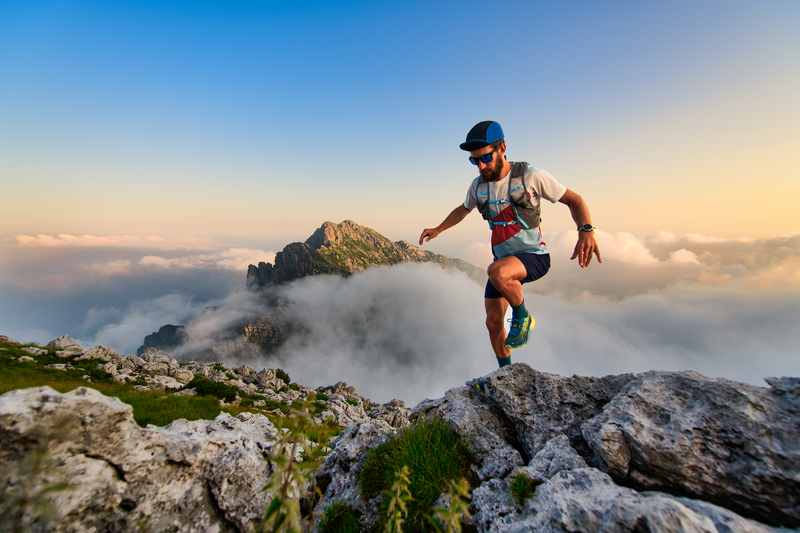
95% of researchers rate our articles as excellent or good
Learn more about the work of our research integrity team to safeguard the quality of each article we publish.
Find out more
ORIGINAL RESEARCH article
Front. For. Glob. Change , 04 January 2023
Sec. Forest Ecophysiology
Volume 5 - 2022 | https://doi.org/10.3389/ffgc.2022.1018789
This article is part of the Research Topic Understanding Forest Ecosystems: The Use of Stable Isotopes and Physiological Measurements View all 6 articles
Determining tree response to climate stress is critical for predicting changes in forest carbon dynamics as well as tree mortality. In temperate deciduous forests, describing this response is complicated by the complex diversity of leaf and wood characteristics among co-existing species. Furthermore, because of the inherent logistical limitations of measuring mature forest trees, many carbon models and stress-response studies are informed by physiological data collected from juvenile trees (seedlings or saplings). However, the extent to which juvenile and mature trees differ in their physiological responses to water stress is not well documented under natural conditions. The majority of carbon sequestered in a forest is in mature trees; therefore, direct canopy measurements comparing responses to climate in juvenile and mature trees would allow us to more accurately predict changes in ecosystem carbon uptake. Here, we present data describing the physiological responses to summer water stress in juvenile trees of six temperate deciduous species. Our results indicate that species exhibited variation and plasticity in stress hydraulic parameters yet maintained similar rates of carbon uptake. We demonstrate how integrative photosynthetic parameters, such as photosynthetic capacity and quantum efficiency of photosystem II, are beneficial for wholistically displaying physiological responses at the leaf level. We further compared seasonal patterns of leaf water potential during decreasing soil water availability between the juvenile trees and co-existing mature trees of the same species. Our data reveal that while some species remain static in their hydraulic behavior from the juvenile to adult stage, other species are dynamic between life stages. Models, as well as experimental studies examining tree response to stressors, should plan for plasticity in physiological parameters among co-existing species, and should further allow variability between life stages for particular species. The capacity to effectively inform models from data collected in mature trees will inevitably lead to improved predictions of tree mortality and forest carbon trajectories.
Measuring the physiological mechanisms governing carbon and water cycles in trees is critical for accurately predicting forest carbon uptake (Bassow and Bazzaz, 1997; Hudiburg et al., 2013). Earth system models (ESMs) currently responsible for making these predictions rely on tree-level physiological measurements (Kwon et al., 2018; Lu et al., 2022). Because ESMs depend on physiological parameters (e.g., VCmax) that are more easily collected from small trees, long-term carbon trajectories are often informed by data collected from juvenile (i.e., seedling or sapling) trees (Hartmann et al., 2018). However, the extent to which tree responses differ between juvenile and mature individuals, especially during environmental stress, is still being resolved. Previous studies highlight the importance of considering size- and age-related differences in physiological responses (Kolb et al., 1997; Day et al., 2001; Steppe et al., 2011) and have reported differences in gas exchange (Bond, 2000) and water potential among juvenile and mature conifers in drought-prone regions (Ryan and Yoder, 1997; Kolb and Stone, 2000), and in some temperate deciduous species (Fredericksen et al., 1996; Hölscher, 2004). However, more research is needed that focuses on age-related differences in physiological activity during times of environmental stress (Adams et al., 2017), particularly in angiosperms (Steppe et al., 2011). Some species may be static in their physiological strategies from a juvenile to an adult stage, while others may be dynamic (Zhang et al., 2018). Quantifying these differences in co-existing species will help us develop dynamic parameterization in models to account for plasticity in carbon-related physiological traits (Babst et al., 2021), especially for species that exhibit variability in carbon allocation strategies over the course of their lives (Klein, 2014).
Several reasons exist for why physiological strategies would differ between age groups within a species (Steppe et al., 2011). Larger mature trees have a greater capacity for carbon storage, which allows for a greater ability to buffer stress. Carbon accumulation increases as trees age due to increasing tree size and leaf area (Stephenson et al., 2014), allowing mature trees to preferentially allocate carbon to storage over growth (Smith and Stitt, 2007; Genet et al., 2009; Hartmann and Trumbore, 2016). The majority of carbon in trees is structural and not available for reallocation; however, stored carbon (quantified as non-structural carbohydrates: NSCs) can be moved to aid in drought tolerance (Piper, 2011; Yoshimura et al., 2016; Tomasella et al., 2020) and potentially contribute to xylem embolism reversal (Zwieniecki and Holbrook, 2009; Savi et al., 2016; Trifilò et al., 2019). Therefore, during times of stress, mature trees with sufficient reservoirs of stored carbon can conserve water at the expense of gaining new carbon. However, juvenile trees have different characteristics (e.g., less sapwood and leaf area) than larger, mature trees (Ryan and Yoder, 1997; Wright et al., 2004; Steppe et al., 2011), reducing their capacity to allocate carbon to storage. In young trees, most carbon is allocated to metabolism and growth, potentially limiting physiological responses during environmental stress. Rapid growth through the understory is necessary during early development making carbon acquisition a major priority for juvenile trees. Therefore, a trade-off exists between growth necessity and stress tolerance that inherently changes with developmental stage, and must be considered when describing physiological stress responses in trees.
Trees directly respond to changes in their environment by altering leaf-level properties and exhibit a large range of responses among individuals (D’Orangeville et al., 2018; Fu and Meinzer, 2018; Hochberg et al., 2018). During water stress, trees can immediately close their stomata, which minimizes water loss but reduces carbon uptake – this strategy is referred to as isohydry (Tardieu and Simonneau, 1998). Alternatively, trees can maintain gas exchange during stressful conditions (post-disturbance, drought, etc.) at the expense of continued water loss, which is termed anisohydry (Ratzmann et al., 2019). A spectrum of strategies exists between these two extremes and strategy varies depending on a number of different traits (Klein, 2014; Johnson et al., 2018a; Bryant et al., 2022). Species-specific characteristics governing carbon and water trade-offs result from different leaf (Wright et al., 2004) and wood (Chave et al., 2009) characteristics that influence carbon allocation and thus, tree response to stress. Differences in wood anatomy (Wheeler et al., 2007), as well as differences in wood density (Chave et al., 2009), rooting depth (Stone and Kalisz, 1991), leaf phenology (Lechowicz, 1984), xylem characteristics (Jacobsen et al., 2012), growth rate, etc., all contribute to variability in carbon allocation among species in temperate forests (Hoch et al., 2003).
