- 1Faculty of Forestry, Institute for Dehesa Research (INDEHESA), Universidad de Extremadura, Plasencia, Spain
- 2Centre of Environmental and Marine Studies (CESAM), Department of Biology, University of Aveiro, Aveiro, Portugal
- 3Department of Plant Biology, Ecology and Earth Sciences, Faculty of Science, Universidad de Extremadura, Badajoz, Spain
- 4School of Agricultural Engineering, Institute for Dehesa Research (INDEHESA), Universidad de Extremadura, Badajoz, Spain
Chestnut forests are undergoing increasing heat stress due to the current global warming, but little is known about the physiology and biochemistry responses of Castanea sativa Mill. to heat or whether differences exist between populations. Six-month-old seedlings from three climatically contrasting populations of C. sativa (from the north, centre, and south of Spain) were subjected to control and heat stress conditions for 7 days. The effects of heat stress on seedlings and their recovery (10 days after heat stress) were described by assessment of visible symptoms, growth, mortality, and leaf gas exchange of plants, quantification of compounds involved in the primary and secondary metabolism, and 2,2-diphenyl-1-picrylhydrazyl (DPPH) free radical scavenging. In response to stress, plant biomass decreased, and plant biochemistry altered depending on the tissue and the population. Major alterations in the primary metabolism of stressed plants occurred in leaves, characterized by increased levels of soluble sugars, nitrogen, and proline, and depletion of starch. Increased levels of soluble sugars and starch depletion occurred mostly in seedlings from the southern population, while proline increase occurred only in the northern population. Secondary metabolism of seedlings experienced the highest variation below ground, and roots of heat-stressed plants increased the content of phenolic compounds. LC-MS analysis permitted identification and quantification of six compounds induced by heat, five of which were detected in the roots. Differential biochemistry responses to heat stress were observed among populations. At recovery, most of the altered parameters had returned to control conditions, suggesting high resilience to heat stress in this Mediterranean tree species. This is the first study to address the effects of heat stress on the physiology and biochemistry of C. sativa and their interpopulation variability. Most parameters were significantly influenced by the interaction of population and heat treatment, indicating that genetic differentiation controlled the phenotypic differences of C. sativa in response to heat stress. Extensive genetic variation in plasticity in physiological and biochemical parameters in response to heat stress reveals an opportunity for chestnut for global warming-mediated selection.
Introduction
Heat stress is defined as an increase in temperature above a threshold sufficient to cause irreversible damage to the plant (Wahid, 2007). Visible damage observed from heat stress includes leaf and branch burn, leaf senescence and abscission, or inhibition of shoot and root growth (Wahid et al., 2007; Hasanuzzaman et al., 2013; He et al., 2021), impacting plant productivity and vitality. Multiple physiological and biochemical mechanisms underlie the visible damage caused by heat stress in plants (Wahid et al., 2007). Plant species generally react to heat by closing stomata (Marchin et al., 2022). When this occurs, plants will maintain hydraulic function at the expense of leaves overheating (because of reduced transpiration) and carbon assimilation reduction (because of reduced photosynthesis) (Ruehr et al., 2015). Heat can directly impact photosynthetic rates by reducing enzyme activity, mainly Rubisco, and damaging photosystem II (Bhagat et al., 2014; Nievola et al., 2017; Birami et al., 2018) through accumulation of reactive oxygen species (ROS). To protect themselves from oxidative stress, plants use both enzymatic (e.g., superoxide dismutase and ascorbate peroxidase) and non-enzymatic (e.g., phenolic acids and flavonoids) antioxidant machinery to break down and scavenge free radicals and/or disrupt their reaction chains (Ahmad et al., 2010; Shivashankara et al., 2016; Poudel and Poudel, 2020).
Plants synthesize a large diversity of low molecular weight compounds such as carbohydrates, secondary metabolites (phenolic acids, flavonols, and lignins) and hormones that are essential for plant acclimatisation, survival, and recovery under abiotic stress (Martín et al., 2008a; Zandalinas et al., 2017). Non-structural carbohydrates (NSCs), including soluble sugars and starch, are a small fraction of the carbohydrates acquired by the plant via photosynthesis (Martínez-Vilalta et al., 2016). However, NSCs play a crucial role in plant survival under environmental stress by buffering the asynchrony of substrate supply and demand (Galiano et al., 2011; Wiley and Helliker, 2012; Hartmann et al., 2013; Dietze et al., 2014; O’Brien et al., 2014; Li et al., 2018). Most secondary metabolite compounds are synthesized from products of primary carbon metabolism (Wahid and Ghazanfar, 2006). Phenolic compounds are an important class of secondary metabolites, and their accumulation in plant tissues is considered an adaptive response of plants to adverse environmental conditions (Akhi et al., 2021). In crop plants, it has been reported that the accumulation of photoprotective and antioxidant secondary metabolites could be an adaptive mechanism to prevent damage from heat stress (Rivero et al., 2001; Zandalinas et al., 2017). In trees, heat-induced production of secondary metabolites appears to be species-, compound-, or even context-dependent (Berini et al., 2018). Under the current climate change scenario, understanding the impact of heat stress and heat waves on vegetation is a crucial challenge (Della-Marta et al., 2007; Spinoni et al., 2014; Rita et al., 2020) to anticipate socioeconomic losses and harm to natural ecosystems.
In response to climate change, forest tree populations have three possible fates: migration, adaptation, or extirpation (Aitken et al., 2008). The ability of trees to adapt to environmental changes depends on the genetics of their populations and on the ability to show plastic phenotypic responses (Aitken and Whitlock, 2013; Alberto et al., 2013). Phenotypic plasticity is the phenomenon by which a genotype can produce different phenotypes in response to environmental conditions (Ghalambor et al., 2007). Phenotypic plasticity can be adaptive if it has a positive effect on plant fitness, maladaptive if it has a negative effect, or neutral if it has no effect (Scheiner, 1993). A thorough understanding of the plastic phenotypic responses of trees and their occurrence among populations is essential to predict their potential for adaptation to climate change (Valladares et al., 2014; Castellana et al., 2021). When a particularly adapted phenotype confers an advantage under climate change, this variant may invade the local population through migration and selection, and a local adaptation may emerge (Villemereuil et al., 2018). There is considerable evidence for local adaptation and genetic variation in plasticity (i.e., variation in phenotypic plasticity between tree populations) in Mediterranean tree species (Matesanz and Valladares, 2014; Zas et al., 2020; Blumstein and Hopkins, 2021; Vázquez-González et al., 2022), but there are no studies for some important forest species.
Chestnut (Castanea sativa Mill.) is a temperate thermophilic tree distributed throughout the Mediterranean basin in natural, semi-natural, and managed stands of considerable environmental, cultural, and economic value (Fernández-López and Alía, 2003; Conedera et al., 2004; Fernández-López et al., 2021). Its main area of distribution in Spain is in the humid northwest region, as well as scattered stands in central and southern Iberia, where high temperatures are frequent (Fernández-López et al., 2021). Chestnut forests in Spain are threatened by global warming (e.g., May and July air temperatures hit a record high in 2022), providing an ideal testbed for studying heat stress effects. Differences in rainfall between northern and south-central Spanish populations have resulted in differentiation of two chestnut ecotypes (mesophytic in northern Spain and xeric in south-central Spain) with different adaptive responses to water stress (Míguez-Soto and Fernández-López, 2015; Alcaide et al., 2019; Míguez-Soto et al., 2019). Drought is therefore a selective force shaping the differentiation of chestnut populations (Díaz et al., 2009; Míguez-Soto and Fernández-López, 2015) and heat stress is also responsible for this differentiation (Dorado et al., 2022). Little is known about the response of C. sativa to heat stress or whether contrasting climate habitats involve differential responses to higher temperatures. The efficacy of fertilizers in chestnut tolerance to high temperatures (Carneiro-Carvalho et al., 2021) and the effect of temperature (Gomes-Laranjo et al., 2006) on some chestnut cultivars has been studied. However, no studies addressed the effects of high temperatures on the physiology and biochemistry of this species. The objectives of this study were to (i) analyse the impact of heat stress on the physiology and biochemistry of C. sativa seedlings from contrasting climate conditions, (ii) quantify the response of chestnut seedlings to heat stress when temperatures lower, and (iii) to identify genetic variation and phenotypic plastic responses to heat stress in C. sativa seedlings from different climatic regions.
Materials and methods
Plant material
In November 2018, C. sativa seeds were collected from 16 adult trees per population in three wild populations in Spain (Puebla de Sanabria, Valle de Matamoros, and Paterna del Río). The three populations had contrasting climates in terms of precipitation and absolute maximum temperature (Table 1). Puebla de Sanabria is in northern Spain, close to the Transmontane region of northeast Portugal, known as Cold Land (“Terra Fria”), and has ca. 1,000 mm rainfall per year and belongs to the supra-Mediterranean bioclimatic zone (Table 1). Valle de Matamoros is in the foothills of Sierra Morena (centre-south of Spain), is characterized by 740 mm annual rainfall and belongs to the meso-Mediterranean bioclimatic zone (Table 1). Paterna del Río is in the Alpujarras, on the southern slopes of Sierra Nevada, has ca. 650 mm rainfall per year and belongs to the meso-Mediterranean bioclimatic (Table 1). For clarity, the populations were named northern, central, and southern populations, respectively. In each chestnut population, 16 fruit-bearing trees were selected at least 70 m apart to minimize the chances of sampling intercrossed individuals.
In November 2018, about 100 seeds collected from each of the 16 trees per population were immersed in a fungicide solution (2 g L–1 Thiram 80GD, ADAMA Inc., Spain) for 5 min, and those that floated were discarded as non-viable. Seeds were rinsed with sterilized water and stored at 4 °C for 2 weeks. In December 2018, they were individually weighed and sown at 1 cm depth in 48-cell plastic root trainers with one seed per cell. Individual cells were 330 ml in volume, 18 cm high, 5.3 cm × 5.3 cm upper surface, and contained peat (PKN1 Florava® Peat Substrate; pH = 4.5). Germination was considered successful when the aerial part emerging from the embryo was green. Aerial emergence of plants was assessed weekly. Plants were kept in natural daylight under greenhouse shade that reduced solar radiation by 50% and hand watered every 4 days to field capacity until they were well established. The greenhouse was at the Faculty of Forestry of Plasencia (40°02′ N, 6°04′ W; 374 m a.s.l., Extremadura region, Spain).
Experimental design and treatments
The experiment included seedlings from three populations, obtained from 16 mother trees in each population. Thirty seedlings per mother tree were used, of which half were subjected to ambient temperature (control), and half were subjected to a heat stress treatment. To reduce spatial thermal variability, the plants were arranged following a split-plot random design replicated in three blocks, with the heat treatments acting as the main factor (two categories: control and heat-stressed; whole plots) and the populations as the split factor (three categories: northern, central, and southern, as shown in Table 1; split plots). In the three blocks, the three populations were represented in each whole plot by five seedlings per mother tree. Seedlings were randomly positioned within the blocks. The experiment comprised 1,440 seedlings corresponding to 3 blocks × 2 treatments × 3 populations × 16 mother trees × 5 seedlings.
In May 2019, when the plants were 6 months old, 720 seedlings were subjected to ambient temperature, and 720 seedlings were subjected to the heat treatment. From 11 a.m. to 5 p.m., mean temperatures of control and heat-stressed plants were 30.2 and 42.5°C, respectively. The time frame selected for applying heat stress simulated natural conditions of daily temperature increase during summer in the Mediterranean Basin, with a maximum temperature from midday to early afternoon. The heat stress treatment comprised placing the plants inside a climate chamber with translucent walls in the same greenhouse. During treatments, both groups of plants were well irrigated. Volumetric soil water content (VWC), a relative measure of soil moisture, was maintained at values around 30% by irrigating once a day the control plants and by irrigating twice a day the heat stressed plants. Previous work indicated that 30% VWC was optimal for chestnut growth (Camisón et al., 2020). VWC was verified using a TDR 100 soil moisture meter (Spectrum Technologies Inc., Plainfield, IL, USA) and 12-cm-long rods in 10 cells per block per treatment. The leaves of control plants were green and turgid throughout the experiment (Figure 1A). During treatments, the relative air humidity and light conditions of both groups of plants were similar (≈80% and PAR ≈ 850 μmol photons m–2 s–1). The heat treatments lasted 7 days (day 7, “heat,” Supplementary Figure 1). After the heat treatments ended, heat-stressed seedlings were kept under control conditions for 10 days to assess their recovery (day 17, “recovery phase,” Supplementary Figure 1).
