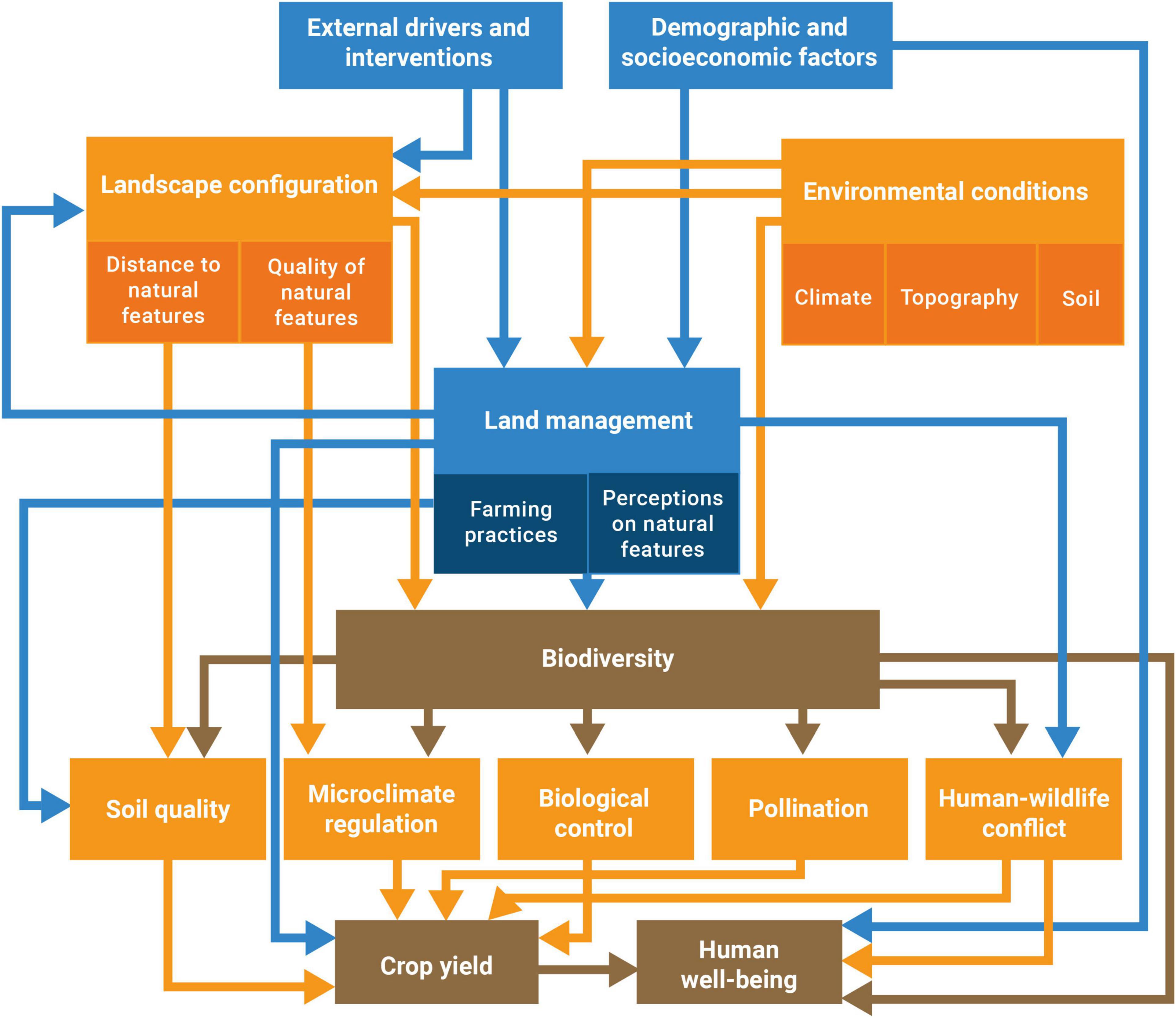
Figure 2. System model approach operationalising the assessment of management of forest-agricultural landscapes for multiple objectives. The model describes a network of relationships among variables and processes important to understanding socio-ecological systems within forest-agricultural landscapes. Arrows represent causal links between variables at landscape and site scale to be tested. Pollination, biological pest control, microclimate regulation, maintenance of soil quality are ecosystem services, while crop yield is an ecosystem service too that is impacted by the other services and a key outcome of our framework. Human-wildlife conflict is an ecosystem disservice. Blue colour refers to components linked to the social dimension, orange components link to the ecological dimension, brown objects refer to outcomes directly linked to nature conservation, food security, and human well-being, within the socioecological system.
Conceptualising Interrelationships in Forest-Agricultural Landscapes
In our framework, natural capital and environmental conditions are conceived as interacting with human and produced capital, external drivers, and interventions. We refer to the concept of socioecological well-being, as defined in Brueckner-Irwin et al. (2019), to emphasise the strong interactions between different outcomes contributing to socioecological system resilience, including biodiversity, food security, and human well-being. Following Dasgupta (2021), we distinguish between natural capital (stock of renewable and non-renewable natural assets), human capital (assets embodied in labour, skills and knowledge) and produced capital (human-made goods or structures). Yield (Box 1) plays a crucial role in that context, as it is the variable typically cited in land sharing – sparing debates, but also because it is inextricably linked to farmers’ livelihoods and thus well-being (IFAD and UNEP, 2013). Natural capital encompasses stocks of resources such as water and soils. These stocks generate flows of services for which people derive benefits such as crop yield, pest control or energy (Bateman and Mace, 2020), but also wild food foraging, timber, water and climate regulation or spiritual connections and sense of heritage tied with the natural landscape (Millennium Ecosystem Assessment [MEA], 2003). These relationships can be non-linear and result from complex locally specific social and ecological dynamics, flowing from ecosystem stocks to goods to well-being and mediated by factors such as stakeholder needs, market dynamics, or access (Daw et al., 2016). Natural capital can also generate flows of disservices (Kennedy et al., 2013; Mbow et al., 2014), with increased potential for human wildlife conflict, including crop raiding, livestock depredation and attacks on humans (Mukeka et al., 2019). Landscape configuration, here defined as spatial configuration and quality of natural habitats in the agricultural matrix, alters abundance distributions of species that may act as pest controls and pollinators (Bianchi et al., 2006; Carvalheiro et al., 2010), as well as agricultural pests (e.g., rats) and animals perceived as dangerous (e.g., elephants) (Teixeira et al., 2019). These processes are largely shaped by distance to habitat edges, declining with distance away from natural habitat and into the farmed land (Bianchi et al., 2006; Carvalheiro et al., 2010).
We are testing the implementation of our framework in a rural case study landscape of the Kilombero Valley in Tanzania (Figure 3). Tanzania is emblematic of the challenges of agricultural expansion and intensification to biodiversity (Doggart et al., 2020).