Species-specific responses, as well as the degree of plasticity within a species, have not been adequately studied in natural settings (Kannenberg et al., 2019). Including variability for species’ responses in model parameters is particularly important in species-rich areas (Bassow and Bazzaz, 1997), such as temperate deciduous forests (Muraoka et al., 2013; Saurer et al., 2014; Zhang et al., 2018), with varying microsite conditions (Tai et al., 2017). We therefore investigated the physiological strategies during summer water stress in co-existing tree species that vary genetically and morphologically. We included Acer saccharum, Fagus grandifolia and Platanus occidentalis, all of which have diffuse wood anatomy. We also studied Quercus rubra, Quercus alba, and Carya ovata, which have ring-porous wood anatomy, and therefore, a higher annual carbon requirement for the creation of large vessels in early spring (Barbaroux and Bréda, 2002). We measured typical metrics of gas exchange (net photosynthesis and stomatal conductance), but also recorded physiological parameters that are representative of photosynthetic capacity and efficiency, and are included in current Earth system carbon models. Fv’/Fm’ – the ratio of variable to maximum fluorescence is the maximum quantum efficiency of photosystem II in the light and is an indicator of the degree of physiological stress (Marshall et al., 2001; Nippert et al., 2004). Another indicator of environmental stress response is PhiPS2 (Comparini et al., 2020), which is the actual quantum efficiency of photosystem II. We also measured ci/ca, which is the ratio of carbon inside the leaf (ci) relative to the available atmospheric carbon (ca). A higher ci/ca value indicates nonstomatal limitation of carbon capture by the leaf (Brodribb, 1996; Tan et al., 2017). Lastly, and importantly, we measured midday leaf water potential and specific leaf area (SLA) in the juvenile trees as well as co-existing mature trees of the same species (Bryant et al., 2022). Water potential is a commonly used metric describing tree water stress and SLA is an important parameter in Earth system models (Fisher et al., 2015) which can influence changes in ecosystem-level NPP (White et al., 2000). SLA has also been correlated with vulnerability to drought-related mortality (Greenwood et al., 2017); however, the relationship is unresolved for angiosperms (O’Brien et al., 2017; Schwartz et al., 2020). Overall, our first goal was to compare changes in these important physiological parameters in six temperate forest species with varying carbon requirements during summer water stress. We predicted that physiological responses would differ among species as the season progressed and that parameters representing photosynthetic capacity and efficiency would be more informative for understanding those differences. Our second goal was to determine the extent to which hydraulic stress responses varied between juvenile and mature trees within each species. We expected that patterns of midday leaf water potential would significantly differ between age groups, but that general patterns and direction of response would be similar within a species.
Our study was conducted in Athens, Ohio in the Ohio University Ridges Land Laboratory (39°32’50” N, 82°11’90” W). The climate in southeastern Ohio is temperate with a mean annual temperature of 11.44°C and mean annual precipitation of 1,018 mm (Huntington et al., 2017). The Ridges Land Lab is a deciduous, second-growth forest with a highly dissected topography and fine loam soil (Meekins and McCarthy, 2001; DeForest, 2009). Our study took place during the 2019 growing season which was much drier than the previous year. To characterize environmental conditions, we recorded soil moisture, temperature, and humidity, and downloaded precipitation data from the PRISM Climate Group (Daly et al., 2000).
Our study design included 18 juvenile trees and 18 mature trees across six different species (three individuals within each age class in each species) that were co-existing in similar climate and soil conditions. We included species representing contrasting wood anatomies. The diffuse-porous species included A. saccharum, F. grandifolia, and P. occidentalis, while the ring-porous species included Q. rubra, Q. alba, and C. ovata. In the 18 juvenile trees, we measured gas exchange and leaf water potential and only included trees with a diameter of less than 10 cm in diameter at breast height (DBH). Specifically, DBH ranged from 3.0 to 8.1 cm for all juvenile trees. In the 18 mature trees, we measured leaf water potential and monitored sap flux and only included trees that were greater than 20 cm DBH; specifically, tree diameter ranged from 20.3 to 94.5 cm for mature trees (Bryant et al., 2022). Here we show hydraulic parameters in mature trees for comparisons with juveniles; however, we discuss the details of hydraulic responses in the mature trees in more detail in a previous manuscript (Bryant et al., 2022). We also measured soil moisture under all 36 trees individually to determine consistent water availability among individuals. All trees were located at similar positions on the landscape – on a flat portion of an upland ridge so that water access was similar among all trees. The exception was P. occidentalis, which was located approximately 100 meters laterally from the other study trees, in a small grove, just past the forest edge. This species requires high moisture and light conditions and therefore occupies a more specific habitat niche than the other species. We consider the different light environment of this species in our conclusions.
We continuously monitored temperature and relative humidity across the 2019 season with a weatherproof HOBO data logger (Onset Computer Co., Bourne, MA, USA) installed in the tree canopy in the middle of our site. To estimate atmospheric water availability, we calculated vapor pressure deficit (Cunningham, 2004; Grossiord et al., 2017) from temperature and relative humidity data collected at 20-min intervals with the data logger. We calculated vapor pressure deficit (VPD) with the equation (Looker et al., 2018):
where e(T) is the saturation vapor pressure at air temperature T(°C) and RH (%) is relative humidity. The parameter e(T) is calculated from the following equation (Tetons, 1930):
We also measured soil volumetric water content (%v/v) to represent soil water availability, using an 11-cm soil moisture probe (Hydrosense II, Campbell Scientific). We measured soil moisture at four locations around each tree (both juveniles and mature trees) and then recorded the mean. We collected soil moisture measurements weekly for the duration of our study, adjusting our schedule to capture precipitation events.
We measured gas exchange (net photosynthesis and stomatal conductance) directly on leaves in the field with two infrared gas analyzers (Li-Cor 6400, Li-Cor Biosciences, Lincoln, NE, USA) using a 2x3 cm chamber. We set the reference CO2 to 400 ppm then measured air temperature and humidity with the IRGA, and photosynthetically active radiation (PAR; μmol m–2 s–1) with a quantum sensor, to set the cuvette conditions similar to the ambient environment. The leaves were allowed to acclimate in the Li-Cor chamber for 2 min prior to each measurement. To represent ambient conditions, the cuvette conditions were set to environmental conditions at the time and varied by month (Supplementary Table 1). From the gas exchange measurements, we also derived ci/ca, which is the ratio of carbon inside the leaf relative to ambient carbon. We conducted measurements with a fluorescence chamber (Maxwell and Johnson, 2000; Murchie and Lawson, 2013), which allowed us to derive Fv’/Fm’ (ratio of light-adapted variable to maximum fluorescence) and PhiPS2 (actual quantum yield of photosystem II). We collected mid-day gas exchange during a single day once a month during July, August and September, at approximately the same week each month. For consistency, we took measurements between the hours of 10:00 AM–2:00 PM. We measured three leaves on each of our 18 trees. Prior to each gas exchange measurement, we measured each leaf with a SPAD (Soil-plant analysis development) meter that is a unitless measurement indicative of chlorophyll and nitrogen content (Chang and Robison, 2003).
We measured specific leaf area in the 18 juvenile trees as well as 18 mature trees (three leaves in each tree for six species in each age class). We extracted leaf disks from each of the leaves using a 10-mm leaf borer and dried then weighed the mass of the leaf disks. We divided the area of the borer by the mass of each leaf disk to yield leaf mass per area and then used the inverse to report specific leaf area.
We measured midday leaf water potential in the field using a pressure chamber (Scholander et al., 1964) once a month following the gas exchange measurements. Briefly, we excised the leaf immediately adjacent to the leaf measured for gas exchange (three from each individual tree). The leaves were recut at the petiole with a razor blade as needed to produce a flat and consistent surface immediately prior to inserting them into the pressure chamber (Turner, 1988). Water potential measurements were recorded in megapascals as soon as a pressure equilibrium in the chamber was reached as indicated by the extrusion of xylem sap on the petiole.
To represent relative water movement in mature trees, we utilized relative sap flux values (Bryant et al., 2022) from the same season in which we took measurements on the juvenile trees. We had installed sensors and measured sap flux in 27 trees total, including four to six individuals of each of the six species (and all 18 trees measured for water potential). We constructed sap flux sensors according to the original procedure established by Granier (1985, 1987) but considered more recently published suggestions in probe construction and installation (Lu et al., 2004; Davis et al., 2012). For a more detailed description of sap flux methods see Bryant et al. (2022). Because absolute sap flux values are highly variable depending on species, environment, and wood type (Lu et al., 2004; Gebauer et al., 2008), we normalized our sap flux rates and report relative sap flux values (Ford et al., 2004; Braun et al., 2010; Brinkmann et al., 2016). We then averaged relative sap flux values for individual trees within a species to produce mean relative sap flux values for each species.
We wanted to compare the relationship between tree water flow and leaf water potential in juvenile and mature trees, but due to logistics, measured different metrics of water movement for each group. We therefore relativized the stomatal conductance values for the juveniles and the sap flux values for the mature trees, such that values were expressed as a proportion of the maximum value measured. Because sap flux was measured continuously, the maximum rate recorded captured the actual maximum tree water flow in the mature group. In the juveniles, stomatal conductance measurements were limited to survey recordings. However, we completed diurnal gas exchange measurements on all trees at 2-h intervals (Supplementary Figure 1) in late July to ensure that we were capturing maximum conductance rates for all species. From these data, we found that all species exhibited peak stomatal conductance between 10:00 AM and 2:00 PM, and that measuring during this time of day was appropriate for capturing maximum rates of conductance.