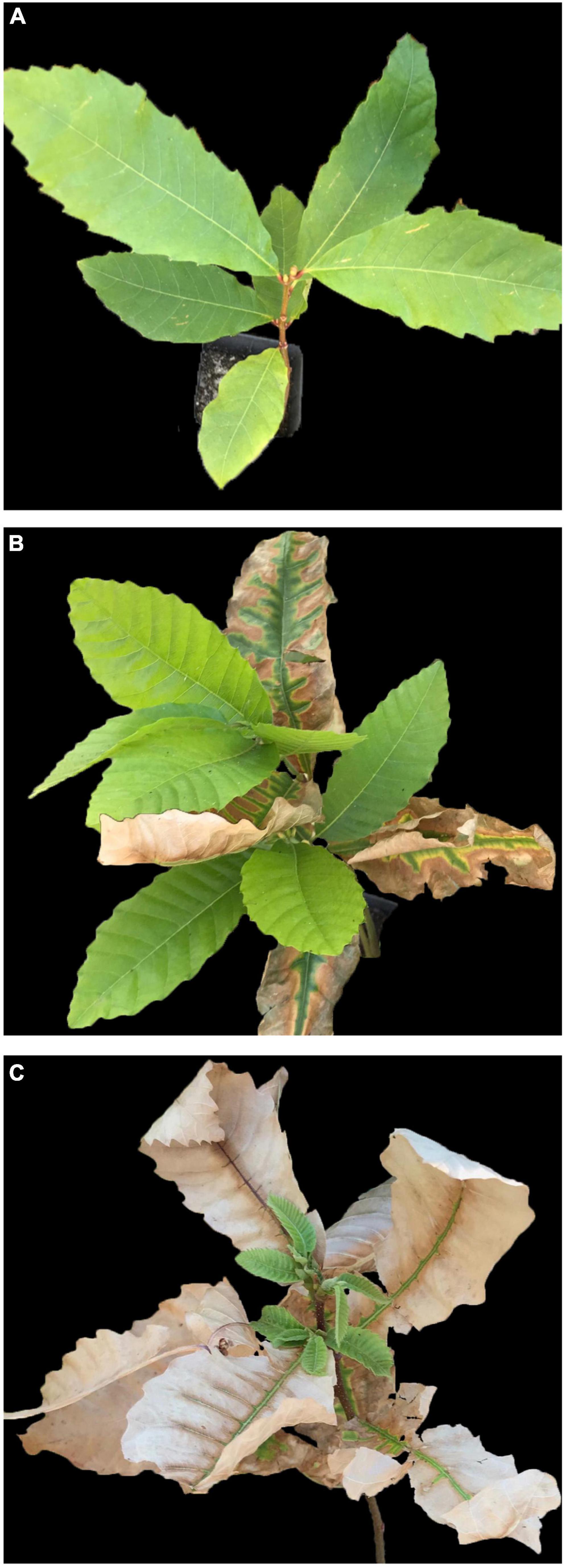
Figure 1. Six-month-old Castanea sativa seedlings exposed to ambient conditions (A), heat stress conditions for 7 days (B), and ambient conditions for 10 days after heat stress ceased (C). Heat scorching is observed on the foliage of stressed plants, starting with browning of the leaf margins, and/or yellowing or darkening of the areas between the main leaf veins. Ten days after heat treatment ceased, the foliage of the scorch-affected plant finally wilted, but the buds were viable, and the plant started to resprout.
Plant measurements and sampling
Measurements and sampling for biochemical analysis were performed on the first day of treatments (day 1), the last day of treatments (day 7, heat; Supplementary Figure 1), and 10 days after treatments ceased (day 17, recovery phase; Supplementary Figure 1) when plants were in recovery from stress (Figure 1C). On day 1, leaf gas exchange parameters were measured to observe the photosynthetic response of the populations to the increase in temperature. In the heat and recovery phases, the effects of heat on seedlings were evaluated by (i) foliar symptoms, leaf wilting, plant growth, and mortality; (ii) leaf gas exchange; (iii) quantification of primary metabolism parameters (NSC and proline) and N content; and (iv) quantification of secondary metabolism parameters (total phenolic compounds and ortho-phenolic and flavonoid compounds), 2,2-diphenyl-1-picrylhydrazyl (DPPH) free radical scavenging, and non-targeted metabolite profiling.
Leaf gas exchange parameters–net photosynthetic rate (Pn), transpiration rate (E), and stomatal conductance (gs)–were determined on the first day of treatments (day 1) in 15 seedlings per population (five per block) and treatment. In the heat and recovery phases, leaf gas exchange parameters were measured in 10 seedlings per population using a portable differential infrared gas analyser (IRGA; Li-6400, Li-Cor INC., Lincoln, NE, USA) connected to a broadleaf chamber and a temperature sensor. On day 1, measurements were performed from 9 a.m. to 2 p.m., with temperature ranging from 32 to 48°C, photosynthetically active radiation (PAR) ranging from 500 to 800 μmol photons m–2 s–1 (daylight and variable PAR conditions), and ambient CO2 concentration ranging from 390 to 410 μmol mol–1. In the heat phase, temperatures ranged from 23 to 27°C and 37 to 42°C in the control and heat treatments, respectively. In the recovery phase, temperatures ranged from 23 to 27°C for both treatments. To reduce errors associated with CO2 concentration and PAR changes over time, leaf gas exchange measurements were carried out by systematically alternating seedlings from the three populations (day 1) or by systematically alternating seedlings from the three populations and two treatments (heat and recovery phases). Instantaneous and intrinsic water use efficiencies were calculated based on leaf gas exchange, using the formulae instantaneous WUE = Pn/E and intrinsic WUE = Pn/gs, respectively.
Eighteen plants per population and treatment were destructively harvested for primary and secondary metabolite analysis. After collection, samples from three different seedlings were pooled to obtain a final sample size of six pools per population and treatment. Samples were immediately frozen in liquid nitrogen and stored at −80°C. To account for the diurnal oscillation of NSC content in plant tissues (Tixier et al., 2018) and to ensure the results were comparable, plants were sampled from 2.00 p.m. to 3.00 p.m. Leaves, aerial woody tissues (stem plus small twigs, “stems”) and roots (taproot plus fine roots, “roots”) of each seedling were used to determine dry biomass and analyse N and NSC. Within 30 min of harvesting, excised organs were subjected to microwave treatment (80 s, 800 W) to inactivate the enzymes and fix the actual NSC content (Hoch et al., 2002). Samples were oven dried (48 h, 60°C), weighed on a precision balance for dry biomass quantification, and ground to a fine powder in a ball mill (Mixer Mill MM 400, Retsch, Germany) to pass through a 0.42 mm mesh screen.
For analyses of proline, secondary metabolites (total phenolic compounds and ortho-phenolic and flavonoid compounds), 2,2-diphenyl-1-picrylhydrazyl (DPPH) free radical scavenging and non-targeted metabolite profiling, leaves, and roots of seedlings from the two treatments were sampled. For leaves, those that were fully developed near the shoot tip were sampled. For roots, the outermost fine roots were collected after carefully lifting the root ball from the tray.
Assessment of external symptoms, wilting, growth, and mortality
External symptom evaluation comprised visual characterisation of the foliar discolouration observed on seedlings. Wilting percentage was determined by visual estimation of the percentage of wilted leaves in plants subjected to heat stress in 10% intervals following Alcaide et al. (2019). Total plant dry biomass, comprising the dry weight of tissues used for NSC and nitrogen determination, was used as an indicator of plant growth.
Primary metabolism
Total NSCs included the sum of soluble sugars and starch. Soluble sugars and starch in leaf, stem, and root tissues were determined following the protocol adapted for chestnut by Camisón et al. (2020). Soluble sugar:starch ratios were calculated in each plant tissue and used as a proxy for mobilisation of starch to soluble sugars (Piper, 2011).
Total N in leaf, stem, and root tissues was determined by the Dumas method (DUMATHERM® CN, C-Gerhardt Analytical Systems) and expressed as a percentage of dry weight. Proline content was determined using the protocol of Bates et al. (1973), with slight modifications for chestnut by Camisón et al. (2021). NSC or N concentration at the whole plant level was calculated by a weighted mean, taking into account the total NSC or N concentration of each tissue and the relative contribution of each tissue’s biomass to plant total dry biomass, following: Whole plant NSC or N (%) = [(Xl × al + Xs × bs + Xr × cr)/(al + bs + cr)]; where Xl, Xs, and Xr are the total NSC or N concentrations in leaves, stems, and roots, respectively, and al, bs, and cr are the proportions in which each tissue contributes to plant dry biomass.
Secondary metabolism
Phenolic compounds and 2,2-diphenyl-1-picrylhydrazyl (DPPH) free radical scavenging
Powdered leaf or root samples (50 mg) were homogenised with 1.5 ml of 70% aqueous methanol and shaken in an orbital shaker for 1 h (700 rpm at 25°C). After centrifugation (15 min at 4°C and 10,000 g), the supernatant was collected, and the process was repeated three times (to 6 ml volume). The extract was stored at −80°C for further determination of total phenolic compounds, ortho-phenolic, and flavonoid compounds, and DPPH free radical scavenging.
Total phenolic and ortho-phenolic contents were estimated by the Folin-Ciocalteu method and the sodium molybdate colourimetric assay, respectively, both adapted from Singleton et al. (1999). Total flavonoid content was measured following the aluminium chloride colourimetric assay adapted from Chang et al. (2002). Total antioxidant capacity was measured using the 2,2-diphenyl-1-picrylhydrazyl (DPPH) free radical scavenging method adapted from Xu and Chang (2007).
Non-targeted metabolite profiling
Leaf or fine root powder (0.2 g) was homogenised with 70% ethanol (5 ml) and sonicated for 15 min. The extracts were cold macerated (4°C, 24 h), centrifuged (3500 rpm, 15 min), and the supernatant was filtered (0.45 μm filter). The filtered extracts were stored at −80°C until analysis.
Analysis, identification and quantification were performed with an LC-MS system (HPLC 1260–QTOF 6520, Agilent Technologies, Santa Clara, CA, USA). A total of 20 μL of filtered extract of each sample was injected onto a Spherisorb C-18 5 μ 250 × 4.6 mm reversed-phase analytical column at a rate of 1 ml/min. The mobile phase used was a water (2% formic acid)/methanol gradient from 1% water to 100% methanol in 100 min for leaves and 50 min for roots. Chromatograms were recorded at 350 nm and 280 nm wavelengths. Concentrations of the compounds were estimated from a standard curve (0.00, 0.01, 0.05, 0.10, and 0.20 mg/ml) using gallic acid, ellagic acid, quercetin, and quercetin 3-O-rutinoside depending on the nature of the compound to be quantified.
Statistical analysis
To determine how the increase in temperature affected gas exchange parameters, a global and population-based regression analysis was performed. To assess the effect of heat stress on the physiological and biochemical parameters of C. sativa seedlings, one-way analysis of variance (ANOVA) was performed using treatment as the only factor. To analyse the differential response of populations, two-way ANOVAs were performed, this time including the main effects of treatment, population, and their interaction as factors. Within each date (heat and recovery phase), Tukey’s multiple comparison tests were used to identify significant differences between treatments and populations (P < 0.05). Lack of differences between treatments for physiological and biochemical parameters indicated no phenotypic plasticity in response to heat stress, whereas significant differences between treatments indicated phenotypic plasticity in response to heat stress. A significant treatment × population interaction was interpreted as genetic variation in phenotypic plasticity of a parameter in response to heat stress.
Discriminant function analysis (DFA) was performed with compounds from the non-targeted metabolite profiling to differentiate the three C. sativa populations and their response to heat in the heat and recovery phases. The population × treatment interaction was used as a grouping variable, and the concentrations of the compounds (identified and unidentified) were used as independent variables. The number of compounds initially used as independent variables in the DFA was 18. The independent variables finally selected were obtained from a sequential variable forward stepwise method. The DFA analysis was performed with STATISTICA v10 software (StatSoft Inc, 2011), and the graphical representation was performed with R software (R Core Team, 2018).
Principal component analysis (PCA) was applied to detect patterns of variation in physiological and biochemical parameters due to origin (population), response to heat, and recovery. The PCA was performed using the R software “factoextra” statistical package (R Core Team, 2018). Before the PCA, the data were centred and standardised to reduce scale effects (Martín et al., 2008b). Relationships between leaf and root parameters used to perform PCA were assessed for the heat phase, separated into control, and heat stress conditions, using a Pearson’s correlation matrix performed with the R software “corrplot” package (R Core Team, 2018).
For each parameter and population, phenotypic plasticity in response to heat stress was expressed as a percentage relative to control plants following the equation proposed by Rehschuh et al. (2020) in the heat phase: Phenotypic plasticity (%) = [(x–Cpop)/Cpop) × 100]; where x is the heat-induced value of the parameter of a particular seedling of the population, and Cpop is the mean constitutive (control) value of the parameter of seedlings of the population. To check whether the effect of treatment differed between populations, one-way ANOVAs were performed using the population as the only factor. Before all analyses, data were checked for normality and homogeneity of variances. ANOVAs and Tukey’s tests were performed using STATISTICA v10 software (StatSoft Inc, 2011).
Results
Rapid response of leaf gas exchange parameters to temperature increase in C. sativa
On day 1, Pn and gs values in seedlings decreased exponentially as temperature increased (R2 = −0.90 and R2 = −0.59, respectively, P < 0.001; Supplementary Table 1), while E values increased as temperature increased (R2 = 0.28, P = 0.037). Gas exchange response to temperature increase depended on population (Figure 2). In seedlings from the northern population, E increased with temperature, while in seedlings from the central and southern populations, E was not significantly altered (Figure 2B). In seedlings from the northern population, gs did not change, while in seedlings from the central and southern populations, stomata closed as temperature increased (Figure 2C).
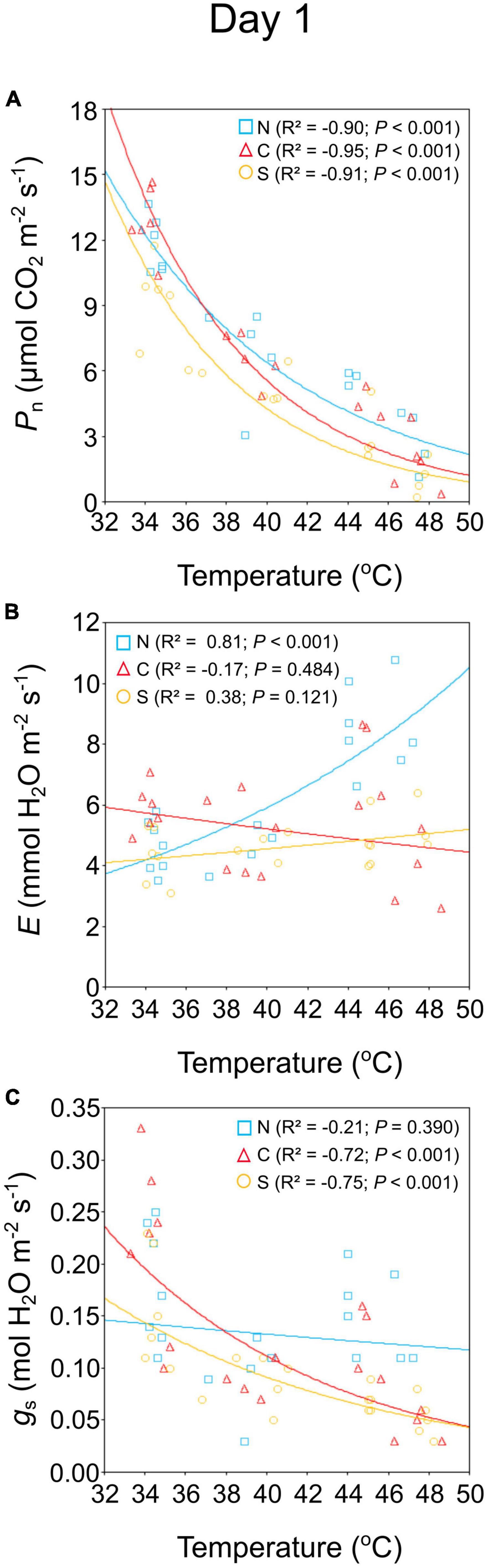
Figure 2. Effect of temperature on net photosynthetic rate (A), transpiration rate (B), and stomatal conductance (C) of 6-month-old Castanea sativa seedlings from the northern (N, blue squares), central (C, red triangles), and southern (S, yellow circles) populations. The variance explained (R2), and the significance (P) of the models are shown.