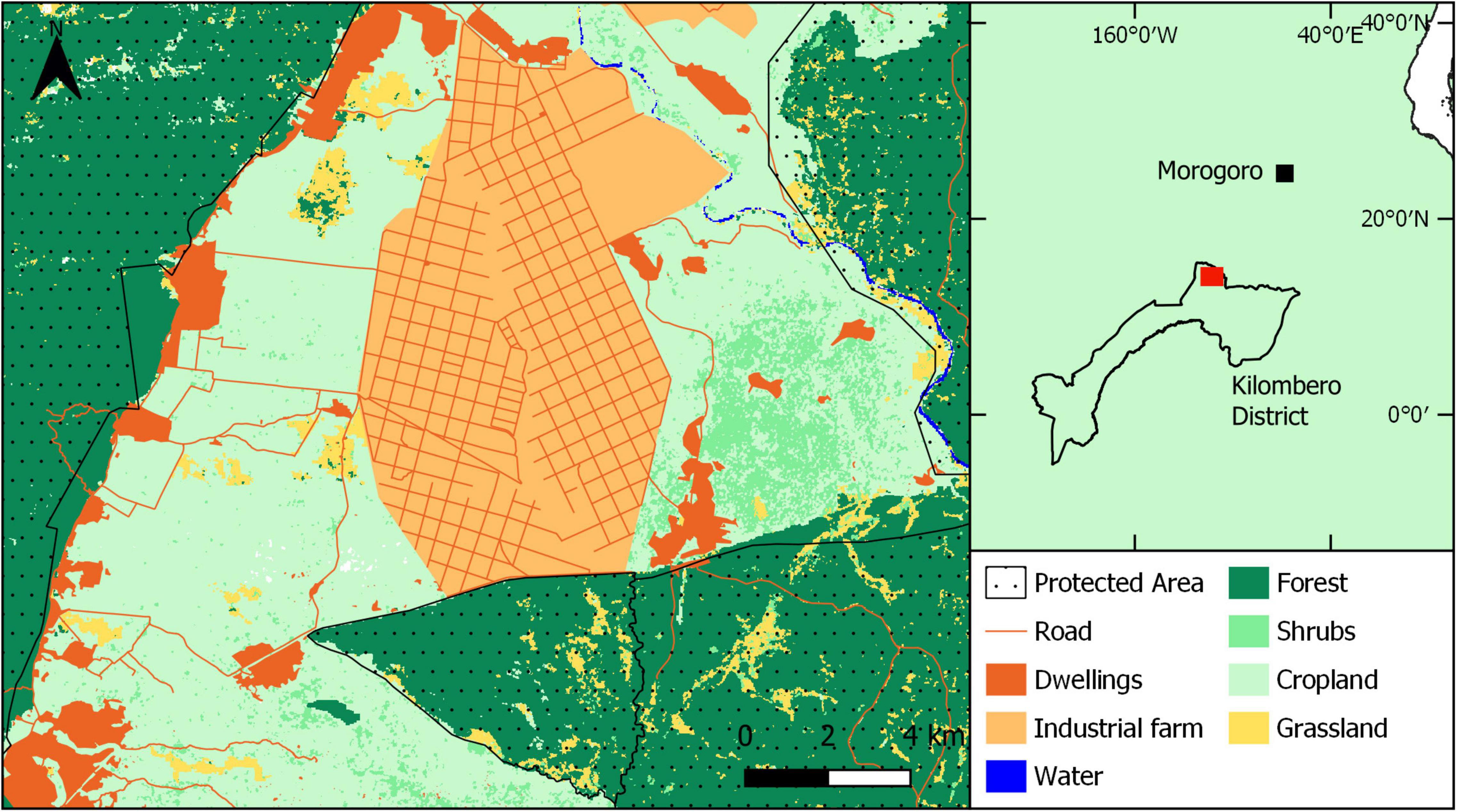
Figure 3. Map of the study area in the Kilombero Valley, Tanzania. Land cover map at 20 m based on 1 year of Sentinel-2A developed by the European Space Agency (European Space Agency [ESA], 2017). Dwellings classified manually. The protected areas represented are Mikumi National Park, Udzungwa National Park, Magombera Nature Reserve, and Nyerere National Park (UNEP-WCMC and IUCN, 2021). Road data from OpenStreetMap Contributors (2019).
Adopting a “Kilimo Kwanza” (Agriculture First) strategy, Tanzania’s government launched the Southern Agricultural Growth Corridor Of Tanzania (SAGCOT) scheme in 2010. The fertile soils of the Kilombero Valley, a wetland of international importance for biodiversity, are key areas targetted for agricultural development under SAGCOT. Planned measures would greatly increase the amount of land dedicated to industrial farming (Southern Agricultural Growth Corridor of Tanzania [SAGCOT], 2011). Land shortage has been a problem in the valley since 1975. A growing human population and an influx of people looking for opportunities, have exerted high pressures on natural resources and land, used for crop production and livestock grazing (Kangalawe and Liwenga, 2005). In the last two decades, the valley has seen investments into industrial rice, teak and sugarcane farming (Bergius et al., 2018). Irrigation systems and draining have been implemented in several areas of the valley to support industrial crop production and large tracts of forests and Miombo woodlands have been converted to make place for sugarcane and teak (Munishi and Jewitt, 2019). Smallholder farmers are traditionally practicing a mix of crop and crop:tree approaches to farming and rely on natural tree-cover habitats for resources such as firewood and building material. The farmed land in the valley is nestled between the Udzungwa Mountains National Park in the west, the Nyerere National Park (formerly Selous Game Reserve) in the east, and Mikumi National Park in the north. Yet, SAGCOT plans are vague on the benefits local farmers obtain from natural areas or the potential role of less conventional approaches to agriculture, including agroforestry (Southern Agricultural Growth Corridor of Tanzania [SAGCOT], 2011). Spill over of wildlife into settlements and farmland is common leading to elephant:crop conflicts (Jenkins et al., 2003; Jones et al., 2012), but these are also overlooked. Nevertheless, at national scale, the role of agroforestry supporting resilient agricultural systems is acknowledged in national policies, such as the Agricultural Sector Development Programme Phase II 2018–2023 and the National Forest Policy Implementation Strategy 2021–2031.
We demonstrate the implementation of our proposed framework through a system model approach (Figure 2), that allows to quantify the direct and indirect pathways and their interactions at various spatial and temporal scales to shape biodiversity, yield, and well-being outcomes. It is sufficiently simple to be testable, with clearly formulated hypotheses for which data can be collected and metrics can be parameterised (Table 1).
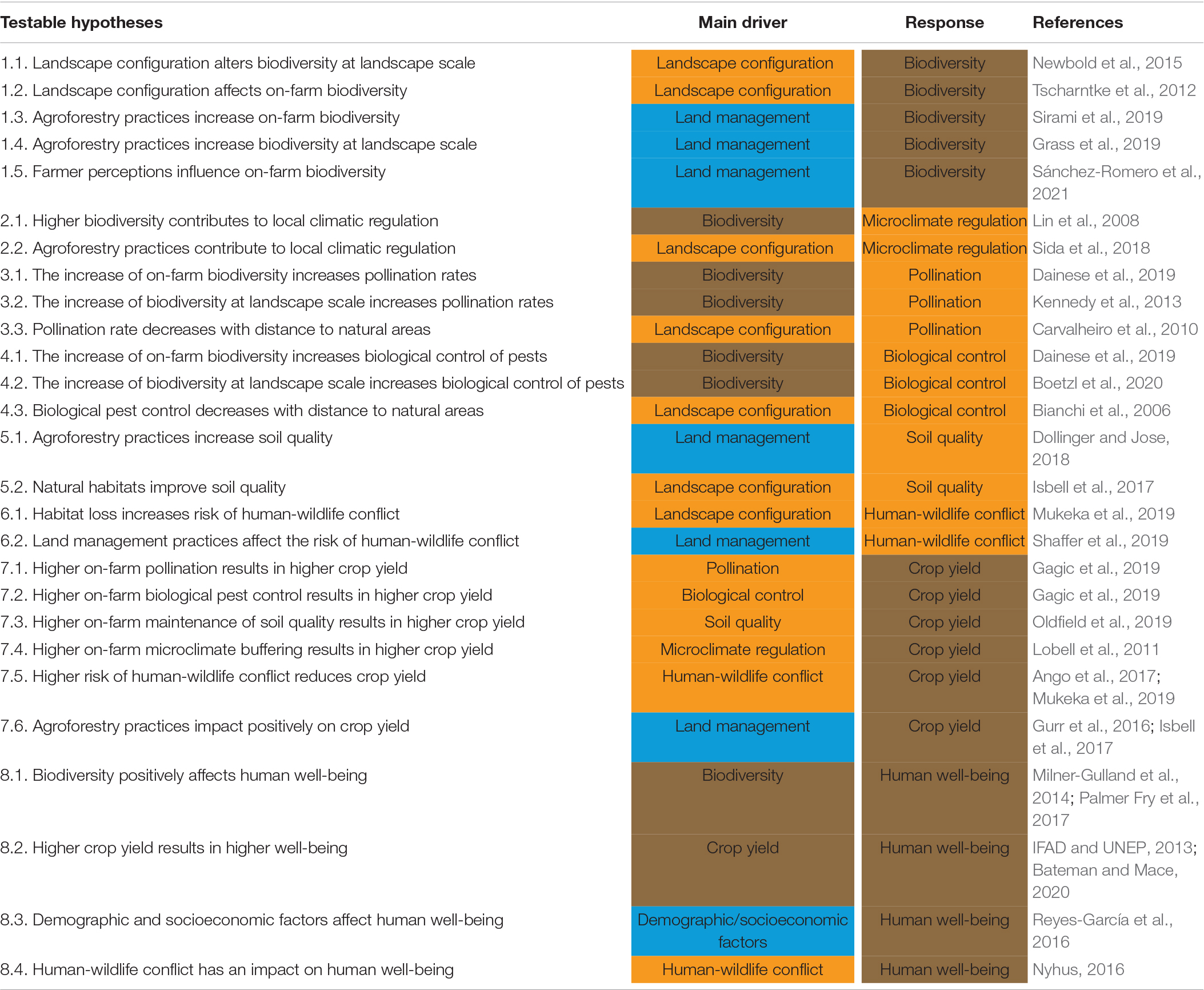
Table 1. Overview of testable hypotheses resulting from system model pathways (their starting and finish nodes, i.e., main direct driver and response) for analysing multiple outcomes in forest-agricultural systems.