To quantify and compare stomatal conductance (gs), net photosynthesis (Anet), maximum quantum yield (Fv’/Fm’), actual photosystem efficiency (PhiPS2), ratio of internal leaf carbon to ambient carbon (ci/ca), and chlorophyll content among species over the growing season, we used least-squares regression and performed a mixed-effects linear model with the package lme4 in R (Bates et al., 2015). In each model, one of the aforementioned variables was the response variable, species and month were fixed effects; we treated tree ID as a random effect and specified an autocorrelation structure for time in the model. When the interaction between species and month was significant, we performed a type III ANOVA to determine how each variable differed among species and months. We then used the package emmeans to perform a post-hoc pairwise comparison, which allowed us to determine how each variable differed among species during each month, and for which species parameters differed among months.
To determine how leaf water potential and specific leaf area (SLA) differed between age classes within a species over the growing season, we again used least-squares regression and performed a mixed-effects linear model, where age, species and month were fixed effects, and tree ID was treated as a random effect. We then performed a type III ANOVA to determine how leaf water potential or SLA differed between ages within a species. We then used the package emmeans to conduct post-hoc pairwise comparisons where necessary. Lastly, we combined the data to determine the relationship between relative water movement (stomatal conductance for juveniles, sap flux for mature trees) and leaf water potential for each age group. In the overall model, species (p = 0.59), nor the interaction between species and water potential (p = 0.74) were significant, so we dropped them from the overall model and included species as a random effect, allowing us to observe the relationship within a larger sample size (n = 18 trees per age group per month, for 108 total data points).
Overall, we found interesting and notable differences in physiological parameters among species as water availability decreased for trees during the 2019 growing season. Precipitation decreased over the course of the growing season and soil water content was less than 10% (%v/v) for the majority of the study period (Supplementary Figure 2). Mean maximum daily vapor pressure deficit ranged between 1.0 and 1.5 kPa for most of the season but reached maximums of 2.0 kPa by September. These conditions created a relatively dry season for the southeast Ohio region, allowing us to observe species- and age-related differences in physiological parameters during seasonal water stress. In temperate systems that have highly variable water regimes, water stress is relative to local conditions. In 2019, September water availability was 0–10% of the long-term average (30 years) according to the PRISM drought indicator tool (Daly et al., 2000). Our results demonstrate that stomatal conductance declined seasonally in all species (p < 0.001), while photosynthesis increased from August to September in most species (Figure 1). Photosynthesis was consistently highest in P. occidentalis, though not statistically different from that of Q. alba.
Figure 1. Mean (+/-SE) physiological variables (collected at midday) indicating environmental stress responses among six temperate juvenile trees during summer in 2019. From top to bottom: gs (stomatal conductance); Anet (net rate of photosynthesis); Fv’/Fm’ (maximum quantum capacity of photosystem II); PhiPS2 (actual quantum efficiency of photosystem II); ci/ca (ratio of intercellular carbon to atmospheric carbon; and SPAD reading (unitless; representing chlorophyll content). Patterns and rates of parameters varied seasonally by species.
In addition to measuring net gas exchange, we also recorded several photosynthetic parameters indicative of leaf-level carbon uptake capacity. In all species, except for P. occidentalis, Fv’/Fm’ appeared to decrease from July to August but was statistically similar across the season. However, for P. occidentalis, the trend was reversed, increasing from July to August (though not significantly). Additionally, we found that PhiPS2 was highest in P. occidentalis (p < 0.01) and again demonstrated a contrasting seasonal pattern relative to the other species (p = 0.05). Our data show that seasonal patterns of ci/ca differed among species (p < 0.05); specifically, ci/ca increased from August to September in both A. saccharum and C. ovata, but decreased during this time period in all other species. SPAD (representing chlorophyll content) differed among species (p < 0.001) and months (p < 0.001), decreasing over time for all species (p < 0.05), except for C. ovata and A. saccharum, both of which maintained relatively consistent values across the season. In general, chlorophyll content was higher (p < 0.05) for the ring-porous trees (C. ovata, Q. alba and Q. rubra) than for the diffuse-porous trees (A. saccharum, F. grandifolia, and P. occidentalis).
We further compared variables against each other to understand the differences in seasonal patterns of photosynthesis and stomatal conductance among species. We found that net photosynthesis significantly increased with stomatal conductance for all trees during July (p = 0.03) and August (p < 0.01); however, the relationship decoupled by September (p = 0.66; Figure 2).
Figure 2. Net photosynthesis significantly increased with stomatal conductance in all trees during July and August, but the relationship decoupled by September. Different colors represent different species and different shapes represent diverse wood types; solid circles are diffuse-porous species and opened circles are ring-porous species. Each data point represents the mean of three individual trees. The model fit line is based on a 95% confidence interval (shaded in gray).
Our analyses also revealed a significantly negative correlation between net photosynthesis and ci/ca (p = 0.002, R2 = 0.67) for all trees (Supplementary Figure 3) and that PhiPS2 was significantly positively correlated with stomatal conductance (p = 0.02, R2 = 0.75) in all species (Supplementary Figure 4). To further visualize the influences of stomatal versus biochemical limitations on photosynthesis, we plotted ci/ca as a function of stomatal conductance for each species (Figure 3). We display this relationship against a theoretical curve that demonstrates the relationship if conductance was the only limitation to photosynthesis, which was first explained by Brodribb (1996). Departures from the theoretical curve at low rates of stomatal conductance indicate nonstomatal inhibition of photosynthesis (Brodribb, 1996).
Figure 3. Response of ci/ca to the seasonal decline in stomatal conductance for each species. The curve represents the theoretical response if stomatal conductance was the only limitation to photosynthesis; departures from the curve at low conductance values indicate nonstomatal limitation (Brodribb, 1996). Curves are fit to the respective range of conductance values for each species. Each data point represents a single leaf at a single time point.
We also measured leaf water potential over the course of the season (Figure 4) to indicate changes in hydraulic stress within the trees as environmental water decreased (Ratzmann et al., 2019). In the juvenile trees, seasonal patterns of leaf water potential differed among species (p < 0.001), with the highest values observed in A. saccharum (p < 0.01). Water potential remained similar over time for both A. saccharum and P. occidentalis, despite decreasing soil moisture (p < 0.001). In contrast, water potential steadily decreased seasonally for all three ring-porous species: Q. rubra, Q. alba, and C. ovata (p < 0.05). Interestingly, water potential in F. grandifolia increased from July to August then significantly decreased by September (p < 0.01).
Figure 4. Physiological parameters collected in co-existing juvenile and mature tree species. (Top row) mean midday leaf water potential during the driest part of the 2019 growing season for juvenile trees compared to co-existing mature trees of the same species (Bryant et al., 2022). (Bottom row) mean specific leaf per area (SLA) during the same time for the same trees. Vertical bars represent SE among individuals (n = 3 trees per species per age class) with a 95% confidence interval.
Water potential measurements for saplings were conducted simultaneously with measurements in co-existing mature trees of the same species (Bryant et al., 2022). We found that leaf water potential differed between juveniles and adults for some species (p < 0.01), but not others (Figure 4). Specifically, water potential was similar between juveniles and adults in A. saccharum and both Quercus species. However, leaf water potential was significantly lower in mature trees than in juveniles for both P. occidentalis and C. ovata. For F. grandifolia, water potential in saplings exhibited a contrasting seasonal pattern relative to mature trees.
We also found differences in specific leaf area between age classes (p < 0.001). Overall, SLA was higher in juvenile trees than mature trees (p < 0.001). However, in the mature trees, SLA increased seasonally (p < 0.001), while decreasing in the younger cohort (p = 0.02). In both age classes, SLA was highest in F. grandifolia (p < 0.01).
To further compare differences among physiological responses between life stages, we quantified relative rate of water movement as a function of leaf water potential for all trees (Figure 5). Relative rate of water movement was expressed as the relative rate of stomatal conductance in juvenile trees and as the relative rate of sap flux in mature trees. Our results demonstrate that the relationship between water potential and relative water movement differed between age groups (p = 0.01). When data for all species was combined (n = 18 trees per age group per month), relative water movement decreased as water potential declined for juveniles (p = 0.04), but not mature trees. However, mature trees tended to exhibit higher relative rates of water movement across the season.
Figure 5. Relationship between relative rate of water movement and leaf water potential for mature (not significant) and juvenile trees (p = 0.04, R2 = 0.41). Rate of water movement in trees is represented by rate of stomatal conductance in saplings and rate of sap flux in mature trees (Bryant et al., 2022). The solid line represents a significant relationship at a 95% confidence interval (shaded). Data were combined and species included as a random effect (n = 18 trees per age group per month).