General response and recovery of C. sativa from heat stress
During the heat phase, the leaves of stressed seedlings showed brown margins, yellowing, and browning of inner areas, and scorching (Figure 1B). Leaf damage was observed only in about 10% of seedlings, but heat-stressed seedlings showed lower leaf, root, and total biomass compared to control plants (Table 2 and Supplementary Table 2). Heat stress did not lead to plant mortality. In the recovery phase, seedlings that had wilted more started to resprout (Figure 1C), although significant changes in seedling biomass were not detected (Supplementary Table 2).
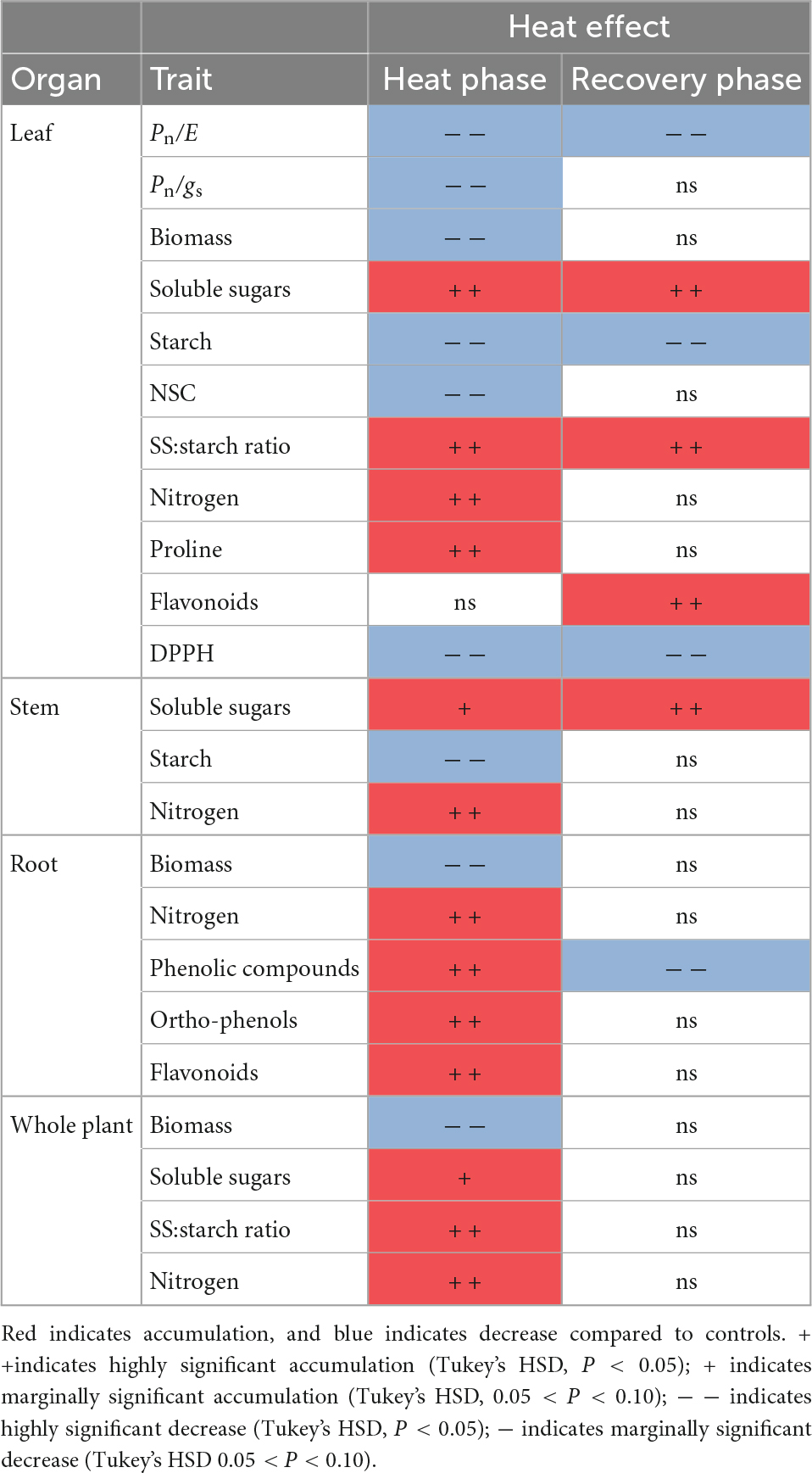
Table 2. Significant changes in leaves and roots of 6-month-old Castanea sativa seedlings subjected to 7 days of heat stress (n = 18; heat phase) and 10 days after application (n = 12; recovery phase).
Heat stress did not affect plant Pn, E, and gs but induced a significant decrease in instantaneous and intrinsic WUE compared to control seedlings (Table 2 and Supplementary Table 2). During the recovery phase, E and intrinsic WUE remained altered in heat stressed seedlings compared to control seedlings (Table 2 and Supplementary Table 2).
Major alterations observed during the heat phase in primary metabolism parameters occurred mainly in leaves (Table 2 and Supplementary Table 2). Heat-stressed seedlings responded by increasing soluble sugars and decreasing starch in leaves and stems (Table 2 and Supplementary Table 2). Soluble sugar:starch ratio was significantly increased by heat stress in leaves and the whole plant. Total NSC content in leaves decreased significantly with heat stress, while total NSC in stems and roots did not change significantly (Table 2 and Supplementary Table 2). In heat-stressed seedlings, N content significantly increased in all organs and at the whole plant level, and proline content in leaves doubled (Table 2 and Supplementary Table 2). Recovering seedlings continued to have higher soluble sugar concentration, lower starch concentration in leaves and stems and higher soluble sugar:starch ratios in leaves than control seedlings (Table 2 and Supplementary Table 2). In contrast, N and proline concentration returned to the levels of control seedlings (Supplementary Table 2).
Secondary metabolism of heat-stressed seedlings was most altered in the roots (Tables 2–4 and Supplementary Table 2). Chestnut seedlings subjected to heat stress significantly increased total phenolic compounds, ortho-phenolic, and flavonoid compounds only in roots (Table 2 and Supplementary Table 2). Given the heat-induced alterations in leaf and root phenolic compounds, non-targeted metabolite profiling was applied, revealing 18 compounds, 15 of which were identified (Table 3). Six identified compounds increased in heat-stressed plants (Table 4). Five of the six compounds showing variation were in the roots and belonged to the phenolic acid (hydroxybenzoic acid subclass) and flavonoid (flavonol subclass) families (Tables 3, 4). DFA revealed different secondary metabolism profiles between treatments in chestnut (Figure 3A). Isorhamnetin in leaves, and hydroxybenzoic acid, kaempferol 3-O-(6″-O-acetyl) glucoside-7-O-rhamnoside and hyperoside in roots, were involved in this discrimination (P < 0.001). However, under heat stress, antioxidant capacity increased significantly only in leaves (Table 2 and Supplementary Table 2). During the recovery phase, only flavonoids in leaves remained altered by heat stress (Table 2 and Supplementary Table 2). DFA of non-targeted metabolite profiling showed differences between recovery and control seedlings (Figure 3B). Astragalin and hydroxybenzoic acid decreased significantly in recovering seedlings compared to controls (Table 4 and Supplementary Table 4).
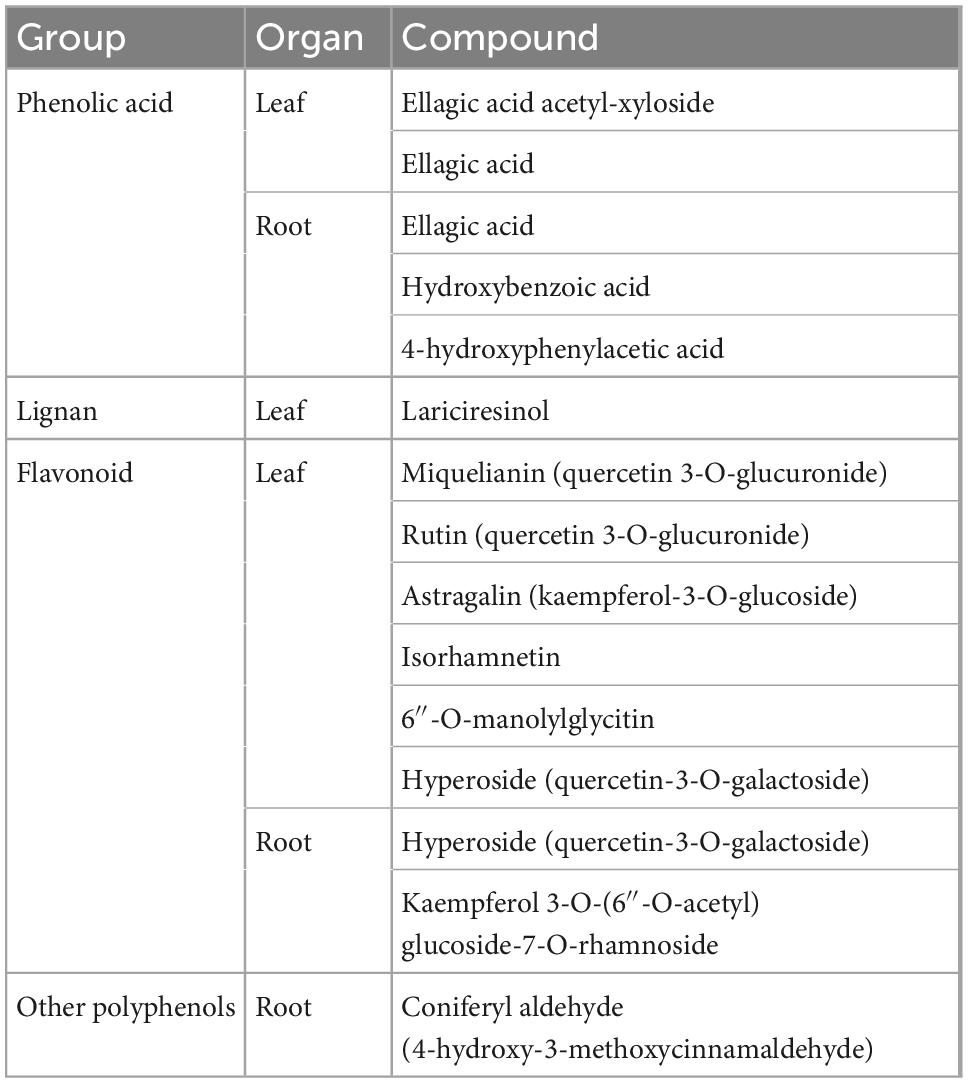
Table 3. List of secondary metabolite compounds detected, identified, and quantified in leaves and roots of 6-month-old Castanea sativa seedlings.
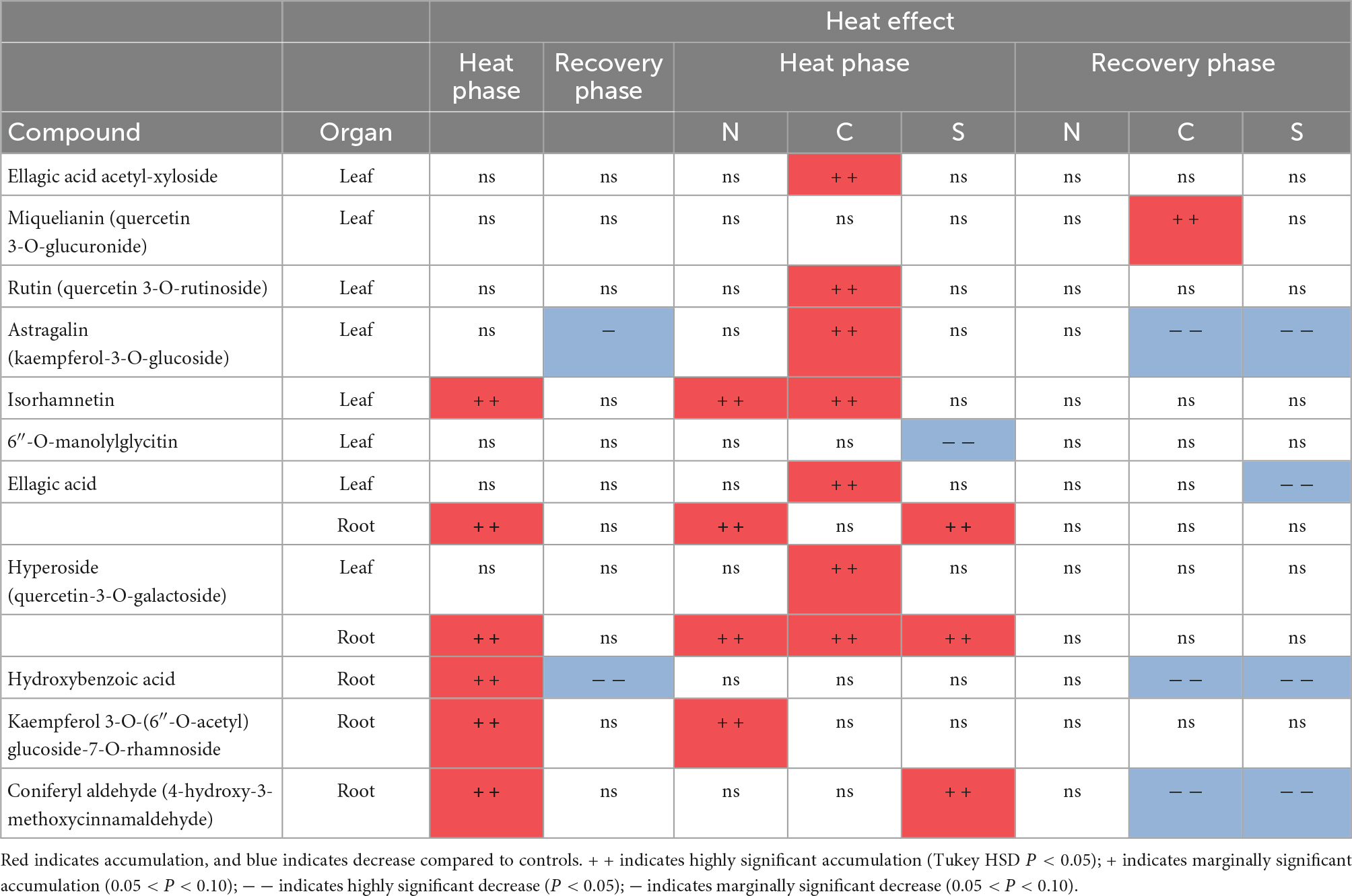
Table 4. Significant changes in leaves and roots of secondary metabolite-derived compounds of 6-month-old Castanea sativa seedlings from three populations (N-Northern; C-Central; S-Southern; n = 6 per population in heat phase; n = 4 per population in recovery phase) subjected to heat stress for 7 days (n = 18; heat phase) and 10 days after application (n = 12; recovery phase).