System Model Components and Pathways
Changes to landscape configuration (e.g., increased exposure to habitat edges through habitat fragmentation) or management practices (e.g., use of synthetic inputs), both within and around croplands, are key drivers of pressure on ecological communities. These linkages are analysed in the system model through testable hypotheses 1.1, 1.2, 1.3, and 1.4 (Table 1). Biodiversity loss, in turn, can have unintended consequences that affect crop yield and other ecosystem services (Rasmussen et al., 2018). Farmer decisions on management strategies will, at least in part, depend on their perceptions on the environment (Sánchez-Romero et al., 2021; Table 1, testable hypothesis 1.5). At landscape scale, changes in configuration through land use and farm management decisions, such as retention of trees on farms and along their boundaries (e.g., Torralba et al., 2016), will affect the trade-offs between flows of services and disservices within the forest-agricultural landscape (Zhang et al., 2007; Table 1, testable hypotheses 2.2, 3.3, 4.3, 5.1, 5.2, 6.1, and 6.2). At farm level, trees can improve microclimate favourably to crops and maintain soil health and soil organic carbon (Jonsson et al., 1999; Dollinger and Jose, 2018). Denser and deep rooted tree canopies are associated with a buffering of ground vegetation from the impacts of climate extremes, directly protecting plants from temperature and moisture stress and thus benefitting crop growth and yield (Jonsson et al., 1999; Lobell et al., 2011; Ewers and Banks-Leite, 2013; Peng et al., 2014; Table 1, testable hypotheses 2.1 and 7.4). This buffering effect can translate to increased soil moisture, soil carbon storage and stability of crop yields under climate fluctuation as well as reduced soil erosion, thereby increasing farmer preparedness for potential impacts from climate change (Malézieux et al., 2009; Sida et al., 2018). Trees can indirectly affect how pest species respond to environmental change, if they are part of that species life history or trophic interactions, for example (Lehmann et al., 2020), whilst some tree species act as sources for biopesticides themselves (Isman, 2008; Table 1, testable hypotheses 4.1 and 4.2). In “push-pull” technology pest repellent species are intercropped while pest attracting species are planted along borders to decrease damage to focal crop (Khan et al., 2008). Higher plant diversity on croplands can increase crop yield, although higher tree density and shade can also lead to real or perceived productivity losses (Isbell et al., 2017; Ndoli et al., 2017; Teixeira et al., 2019; Table 1, testable hypotheses 7.2 and 7.6). Adding trees on and around farms increases the prevalence of earthworms leading to improved soil functioning (Marsden et al., 2020) and soil quality (Fründ et al., 2011), and subsequent increases in crop yield and aboveground biomass (Van Groenigen et al., 2014; Table 1, testable hypotheses 5.1, 5.2, and 7.3). Planting nitrogen-fixing tree species, e.g., Acacia spp., can increase soil nutrient availability and reduce reliance on fertiliser inputs (Rosenstock et al., 2014; Table 1, testable hypothesis 5.1). On pollination, the effects of species turnover and dominance mean that a diverse pollinator community is needed for maintaining pollination services at landscape scale (Winfree et al., 2018), which due to taxon-specific responses include species that need nearby forests to survive (Brosi et al., 2008; Table 1, testable hypotheses 3.1, 3.2, and 7.1). Human-wildlife conflict can cause crop damage and be more prevalent along the boundaries of areas converted into uses incompatible with wildlife and those that prioritise conservation (Ango et al., 2017; Mukeka et al., 2019; Table 1, testable hypothesis 7.5).
Understanding and monitoring the impact of both agricultural development and conservation interventions on the model pathways leading to biodiversity, yield, and well-being outcomes, as well as the impact of indirect drivers that are external to the socioecological system, will help inform policies and decision-making in forest-agricultural landscapes. These drivers and interventions originate at scales coarser than the landscape and are linked primarily to economic development priorities or institutional frameworks and laws, including on nature protection. The consequences of development corridors promoted across Africa, which can include both “hard” (e.g., road network expansions) and “soft” (e.g., regulatory reforms) interventions (Enns, 2018), exemplify well our definition of external drivers resulting in interventions that impact on the local scale. The demographic and socioeconomic factors included in the model reflect community characteristics independently from those external interventions, although in practice there may be an association between the two on the longer term. Their impacts can include unexpected consequences not predicted during the decision making process and lead to the cumulative negative effects surpassing the positive ones in many cases (Laurance et al., 2015). Many of these interventions impact on landscape configuration or management and can hence be analysed through our system model. The pathways and trade-offs involved can be manifold and complex, ultimately affecting human well-being (Daw et al., 2015; Suich et al., 2015; Table 1, testable hypotheses 8.1, 8.2, 8.3, and 8.4).
System Model Implementation
Our approach operationalises relationships between biodiversity conservation, food security and well-being outcomes in a forest-agricultural landscape and makes them spatially explicit. We postulate testable hypotheses to examine whether the theory formulated holds across geolocations looking for synergies and differences and how these relate to external drivers (Table 1). We also integrate landscape configuration and management, ecosystem services (pollination, pest control, soil quality, microclimate regulation, and crop yield), ecosystem disservices (pest damages and human-wildlife conflicts) and resulting well-being outcomes.
In Table 2, we highlight the indicators used to parameterise our system model for our case study (Figure 2). After obtaining relevant research permits and ethical clearance, we are currently collecting data for these indicators using standardised social and ecological methodologies, adapted to the specifics of our study landscape. The sampling design for ecological indicators consists of collecting data from plots (20 × 20 m) located on the farm, including smallholder and industrial farms, and in habitats surroundings those farms. We plan to collect ecological data from 140 plots sampled in crop and non-crop habitats and edges among them. Table 2 briefly mentions which methodologies are being used to measure each variable. More detailed method descriptions can be found in the Supplementary Material. For crop yield measurements, following local scouting and considering varying crop pollination dependencies (Aizen et al., 2009), we are focussing on three common crops in the study area – maize, okra and sugarcane. On the social side, we are applying household surveys in seven villages adjacent to the ecological plots using a stratified sampling approach. The household survey is collecting data on socioeconomic details, household characteristics, livelihood strategies, perceptions of nature, and farming practices. We are also carrying out key informant interviews, focus groups, and participatory workshops with stakeholders to achieve a more detailed and contextualised understanding of local characteristics, including its social dynamics.
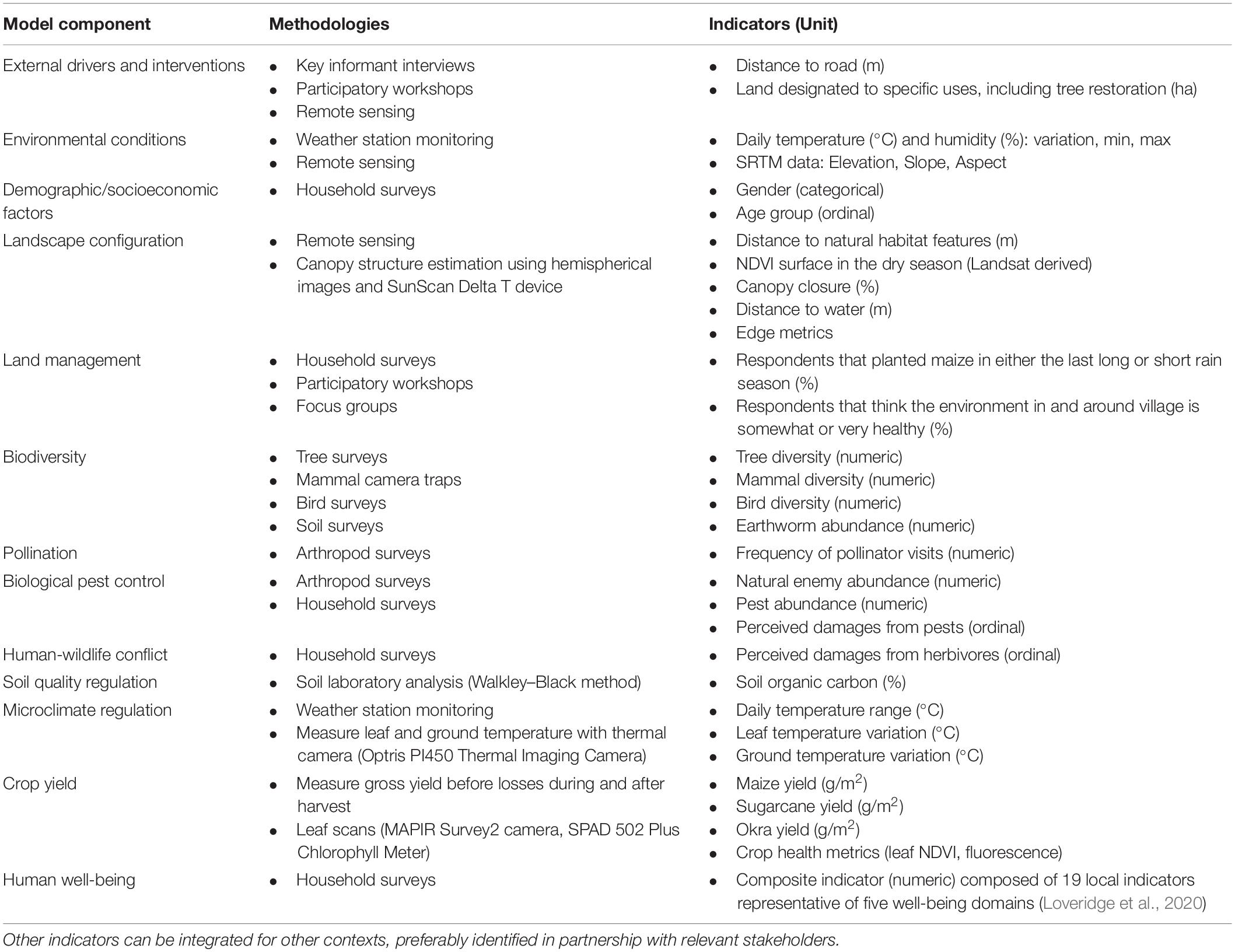
Table 2. List of locally adjusted methodologies and indicators that are being used to generate data for the system model that will analyse biodiversity, well-being, and crop yield outcomes resulting from interventions and practices implemented in the Kilombero landscape.