Considerable effort has been put into describing a tree’s physiological performance based off the response of hydraulic parameters to decreasing soil water availability (Tardieu and Simonneau, 1998; Domec et al., 2004; Martínez-Vilalta and Garcia-Forner, 2017; Fu and Meinzer, 2018). Declines in stomatal conductance (Loewenstein and Pallardy, 1998; Bond and Kavanagh, 1999; Panek and Goldstein, 2001; Klein, 2014) and sap flow (Martínez-Vilalta et al., 2003; Steppe et al., 2015; Brinkmann et al., 2016; Looker et al., 2018) have both been linked with increasing water stress and used to infer the capacity for tree functioning. These hydraulic metrics are commonly connected with carbon uptake variables – usually maximum or net photosynthesis (Catovsky et al., 2002; Irvine et al., 2002; Johnson et al., 2018b). However, some studies have emphasized the importance of relating hydraulic parameters to variables indicative of photosynthetic capacity (Brodribb and Feild, 2000; Wang et al., 2019) and not just photosynthetic rate.
Our results demonstrate how including multiple metrics of photosynthetic activity can be more informative for understanding tree performance during summer water stress (Schulze, 1994; Prentice et al., 2014), especially in temperate forests with diverse co-existing species (Bassow and Bazzaz, 1997) and habitat conditions (Oren and Pataki, 2001; Tai et al., 2017). During the growing season, soil moisture steadily decreased (Supplementary Figure 2), resulting in drier than normal conditions for the region by September (Daly et al., 2000). We observed a simultaneous decrease in both stomatal conductance (Figure 1) and leaf water potential (Figure 4) for all trees, but the magnitude and rate of decline differed for each species. The most notable differences were between A. saccharum and C. ovata. Specifically, A. saccharum exhibited the most static leaf water potentials across the season, never exceeding –1 MPa. However, the trade-off was consistently low rates of stomatal conductance, eventually reaching zero by September. These data indicate that this species is physiologically functioning across the season with consistently low levels of water stress (Loewenstein and Pallardy, 1998). Opposingly, C. ovata demonstrated the greatest variability and declines in leaf water potential (Figure 4) and stomatal conductance (Figure 1), indicating contrasting hydraulic behavior (Klein, 2014; Ratzmann et al., 2019) as the season progressed and soil water availability decreased (Supplementary Figure 2).
Deciduous trees have unique seasonal physiology because of the natural senescence of leaves during the transition from summer to fall (Lechowicz, 1984; Moon et al., 2022). Each species deals with this transition differently (Bassow and Bazzaz, 1997; Noda et al., 2015), so physiological patterns naturally varied as the season ends. However, late-summer declines in water-availability exacerbated those natural responses, allowing us to view differences in the nuances of photosynthetic functioning during a possibly “stressful” time. Specifically, our results highlight the breakdown between photosynthesis and stomatal behavior in deciduous species during late summer (Figure 2). Without other information, an increase in photosynthesis with a simultaneous decline in stomatal conductance would typically indicate an increase in leaf-level water use efficiency (Ripullone et al., 2004) – especially in the context of reduced water availability (Saurer et al., 2014; Kwon et al., 2018; Lu et al., 2019). Considering only these data, that seems to be the trend (Supplementary Figure 5), indicating that all trees are equally efficient in their water use. However, when we consider variables representing photosynthetic capacity of the leaves, we see the detailed physiological differences among species (Noda et al., 2015; Wang et al., 2019).
Despite exhibiting opposing hydraulic responses, A. saccharum and C. ovata maintained similar specific leaf areas (SLA; Figure 4), indicating similar carbon “cost” (per unit of leaf area) to construct leaves. Specific leaf area is the inverse of leaf mass per area, which quantifies the amount of photosynthetic machinery relative to the mass of the leaf (Wright et al., 2004). Species with high specific leaf area therefore have low mass per area, meaning a lower capacity to take in carbon per unit of leaf area (Poorter et al., 2009). Less machinery to take in carbon therefore translates to higher rates of gas exchange in an effort to capture more carbon for the same amount of leaf area (Reich et al., 1998; Wright et al., 2004), which may result in additional water loss (Wilson et al., 1999; Poorter et al., 2009). In a global meta-analysis, Greenwood et al. (2017) found that tree species with higher SLA were more prone to mortality during drought. However, other studies have found weak relationships between SLA and drought-related mortality parameters (O’Brien et al., 2017; Schwartz et al., 2020). One reason that may explain the lack of consensus could be that SLA does not account for leaf size or number (Milla et al., 2008) and therefore, total active stomata of the leaf (and tree). The significantly lower leaf water potential values in C. ovata suggest that this species is using proportionately more of its stomata. Considering stomatal conductance relative to total stomatal density may be more informative for directly comparing stress responses in broad-leaved species (Brodribb, 1996), which greatly vary in leaf size and SLA. Furthermore, species greatly vary in total leaf number, which further convolutes the relationship between SLA and hydraulic performance (Milla et al., 2008). For example, F. grandifolia had the highest SLA relative to all other species and noticeably, the smallest leaf size. However, the small leaves and high SLA are offset by a higher total number of leaves in this species, so the physiology on a per-leaf basis may not be indicative of whole-tree susceptibility to water stress (Wilson et al., 1999; Poorter et al., 2009).
Photosynthetic metrics such as ci/ca are also useful for examining differences in physiological mechanisms between deciduous species (Prentice et al., 2014; Tan et al., 2017). This parameter is the ratio of CO2 inside (ci) the leaf relative to the available (ca) atmospheric CO2 (Brodribb, 1996). In our study, ci/ca trended similarly between C. ovata and A. saccharum despite their different water potentials and rates of conductance. For these two species, ci/ca increased toward the end of the season, while decreasing in all other species (Figure 1). The higher ci/ca value in September indicates less stomatal inhibition of carbon capture by the leaf (Brodribb, 1996) and is further indicative of the decoupling between photosynthesis and stomatal conductance. Assuming no biochemical limitation to carbon uptake, ci/ca would decrease when net photosynthesis is high; the departure from this for A. saccharum and C. ovata during September may represent nonstomatal limitation to carbon uptake. Brodribb (1996) demonstrated that theoretically, if stomatal conductance is the only limitation to photosynthesis, then ci/ca should exhibit a logarithmic response to decreasing stomatal conductance, which has been supported by recent studies (Tan et al., 2017). Departures from this relationship at low conductance values indicate biochemical rather than stomatal inhibition of carbon uptake. In contrast to conifers, broad-leaved species often showed departures from the theoretical relationship (Brodribb, 1996), suggesting that nonstomatal limitation is more common in broad-leaved trees (Prentice et al., 2014). We saw a similar relationship in our data, with few trees showing the theoretical trend between ci/ca and stomatal conductance (Figure 3).
The lack of relationship between ci/ca and stomatal conductance may also be due to the decrease in stomatal density (rather than per-unit conductance), which would not be reflected in typical survey gas exchange measurements. PhiPS2 is therefore a better indicator of stomatal limitation and has been linked with hydraulic conductivity (Brodribb and Feild, 2000). In our data, we see that PhiPS2 was correlated with stomatal conductance in all species except P. occidentalis (Figure 1). PhiPS2 is an important parameter for considering stress response (Comparini et al., 2020) because photosystem II is sensitive to environmental stresses, so reductions in photosynthetic efficiency will be apparent through PhiPS2 values (Maxwell and Johnson, 2000). The ratio of variable to maximum fluorescence Fv/Fm can also be a useful parameter for comparing stress levels among plants (Schulze, 1994; Marshall et al., 2001) because it indicates photosynthetic capacity (Nippert et al., 2004). In our data, Fv’/Fm’ was similar from the beginning to the end of the season in all species, indicating no change in plant stress (Maxwell and Johnson, 2000; Nippert et al., 2004) by September. However, we measured light-adapted ratios (Fv’/Fm’), which may not show as strong of a response as dark-adapted fluorescence measurements (Murchie and Lawson, 2013). PhiPS2 represents actual efficiency of photosystem II (often determined by stomatal conductance), while Fv’/Fm’ represents maximum capacity of photosystem II (Schulze, 1994). The decrease in PhiPS2 with the concurrent decrease in stomatal conductance suggests some degree of stomatal limitation to photosynthesis, but the variable patterns of the other parameters across species demonstrates the complexity of controls on photosynthesis in deciduous forests (Brodribb and Feild, 2000).