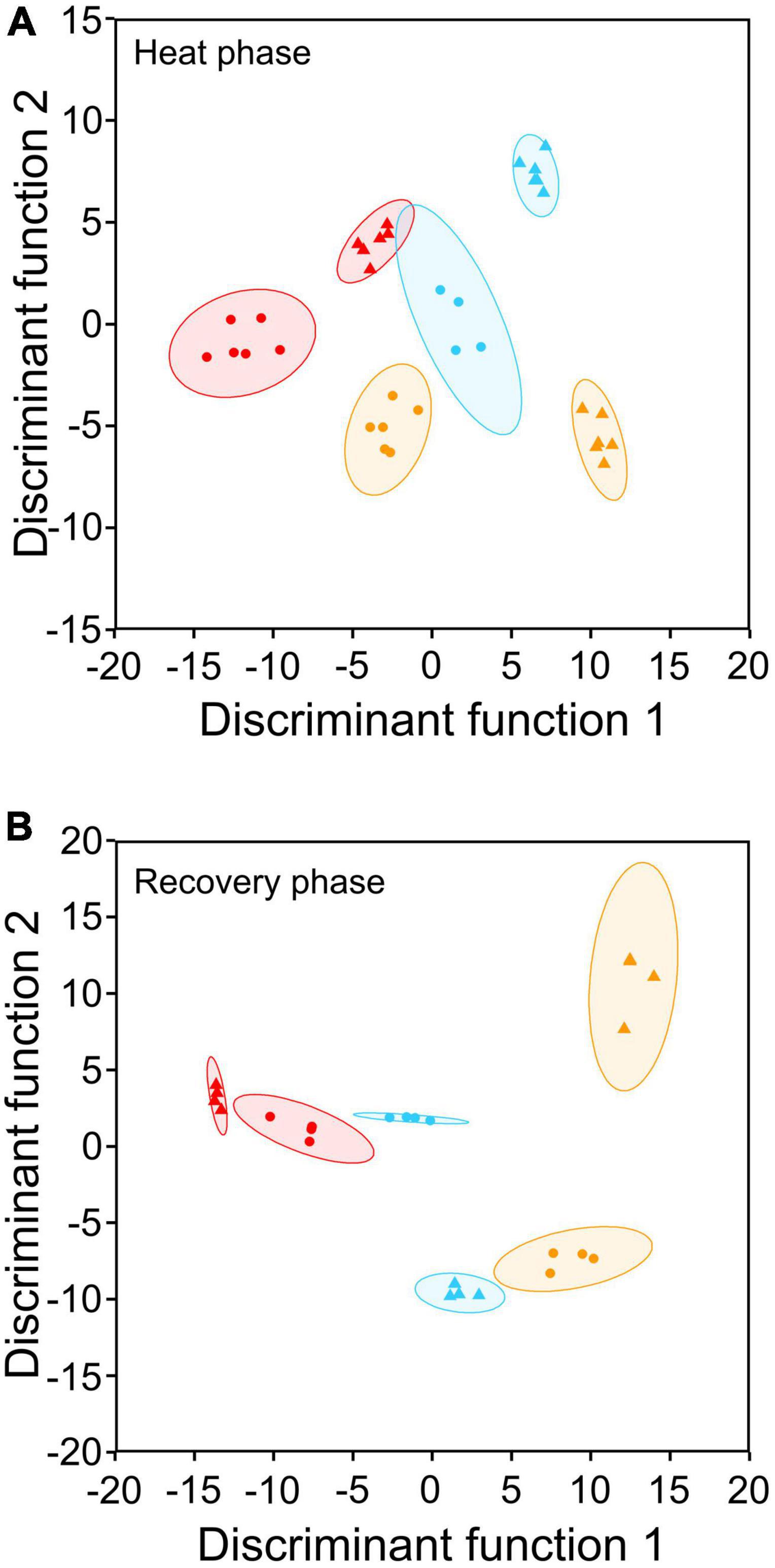
Figure 3. DFA of 6-month-old Castanea sativa seedlings showing differences between control (circles) and heat-stressed (triangles) seedlings from the northern (blue symbols), central (red symbols), and southern (yellow symbols) populations based on the 18 compounds detected in roots and leaves by non-targeted metabolite profiling. In panel (A), heat-stressed plants were at the time of maximum stress; in panel (B), heat-stressed plants were left for 10 days to recover (Supplementary Figure 1).
Principal component analysis revealed a clear difference in the physiological and biochemical status of C. sativa seedlings under control and heat treatments (Figure 4A). During the heat phase, the separation between control and heat was mainly associated with starch depletion in leaves (28.4%), high ratios of soluble sugars:starch in leaves (27.9%) and at the whole plant level (8.1%), proline accumulation in leaves and roots (2.6 and 12.1%, respectively), and accumulation of phenols (1.9%), ortho-phenols (2.8%), and flavonoids (3.1%) in roots (Figure 4A). After recovery, these differences mostly disappeared, and groups of plants clustered close to each other (Figure 4B). Correlation analysis between PCA variables revealed a biologically interesting correlation between proline content in leaves and soluble sugars in leaves and stems (Figure 5). This correlation changed from negative under control conditions to positive under heat stress conditions (Figure 5).
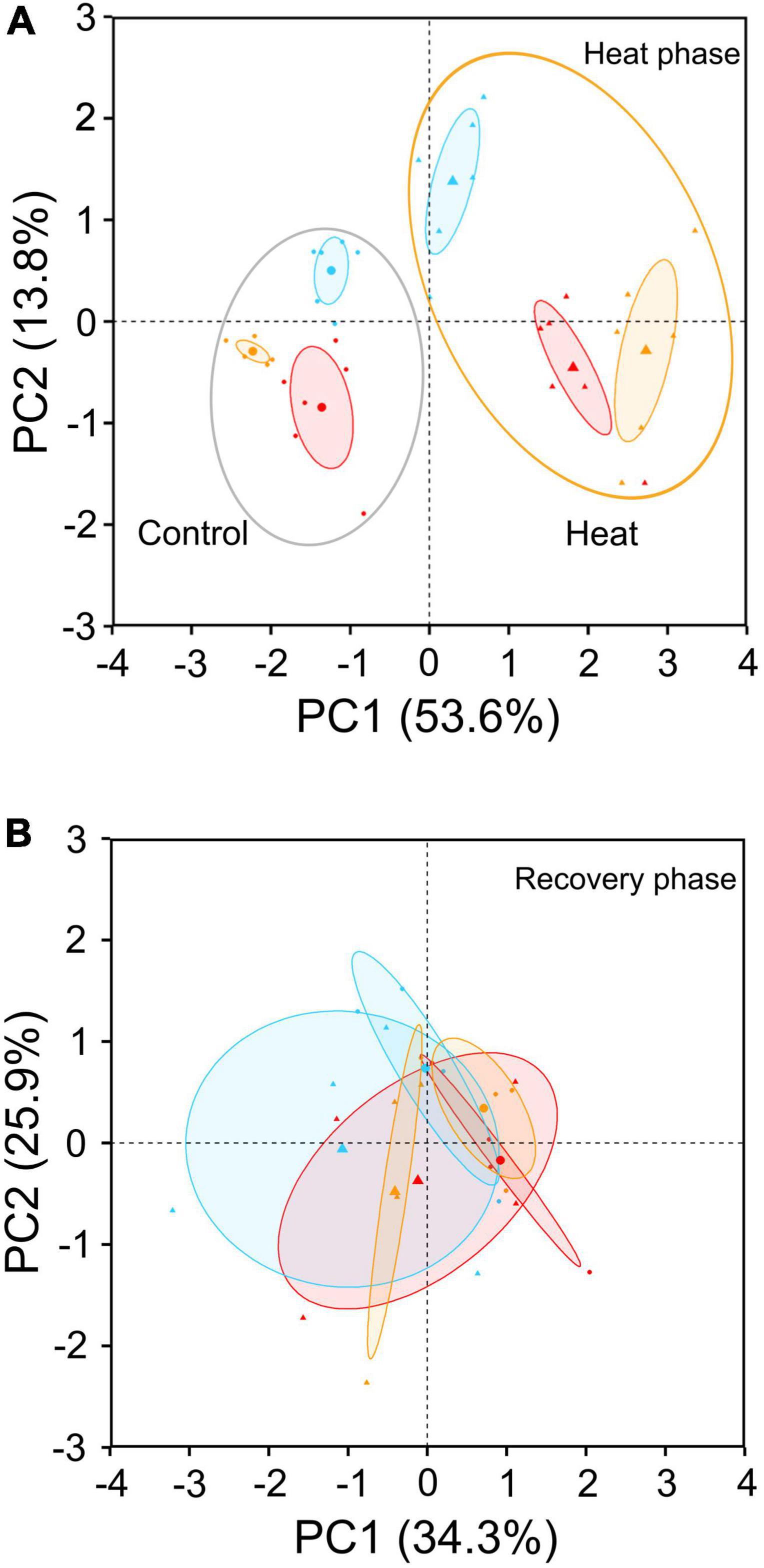
Figure 4. PCA of 6-month-old Castanea sativa seedlings showing differences between control (circles) and heat-stressed (triangles) seedlings at heat (A) and recovery (B) phases from northern (blue symbols), central (red symbols), and southern (yellow symbols) populations according to the physiological variables included in Figure 5. In panel (A), heat-stressed plants were at the time of maximum stress; in panel (B), heat-stressed plants were left for 10 days to recover (Supplementary Figure 1). In panel (A), the 10 variables contributing most to the PC1 and PC2 axes were leaf starch (28.4%), leaf soluble sugars:starch ratio (27.9%), root proline (12.1%), whole plant soluble sugars:starch ratio (8.1%), root flavonoids (3.1%), root ortho-phenols (2.8%), leaf proline (2.6%), root phenols (1.9%), instantaneous water use efficiency (1.5%), and root biomass (1.4%).