Overall, the sampling design will allow testing for the effects of landscape scale variables on ecosystem service provision, including crop yield, and well-being outcomes, linked to farming practices such as agroforestry. This data will be complemented by remote sensing data for ecological metrics and key informant interviews, surveys, focus groups and participatory workshops for social data. When evaluating the impact of conservation interventions on well-being, Woodhouse et al. (2015) advises defining locally relevant well-being indicators, designing context dependent approaches, understanding processes of change and causal mechanisms. We will use the well-being indicators formerly developed by Loveridge et al. (2020) in the Kilombero District (Box 2).
We will integrate our data analysis with participatory visioning approaches with local stakeholders to develop a final network of pathways linked to management practices on the farm and in the landscape. It will use the causal links and hypotheses developed in this article to reach a system that can be analysed through complex modelling tools. This approach will allow us to enhance our understanding of the dynamics in land management decision-making and to explore the role of farming practices and drivers of land use change, both under current or future settings, to identify balanced and sustainable solutions that optimise well-being, as well minimising the trade-offs between services and disservices.
We will use Structural Equation Modelling and Integrated Nested Laplace Approximation to analyse pathways and outcomes in our case study, but other statistical tools could be used, calibrated to local contexts and modelling expertise. The absence of feedback loops in our current model intends to facilitate analysis, as more analytical options become available. However, in reality, we anticipate that some processes will involve feedback or reciprocal relationships such that the impacts of one variable can lead to reverse impacts on the driver (reciprocal paths) or indeed more complex loops involving three or more processes (feedback). Whilst this complicates analysis, as it makes the model non-recursive, there are procedures such as block recursion, two-way least squares and Full Information Methods that can be used to evaluate models with this structure.
Potential Model Applications
Agricultural landscapes are essential to human well-being (Power, 2010). The people that live in agricultural landscapes shape the flows of services and disservices that originate from natural capital stocks (Vialatte et al., 2019), shaping their well-being (Bateman and Mace, 2020). Our framework allows the exploration of the causal pathways through which that happens. This is possible through testable hypotheses that link between drivers of change, landscape variables, biodiversity, and farming practices, with ecosystem service provision, specifically biological pest control, pollination, soil quality, microclimate regulation, and crop yield, ecosystem disservices, mainly human-wildlife conflict with large herbivores, and finally human well-being. Our system model offers a multidimensional perspective on the complexity of human – environment interactions that play out in forest-agricultural landscapes. It can be used to analyse the needs and actions of individuals and communities, and by using social and ecological indicators linked to well-being metrics, allows to retain local nuances that are often ignored in landscape planning and management (Palmer Fry et al., 2017).
We are increasingly aware that trade-offs exist between multiple outcomes in forest-agricultural landscapes and need to be managed for, and that this requires to be evaluated in terms of spatial scale, temporal scale and reversibility (Power, 2010). Our approach provides a quantitative tool to allow for that evaluation, albeit with the limitation of not incorporating feedback loops for analytical reasons. Still, the system model structure is flexible and can be adapted to local contexts through the selection of indicators and metrics that meet potentially divergent stakeholder needs and expectations. Thus, it allows us to analyse issues at local scales and across management contexts to identify general patterns and highlight the relative importance of governance, institutions, and external drivers in shaping system model components and their interactions. The use of this socioecological model in different contexts can therefore help reach generalisable policy-relevant insights on well-being, as well as help identify barriers to further well-being improvements and the relative importance of local factors against external drivers playing at larger scales. Nevertheless, the translation of these insights into actual impacts in decision or policy making processes will always be dependent, not only on how insights are communicated to relevant stakeholders, but also on the receptivity of those processes to data-driven information, amongst other factors (Kørnøv and Thissen, 2000). In the context of our study area, we will organise participative and knowledge exchange initiatives with stakeholders acting on national, district or local levels to further explore how insights originating from the system model, for example on the association between specific farming practices, nature conservation, and human well-being, can be incorporated into ongoing and future development and tree restoration plans and programmes originating from the Tanzanian government or other social actors, including NGOs (e.g., Jones et al., 2012).
Two recent policy narratives have emerged from conservation science for crop production landscapes in the rural tropics. One focussed solely on the biodiversity and climate change mitigation benefits offered by ecosystem restoration. The second promotes the intensification of crop production on farmed land as the best strategy to protect remaining natural areas. There have been several calls for management of crop production landscapes to be based on ecological principles, integrating the benefits of natural areas within farmland (e.g., Bommarco et al., 2013; Garibaldi et al., 2019), as well on social dynamics, integrating the benefits of inclusive community-led decision-making (e.g., Fleischman et al., 2020; Chambers et al., 2021). Yet, strategies used in practice often retain a narrow focus on food productivity, whilst assigning biodiversity conservation targets to protected areas set aside from farmed land (Fischer et al., 2014), including recent high profile discussions planning prioritisation of restoration interventions (e.g., Bastin et al., 2019; Strassburg et al., 2020). A reductionist approach on land use planning, including for ecosystem restoration, nature conservation, or food production, is not supported by sufficient evidence of “win-win” outcomes. In fact, it may produce unwanted negative biodiversity, crop yield and well-being outcomes, if it discounts the complex interdependency between people and nature and the relevance of social factors mediating the impact of environmental change on well-being (Daw et al., 2016; Rendón et al., 2019).
We believe the system model proposed here can contribute to evidence-based approaches for policy, management and monitoring in forest-agricultural landscapes in the rural tropics by framing the discourse on land use intensification around people-nature relationships, to reflect the conditions of smallholder farmers and contribute to sustainable development goals (Smith et al., 2019). This bottom-up approach shifts emphasis from global prioritisation and optimisation modelling frameworks, based on biophysical properties, to the local socio-economic contexts that are relevant in biodiversity-food production interactions across large parts of the rural tropics. It also recognises the importance of livelihoods alongside climate change resilience and biodiversity conservation, and ultimately is more likely to garner support and achieve positive impacts when implementing conservation interventions (Bennett et al., 2017). Furthermore, it expands the potential of well-being metrics for locally grounded evaluations on the interlinkages between people and nature, contextualised within the wider landscape of environmental and social change (Milner-Gulland et al., 2014).
Data Availability Statement
The original contributions presented in the study are included in the article/Supplementary Material, further inquiries can be directed to the corresponding author.
Ethics Statement
Written informed consent was obtained from the individual(s) for the publication of any potentially identifiable images or data included in this article.
Author Contributions
SM, SS, DS, AM, EK, and MP conceived the original idea, with additional editorial and conceptual inputs from EM, RL, PO, SR, and YT. SM and MP wrote the first draft of the manuscript. SM developed the figures. All authors contributed to manuscript revision, read, and approved the submitted version.
Funding
Agrisys Tanzania project was funded through BBSRC Global Challenges Research Fund under reference BB/S014586/1. This research was registered in the Tanzania Commission for Science and Technology under references 2019-578-NA-2019-243 and 2019-577-NA-2019-243.
Conflict of Interest
RL was employed by company The Biodiversity Consultancy.