Fagus grandifolia was the only species in which quantum efficiency (PhiPS2) decreased in accordance with stomatal conductance, quantum capacity (Fv’/Fm’), and ci/ca. Interestingly, this species also showed the strongest declines in chlorophyll content, suggesting decreasing leaf nitrogen (Chang and Robison, 2003; Noda et al., 2015), but at the same time, specific leaf area (SLA) was decreasing. Perhaps future studies should consider leaf C:N ratios coupled with these parameters to understand how seasonal patterns of leaf allometry change with water stress in F. grandifolia. The anomalous pattern of leaf water potential in juveniles of this species also indicate complex interactions between carbon uptake and hydraulic functioning.
In a drought experiment with potted saplings, Kannenberg et al. (2019) found anisohydric behavior (declining water potentials despite loss of conductivity) in C. ovata and isohydry in A. saccharum. We suggest that opposing hydraulic responses (e.g., conservative versus declining water potentials) among species are equivocal strategies reflecting the differential carbon requirements among trees (due to differences in growth rate, wood anatomy, leaf economy, etc.). For example, A. saccharum is diffuse-porous, while C. ovata is ring-porous, which may contribute to the opposing hydraulic strategies because the larger vessels of ring-porosity require more carbon to recreate each season (Baas et al., 2004) than the small vessels in diffuse-porous trees (Barbaroux and Bréda, 2002). However, because carbon is already allocated toward replacing damaged vessels in the early wood each year, physiological functioning can continue despite embolized vessels in the outer ring later in the season (Hoffmann et al., 2011; O’Brien et al., 2017). So, from the tree perspective of carbon allocation, avoiding potential risk of embolism toward the end of the season is futile, as carbon will already be allocated toward construction of new vessels the following spring. Supporting this concept, the three ring-porous species in our study (C. ovata, Q. alba, and Q. rubra) exhibited the strongest seasonal decline in leaf water potentials in both juvenile and mature trees (Bryant et al., 2022). Furthermore, the ring-porous juveniles also had consistently higher chlorophyll content in their leaves, indicating more nitrogen (Chang and Robison, 2003) and possibly a higher potential for photosynthesis (Wright et al., 2004; Noda et al., 2015). Therefore, continued stomatal conductance despite decreasing water potentials may not matter for deciduous vessels (O’Brien et al., 2017) and may actually allow for increased photosynthetic capacity. Regardless, our study emphasizes the importance of holistically considering the variable carbon requirements and allocation patterns for co-existing deciduous species when determining and modeling (Mackay et al., 2015) forest stress responses (Johnson et al., 2018b; Fu et al., 2019).
The capacity for physiological plasticity in response to environmental cues has also become increasingly documented for some species (D’Orangeville et al., 2018; Fu and Meinzer, 2018; Hochberg et al., 2018). We speculate that some species may have greater variability in physiological traits among individuals than others during times of environmental stress. When possible, future studies should replicate sufficiently within a species to resolve the degree of plasticity among individuals. Our data for P. occidentalis demonstrate the influence of environment on physiological behavior (Hochberg et al., 2018). This species prefers high light and wet environments – particularly during early development; therefore, saplings grow in flat, grassy patches outside the edge of the forest, where sunlight and water are readily available. Reflecting adaptation to this high-resource environment, photosynthesis was consistently and substantially higher in P. occidentalis than that of the other species, despite similar declines in conductance (Figure 1) and relatively conservative leaf water potentials and SLA (Figure 4). These data demonstrate that while the hydraulic behavior in P. occidentalis is comparable to the other species, the overall rate and capacity of carbon uptake is much different. Our analyses showed that photosynthetic efficiency (PhiPS2) and ci/ca increased in August in this species, while decreasing in all others, which was actually a response to the light environment of the chamber. P. occidentalis was the only species in which we observed correlations between light level and physiological variables. In this case, the seasonal trends in physiological behavior are influenced less by tree carbon requirements or water availability and instead reflect microsite environmental characteristics (Tai et al., 2017).
Our results also reveal that within a species, age-related differences emerge in seasonal patterns of midday leaf water potential. For A. saccharum, Q. rubra, and Q. alba, seasonal patterns of water potential were similar between juvenile and mature trees (Figure 4). Therefore, data regarding hydraulic thresholds in saplings of Q. rubra may accurately reflect the responses of mature forest trees. In P. occidentalis leaf water potential was significantly lower in the mature trees relative to the juveniles during the dry months. In C. ovata, mature trees exhibited similar overall seasonal patterns of water potential as the younger trees, but at a significantly greater magnitude during all months (Figure 4), whichmay be attributed to deeper roots in the mature trees (Stone and Kalisz, 1991). In all species, the mature trees exhibited physiological activity at lower leaf water potentials during the driest months (Figure 5), reflecting the ability for larger, mature trees to buffer stress and continue transpiration despite water scarcity (Steppe et al., 2011). Future studies examining differences in drought response using juvenile trees should account for the deeper water access in mature individuals if attempting to scale to whole forests.
The difference in seasonal patterns of leaf water potential was most notable between juvenile and mature F. grandifolia trees. In fact, juvenile F. grandifolia trees were the only trees to have significantly lower water potentials by September than mature individuals of the same species. These results indicate that data collected from juveniles of F. grandifolia, C. ovata, and P. occidentalis may not accurately represent the physiological responses of mature trees, especially in the case of F. grandifolia. The combination of small and carbon-cheap, but abundant, leaves with diffuse-porous wood anatomy, thin bark, and a slow growth rate make for complex carbon allocation patterns which may have unusual consequences for physiological responses during water stress. These data have specific impacts for experimentalists who are seeking to understand physiological responses to stressors (Mackay et al., 2015) or define hydraulic-based mortality thresholds.
Many Earth system models are parameterized with hydraulic variables (e.g., minimum stomatal conductance and percent loss of conductivity values), and recent experimental studies have called for the inclusion of water potentials or other hydraulic-based traits as threshold parameters for mortality-prediction models (Mackay et al., 2015; Adams et al., 2017). While hydraulic-based thresholds are useful, hydraulic parameters are changing in response to carbon requirements and the capacity of the tree to meet those requirements. Therefore, integrative traits that reflect the capacity for carbon uptake are valuable for accurately predicting how trees will respond to environmental stressors (Marshall et al., 2001; Walker et al., 2017; Wang et al., 2019). Using integrative photosynthetic parameters such as Fv’/Fm’, PhiPS2, ci/ca, etc., is more informative and accurate for comparing physiological responses to stress (Brodribb and Feild, 2000; Prentice et al., 2014; Tan et al., 2017) than typical survey measurements (Amax, gs, etc.), because integrative parameters reflect differences in specific environmental pressures. Additionally, many of these measurements are already embedded into Earth-system models, allowing for better continuity in scaling of field-based results to forest-level models.
Our data also demonstrate that specific leaf area (SLA) differed among months and species, as well as between age classes. In the juvenile trees, specific leaf area generally decreased as the season progressed (Figure 4); however, in the mature trees, specific leaf area increased and then remained the same or decreased, depending on species. While SLA is allowed to vary among plant functional types (including species) in the Community Earth System Model (Fisher et al., 2015), there is currently no allowable variability depending on tree age. Because of the importance of SLA to ecosystem-level productivity (White et al., 2000), we emphasize the importance of including age-related variability in models. Although the ability of this parameter to represent drought is unresolved (Schwartz et al., 2020), we know that SLA is indirectly related to hydraulic vulnerability (Greenwood et al., 2017; O’Brien et al., 2017).
Our results highlight the fact that hydraulic response during water stress reflects a balance between the carbon requirements of the tree and the capacity to meet those requirements. In species-rich forests, variations in wood (Chave et al., 2009) and leaf allometry (Wright et al., 2004) coupled with individual life-history traits complicate these relationships (Poorter et al., 2009; Johnson et al., 2018a). As trees grow, that balance changes because large trees have stored carbon that buffers their carbon requirements during environmental stress (Stephenson et al., 2014). However, younger trees are limited in their stress tolerance (Kolb et al., 1997) because of higher growth requirements and a reduced capacity to meet those needs (Ryan and Yoder, 1997; Wright et al., 2004; Woodruff and Meinzer, 2011). Our results demonstrate that physiological parameters differed during summer water stress between juvenile and adult trees for certain species. We highlight the importance of allowing variability for both species and life stage when predicting how environmental stressors may influence forest carbon (Day et al., 2001; Irvine et al., 2004; Steppe et al., 2011). Finally, we emphasize that measuring integrative traits representative of the capacity for carbon uptake yields critical insights about seasonal changes in carbon and water use efficiency (Tan et al., 2017; Wang et al., 2019; Lu et al., 2022). Reporting these metrics in stress-response studies will not only improve the accuracy of carbon and mortality models (Mackay et al., 2015), but will also bridge the divide between experimental studies and modeling efforts.