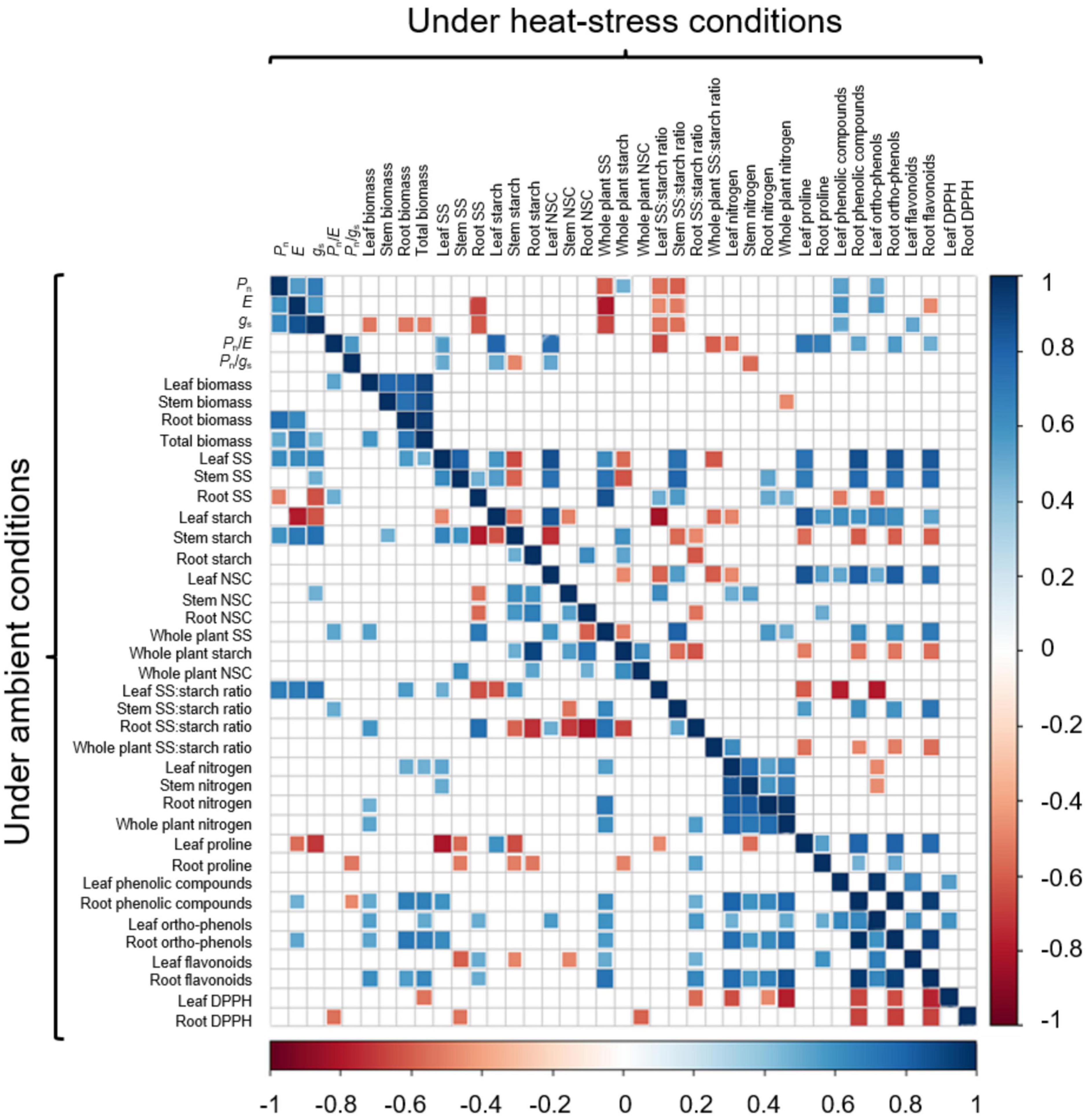
Figure 5. Matrix of significant (P < 0.05) Pearson correlation coefficients between gas exchange parameters, plant biomass, NSCs, nitrogen and proline content, total phenolic compounds, ortho-phenolic and flavonoid compounds and 2,2-diphenyl-1-picrylhydrazyl (DPPH) free radical scavenging of 6-month-old Castanea sativa seedlings subjected to ambient (below the main diagonal) and heat-stress conditions (above the main diagonal). Colored correlations are significant. Color gradient from blue to red indicates the gradient from positive to negative correlation (1,-1).
Heat stress response of C. sativa depends on climate origin
During the heat phase, no interpopulation differences were observed in external symptoms and foliar damage. However, seedlings from the northern population were the most adversely affected by heat stress in terms of biomass reduction in leaves, roots and at the whole plant level (Supplementary Table 3). Biomass of seedlings of southern origin was unaltered during and after heat stress (Supplementary Table 3). At recovery, no differences in biomass between heat-stressed and control seedlings were found for any population (Supplementary Table 3). Among leaf gas exchange parameters, E showed the highest interpopulation variability in response to heat stress (Supplementary Figure 2), increasing ca. 50 and 25% in the southern and central populations and decreasing ca. 25% in the northern population compared to controls (Supplementary Figure 2).
Interpopulation differences in primary metabolism were mostly observed in leaves and stems (Figure 6 and Supplementary Table 3). Leaf starch and soluble sugars:starch ratio were the parameters with the greatest interpopulation variability, each contributing approximately 45% to the separation between populations in the PCA (Supplementary Figure 3A). The southern population was the most reactive population to heat stress, reducing starch concentration in leaves by almost 100% and markedly increasing the soluble sugars:starch ratio relative to the control (Figure 6A and Supplementary Table 3). Variations in leaves for starch and the soluble sugars:starch ratio of seedlings from the northern population were low (Supplementary Table 3). In the stem, starch and NSC content decreased in the northern and central populations but increased in the southern population. Soluble sugar changes were similar in the northern and southern populations (Figure 6B).
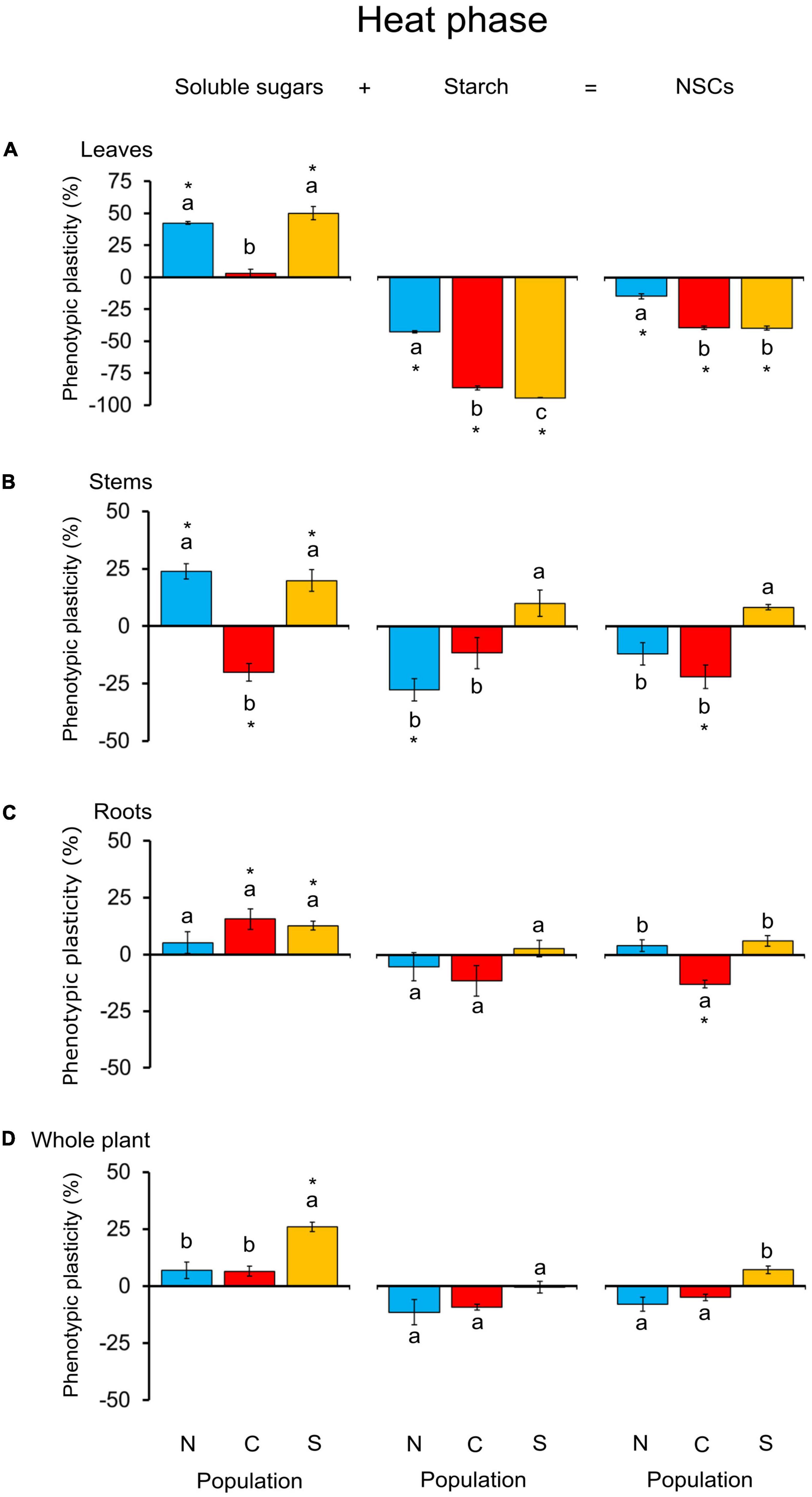
Figure 6. Phenotypic plasticity in soluble sugars, starch, and total NSCs in leaves (A), stems (B), roots (C), and whole plant (D) of 6-month-old Castanea sativa seedlings from the northern, central and southern populations in Spain (N, C, and S, respectively) after they were subjected to heat stress for 7 days. Heat treatment effects are shown in percentages relative to the values obtained for control plants. Vertical lines indicate standard errors of the mean (n = 6). Asterisks indicate significant differences from the control treatment (Tukey’s HSD test, P < 0.05). Different letters indicate different significant effects between populations within each variable (Tukey’s HSD test, P < 0.05).
Central and southern heat-stressed seedlings significantly increased their N content in leaves and stems compared to control seedlings (Supplementary Table 3). Heat-stressed seedlings from the northern population had a fourfold increase in leaf proline content, while no change was observed in the proline content of seedlings from the central and southern populations (Supplementary Table 3). After recovery, only southern seedlings had lower total starch and NSC content in leaves compared to the control (Supplementary Table 3). Both N and proline in recovering seedlings returned to control levels, regardless of the population (Supplementary Table 3).
Regarding secondary metabolism compounds, the populations most adapted to heat stress (the meso-Mediterranean central and southern populations) showed the highest variations in total phenylpropanoids and antioxidant capacity in leaves and roots after heat treatment (Figure 7). Non-targeted metabolite profiling in response to heat showed different changes in the contents of the compounds identified depending on the population of origin (Figure 4A and Table 4). Heat-stressed seedlings from the central population showed the greatest alteration of secondary metabolism, with six compounds (hyperoside, rutin, isorhamnetin, astragalin, ellagic acid, and ellagic acid acetyl-xyloside) accumulating in leaves (Supplementary Table 5). Seedlings from the southern and northern populations showed an altered metabolomic profile in roots (Supplementary Table 4). DFA of the recovery phase showed different metabolite profiles depending on the seedling origin and pretreatment (Figure 3B). PCA analysis also showed a differential interpopulation response during the heat phase (Figure 4A). The greatest response to heat stress by PCA analysis was in seedlings from the southern population, associated with higher starch depletion and a higher soluble sugars:starch ratio in leaves and at the whole plant level, and higher accumulation of proline, total phenolic compounds and ortho-phenolic and flavonoid compounds in roots (Figure 4A). After recovery, only seedlings from the heat-stressed southern population were separated from their respective controls, mainly due to starch depletion in leaves (Figure 4B).
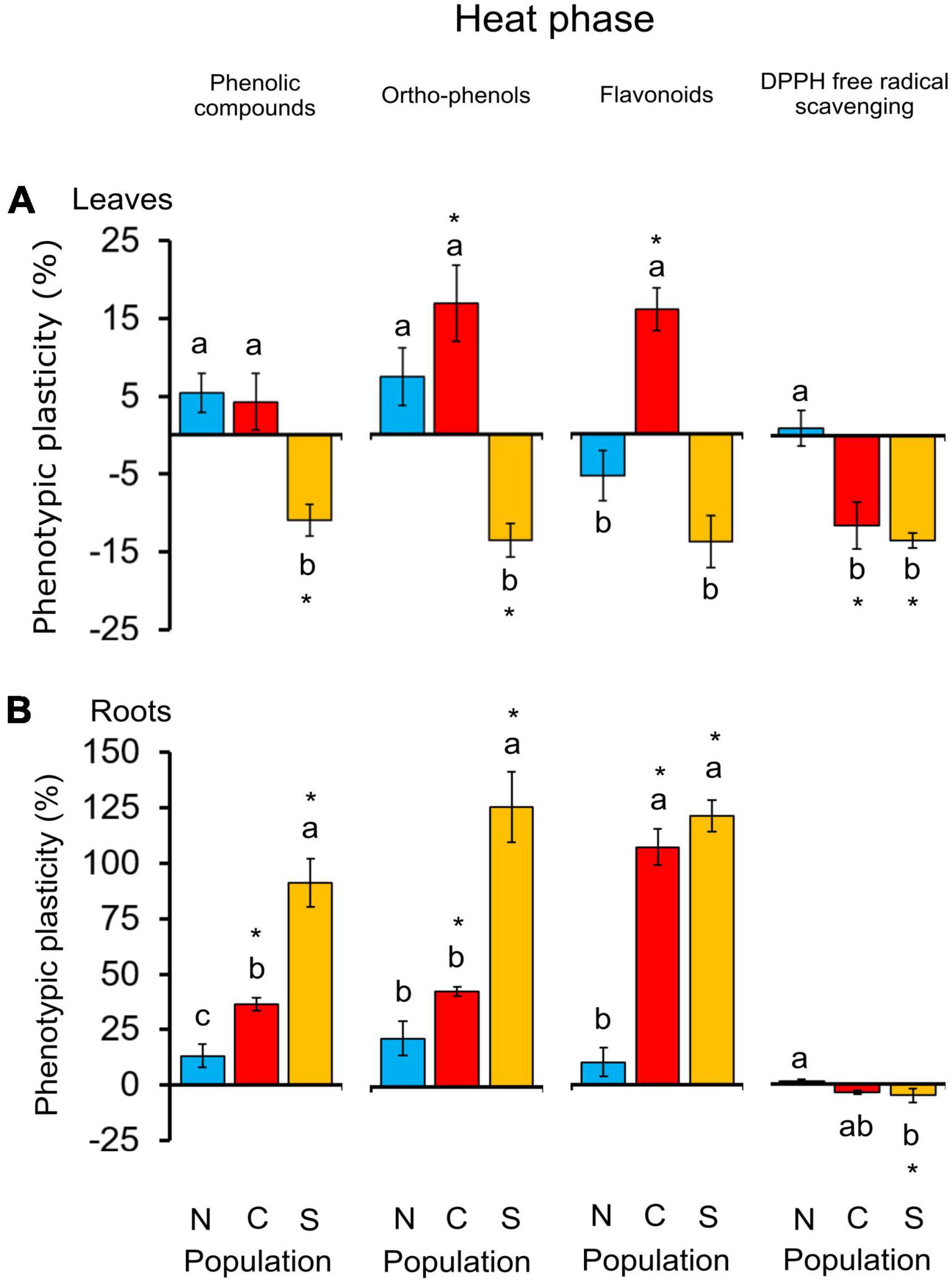
Figure 7. Phenotypic plasticity in total phenolic compounds and ortho-phenolic and flavonoid compounds, and 2,2-diphenyl-1-picrylhydrazyl (DPPH) free radical scavenging in leaves (A) and roots (B) of 6-month-old Castanea sativa seedlings from the northern, central, and southern populations in Spain (N, C, and S, respectively) after they were subjected to heat stress for 7 days. Heat treatment effects are shown in percentages relative to the values obtained for control plants. Vertical lines indicate standard errors of the mean (n = 6). Asterisks indicate significant differences from the control treatment (Tukey’s HSD test, P < 0.05). Different letters indicate different significant effects between populations within each variable (Tukey’s HSD test, P < 0.05).