The remaining authors declare that the research was conducted in the absence of any commercial or financial relationships that could be construed as a potential conflict of interest.
Publisher’s Note
All claims expressed in this article are solely those of the authors and do not necessarily represent those of their affiliated organizations, or those of the publisher, the editors and the reviewers. Any product that may be evaluated in this article, or claim that may be made by its manufacturer, is not guaranteed or endorsed by the publisher.
Acknowledgments
We would like to thank Laura Braunholtz for helping prepare the camera trap protocol, Gregoire Dubois for two of the images that were used in Figure 1, and the staff at Reforest Africa, Illovo Sugar Ltd., and Udzungwa Ecological Monitoring Centre for their helpfulness.
Supplementary Material
The Supplementary Material for this article can be found online at: https://www.frontiersin.org/articles/10.3389/ffgc.2022.709971/full#supplementary-material
References
Aizen, M. A., Garibaldi, L. A., Cunningham, S. A., and Klein, A. M. (2009). How much does agriculture depend on pollinators? Lessons from long-term trends in crop production. Ann. Bot. 103, 1579–1588. doi: 10.1093/aob/mcp076
Ango, T. G., Börjeson, L., and Senbeta, F. (2017). Crop raiding by wild mammals in Ethiopia: impacts on the livelihoods of smallholders in an agriculture-forest mosaic landscape. Oryx 51, 527–537. doi: 10.1017/S0030605316000028
Balmford, A., Amano, T., Bartlett, H., Chadwick, D., Collins, A., Edwards, D., et al. (2018). The environmental costs and benefits of high-yield farming. Nat. Sustain. 1, 477–485. doi: 10.1038/s41893-018-0138-5
Bastin, J. F., Finegold, Y., Garcia, C., Mollicone, D., Rezende, M., Routh, D., et al. (2019). The global tree restoration potential. Science 365, 76–79. doi: 10.1126/science.aax0848
Bateman, I. J., and Mace, G. M. (2020). The natural capital framework for sustainably efficient and equitable decision making. Nat. Sustain. 3, 776–783. doi: 10.1038/s41893-020-0552-3
Bennett, N. J., Roth, R., Klain, S. C., Chan, K., Christie, P., Clark, D. A., et al. (2017). Conservation social science: understanding and integrating human dimensions to improve conservation. Biol. Conserv. 205, 93–108. doi: 10.1016/j.biocon.2016.10.006
Bergius, M., Benjaminsen, T. A., and Widgren, M. (2018). Green economy, Scandinavian investments and agricultural modernization in Tanzania. J. Peasant Stud. 45, 825–852. doi: 10.1080/03066150.2016.1260554
Bianchi, F. J. J. A., Booij, C. J. H., and Tscharntke, T. (2006). Sustainable pest regulation in agricultural landscapes: a review on landscape composition, biodiversity and natural pest control. Proc. R. Soc. B Biol. Sci. 273:1595. doi: 10.1098/rspb.2006.3530
Boetzl, F. A., Schuele, M., Krauss, J., and Steffan-Dewenter, I. (2020). Pest control potential of adjacent agri-environment schemes varies with crop type and is shaped by landscape context and within-field position. J. Appl. Ecol. 57, 1482–1493. doi: 10.1111/1365-2664.13653
Bommarco, R., Kleijn, D., and Potts, S. G. (2013). Ecological intensification: harnessing ecosystem services for food security. Trends Ecol. Evol. 28, 230–238. doi: 10.1016/j.tree.2012.10.012
Borrelli, P., Robinson, D. A., Fleischer, L. R., Lugato, E., Ballabio, C., Alewell, C., et al. (2017). An assessment of the global impact of 21st century land use change on soil erosion. Nat. Commun. 8:2013. doi: 10.1038/s41467-017-02142-7
Borrelli, P., Robinson, D. A., Panagos, P., Lugato, E., Yang, J. E., Alewell, C., et al. (2020). Land use and climate change impacts on global soil erosion by water (2015-2070). Proc. Natl. Acad. Sci. U.S.A. 117:36. doi: 10.1073/pnas.2001403117
Brosi, B. J., Daily, G. C., Shih, T. M., Oviedo, F., and Durán, G. (2008). The effects of forest fragmentation on bee communities in tropical countryside. J. Appl. Ecol. 45, 773–783. doi: 10.1111/j.1365-2664.2007.01412.x
Brown, S. E., Miller, D. C., Ordonez, P. J., and Baylis, K. (2018). Evidence for the impacts of agroforestry on agricultural productivity, ecosystem services, and human well-being in high-income countries: a systematic map protocol. Environ. Evid. 7:24. doi: 10.1186/s13750-018-0136-0
Brueckner-Irwin, I., Armitage, D., and Courtenay, S. (2019). Applying a social-ecological well-being approach to enhance opportunities for marine protected area governance. Ecol. Soc. 24:7. doi: 10.5751/ES-10995-240307
Carvalheiro, L. G., Seymour, C. L., Veldtman, R., and Nicolson, S. W. (2010). Pollination services decline with distance from natural habitat even in biodiversity-rich areas. J. Appl. Ecol. 47, 810–820. doi: 10.1111/j.1365-2664.2010.01829.x
Chambers, J. M., Wyborn, C., Ryan, M. E., Reid, R. S., Riechers, M., Serban, A., et al. (2021). Six modes of co-production for sustainability. Nat. Sustain. 4, 983–996. doi: 10.1038/s41893-021-00755-x
Chaudhary, A., and Mooers, A. O. (2018). Terrestrial vertebrate biodiversity loss under future global land use change scenarios. Sustainability 10:8. doi: 10.3390/su10082764
Chazdon, R. L. (2008). Beyond deforestation: restoring forests and ecosystem services on degraded lands. Science 320, 1458–1460. doi: 10.1126/science.1155365
Collins, M., Sutherland, M., Bouwer, L., Cheong, S.-M., Frölicher, T. L., Jacot Des Combes, H., et al. (2019). “Extremes, abrupt changes and managing risks,” in IPCC Special Report on the Ocean and Cryosphere in a Changing Climate, eds H.-O. Pörtner, D. C. Roberts, V. Masson-Delmotte, P. Zhai, M. Tignor, E. Poloczanska, et al. (Cambridge: Cambridge University Press).
Crist, E., Mora, C., and Engelman, R. (2017). The interaction of human population, food production, and biodiversity protection. Science 356, 260–264. doi: 10.1126/science.aal2011
Dainese, M., Martin, E. A., Aizen, M. A., Albrecht, M., Bartomeus, I., Bommarco, R., et al. (2019). A global synthesis reveals biodiversity-mediated benefits for crop production. Sci. Adv. 5:10. doi: 10.1126/sciadv.aax0121
Dasgupta, P. (2021). The Economics of Biodiversity: The Dasgupta Review - Full Report. London: HM Treasury.
Daw, T. M., Coulthard, S., Cheung, W. W. L., Brown, K., Abunge, C., Galafassi, D., et al. (2015). Evaluating taboo trade-offs in ecosystems services and human well-being. Proc. Natl. Acad. Sci. U.S.A. 112:201414900. doi: 10.1073/pnas.1414900112
Daw, T. M., Hicks, C. C., Brown, K., Chaigneau, T., Januchowski-Hartley, F. A., Cheung, W. W. L., et al. (2016). Elasticity in ecosystem services: exploring the variable relationship between ecosystems and human well-being. Ecol. Soc. 21:art11. doi: 10.5751/ES-08173-210211
Doggart, N., Morgan-Brown, T., Lyimo, E., Mbilinyi, B., Meshack, C. K., Sallu, S. M., et al. (2020). Agriculture is the main driver of deforestation in Tanzania. Environ. Res. Lett. 15:034028. doi: 10.1088/1748-9326/ab6b35
Dollinger, J., and Jose, S. (2018). Agroforestry for soil health. Agrofor. Syst. 92, 213–219. doi: 10.1007/s10457-018-0223-9
Dudley, N., Attwood, S. J., Goulson, D., Jarvis, D., Bharucha, Z. P., and Pretty, J. (2017). How should conservationists respond to pesticides as a driver of biodiversity loss in agroecosystems? Biol. Conserv. 209, 449–453. doi: 10.1016/j.biocon.2017.03.012
Duriaux Chavarría, J. Y., Baudron, F., and Sunderland, T. (2018). Retaining forests within agricultural landscapes as a pathway to sustainable intensification: evidence from Southern Ethiopia. Agric. Ecosyst. Environ. 263, 41–52. doi: 10.1016/j.agee.2018.04.020
Enns, C. (2018). Mobilizing research on Africa’s development corridors. Geoforum 88, 105–108. doi: 10.1016/j.geoforum.2017.11.017
European Space Agency [ESA] (2017). ESA Climate Change Initiative - Land Cover Project 2017. S2 Prototype LC 20m Map of Africa 2016. Paris: European Space Agency.