The raw data supporting the conclusions of this article will be made available by the authors, without undue reservation.
KB and DR designed the study. KB, DR, and BF collected the data. KB performed the statistical analyses with input from DR, BF, and TH. KB wrote the manuscript with input from BF, TH, and DR. All authors revised the manuscript.
The authors declare that the research was conducted in the absence of any commercial or financial relationships that could be construed as a potential conflict of interest.
All claims expressed in this article are solely those of the authors and do not necessarily represent those of their affiliated organizations, or those of the publisher, the editors and the reviewers. Any product that may be evaluated in this article, or claim that may be made by its manufacturer, is not guaranteed or endorsed by the publisher.
The Supplementary Material for this article can be found online at: https://www.frontiersin.org/articles/10.3389/ffgc.2022.1018789/full#supplementary-material
Adams, H. D., Zeppel, M. J. B., Anderegg, W. R. L., Hartmann, H., Landhausser, S. M., Tissue, D. T., et al. (2017). A multi-species synthesis of physiological mechanisms in drought-induced tree mortality. Nat. Ecol. Evol. 1, 1285–1291. doi: 10.1038/s41559-017-0248-x
Baas, P., Ewers, F. W., Davis, S. D., and Wheeler, E. A. (2004). “Evolution of xylem physiology,” in The evolution of plant physiology, eds I. Poole and A. Hemsley (Amsterdam: Elsevier), 273–295. doi: 10.1016/B978-012339552-8/50016-0
Babst, F., Friend, A. D., Karamihalaki, M., Wei, J., von Arx, G., Papale, D., et al. (2021). Modeling ambitions outpace observations of forest carbon allocation. Trends Plant Sci. 26, 210–219. doi: 10.1016/j.tplants.2020.10.002
Barbaroux, C., and Bréda, N. (2002). Contrasting distribution and seasonal dynamics of carbohydrate reserves in stem wood of adult ring-porous sessile oak and diffuse-porous beech trees. Tree Physiol. 22, 1201–1210. doi: 10.1093/treephys/22.17.1201
Bassow, S. L., and Bazzaz, F. A. (1997). Intra- and inter-specific variation in canopy photosynthesis in a mixed deciduous forest. Oecologia 109, 507–515. doi: 10.1007/s004420050111
Bates, D., Mächler, M., Bolker, B. M., and Walker, S. C. (2015). Fitting linear mixed-effects models using lme4. J. Stat. Softw. 67, 1–48. doi: 10.18637/jss.v067.i01
Bond, B. J. (2000). Age-related changes in photosynthesis of woody plants. Trends Plant Sci. 5, 349–353. doi: 10.1016/S1360-1385(00)01691-5
Bond, B. J., and Kavanagh, K. L. (1999). Stomatal behavior of four woody species in relation to leaf-specific hydraulic conductance and threshold water potential. Tree Physiol. 19, 503–510. doi: 10.1093/treephys/19.8.503
Braun, S., Schindler, C., and Leuzinger, S. (2010). Use of sap flow measurements to validate stomatal functions for mature beech (Fagus sylvatica) in view of ozone uptake calculations. Environ. Pollut. 158, 2954–2963. doi: 10.1016/j.envpol.2010.05.028
Brinkmann, N., Eugster, W., Zweifel, R., Buchmann, N., and Kahmen, A. (2016). Temperate tree species show identical response in tree water deficit but different sensitivities in sap flow to summer soil drying. Tree Physiol. 36, 1508–1519. doi: 10.1093/treephys/tpw062
Brodribb, T. (1996). Dynamics of changing intercellular CO2 concentration (ci) during drought and determination of minimum functional Ci. Plant Physiol. 111, 179–185. doi: 10.1104/pp.111.1.179
Brodribb, T. J., and Feild, T. S. (2000). Stem hydraulic supply is linked to leaf photosynthetic capacity: Evidence from New Caledonian and Tasmanian rainforests. Plant Cell Environ. 23, 1381–1388. doi: 10.1046/j.1365-3040.2000.00647.x
Bryant, K. N., Fredericksen, B. W., and Rosenthal, D. M. (2022). Ring- and diffuse-porous species exhibit a spectrum of hydraulic behaviors from isohydry to anisohydry in a temperate deciduous forest. Trees 36, 485–495. doi: 10.1007/s00468-021-02223-7
Catovsky, S., Holbrook, N. M., and Bazzaz, F. A. (2002). Coupling whole-tree transpiration and canopy photosynthesis in coniferous and broad-leaved tree species. Can. J. For. Res. 32, 295–309. doi: 10.1139/x01-199
Chang, S. X., and Robison, D. J. (2003). Nondestructive and rapid estimation of hardwood foliar nitrogen status using the SPAD-502 chlorophyll meter. For. Ecol. Manage. 181, 331–338. doi: 10.1016/S0378-1127(03)00004-5
Chave, J., Coomes, D., Jansen, S., Lewis, S. L., Swenson, N. G., and Zanne, A. E. (2009). Towards a worldwide wood economics spectrum. Ecol. Lett. 12, 351–366. doi: 10.1111/j.1461-0248.2009.01285.x
Comparini, D., Masi, E., Pandolfi, C., Sabbatini, L., Dolfi, M., Morosi, S., et al. (2020). Stem electrical properties associated with water stress conditions in olive tree. Agric. Water Manag. 234:106109. doi: 10.1016/j.agwat.2020.106109
Cunningham, S. C. (2004). Stomatal sensitivity to vapour pressure deficit of temperate and tropical evergreen rainforest trees of Australia. Trees 18, 399–407. doi: 10.1007/s00468-004-0318-y
Daly, C., Taylor, G. H., Gibson, W. P., Parzybok, T. W., Johnson, G. L., and Pasteris, P. A. (2000). High-quality spatial climate data sets for the United States and beyond. Trans. ASAE 43, 1957–1962. doi: 10.13031/2013.3101
Davis, T. W., Kuo, C. M., Liang, X., and Yu, P. S. (2012). Sap flow sensors: Construction, quality control and comparison. Sensors 12, 954–971. doi: 10.3390/s120100954
Day, M. E., Greenwood, M. S., and White, A. S. (2001). Age-related changes in foliar morphology and physiology in red spruce and their influence on declining photosynthetic rates and productivity with tree age. Tree Physiol. 21, 1195–1204. doi: 10.1093/treephys/21.16.1195
DeForest, J. L. (2009). The influence of time, storage temperature, and substrate age on potential soil enzyme activity in acidic forest soils using MUB-linked substrates and l-DOPA. Soil Biol. Biochem. 41, 1180–1186. doi: 10.1016/j.soilbio.2009.02.029
Domec, J. C., Warren, J. M., Meinzer, F. C., Brooks, J. R., and Coulombe, R. (2004). Native root xylem embolism and stomatal closure in stands of Douglas-fir and ponderosa pine: Mitigation by hydraulic redistribution. Oecologia 141, 7–16. doi: 10.1007/s00442-004-1621-4
D’Orangeville, L., Maxwell, J., Kneeshaw, D., Pederson, N., Duchesne, L., Logan, T., et al. (2018). Drought timing and local climate determine the sensitivity of eastern temperate forests to drought. Glob. Chang. Biol. 24, 2339–2351. doi: 10.1111/gcb.14096
Fisher, R. A., Muszala, S., Verteinstein, M., Lawrence, P., Xu, C., McDowell, N. G., et al. (2015). Taking off the training wheels: The properties of a dynamic vegetation model without climate envelopes, CLM4.5(ED). Geosci. Model. Dev. 8, 3593–3619. doi: 10.5194/gmd-8-3593-2015
Ford, C. R., Goranson, C. E., Mitchell, R. J., Will, R. E., and Teskey, R. O. (2004). Diurnal and seasonal variability in the radial distribution of sap flow: Predicting total stem flow in Pinus taeda trees. Tree Physiol. 24, 941–950. doi: 10.1093/treephys/24.9.951
Fredericksen, T. S., Steiner, K. C., Skelly, J. M., Joyce, B. J., Kolb, T. E., Kouterick, K. B., et al. (1996). Diel and seasonal patterns of leaf gas exchange and xylem water potentials of different-sized Prunus serotina Ehrh. Trees. For. Sci. 42, 359–365.