Genetic variation in phenotypic plasticity of C. sativa in response to heat stress was observed for ten physiological and biochemical parameters (Figure 8). The southern population was not responsive in terms of biomass in comparison to the northern and central populations. However, the southern population was the most responsive in terms of soluble sugars: starch ratios and N content (Figure 8). NSC always declined for the central population, but behaved differently for the other populations, being the parameter showing the highest genetic variation in phenotypic plasticity (Figure 8).
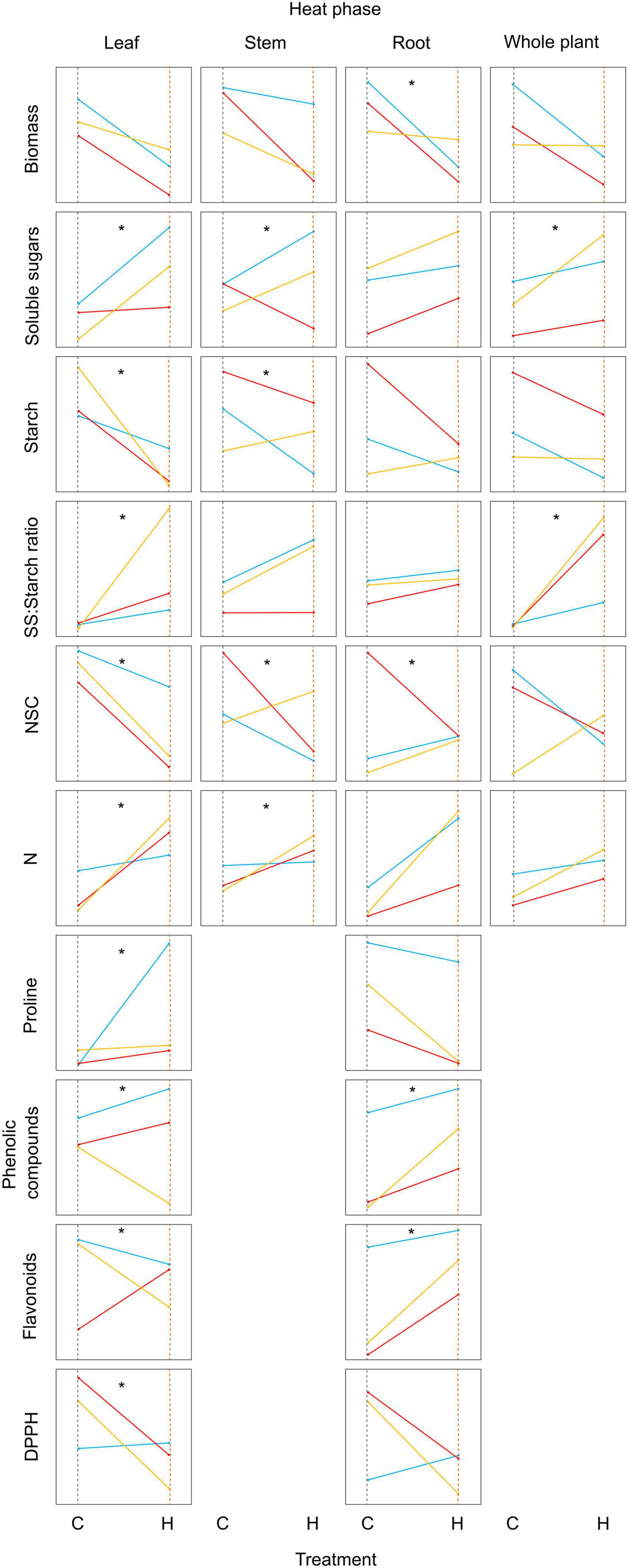
Figure 8. Phenotypic values of ten physiological and biochemical parameters obtained from the leaf, stem, fine root, and whole plant of Castanea sativa seedlings from the northern (blue line), central (red line), and southern (yellow line) populations in Spain after seedlings were subjected to ambient temperature (C) and heat stress (H) treatments for 7 days. Asterisks indicate significant population × treatment interactions, and provide evidence for genetic variation in phenotypic plasticity of parameters in response to heat stress.
Discussion
For the first time in chestnut, the impact of heat stress and recovery effects on the physiology and biochemistry of seedlings was assessed. Phenotypic plastic responses in plant material from different climatic regions were quantified, and genetic variation in phenotypic plasticity in response to heat stress was observed.
Heat stress impact on C. sativa
Observations reported here in C. sativa seedlings subjected to 42.5°C for 7 days confirm that heat stress caused leaf and branch scorch, leaf senescence and abscission, and inhibition of shoot and root growth (Wahid et al., 2007). However, differentiation across populations in genetic variation of biomass was minimal. Leaf gas exchange parameters were different on the first and seventh days after heat stress. During the first episode of temperature increase, seedlings decreased net photosynthetic ratio, but after a heat wave (7 days of heat stress), this parameter did not alter, agreeing with results obtained by Ameye et al. (2012) in Quercus rubra. This suggests that in chestnut seedlings Pn is more sensitive to daily temperatures than to prolonged heat exposure and could also indicate plant adaptation to elevated temperatures.
Heat stress induced considerable changes in compounds derived from primary and secondary metabolisms. NSC was especially plastic above ground, and at the whole plant level, NSC decreased in response to heat stress except in the southern population. The NSC reserve pool in trees constitutes the primary resource supply for energetic disparities and generally draws down when trees experience abiotic stress (Galiano et al., 2011; Wiley and Helliker, 2012; Hartmann et al., 2013; Dietze et al., 2014; O’Brien et al., 2014). The high genetic variation in phenotypic plasticity of NSC (Figure 8) is in accordance with a recent study performed in Populus trichocarpa trees (Blumstein and Hopkins, 2021). This genetic variation in heat response could provide a key mechanism through which populations can evolve a more adaptive response to future global warming.
Non-structural carbohydrates act as protective molecules against heat stress (Wani and Kumar, 2020). Soluble sugars stabilise proteins and cell membranes, maintain cell turgor, participate in stress signalling pathways, and scavenge excess ROS (Bhattacharya and Kundu, 2020), and starch degrades to aid plant fitness and survival under harsh growing conditions (Thalmann and Santelia, 2017), e.g., a rapid degradation of starch generates organic acids and sugars that maintain stomatal pore guard cell turgor and promote stomatal opening (Horrer et al., 2016). In response to heat stress, glucose, and fructose increase has been reported in conifer needles (Marias et al., 2017). Rapid starch degradation and soluble sugars accumulation has been reported in Populus tremula leaves at elevated temperatures as a strategy of trees to protect respiratory membranes and buffer consumption in respiratory processes (Hüve et al., 2012). In a drought-tolerant Eucalyptus globulus clone subjected to heat stress, a decrease in leaf starch content and an increase in soluble sugars (mannitol, sorbitol, inositol, and several amino acids) were observed (Correia et al., 2018). In our heat-stressed chestnuts, plastic variation in the amount of NSCs also had a genetic component.
A positive phenotypic correlation was observed between soluble sugar content and proline content in leaves. Proline protects the structure of enzymes and proteins by maintaining membrane integrity and eliminating ROS (Hayat et al., 2012). Proline and soluble sugar signalling pathways interact synergistically, forming part of the plant antioxidant system under stress situations (Moustakas et al., 2011). The increase in soluble sugars, N, proline and antioxidant capacity observed here in leaves, together with the absence of changes in phenolic compounds in leaves, suggests chestnut trees use carbohydrates as antioxidant compounds. In addition, the decrease in starch, increase in soluble sugars, and unaltered stomatal conductance between treatments suggest chestnut trees use sugars to keep stomata open despite heat stress.
In relation to the secondary metabolism, an accumulation of total phenolic compounds and ortho-phenolic and flavonoid compounds was observed below ground in chestnut seedlings. We identified and quantified 15 phenolic compounds, some of which (ellagic acid, 4-hydroxyphenylacetic acid, hydroxybenzoic acid, quercetin 3-O-galactoside, kaempferol 3-O-(6″acetyl) glucoside 7-O-rhamnoside, tyrosol, and 4-hydroxy-3-methoxycinnamaldehyde) are reported here for the first time in chestnut roots. Moreover, hydroxybenzoic acid, kaempferol 3-O-(6″-O-acetyl) glucoside-7-O-rhamnoside and hyperoside in roots were significantly involved in discriminating between heat-stressed and non-stressed seedlings. Accumulation of phenolic compounds in roots has been associated with plant root biomass loss (Rhodes et al., 1987), as occurred here. Derivatives of two compounds involved in the DFA separation between treatments [kaempferol 3-O-(6″-O-acetyl) glucoside-7-O-rhamnoside and hyperoside] accumulated in roots and were associated with inhibition of polar auxin transport (PAT) in roots (Yin et al., 2014). Phenolic accumulation, especially of compounds with antioxidant functions, has been reported as frequent when applying abiotic stress and may occur as an adaptive mechanism (Rivero et al., 2001; Zandalinas et al., 2017; Bhattacharya and Kundu, 2020; Carneiro-Carvalho et al., 2021).
Regarding the recovery of chestnut seedlings from heat stress, most parameters returned to initial levels, agreeing with observations in other forest species (Escandón et al., 2016; Correia et al., 2018). However, leaf soluble sugar, starch and flavonoid content, and leaf antioxidant capacity remained altered. The durable accumulation of soluble sugars, flavonoids, and antioxidant capacity concur with the role of these compounds in tolerance, adaptation, and recovery from abiotic stress (Singh et al., 2017; Bhattacharya and Kundu, 2020). Previous studies in chestnut reported durable accumulation of carbohydrates in response to water stress (Camisón et al., 2020).
Interpopulation variability of C. sativa in response to heat stress
Physiological and biochemical heat stress responses of trees can vary depending on the climate of origin, and different populations can show varied responses, from high tolerance in warm climates to low tolerance in cold climates (Marias et al., 2017). Knowledge about phenotypic plastic responses and their genetic control is necessary to forecast the full potential of a species to adapt and/or evolve to changing conditions (Zas et al., 2020; Blumstein and Hopkins, 2021). Likewise, because of the increasing impact of global warming on plants, there is increasing interest in quantifying phenotypic plasticity and local adaptation (i.e., adaptive genetic changes) to heat and drought stress (Castellana et al., 2021). We found interpopulation differences in chestnut seedlings in morphological, physiological, and biochemical parameters in response to heat stress. Seedlings whose mother trees originated from less exposed heat conditions in their area of origin, i.e., from the northern population, were the most adversely affected by heat in terms of biomass reduction. During the first hours of heat, plants from the supra-Mediterranean (northern) population increased their transpiration rates exponentially compared to seedlings from the meso-Mediterranean (central and southern) populations. We hypothesise that seedlings from the northern population use immediate transpiration as a strategy to cool leaves and avoid heat stress consequences, as observed in Q. rubra (Ameye et al., 2012) and Acer rubrum (Weston and Bauerle, 2007). In the northern population, the decrease in Pn while gs remained unchanged suggests an alteration of the photosynthetic capacity of the mesophyll (Camejo et al., 2005). Decreased Pn due to non-stomatic limitations has been observed in plants not adapted to heat (Camejo et al., 2005). However, during the heat phase, no interpopulation differences were observed in gas exchange parameters. This could suggest that regardless of the population, chestnut is able to adapt its photosynthetic apparatus to sustained heat stress.
Different interpopulation responses to heat stress were detected in the primary metabolism of seedlings. Seedlings from the southern population were the most responsive, followed by those from the central population and, finally, by those from the northern population. Seedlings from the southern population were characterised by almost total starch depletion and an increase in soluble sugars and the soluble sugars:starch ratio and, as discussed above, starch mobilisation and accumulation of soluble sugars are associated with tolerance to heat stress (Xiong et al., 2015). Proline accumulation in leaves of heat-stressed plants occurred only in seedlings from the northern population, and proline was the main variable explaining the separation of populations in the PCA of heat-stressed seedlings (Figure 3A). Low proline accumulation in chestnut has been associated with tolerance to heat stress in plants treated with SiK (Carneiro-Carvalho et al., 2021), and heat-induced proline accumulation in Arabidopsis plants has been considered detrimental and responsible for reduced thermotolerance (Lv et al., 2011). Thus, the accumulation of this osmolyte in C. sativa seedlings from the northern population could indicate reduced thermotolerance.
Regarding secondary metabolism, the southern and northern populations also showed the highest and lowest response to heat stress, respectively. The response of seedlings from the southern population was a high accumulation of total phenolic compounds and ortho-phenolic and flavonoid compounds in roots. However, analysis of non-targeted metabolite profiling showed that seedlings from the central population, exposed to maximum temperatures of almost 40°C in the area of origin, experienced the most pronounced changes, comprising an increase in ellagic acid, ellagic acid acetyl-xyloside, derivatives of quercetin, kaempferol-3-O-glucoside and isorhamnetin in leaves. Targeted and non-targeted approaches provided complementary information and should be used together, when possible, to better characterise tree response to heat stress.