Ewers, R. M., and Banks-Leite, C. (2013). Fragmentation impairs the microclimate buffering effect of tropical forests. PLoS One 8:3. doi: 10.1371/journal.pone.0058093
Fischer, J., Abson, D. J., Butsic, V., Chappell, M. J., Ekroos, J., Hanspach, J., et al. (2014). Land sparing versus land sharing: moving forward. Conserv. Lett. 7, 149–157. doi: 10.1111/conl.12084
Fleischman, F., Basant, S., Chhatre, A., Coleman, E. A., Fischer, H. W., Gupta, D., et al. (2020). Pitfalls of tree planting show why we need people-centered natural climate solutions. Bioscience 70, 947–950. doi: 10.1093/BIOSCI/BIAA094
Folke, C., Biggs, R., Norström, A. V., Reyers, B., and Rockström, J. (2016). Social-ecological resilience and biosphere-based sustainability science. Ecol. Soc. 21:3. doi: 10.5751/ES-08748-210341
Food and Agriculture Organization [FAO] (2017). The Future of Food and Agriculture - Trends and Challenges. Rome: Food and Agriculture Organization.
Fründ, H.-C., Graefe, U., and Tischer, S. (2011). “Earthworms as bioindicators of soil quality,” in Biology of Earthworms, Soil Biology, 24, ed. A. Karaca (Berlin: Springer), doi: 10.1007/978-3-642-14636-7_16
Funk, C. C., and Brown, M. E. (2009). Declining global per capita agricultural production and warming oceans threaten food security. Food Secur. 1, 271–289. doi: 10.1007/s12571-009-0026-y
Gagic, V., Marcora, A., and Howie, L. (2019). Additive and interactive effects of pollination and biological pest control on crop yield. J. Appl. Ecol. 56, 2528–2535. doi: 10.1111/1365-2664.13482
Garibaldi, L. A., Pérez-Méndez, N., Garratt, M. P. D., Gemmill-Herren, B., Miguez, F. E., and Dicks, L. V. (2019). Policies for ecological intensification of crop production. Trends Ecol. Evol. 34, 282–286. doi: 10.1016/j.tree.2019.01.003
Gough, I., McGregor, J. A., and Camfield, L. (2007). “Theorising wellbeing in international development,” in Wellbeing in Developing Countries: From Theory to Research, eds I. Gough and J. McGregor (Cambridge: Cambridge University Press), 3–44. doi: 10.1017/CBO9780511488986.002
Grass, I., Loos, J., Baensch, S., Batáry, P., Librán-Embid, F., Ficiciyan, A., et al. (2019). Land-sharing/-sparing connectivity landscapes for ecosystem services and biodiversity conservation. People Nat. 1, 262–272. doi: 10.1002/pan3.21
Gunderson, L. H., Allen, C. R., and Holling, C. S. (2012). Foundations of Ecological Resilience. Washington, DC: Island Press.
Gurr, G. M., Lu, Z., Zheng, X., Xu, H., Zhu, P., Chen, G., et al. (2016). Multi-country evidence that crop diversification promotes ecological intensification of agriculture. Nat. Plants 2:16014. doi: 10.1038/NPLANTS.2016.14
Horlings, L. G., and Marsden, T. K. (2011). Towards the real green revolution? Exploring the conceptual dimensions of a new ecological modernisation of agriculture that could “feed the world”. Glob. Environ. Chang. 21, 441–452. doi: 10.1016/j.gloenvcha.2011.01.004
Howe, C., Suich, H., Vira, B., and Mace, G. M. (2014). Creating win-wins from trade-offs? Ecosystem services for human well-being: a meta-analysis of ecosystem service trade-offs and synergies in the real world. Glob. Environ. Chang. 28, 263–275. doi: 10.1016/j.gloenvcha.2014.07.005
IFAD, and UNEP (2013). Smallholders, Food Security and the Environment. Rome: International Fund for Agricultural Development.
IPBES (2018). “The IPBES regional assessment report on biodiversity and ecosystem services for Africa,” in Secretariat of the Intergovernmental Science-Policy Platform on Biodiversity and Ecosystem Services, eds E. Archer, L. Dziba, K. J. Mulongoy, M. A. Maoela, and M. Walters (Bonn: IPBES secretariat).
IPBES (2019). in Global Assessment Report on Biodiversity and Ecosystem Services of the Intergovernmental Science-Policy Platform on Biodiversity and Ecosystem Services, eds E. S. Brondizio, J. Settele, S. Díaz, and H. T. Ngo (Bonn: IPBES secretariat).
Isbell, F., Adler, P. R., Eisenhauer, N., Fornara, D., Kimmel, K., Kremen, C., et al. (2017). Benefits of increasing plant diversity in sustainable agroecosystems. J. Ecol. 105, 871–879. doi: 10.1111/1365-2745.12789
Isman, M. B. (2008). Botanical insecticides: for richer, for poorer. Pest Manag. Sci. 64, 8–11. doi: 10.1002/ps.1470
Jayne, T. S., Mather, D., and Mghenyi, E. (2010). Principal challenges confronting smallholder agriculture in sub-saharan Africa. World Dev. 38, 1384–1398. doi: 10.1016/j.worlddev.2010.06.002
Jenkins, R. K. B., Maliti, H. T., and Corti, G. R. (2003). Conservation of the puku antelope (Kobus vardoni, Livingstone) in the Kilombero Valley, Tanzania. Biodivers. Conserv. 12, 787–797. doi: 10.1023/A:1022426026881
Jones, T., Bamford, A. J., Ferrol-Schulte, D., Hieronimo, P., Mcwilliam, N., and Rovero, F. (2012). Vanishing wildlife corridors and options for restoration: a case study from Tanzania. Trop. Conserv. Sci. 5, 463–474. doi: 10.1177/194008291200500405
Jonsson, K., Ong, C. K., and Odongo, J. C. W. (1999). Influence of scattered nere and karite trees on microclimate, soil fertility and millet yield in Burkina Faso. Exp. Agric. 35:1. doi: 10.1017/S0014479799001039
Kangalawe, R. Y. M., and Liwenga, E. T. (2005). Livelihoods in the wetlands of Kilombero Valley in Tanzania: opportunities and challenges to integrated water resource management. Phys. Chem. Earth. 30, 968–975. doi: 10.1016/j.pce.2005.08.044
Kennedy, C. M., Lonsdorf, E., Neel, M. C., Williams, N. M., Ricketts, T. H., Winfree, R., et al. (2013). A global quantitative synthesis of local and landscape effects on wild bee pollinators in agroecosystems. Ecol. Lett. 16, 584–599. doi: 10.1111/ele.12082
Khan, Z. R., Midega, C. A. O., Amudavi, D. M., Hassanali, A., and Pickett, J. A. (2008). On-farm evaluation of the “push-pull” technology for the control of stemborers and striga weed on maize in western Kenya. F. Crop. Res. 106, 224–233. doi: 10.1016/j.fcr.2007.12.002
King, M. F., Renó, V. F., and Novo, E. M. L. M. (2014). The concept, dimensions and methods of assessment of human well-being within a socioecological context: a literature review. Soc. Indic. Res. 116, 681–698. doi: 10.1007/s11205-013-0320-0
Kørnøv, L., and Thissen, W. A. H. (2000). Rationality in decision- and policy-making: implications for strategic environmental assessment. Impact Assess. Proj. Apprais. 18, 191–200. doi: 10.3152/147154600781767402
Kravchenko, A. N., Snapp, S. S., and Robertson, G. P. (2017). Field-scale experiments reveal persistent yield gaps in low-input and organic cropping systems. Proc. Natl. Acad. Sci. U.S.A. 114, 926–931. doi: 10.1073/pnas.1612311114
Kremen, C. (2015). Reframing the land-sparing/land-sharing debate for biodiversity conservation. Ann. N. Y. Acad. Sci. 1355, 52–76. doi: 10.1111/nyas.12845
Lasco, R. D., Delfino, R. J. P., Catacutan, D. C., Simelton, E. S., and Wilson, D. M. (2014). Climate risk adaptation by smallholder farmers: the roles of trees and agroforestry. Curr. Opin. Environ. Sustain. 6, 83–88. doi: 10.1016/j.cosust.2013.11.013
Laurance, W. F., Sloan, S., Weng, L., and Sayer, J. A. (2015). Estimating the environmental costs of Africa’s massive “Development Corridors”. Curr. Biol. 25, 3202–3208. doi: 10.1016/j.cub.2015.10.046
Lehmann, P., Ammunét, T., Barton, M., Battisti, A., Eigenbrode, S. D., Jepsen, J. U., et al. (2020). Complex responses of global insect pests to climate warming. Front. Ecol. Environ. 18:141–150. doi: 10.1002/fee.2160
Li, M., Peterson, C. A., Tautges, N. E., Scow, K. M., and Gaudin, A. C. M. (2019). Yields and resilience outcomes of organic, cover crop, and conventional practices in a Mediterranean climate. Sci. Rep. 9:12283. doi: 10.1038/s41598-019-48747-4
Lin, B. B., Perfecto, I., and Vandermeer, J. (2008). Synergies between agricultural intensification and climate change could create surprising vulnerabilities for crops. Bioscience 58, 847–854. doi: 10.1641/B580911
Lobell, D. B., Bänziger, M., Magorokosho, C., and Vivek, B. (2011). Nonlinear heat effects on African maize as evidenced by historical yield trials. Nat. Clim. Chang. 1, 42–45. doi: 10.1038/nclimate1043
Loveridge, R., Sallu, S. M., Pesha, I. J., and Marshall, A. R. (2020). Measuring human wellbeing: a protocol for selecting local indicators. Environ. Sci. Policy 114, 461–469. doi: 10.1016/j.envsci.2020.09.002