Fu, X., and Meinzer, F. C. (2018). Metrics and proxies for stringency of regulation of plant water status (iso/anisohydry): A global data set reveals coordination and trade-offs among water transport traits. Tree Physiol. 39, 122–134. doi: 10.1093/treephys/tpy087
Fu, X., Meinzer, F. C., Woodruff, D. R., Liu, Y. Y., Smith, D. D., McCulloh, K. A., et al. (2019). Coordination and trade-offs between leaf and stem hydraulic traits and stomatal regulation along a spectrum of isohydry to anisohydry. Plant Cell Environ. 42, 2245–2258. doi: 10.1111/pce.13543
Gebauer, T., Horna, V., and Leuschner, C. (2008). Variability in radial sap flux density patterns and sapwood area among seven co-occurring temperate broad-leaved tree species. Tree Physiol. 28, 1821–1830. doi: 10.1093/treephys/28.12.1821
Genet, H., Bréda, N., and Dufrêne, E. (2009). Age-related variation in carbon allocation at tree and stand scales in beech (Fagus sylvatica L.) and sessile oak (Quercus petraea (Matt.) Liebl.) using a chronosequence approach. Tree Physiol. 30, 177–192. doi: 10.1093/treephys/tpp105
Granier, A. (1985). Une nouvelle methode pour la mesure du flux de xeve brute dans le tronc. Ann. Sci. For. 42, 193–200. doi: 10.1051/forest:19850204
Granier, A. (1987). Evaluation of transpiration in a Douglas-fir stand by means of sap flow measurements. Tree Physiol. 3, 309–320. doi: 10.1093/treephys/3.4.309
Greenwood, S., Ruiz-Benito, P., Martínez-Vilalta, J., Lloret, F., Kitzberger, T., Allen, C. D., et al. (2017). Tree mortality across biomes is promoted by drought intensity, lower wood density and higher specific leaf area. Ecol. Lett. 20:539553. doi: 10.1111/ele.12748
Grossiord, C., Sevanto, S., Borrego, I., Chan, A. M., Collins, A. D., Dickman, L. T., et al. (2017). Tree water dynamics in a drying and warming world. Plant Cell Environ. 40, 1861–1873. doi: 10.1111/pce.12991
Hartmann, H., Adams, H. D., Hammond, W. M., Hoch, G., Landhäusser, S. M., Wiley, E., et al. (2018). Identifying differences in carbohydrate dynamics of seedlings and mature trees to improve carbon allocation in models for trees and forests. Environ. Exp. Bot. 152, 7–18. doi: 10.1016/j.envexpbot.2018.03.011
Hartmann, H., and Trumbore, S. (2016). Understanding the roles of nonstructural carbohydrates in forest trees – from what we can measure to what we want to know. New Phytol. 211, 386–403. doi: 10.1111/nph.13955
Hoch, G., Richter, A., and Körner, C. (2003). Non-structural carbon compounds in temperate forest trees. Plant Cell Environ. 26, 1067–1081. doi: 10.1046/j.0016-8025.2003.01032.x
Hochberg, U., Rockwell, F. E., Holbrook, N. M., and Cochard, H. (2018). Iso/Anisohydry: A plant–environment interaction rather than a simple hydraulic trait. Trends Plant Sci. 23, 112–120. doi: 10.1016/j.tplants.2017.11.002
Hoffmann, W. A., Marchin, R. M., Abit, P., and Lau, O. L. (2011). Hydraulic failure and tree dieback are associated with high wood density in a temperate forest under extreme drought. Glob. Chang. Biol. 17, 2731–2742. doi: 10.1111/j.1365-2486.2011.02401.x
Hölscher, D. (2004). Leaf traits and photosynthetic parameters of saplings and adult trees of co-existing species in a temperate broad-leaved forest. Basic Appl. Ecol. 5, 163–172. doi: 10.1078/1439-1791-00218
Hudiburg, T. W., Law, B. E., and Thornton, P. E. (2013). Evaluation and improvement of the Community Land Model (CLM4) in Oregon forests. Biogeosciences 10, 453–470. doi: 10.5194/bg-10-453-2013
Huntington, J. L., Hegewisch, K. C., Daudert, B., Morton, C. G., Abatzoglou, J. T., McEvoy, D. J., et al. (2017). Climate engine: Cloud computing and visualization of climate and remote sensing data for advanced natural resource monitoring and process understanding. Bull. Am. Meteorol. Soc. 98, 2397–2409. doi: 10.1175/BAMS-D-15-00324.1
Irvine, J., Law, B. E., Anthoni, P. M., and Meinzer, F. C. (2002). Water limitations to carbon exchange in old-growth and young ponderosa pine stands. Tree Physiol. 22, 189–196. doi: 10.1093/treephys/22.2-3.189
Irvine, J., Law, B. E., Kurpius, M. R., Anthoni, P. M., Moore, D., and Schwarz, P. A. (2004). Age-related changes in ecosystem structure and function and effects on water and carbon exchange in ponderosa pine. Tree Physiol. 24, 753–763. doi: 10.1093/treephys/24.7.753
Jacobsen, A. L., Brandon Pratt, R., Tobin, M. F., Hacke, U. G., and Ewers, F. W. (2012). A global analysis of xylem vessel length in woody plants. Am. J. Bot. 99, 1583–1591. doi: 10.3732/ajb.1200140
Johnson, D. M., Berry, Z. C., Baker, K. V., Smith, D. D., McCulloh, K. A., and Domec, J. C. (2018a). Leaf hydraulic parameters are more plastic in species that experience a wider range of leaf water potentials. Funct. Ecol. 32, 894–903. doi: 10.1111/1365-2435.13049
Johnson, D. M., Domec, J. C., Carter Berry, Z., Schwantes, A. M., McCulloh, K. A., Woodruff, D. R., et al. (2018b). Co-occurring woody species have diverse hydraulic strategies and mortality rates during an extreme drought. Plant Cell Environ. 41, 576–588. doi: 10.1111/pce.13121
Kannenberg, S. A., Novick, K. A., and Phillips, R. P. (2019). Anisohydric behavior linked to persistent hydraulic damage and delayed drought recovery across seven North American tree species. New Phytol. 222, 1862–1872. doi: 10.1111/nph.15699
Klein, T. (2014). The variability of stomatal sensitivity to leaf water potential across tree species indicates a continuum between isohydric and anisohydric behaviours. Funct. Ecol. 28, 1313–1320. doi: 10.1111/1365-2435.12289
Kolb, T. E., Fredericksen, T. S., Steiner, K. C., and Skelly, J. M. (1997). Issues in scaling tree size and age responses to ozone: A review. Environ. Pollut. 98, 195–208. doi: 10.1016/S0269-7491(97)00132-2
Kolb, T. E., and Stone, J. E. (2000). Differences in leaf gas exchange and water relations among species and tree seizes in an Arizona pine-oak forest. Tree Physiol. 20, 1–12. doi: 10.1093/treephys/20.1.1
Kwon, H., Law, B. E., Thomas, C. K., and Johnson, B. G. (2018). The influence of hydrological variability on inherent water use efficiency in forests of contrasting composition, age, and precipitation regimes in the Pacific Northwest. Agric. For. Meteorol. 249, 488–500. doi: 10.1016/j.agrformet.2017.08.006
Lechowicz, M. J. (1984). Why do temperate deciduous trees leaf out at different times? Adaptation and ecology of forest communities. Am. Nat. 124, 821–842. doi: 10.1086/284319
Loewenstein, N. J., and Pallardy, S. G. (1998). Drought tolerance, xylem sap abscisic acid and stomatal conductance during soil drying: A comparison of young plants of four temperate deciduous angiosperms. Tree Physiol. 18, 421–430. doi: 10.1093/treephys/18.7.421
Looker, N., Martin, J., Hoylman, Z., Jencso, K., and Hu, J. (2018). Diurnal and seasonal coupling of conifer sap flow and vapour pressure deficit across topoclimatic gradients in a subalpine catchment. Ecohydrology 11:e1994. doi: 10.1002/eco.1994
Lu, K., Chen, N., Zhang, X., Wang, J., Wang, M., Khan, S., et al. (2019). Increased drought and atmospheric CO2 positively impact intrinsic water use efficiency but do not promote tree growth in semi-arid areas of Northwestern China. Trees 33, 669–679. doi: 10.1007/s00468-018-1807-8
Lu, P., Urban, L., and Zhao, P. (2004). Granier’s thermal dissipation probe (TDP) method for measuring sap flow in trees: Theory and practice. Acta Bot. Sin. 46, 631–646.