Ten days after the heat stress treatment ceased, seedlings from the three populations reached levels of control seedlings in practically all physiological and biochemical parameters. Only those from the southern population remained separated from the control in the PCA analysis due to starch depletion and mobilisation to soluble sugars. In Spain, differences in the pluviometry of chestnut habitats have resulted in differentiation of two C. sativa ecotypes with different adaptive responses to drought stress (Míguez-Soto and Fernández-López, 2015; Alcaide et al., 2019; Míguez-Soto et al., 2019). These ecotypes were also differentiated by EST-SSRs associated with heat stress using the same populations of the present study (Dorado et al., 2022). Based on the results obtained here, differences in the thermal regime of chestnut habitats may also have resulted in different ecotypes associated with heat stress tolerance. It should be acknowledged that our results were obtained in seedlings. Since tolerance to environmental stress may change throughout the lifetime of trees (Solla et al., 2005; Niinemets, 2010), variation of phenotypic plasticity and acclimatisation to heat stress may also change depending on the age of trees and forests.
Conclusion
Heat stress significantly impacted the primary and secondary metabolism of C. sativa seedlings. Changes in the primary metabolism were observed mainly above ground and included depletion of starch and an increase in soluble sugars, N, and proline. Changes in the secondary metabolism were observed below ground and included an increase in total phenolic, ortho-phenolic, and flavonoid content. For the first time in chestnut, metabolite profiles in response to heat stress have been analysed, and several compounds, including isorhamnetin in leaves, and hydroxybenzoic acid, kaempferol 3-O-(6″-O-acetyl) glucoside-7-O-rhamnoside in roots, have been identified as relevant for distinguishing between heat-stressed and non-stressed plants. Ten days after the heat stress treatment ceased, the physiology and biochemistry of C. sativa recovered, indicating the high heat stress tolerance of European chestnut. This work reveals different patterns of heat stress tolerance depending on the climate conditions in the region of origin of mother trees. The division of C. sativa into two ecotypes, previously demonstrated for water stress tolerance, is reinforced here by differences in the physiology and biochemistry of populations in response to heat stress. Phenotypic plasticity in response to heat stress was higher in the meso-Mediterranean than in the supra-Mediterranean populations. Genetic variation in plasticity in several physiological and biochemical parameters in response to heat stress was also observed. If proven to be adaptive, such variation would be an opportunity for C. sativa to respond to global warming-mediated selection.
Data availability statement
The original contributions presented in this study are included in the article/Supplementary material, further inquiries can be directed to the corresponding author.
Author contributions
FJD and AS conceived and planned the experiment and took the lead in writing the manuscript. FJD carried out the experiment. FJD and ÁC carried out the sampling. GP provided the necessary methods for the determination of proline and total phenolic compounds. PM and SR assisted in sample processing and analysis. NC and JA performed the determination and quantification of non-targeted metabolite profiles. All authors provided critical feedback and helped shape the research, analysis, and manuscript.
Funding
This work was funded by grant VI-Plan Regional I + D + i (IB18091 from the General Secretariat of Science, Technology and Innovation, Extremadura Regional Ministry of Economy and Infrastructure, Government of Extremadura) and by the European Union’s Regional Development Fund. FJD was funded by the Spanish Ministry of Education through FPU16/03188 grant and the complementary mobility grants for beneficiaries of the FPU program. ÁC was supported by a “Margarita Salas para la formación de jóvenes doctores” grant from Universidad de Extremadura (Spain).
Acknowledgments
We are very grateful to Cristina Serrano and Vicente Dorado for their help in seed collection, José Manuel Cañamares for technical help, and Jane McGrath for English editing. We also thank the two reviewers for their very helpful comments and Ned Fetcher for all his effort and for improving the readability of the text.
Conflict of interest
The authors declare that the research was conducted in the absence of any commercial or financial relationships that could be construed as a potential conflict of interest.
Publisher’s note
All claims expressed in this article are solely those of the authors and do not necessarily represent those of their affiliated organizations, or those of the publisher, the editors and the reviewers. Any product that may be evaluated in this article, or claim that may be made by its manufacturer, is not guaranteed or endorsed by the publisher.
Supplementary material
The Supplementary Material for this article can be found online at: https://www.frontiersin.org/articles/10.3389/ffgc.2022.1072661/full#supplementary-material
References
Ahmad, P., Umar, S., and Sharma, S. (2010). “Mechanism of free radical scavenging and role of phytohormones in plants under abiotic stresses,” in Plant adaptation and phytoremediation, eds M. Ashraf, M. Ozturk, and M. S. A. Ahmad (Berlin: Springer), 99–118. doi: 10.1007/978-90-481-9370-7
Aitken, S. N., and Whitlock, M. C. (2013). Assisted gene flow to facilitate local adaptation to climate change. Annu. Rev. Ecol. Evol. Syst. 44, 367–388. doi: 10.1146/annurev-ecolsys-110512-135747
Aitken, S. N., Yeaman, S., Holliday, J. A., Wang, T., and Curtis-mclane, S. (2008). Adaptation, migration or extirpation: Climate change outcomes for tree populations. Evol. Appl. 1, 95–111. doi: 10.1111/j.1752-4571.2007.00013.x
Akhi, M. Z., Haque, M. M., and Biswas, M. S. (2021). “Role of secondary metabolites to attenuate stress damages in plants,” in Antioxidants - benefits, sources, mechanisms of action, ed. V. Waisundara (Rijeka: IntechOpen), 646. doi: 10.5772/intechopen.92918
Alberto, F. J., Aitken, S. N., Alía, R., González-Martínez, S. C., Hänninen, H., Kremer, A., et al. (2013). Potential for evolutionary responses to climate change – evidence from tree populations. Glob. Chang. Biol. 19, 1645–1661. doi: 10.1111/gcb.12181
Alcaide, F., Solla, A., Mattioni, C., Castellana, S., and Martín, M. A. (2019). Adaptive diversity and drought tolerance in Castanea sativa assessed through EST-SSR genic markers. Forestry 92, 287–296. doi: 10.1093/forestry/cpz007
Ameye, M., Wertin, T. M., Bauweraerts, I., McGuire, M. A., Teskey, R. O., and Steppe, K. (2012). The effect of induced heat waves on Pinus taeda and Quercus rubra seedlings in ambient and elevated CO2 atmospheres. New Phytol. 196, 448–461. doi: 10.1111/j.1469-8137.2012.04267.x
Bates, L. S., Waldren, R. P., and Teare, I. D. (1973). Rapid determination of free proline for water-stress studies. Plant Soil 39, 205–207.
Berini, J. L., Brockman, S. A., Hegeman, A. D., Reich, P. B., Muthukrishnan, R., Montgomery, R. A., et al. (2018). Combinations of abiotic factors differentially alter production of plant secondary metabolites in five woody plant species in the boreal-temperate transition zone. Front. Plant Sci. 9:1257. doi: 10.3389/fpls.2018.01257
Bhagat, K. P., Kumar, R. A., Ratnakumar, P., Kumar, S., Bal, S. K., and Agrawal, P. K. (2014). “Photosynthesis and associated aspects under abiotic stresses environment,” in Approaches to plant stress and their management, eds R. K. Gaur and P. Sharma (New Delhi: Springer), 191–205. doi: 10.1007/978-81-322-1620-9
Bhattacharya, S., and Kundu, A. (2020). “Sugars and sugar polyols in overcoming environmental stresses,” in Protective chemical agents in the amelioration of plant abiotic stress: Biochemical and molecular perspectives, eds A. Roychoudhury and D. K. Tripathi (New York: John Wiley & Sons), 71–101. doi: 10.1002/9781119552154.ch4
Birami, B., Gattmann, M., Heyer, A. G., Grote, R., Arneth, A., and Ruehr, N. K. (2018). Heat waves alter carbon allocation and increase mortality of aleppo pine under dry conditions. Front. For. Glob. Change 1:8. doi: 10.3389/ffgc.2018.00008
Blumstein, M., and Hopkins, R. (2021). Adaptive variation and plasticity in non-structural carbohydrate storage in a temperate tree species. Plant Cell Environ. 44, 2494–2505. doi: 10.1111/pce.13959
Camejo, D., Rodríguez, P., Morales, M. A., Dell’Amico, J. M., Torrecillas, A., and Alarcón, J. J. (2005). High temperature effects on photosynthetic activity of two tomato cultivars with different heat susceptibility. J. Plant Physiol. 162, 281–289. doi: 10.1016/j.jplph.2004.07.014
Camisón, Á, Martín, M. Á, Flors, V., Sánchez-Bel, P., Pinto, G., Vivas, M., et al. (2021). Exploring the use of scions and rootstocks from xeric areas to improve drought tolerance in Castanea sativa Miller. Environ. Exp. Bot. 187, 1–10. doi: 10.1016/j.envexpbot.2021.104467
Camisón, A., Martín, A. M., Dorado, F. J., Moreno, G., and Solla, A. (2020). Changes in carbohydrates induced by drought and waterlogging in Castanea sativa. Trees 34, 579–591. doi: 10.1007/s00468-019-01939-x
Carneiro-Carvalho, A., Anjos, R., Pinto, T., and Gomes-Laranjo, J. (2021). Stress oxidative evaluation on SiK® -supplemented Castanea sativa Mill. plants growing under high temperature. J. Soil Sci. Plant Nutr. 21, 415–425. doi: 10.1007/s42729-020-00370-3
Castellana, S., Martin, M. Á, Solla, A., Alcaide, F., Villani, F., Cherubini, M., et al. (2021). Signatures of local adaptation to climate in natural populations of sweet chestnut (Castanea sativa Mill.) from southern Europe. Ann. For. Sci. 78:27. doi: 10.1007/s13595-021-01027-6
Chang, C. C., Yang, M.-H., Wen, H.-M., and Chern, J. C. (2002). Estimation of total flavonoid content in propolis by two complementary colometric methods. J. Food Drug Anal. 10, 178–182. doi: 10.38212/2224-6614.2748
Conedera, M., Krebs, P., Tinner, W., Pradella, M., and Torriani, D. (2004). The cultivation of Castanea sativa (Mill.) in Europe, from its origin to its diffusion on a continental scale. Veg. Hist. Archaeobot. 13, 161–179. doi: 10.1007/s00334-004-0038-7
Correia, B., Hancock, R. D., Amaral, J., Gomez-Cadenas, A., Valledor, L., and Pinto, G. (2018). Combined drought and heat activates protective responses in Eucalyptus globulus that are not activated when subjected to drought or heat stress alone. Front. Plant Sci. 9:819. doi: 10.3389/fpls.2018.00819
Della-Marta, P. M., Haylock, M. R., Luterbacher, J., and Wanner, H. (2007). Doubled length of western European summer heat waves since 1880. J. Geophys. Res. Atmos. 112, 1–11. doi: 10.1029/2007JD008510
Díaz, R., Johnsen, Ø, and Fernández-López, J. (2009). Variation in spring and autumn freezing resistance among and within Spanish wild populations of Castanea sativa. Ann. For. Sci. 66, 708–720. doi: 10.1051/forest/2009059
Dietze, M. C., Sala, A., Carbone, M. S., Czimczik, C. I., Mantooth, J. A., Richardson, A. D., et al. (2014). Nonstructural carbon in woody plants. Annu. Rev. Plant Biol. 65, 667–687. doi: 10.1146/annurev-arplant-050213-040054
Dorado, F. J., Solla, A., Alcaide, F., and Martín, M. Á (2022). Assessing heat stress tolerance in Castanea sativa. Forestry 95, 667–677. doi: 10.1093/forestry/cpac021
Escandón, M., Cañal, M. J., Pascual, J., Pinto, G., Correia, B., Amaral, J., et al. (2016). Integrated physiological and hormonal profile of heat-induced thermotolerance in Pinus radiata. Tree Physiol. 36, 63–77. doi: 10.1093/treephys/tpv127
Fernández-López, J., and Alía, R. (2003). EUFORGEN Technical guidelines for genetic conservation and use for chestnut (Castanea sativa). Rome: International Plant Genetic Resources Institute.