Malézieux, E., Crozat, Y., Dupraz, C., Laurans, M., Makowski, D., Ozier-Lafontaine, H., et al. (2009). Mixing plant species in cropping systems: concepts, tools and models. A review. Agron. Sustain. Dev. 29, 43–62. doi: 10.1051/agro:2007057
Marsden, C., Martin-Chave, A., Cortet, J., Hedde, M., and Capowiez, Y. (2020). How agroforestry systems influence soil fauna and their functions - a review. Plant Soil 453, 29–44. doi: 10.1007/s11104-019-04322-4
Mbow, C., Van Noordwijk, M., Luedeling, E., Neufeldt, H., Minang, P. A., and Kowero, G. (2014). Agroforestry solutions to address food security and climate change challenges in Africa. Curr. Opin. Environ. Sustain. 6, 61–67. doi: 10.1016/j.cosust.2013.10.014
McGregor, J. A. (2018). “Reconciling universal frameworks and local realities in understanding and measuring wellbeing,” in The Politics of Wellbeing: Theory, Policy and Practice, eds I. Bache and K. Scott (Cham: Palgrave Macmillan), doi: 10.1007/978-3-319-58394-5_9
McKinnon, M. C., Cheng, S. H., Dupre, S., Edmond, J., Garside, R., Glew, L., et al. (2016). What are the effects of nature conservation on human well-being? A systematic map of empirical evidence from developing countries. Environ. Evid. 5:8. doi: 10.1186/s13750-016-0058-7
McShane, T. O., Hirsch, P. D., Trung, T. C., Songorwa, A. N., Kinzig, A., Monteferri, B., et al. (2011). Hard choices: making trade-offs between biodiversity conservation and human well-being. Biol. Conserv. 144, 966–972. doi: 10.1016/j.biocon.2010.04.038
Millennium Ecosystem Assessment [MEA] (2003). Millennium Ecosystem Assessment: Ecosystems and Human Well-Being - A Framework for Assessment. Washington DC: World Resources Institute.
Milner-Gulland, E. J., McGregor, J. A., Agarwala, M., Atkinson, G., Bevan, P., Clements, T., et al. (2014). Accounting for the impact of conservation on human well-being. Conserv. Biol. 28, 1160–1166. doi: 10.1111/cobi.12277
Mukeka, J. M., Ogutu, J. O., Kanga, E., and Røskaft, E. (2019). Human-wildlife conflicts and their correlates in Narok County, Kenya. Glob. Ecol. Conserv. 18:e620. doi: 10.1016/j.gecco.2019.e00620
Munishi, S., and Jewitt, G. (2019). Degradation of Kilombero Valley Ramsar wetlands in Tanzania. Phys. Chem. Earth 112, 216–227. doi: 10.1016/j.pce.2019.03.008
Ndoli, A., Baudron, F., Schut, A. G. T., Mukuralinda, A., and Giller, K. E. (2017). Disentangling the positive and negative effects of trees on maize performance in smallholdings of Northern Rwanda. F. Crop. Res. 213, 1–11. doi: 10.1016/j.fcr.2017.07.020
Newbold, T., Hudson, L. N., Hill, S. L. L., Contu, S., Lysenko, I., Senior, R. A., et al. (2015). Global effects of land use on local terrestrial biodiversity. Nature 520, 45–50. doi: 10.1038/nature14324
Nyhus, P. J. (2016). Human-wildlife conflict and coexistence. Annu. Rev. Environ. Resour. 41, 143–171. doi: 10.1146/annurev-environ-110615-085634
Ojedokun, C. A., Ugege, B. H., Kolade, R. I., Tunde-Francis, A. A., and Odediran, F. A. (2020). Contribution of agroforestry to farmers wellbeing in forest enclave, Edo State, Nigeria. J. Appl. Sci. Environ. Manag. 24, 1363–1367. doi: 10.4314/jasem.v24i8.9
Oldfield, E. E., Bradford, M. A., and Wood, S. A. (2019). Global meta-analysis of the relationship between soil organic matter and crop yields. Soil 5, 15–32. doi: 10.5194/soil-5-15-2019
Paavola, J. (2008). Livelihoods, vulnerability and adaptation to climate change in Morogoro, Tanzania. Environ. Sci. Policy 11, 642–654. doi: 10.1016/j.envsci.2008.06.002
Palmer Fry, B., Agarwala, M., Atkinson, G., Clements, T., Homewood, K., Mourato, S., et al. (2017). Monitoring local well-being in environmental interventions: a consideration of practical trade-offs. Oryx 51, 68–76. doi: 10.1017/S003060531500112X
Pellegrini, P., and Fernández, R. J. (2018). Crop intensification, land use, and on-farm energy-use efficiency during the worldwide spread of the green revolution. Proc. Natl. Acad. Sci. U.S.A. 115, 2335–2340. doi: 10.1073/pnas.1717072115
Peng, S. S., Piao, S., Zeng, Z., Ciais, P., Zhou, L., Li, L. Z. X., et al. (2014). Afforestation in China cools local land surface temperature. Proc. Natl. Acad. Sci. U.S.A. 111, 2915–2919. doi: 10.1073/pnas.1315126111
Pfeifer, M., Lefebvre, V., Peres, C. A., Banks-Leite, C., Wearn, O. R., Marsh, C. J., et al. (2017). Creation of forest edges has a global impact on forest vertebrates. Nature 551:187. doi: 10.1038/nature24457
Power, A. G. (2010). Ecosystem services and agriculture: tradeoffs and synergies. Philos. Trans. R. Soc. Lond. B. Biol. Sci. 365, 2959–2971. doi: 10.1098/rstb.2010.0143
Pretty, J., and Bharucha, Z. P. (2015). Integrated pest management for sustainable intensification of agriculture in Asia and Africa. Insects 6, 152–182. doi: 10.3390/insects6010152
Ramankutty, N., Mehrabi, Z., Waha, K., Jarvis, L., Kremen, C., Herrero, M., et al. (2018). Trends in global agricultural land use: implications for environmental health and food security. Annu. Rev. Plant Biol. 69, 789–815. doi: 10.1146/annurev-arplant-042817-040256
Rasmussen, L. V., Coolsaet, B., Martin, A., Mertz, O., Pascual, U., Corbera, E., et al. (2018). Social-ecological outcomes of agricultural intensification. Nat. Sustain. 1, 275–282. doi: 10.1038/s41893-018-0070-8
Rendón, O. R., Garbutt, A., Skov, M., Möller, I., Alexander, M., Ballinger, R., et al. (2019). A framework linking ecosystem services and human well-being: saltmarsh as a case study. People Nat. 1, 486–496. doi: 10.1002/pan3.10050
Reyes-García, V., Babigumira, R., Pyhälä, A., Wunder, S., Zorondo-Rodríguez, F., and Angelsen, A. (2016). Subjective wellbeing and income: empirical patterns in the rural developing world. J. Happ. Stud. 17, 773–791. doi: 10.1007/s10902-014-9608-2
Robards, M. D., Schoon, M. L., Meek, C. L., and Engle, N. L. (2011). The importance of social drivers in the resilient provision of ecosystem services. Glob. Environ. Chang. 21, 522–529. doi: 10.1016/j.gloenvcha.2010.12.004
Rosenstock, T. S., Tully, K. L., Arias-Navarro, C., Neufeldt, H., Butterbach-Bahl, K., and Verchot, L. V. (2014). Agroforestry with N2-fixing trees: sustainable development’s friend or foe? Curr. Opin. Environ. Sustain. 6, 15–21. doi: 10.1016/j.cosust.2013.09.001
Sánchez-Romero, R., Balvanera, P., Castillo, A., Mora, F., García-Barrios, L. E., and González-Esquivel, C. E. (2021). Management strategies, silvopastoral practices and socioecological drivers in traditional livestock systems in tropical dry forests: an integrated analysis. For. Ecol. Manage. 479:118506. doi: 10.1016/j.foreco.2020.118506
Schrama, M., de Haan, J. J., Kroonen, M., Verstegen, H., and Van der Putten, W. H. (2018). Crop yield gap and stability in organic and conventional farming systems. Agric. Ecosyst. Environ. 256, 123–130. doi: 10.1016/j.agee.2017.12.023
Shaffer, L. J., Khadka, K. K., Van Den Hoek, J., and Naithani, K. J. (2019). Human-elephant conflict: a review of current management strategies and future directions. Front. Ecol. Evol. 6:235. doi: 10.3389/fevo.2018.00235
Sida, T. S., Baudron, F., Kim, H., and Giller, K. E. (2018). Climate-smart agroforestry: Faidherbia albida trees buffer wheat against climatic extremes in the Central Rift Valley of Ethiopia. Agric. For. Meteorol. 248, 339–347. doi: 10.1016/j.agrformet.2017.10.013
Sinclair, F. L. (1999). A general classification of agroforestry practice. Agrofor. Syst. 46, 161–180. doi: 10.1023/A:1006278928088
Sirami, C., Gross, N., Baillod, A. B., Bertrand, C., Carrié, R., Hass, A., et al. (2019). Increasing crop heterogeneity enhances multitrophic diversity across agricultural regions. Proc. Natl. Acad. Sci. U.S.A. 116, 16442–16447. doi: 10.1073/pnas.1906419116
Smith, H. E., Ryan, C. M., Vollmer, F., Woollen, E., Keane, A., Fisher, J. A., et al. (2019). Impacts of land use intensification on human wellbeing: evidence from rural Mozambique. Glob. Environ. Chang. 59:101976. doi: 10.1016/j.gloenvcha.2019.101976
Southern Agricultural Growth Corridor of Tanzania [SAGCOT] (2011). Southern Agricultural Growth Investment Blueprint. dar es salaam: Southern Agricultural Growth Corridor of Tanzania.
Strassburg, B. B. N., Iribarrem, A., Beyer, H. L., Cordeiro, C. L., Crouzeilles, R., Jakovac, C. C., et al. (2020). Global priority areas for ecosystem restoration. Nature 586, 724–729. doi: 10.1038/s41586-020-2784-9
Suich, H., Howe, C., and Mace, G. (2015). Ecosystem services and poverty alleviation: a review of the empirical links. Ecosyst. Serv. 12, 137–147. doi: 10.1016/j.ecoser.2015.02.005
Teixeira, F. Z., Bachi, L., Blanco, J., Zimmermann, I., Welle, I., and Carvalho-Ribeiro, S. M. (2019). Perceived ecosystem services (ES) and ecosystem disservices (EDS) from trees: insights from three case studies in Brazil and France. Landsc. Ecol. 34, 1583–1600. doi: 10.1007/s10980-019-00778-y
Tilman, D., Cassman, K. G., Matson, P. A., Naylor, R., and Polasky, S. (2002). Agricultural sustainability and intensive production practices. Nature 418, 671–677. doi: 10.1038/nature01014
Torralba, M., Fagerholm, N., Burgess, P. J., Moreno, G., and Plieninger, T. (2016). Do European agroforestry systems enhance biodiversity and ecosystem services? A meta-analysis. Agric. Ecosyst. Environ. 230, 150–161. doi: 10.1016/j.agee.2016.06.002
Trimmer, J. T., Bauza, V., Byrne, D. M., Lardizabal, A., and Guest, J. S. (2017). Harmonizing goals for agricultural intensification and human health protection in Sub-Saharan Africa. Trop. Conserv. Sci. 10, 1–6. doi: 10.1177/1940082917720666
Tscharntke, T., Clough, Y., Bhagwat, S. A., Buchori, D., Faust, H., Hertel, D., et al. (2011). Multifunctional shade-tree management in tropical agroforestry landscapes - A review. J. Appl. Ecol. 48, 619–629. doi: 10.1111/j.1365-2664.2010.01939.x
Tscharntke, T., Klein, A. M., Kruess, A., Steffan-Dewenter, I., and Thies, C. (2005). Landscape perspectives on agricultural intensification and biodiversity–ecosystem service management. Ecol. Lett. 8, 857–874.
Tscharntke, T., Tylianakis, J. M., Rand, T. A., Didham, R. K., Fahrig, L., Batáry, P., et al. (2012). Landscape moderation of biodiversity patterns and processes - eight hypotheses. Biol. Rev. 87, 661–685. doi: 10.1111/j.1469-185X.2011.00216.x
UNEP-WCMC, and IUCN (2021). Protected Planet: The World Database on Protected Areas (WDPA) and World Database on Other Effective Area-based Conservation Measures (WD-OECM) [Online]. Cambridge: UNEP-WCMC.
Van Groenigen, J. W., Lubbers, I. M., Vos, H. M. J., Brown, G. G., De Deyn, G. B., and Van Groenigen, K. J. (2014). Earthworms increase plant production: a meta-analysis. Sci. Rep. 4:6365. doi: 10.1038/srep06365
Van Ittersum, M. K., Van Bussel, L. G. J., Wolf, J., Grassini, P., Van Wart, J., Guilpart, N., et al. (2016). Can sub-Saharan Africa feed itself? Proc. Natl. Acad. Sci. U.S.A. 113, 14964–14969. doi: 10.1073/pnas.1610359113
Vialatte, A., Barnaud, C., Blanco, J., Ouin, A., Choisis, J. P., Andrieu, E., et al. (2019). A conceptual framework for the governance of multiple ecosystem services in agricultural landscapes. Landsc. Ecol. 34, 1653–1673. doi: 10.1007/s10980-019-00829-4
Webb, N. P., Marshall, N. A., Stringer, L. C., Reed, M. S., Chappell, A., and Herrick, J. E. (2017). Land degradation and climate change: building climate resilience in agriculture. Front. Ecol. Environ. 15:450–459. doi: 10.1002/fee.1530
Williams, A., Jordan, N. R., Smith, R. G., Hunter, M. C., Kammerer, M., Kane, D. A., et al. (2018). A regionally-adapted implementation of conservation agriculture delivers rapid improvements to soil properties associated with crop yield stability. Sci. Rep. 8:8467. doi: 10.1038/s41598-018-26896-2
Winfree, R., Reilly, J. R., Bartomeus, I., Cariveau, D. P., Williams, N. M., and Gibbs, J. (2018). Species turnover promotes the importance of bee diversity for crop pollination at regional scales. Science 359, 791–793. doi: 10.1126/SCIENCE.AAO2117
Woodhouse, E., Homewood, K. M., Beauchamp, E., Clements, T., McCabe, J. T., Wilkie, D., et al. (2015). Guiding principles for evaluating the impacts of conservation interventions on human well-being. Philos. Trans. R. Soc. B 370:20150103. doi: 10.1098/rstb.2015.0103
Keywords: biodiversity, ecosystem restoration, sustainable livelihoods, Africa, rural development, multifunctionality
Citation: Milheiras SG, Sallu SM, Marshall AR, Shirima DD, Kioko EN, Loveridge R, Moore E, Olivier P, Teh YA, Rushton S and Pfeifer M (2022) A Framework to Assess Forest-Agricultural Landscape Management for Socioecological Well-Being Outcomes. Front. For. Glob. Change 5:709971. doi: 10.3389/ffgc.2022.709971
Received: 14 May 2021; Accepted: 11 April 2022;
Published: 26 May 2022.
Edited by:
Marco Marchetti, University of Molise, ItalyReviewed by:
Lowe Börjeson, Stockholm University, SwedenLouise Elizabeth Buck, Cornell University, United States
Copyright © 2022 Milheiras, Sallu, Marshall, Shirima, Kioko, Loveridge, Moore, Olivier, Teh, Rushton and Pfeifer. This is an open-access article distributed under the terms of the Creative Commons Attribution License (CC BY). The use, distribution or reproduction in other forums is permitted, provided the original author(s) and the copyright owner(s) are credited and that the original publication in this journal is cited, in accordance with accepted academic practice. No use, distribution or reproduction is permitted which does not comply with these terms.
*Correspondence: Sergio G. Milheiras, c21pbGhlaXJhc0BnbWFpbC5jb20=