Fernández-López, J., Fernández-Cruz, J., and Míguez-Soto, B. (2021). The demographic history of Castanea sativa Mill. in southwest Europe: A natural population structure modified by translocations. Mol. Ecol. 30, 3930–3947. doi: 10.1111/mec.16013
Galiano, L., Martínez-Vilalta, J., and Lloret, F. (2011). Carbon reserves and canopy defoliation determine the recovery of Scots pine 4 yr after a drought episode. New Phytol. 190, 750–759. doi: 10.1111/j.1469-8137.2010.03628.x
Ghalambor, C. K., Mckay, J. K., Carroll, S. P., and Reznick, D. N. (2007). Adaptive versus non-adaptive phenotypic plasticity and the potential for contemporary adaptation in new environments. Funct. Ecol. 21, 394–407. doi: 10.1111/j.1365-2435.2007.01283.x
Gomes-Laranjo, J., Peixoto, F., Wong Fong Sang, H. W., and Torres-Pereira, J. (2006). Study of the temperature effect in three chestnut (Castanea sativa Mill.) cultivars behaviour. J. Plant Physiol. 163, 945–955. doi: 10.1016/j.jplph.2005.06.020
Hartmann, H., Ziegler, W., and Trumbore, S. (2013). Lethal drought leads to reduction in nonstructural carbohydrates in Norway spruce tree roots but not in the canopy. Funct. Ecol. 27, 413–427. doi: 10.1111/1365-2435.12046
Hasanuzzaman, M., Nahar, K., Alam, M. M., Roychowdhury, R., and Fujita, M. (2013). Physiological, biochemical, and molecular mechanisms of heat stress tolerance in plants. Int. J. Mol. Sci. 14, 9643–9684. doi: 10.3390/ijms14059643
Hayat, S., Hayat, Q., Alyemeni, M. N., Wani, A. S., Pichtel, J., and Ahmad, A. (2012). Role of proline under changing environments: A review. Plant Signal. Behav. 7, 1–11. doi: 10.4161/psb.21949
He, Y., Zhang, X., Shi, Y., Xu, X., Li, L., and Wu, J. L. (2021). PREMATURE SENESCENCE LEAF 50 promotes heat stress tolerance in rice (Oryza sativa L.). Rice 14:53. doi: 10.1186/s12284-021-00506-8
Hoch, G., Popp, M., and Körner, C. (2002). Altitudinal increase of mobile carbon pools in Pinus cembra suggests sink limitation of growth at the Swiss treeline. Oikos 98, 361–374. doi: 10.1034/j.1600-0706.2002.980301.x
Horrer, D., Flütsch, S., Pazmino, D., Matthews, J. S. A., Thalmann, M., Nigro, A., et al. (2016). Blue light induces a distinct starch degradation pathway in guard cells for stomatal opening. Curr. Biol. 26, 362–370. doi: 10.1016/j.cub.2015.12.036
Hüve, K., Bichele, I., Ivanova, H., Keerberg, O., Pärnik, T., Rasulov, B., et al. (2012). Temperature responses of dark respiration in relation to leaf sugar concentration. Physiol. Plant 144, 320–334. doi: 10.1111/j.1399-3054.2011.01562.x
Li, W., Hartmann, H., Adams, H. D., Zhang, H., Jin, C., Zhao, C., et al. (2018). The sweet side of global change-dynamic responses of non-structural carbohydrates to drought, elevated CO2 and nitrogen fertilization in tree species. Tree Physiol. 38, 1706–1723. doi: 10.1093/treephys/tpy059
Lv, W.-T., Lin, B., Zhang, M., and Hua, X.-J. (2011). Proline accumulation is inhibitory to arabidopsis seedlings during heat stress. Plant Physiol. 156, 1921–1933. doi: 10.1104/pp.www.plantphysiol.org/cgi/of
Marchin, R. M., Backes, D., Ossola, A., Leishman, M. R., Tjoelker, M. G., and Ellsworth, D. S. (2022). Extreme heat increases stomatal conductance and drought-induced mortality risk in vulnerable plant species. Glob. Chang. Biol. 28, 1133–1146. doi: 10.1111/gcb.15976
Marias, D. E., Meinzer, F. C., Woodruff, D. R., and McCulloh, K. A. (2017). Thermotolerance and heat stress responses of Douglas-fir and ponderosa pine seedling populations from contrasting climates. Tree Physiol. 37, 301–315. doi: 10.1093/treephys/tpw117
Martín, J. A., Solla, A., Coimbra, M. A., and Gil, L. (2008b). Metabolic fingerprinting allows discrimination between Ulmus pumila and U. minor, and between U. minor clones of different susceptibility to Dutch elm disease. Forest Pathol. 38, 244–256. doi: 10.1111/j.1439-0329.2007.00542.x
Martín, J. A., Solla, A., Domingues, M. R., Coimbra, M. A., and Gil, L. (2008a). Exogenous phenol increase resistance of Ulmus minor to Dutch elm disease through formation of suberin-like compounds on xylem tissues. Environ. Exp. Bot. 64, 97–104. doi: 10.1016/j.envexpbot.2008.05.004
Martínez-Vilalta, J., Sala, A., Asensio, D., Galiano, L., Hoch, G., Palacio, S., et al. (2016). Dynamics of non-structural carbohydrates in terrestrial plants: A global synthesis. Ecol. Monogr. 86, 495–516. doi: 10.1002/ecm.1231
Matesanz, S., and Valladares, F. (2014). Ecological and evolutionary responses of mediterranean plants to global change. Environ. Exp. Bot. 103, 53–67. doi: 10.1016/j.envexpbot.2013.09.004
Míguez-Soto, B., and Fernández-López, J. (2015). Variation in adaptive traits among and within Spanish and European populations of Castanea sativa: Selection of trees for timber production. New For. 46, 23–50. doi: 10.1007/s11056-014-9445-5
Míguez-Soto, B., Fernández-Cruz, J., and Fernández-López, J. (2019). Mediterranean and northern Iberian gene pools of wild Castanea sativa Mill. are two differentiated ecotypes originated under natural divergent selection. PLoS One 14:e0211315. doi: 10.1371/journal.pone.0211315
Moustakas, M., Sperdouli, I., Kouna, T., Antonopoulou, C. I., and Therios, I. (2011). Exogenous proline induces soluble sugar accumulation and alleviates drought stress effects on photosystem II functioning of Arabidopsis thaliana leaves. Plant Growth Regul. 65, 315–325. doi: 10.1007/s10725-011-9604-z
Nievola, C. C., Carvalho, C. P., Carvalho, V., and Rodrigues, E. (2017). Rapid responses of plants to temperature changes. Temperature 4, 371–405. doi: 10.1080/23328940.2017.1377812
Niinemets, Ü (2010). Responses of forest trees to single and multiple environmental stresses from seedlings to mature plants: Past stress history, stress interactions, tolerance and acclimation. For. Ecol. Manag. 260, 1623–1639. doi: 10.1016/j.foreco.2010.07.054
O’Brien, M. J., Leuzinger, S., Philipson, C. D., Tay, J., and Hector, A. (2014). Drought survival of tropical tree seedlings enhanced by non-structural carbohydrate levels. Nat. Clim. Change 4, 710–714. doi: 10.1038/nclimate2281
Piper, F. I. (2011). Drought induces opposite changes in the concentration of non-structural carbohydrates of two evergreen nothofagus species of differential drought resistance. Ann. For. Sci. 68, 415–424. doi: 10.1007/s13595-011-0030-1
Poudel, P. B., and Poudel, M. R. (2020). Heat stress effects and tolerance in wheat: A review. J. Biol. Today’s World 9:217.
Rehschuh, R., Cecilia, A., Zuber, M., Faragó, T., Baumbach, T., Hartmann, H., et al. (2020). Drought-induced xylem embolism limits the recovery of leaf gas exchange in scots pine. Plant Physiol. 184, 852–864. doi: 10.1104/pp.20.00407
Rhodes, M., Robins, R., Hamill, J., Parr, A., and Walton, N. (1987). Secondary product formation using Agrobacterium rhizogenes transformed hairy root cultures. IAPTC Newsl. 53, 2–15.
Rita, A., Camarero, J. J., Nolè, A., Borghetti, M., Brunetti, M., Pergola, N., et al. (2020). The impact of drought spells on forests depends on site conditions: The case of 2017 summer heat wave in southern Europe. Glob. Change Biol. 26, 851–863. doi: 10.1111/gcb.14825
Rivero, R. M., Ruiz, J. M., García, P. C., López-Lefebre, L. R., Sánchez, E., and Romero, L. (2001). Resistance to cold and heat stress: Accumulation of phenolic compounds in tomato and watermelon plants. Plant Sci. 160, 315–321. doi: 10.1016/s0168-9452(00)00395-2
Ruehr, N. K., Gast, A., Weber, C., Daub, B., and Arneth, A. (2015). Water availability as dominant control of heat stress responses in two contrasting tree species. Tree Physiol. 36, 164–178. doi: 10.1093/treephys/tpv102
Scheiner, S. M. (1993). Genetics and evolution of phenotypic plasticity. Annu. Rev. Ecol. Syst. 24, 35–68.
Shivashankara, K. S., Pavithra, K. C., and Geetha, G. A. (2016). “Antioxidant protection mechanism during abiotic stresses,” in Abiotic stress physiology of horticultural crops, eds N. K. Srinivasa Rao, K. S. Shivashankara, and R. H. Laxman (Berlin: Springer), 47–69. doi: 10.1007/978-81-322-2725-0
Singh, B., Kumar, A., and Malik, A. K. (2017). Flavonoids biosynthesis in plants and its further analysis by capillary electrophoresis. Electrophoresis 38, 820–832. doi: 10.1002/elps.201600334
Singleton, V. L., Orthofer, R., and Lamuela-Raventós, R. M. (1999). Analysis of total phenols and other oxidation substrates and antioxidants by means of Folin-Ciocalteu reagent. Methods Enzymol. 299, 152–178. doi: 10.1016/j.scienta.2016.11.004
Solla, A., Martín, J. A., Ouellette, G. B., and Gil, L. (2005). Influence of plant age on symptom development in Ulmus minor following inoculation by Ophiostoma novo-ulmi. Plant Dis. 89, 1035–1040. doi: 10.1094/PD-89-1035
Spinoni, J., Naumann, G., Carrao, H., Barbosa, P., and Vogt, J. (2014). World drought frequency, duration, and severity for 1951-2010. Int. J. Climatol. 34, 2792–2804. doi: 10.1002/joc.3875
Thalmann, M., and Santelia, D. (2017). Starch as a determinant of plant fitness under abiotic stress. New Phytol. 214, 943–951. doi: 10.1111/nph.14491
Tixier, A., Orozco, J., Roxas, A. A., Earles, J. M., and Zwieniecki, M. A. (2018). Diurnal variation in nonstructural carbohydrate storage in trees: Remobilization and vertical mixing. Plant Physiol. 178, 1602–1613. doi: 10.1104/pp.18.00923
Valladares, F., Matesanz, S., Guilhaumon, F., Araújo, M. B., Balaguer, L., Benito-Garzón, M., et al. (2014). The effects of phenotypic plasticity and local adaptation on forecasts of species range shifts under climate change. Ecol. Lett. 17, 1351–1364. doi: 10.1111/ele.12348
Vázquez-González, C., Sampedro, L., López-Goldar, X., Solla, A., Vivas, M., Rozas, V., et al. (2022). Inducibility of chemical defences by exogenous application of methyl jasmonate is long-lasting and conserved among populations in mature Pinus pinaster trees. For. Ecol. Manage 518:120280. doi: 10.1016/j.foreco.2022.120280
Villemereuil, P. D., Mouterde, M., Gaggiotti, O., and Till-Bottraud, I. (2018). Patterns of phenotypic plasticity and local adaptation in the wide elevation range of the alpine plant Arabis alpina. J. Ecol. 106, 1952–1971. doi: 10.1111/1365-2745.12955
Wahid, A. (2007). Physiological implications of metabolite biosynthesis for net assimilation and heat-stress tolerance of sugarcane (Saccharum officinarum) sprouts. J. Plant Res. 120, 219–228. doi: 10.1007/s10265-006-0040-5
Wahid, A., and Ghazanfar, A. (2006). Possible involvement of some secondary metabolites in salt tolerance of sugarcane. J. Plant Physiol. 163, 723–730. doi: 10.1016/j.jplph.2005.07.007
Wahid, A., Gelani, S., Ashraf, M., and Foolad, M. R. (2007). Heat tolerance in plants: An overview. Environ. Exp. Bot. 61, 199–223. doi: 10.1016/j.envexpbot.2007.05.011
Wani, S. H., and Kumar, V. (2020). Heat stress tolerance in plants: Physiological, molecular and genetic perspectives. First edit. Hoboken, NJ: JohnWiley & Sons Ltd. doi: 10.1002/9781119432401
Weston, D. J., and Bauerle, W. L. (2007). Inhibition and acclimation of C3 photosynthesis to moderate heat: A perspective from thermally contrasting genotypes of Acer rubrum (red maple). Tree Physiol. 27, 1083–1092. doi: 10.1093/treephys/27.8.1083
Wiley, E., and Helliker, B. (2012). A re-evaluation of carbon storage in trees lends greater support for carbon limitation to growth. New Phytol. 195, 285–289. doi: 10.1111/j.1469-8137.2012.04180.x
Xiong, D., Yu, T., Ling, X., Fahad, S., Peng, S., Li, Y., et al. (2015). Sufficient leaf transpiration and nonstructural carbohydrates are beneficial for high-temperature tolerance in three rice (Oryza sativa) cultivars and two nitrogen treatments. Funct. Plant Biol. 42, 347–356. doi: 10.1071/FP14166
Xu, B. J., and Chang, S. K. C. (2007). A comparative study on phenolic profiles and antioxidant activities of legumes as affected by extraction solvents. J. Food Sci. 72, S159–S166. doi: 10.1111/j.1750-3841.2006.00260.x
Yin, R., Han, K., Heller, W., Albert, A., Dobrev, P. I., Zažímalová, E., et al. (2014). Kaempferol 3-O-rhamnoside-7-O-rhamnoside is an endogenous flavonol inhibitor of polar auxin transport in Arabidopsis shoots. New Phytol. 201, 466–475. doi: 10.1111/nph.12558
Zandalinas, S. I., Sales, C., Beltrán, J., Gómez-Cadenas, A., and Arbona, V. (2017). Activation of secondary metabolism in citrus plants is associated to sensitivity to combined drought and high temperatures. Front. Plant Sci. 7:1954. doi: 10.3389/fpls.2016.01954
Zas, R., Sampedro, L., Solla, A., Vivas, M., Lombardero, M. J., Alía, R., et al. (2020). Dendroecology in common gardens: Population differentiation and plasticity in resistance, recovery and resilience to extreme drought events in Pinus pinaster. Agric. For. Meteorol. 291:108060. doi: 10.1016/j.agrformet.2020.108060
Keywords: abiotic stress, heat wave, isorhamnetin, LC-MS, plant stress, primary metabolism, secondary metabolism, genetic variation in phenotypic plasticity
Citation: Dorado FJ, Pinto G, Monteiro P, Chaves N, Alías JC, Rodrigo S, Camisón Á and Solla A (2023) Heat stress and recovery effects on the physiology and biochemistry of Castanea sativa Mill.. Front. For. Glob. Change 5:1072661. doi: 10.3389/ffgc.2022.1072661
Received: 17 October 2022; Accepted: 21 December 2022;
Published: 09 January 2023.
Edited by:
Ned Fetcher, Wilkes University, United StatesReviewed by:
Romà Ogaya, Ecological and Forestry Applications Research Centre (CREAF), SpainJose Climent, Instituto Nacional de Investigación y Tecnología Agroalimentaria (INIA), Spain
Copyright © 2023 Dorado, Pinto, Monteiro, Chaves, Alías, Rodrigo, Camisón and Solla. This is an open-access article distributed under the terms of the Creative Commons Attribution License (CC BY). The use, distribution or reproduction in other forums is permitted, provided the original author(s) and the copyright owner(s) are credited and that the original publication in this journal is cited, in accordance with accepted academic practice. No use, distribution or reproduction is permitted which does not comply with these terms.
*Correspondence: Alejandro Solla, YXNvbGxhQHVuZXguZXM=