Lu, X., Croft, H., Chen, J. M., Luo, Y., and Ju, W. (2022). Estimating photosynthetic capacity from optimized Rubisco-chlorophyll relationships among vegetation types and under global change. Environ. Res. Lett. 17:014028. doi: 10.1088/1748-9326/ac444d
Mackay, D. S., Roberts, D. E., Ewers, B. E., Sperry, J. S., McDowell, N. G., and Pockman, W. T. (2015). Interdependence of chronic hydraulic dysfunction and canopy processes can improve integrated models of tree response to drought. Water Resour. Res. 51, 6156–6176. doi: 10.1002/2015WR017244
Marshall, J. D., Rehfeldt, G. E., and Monserud, R. A. (2001). Family differences in height growth and photosynthetic traits in three conifers. Tree Physiol. 21, 727–734. doi: 10.1093/treephys/21.11.727
Martínez-Vilalta, J., and Garcia-Forner, N. (2017). Water potential regulation, stomatal behaviour and hydraulic transport under drought: Deconstructing the iso/anisohydric concept. Plant Cell Environ. 40, 962–976. doi: 10.1111/pce.12846
Martínez-Vilalta, J., Mangirón, M., Ogaya, R., Sauret, M., Serrano, L., Peñuelas, J., et al. (2003). Sap flow of three co-occurring Mediterranean woody species under varying atmospheric and soil water conditions. Tree Physiol. 23, 747–758. doi: 10.1093/treephys/23.11.747
Maxwell, K., and Johnson, G. N. (2000). Chlorophyll fluorescence – A practical guide. J. Exp. Bot. 51, 659–668. doi: 10.1093/jexbot/51.345.659
Meekins, J. F., and McCarthy, B. C. (2001). Effect of environmental variation on the invasive success of a nonindigenous forest herb. Ecol. Appl. 11, 1336–1348. doi: 10.1890/1051-0761(2001)011[1336:EOEVOT]2.0.CO;2
Milla, R., Reich, P. B., Niinemets, Ü., and Castro-Díez, P. (2008). Environmental and developmental controls on specific leaf area are little modified by leaf allometry. Funct. Ecol. 22, 565–576. doi: 10.1111/j.1365-2435.2008.01406.x
Moon, M., Richardson, A. D., O’Keefe, J., and Friedl, M. A. (2022). Senescence in temperate broadleaf trees exhibits species-specific dependence on photoperiod versus thermal forcing. Agric. For. Meteorol. 322:109026. doi: 10.1016/j.agrformet.2022.109026
Muraoka, H., Noda, H. M., Nagai, S., Motohka, T., Saitoh, T. M., Nasahara, K. N., et al. (2013). Spectral vegetation indices as the indicator of canopy photosynthetic productivity in a deciduous broadleaf forest. J. Plant Ecol. 6, 393–407. doi: 10.1093/jpe/rts037
Murchie, E. H., and Lawson, T. (2013). Chlorophyll fluorescence analysis: A guide to good practice and understanding some new applications. J. Exp. Bot. 64, 3983–3998. doi: 10.1093/jxb/ert208
Nippert, J. B., Duursma, R. A., and Marshall, J. D. (2004). Seasonal variation in photosynthetic capacity of montane conifers. Funct. Ecol. 18, 876–886. doi: 10.1111/j.0269-8463.2004.00909.x
Noda, H. M., Muraoka, H., Nasahara, K. N., Saigusa, N., Murayama, S., and Koizumi, H. (2015). Phenology of leaf morphological, photosynthetic, and nitrogen use characteristics of canopy trees in a cool-temperate deciduous broadleaf forest at Takayama, central Japan. Ecol. Res. 30, 247–266. doi: 10.1007/s11284-014-1222-6
O’Brien, M. J., Engelbrecht, B. M. J., Joswig, J., Pereyra, G., Schuldt, B., Jansen, S., et al. (2017). A synthesis of tree functional traits related to drought-induced mortality in forests across climatic zones. J. Appl. Ecol. 54, 1669–1686. doi: 10.1111/1365-2664.12874
Oren, R., and Pataki, D. E. (2001). Transpiration in response to variation in microclimate and soil moisture in Southeastern deciduous forests. Oecologia 127, 549–559. doi: 10.1007/s004420000622
Panek, J. A., and Goldstein, A. H. (2001). Response of stomatal conductance to drought in ponderosa pine: Implications for carbon and ozone uptake. Tree Physiol. 21, 337–344. doi: 10.1093/treephys/21.5.337
Piper, F. I. (2011). Drought induces opposite changes in the concentration of non-structural carbohydrates of two evergreen Nothofagus species of differential drought resistance. Ann. For. Sci. 68, 415–424. doi: 10.1007/s13595-011-0030-1
Poorter, H., Niinemets, Ü., Poorter, L., Wright, I. J., and Villar, R. (2009). Causes and consequences of variation in leaf mass per area (LMA): A meta-analysis. New Phytol. 182, 565–588. doi: 10.1111/j.1469-8137.2009.02830.x
Prentice, I. C., Dong, N., Gleason, S. M., Maire, V., and Wright, I. J. (2014). Balancing the costs of carbon gain and water transport: Testing a new theoretical framework for plant functional ecology. Ecol. Lett. 17, 82–91. doi: 10.1111/ele.12211
Ratzmann, G., Meinzer, F. C., and Tietjen, B. (2019). Iso/Anisohydry: Still a useful concept. Trends Plant Sci. 24, 191–194. doi: 10.1016/j.tplants.2019.01.001
Reich, P. B., Ellsworth, D. S., and Walters, M. B. (1998). Leaf structure (specific leaf area) modulates photosynthesis-nitrogen relations: Evidence from within and across species and functional groups. Funct. Ecol. 12, 948–958. doi: 10.1046/j.1365-2435.1998.00274.x
Ripullone, F., Lauteri, M., Grassi, G., Amato, M., and Borghetti, M. (2004). Variation in nitrogen supply changes water-use efficiency of Pseudotsuga menziesii and Populus × euroamericana; a comparison of three approaches to determine water-use efficiency. Tree Physiol. 24, 671–679. doi: 10.1093/treephys/24.6.671
Ryan, M. G., and Yoder, B. J. (1997). Hydraulic limits to tree height and tree growth: What keeps trees from growing beyond a certain height? Bioscience 47, 235–242. doi: 10.2307/1313077
Saurer, M., Spahni, R., Frank, D. C., Joos, F., Leuenberger, M., Loader, N. J., et al. (2014). Spatial variability and temporal trends in water-use efficiency of European forests. Glob. Chang. Biol. 20, 3700–3712. doi: 10.1111/gcb.12717
Savi, T., Casolo, V., Luglio, J., Bertuzzi, S., Trifilo’, P., lo Gullo, M. A., et al. (2016). Species-specific reversal of stem xylem embolism after a prolonged drought correlates to endpoint concentration of soluble sugars. Plant Physiol. Biochem. 106, 198–207. doi: 10.1016/j.plaphy.2016.04.051
Scholander, P. F., Hammel, H. T., Hemmingsen, E. A., and Bradstreet, E. D. (1964). Hydrostatic pressure and osmotic potential in leaves of mangroves and some other plants. Proc. Natl. Acad. Sci. U.S.A. 52, 119–125. doi: 10.1073/pnas.52.1.119
Schulze, E.-D. (1994). Ecophysiology of photosynthesis. Berlin: Springer. doi: 10.1007/978-3-642-79354-7
Schwartz, N. B., Feng, X., Muscarella, R., Swenson, N. G., Umaña, M. N., Zimmerman, J. K., et al. (2020). Topography and traits modulate tree performance and drought response in a tropical forest. Front. For. Glob. Change 3:596256. doi: 10.3389/ffgc.2020.596256
Smith, A. M., and Stitt, M. (2007). Coordination of carbon supply and plant growth. Plant Cell Environ. 30, 1126–1149. doi: 10.1111/j.1365-3040.2007.01708.x
Stephenson, N. L., Das, A. J., Condit, R., Russo, S. E., Baker, P. J., Beckman, N. G., et al. (2014). Rate of tree carbon accumulation increases continuously with tree size. Nature 507, 90–93. doi: 10.1038/nature12914
Steppe, K., Niinemets, Ü., and Teskey, R. O. (2011). “Tree size- and age-related changes in leaf physiology and their influence on carbon gain,” in Size- and age-related changes in tree structure and function tree physiology, Vol. 4, eds F. Meinzer, B. Lachenbruch, and T. Dawson (Berlin: Springer Science + Business Media B.V), 235–253. doi: 10.1007/978-94-007-1242-3_9
Steppe, K., Vandegehuchte, M. W., Tognetti, R., and Mencuccini, M. (2015). Sap flow as a key trait in the understanding of plant hydraulic functioning. Tree Physiol. 35, 341–345. doi: 10.1093/treephys/tpv033
Stone, E. L., and Kalisz, P. J. (1991). On the maximum extent of tree roots. For. Ecol. Manage. 46, 59–102. doi: 10.1016/0378-1127(91)90245-Q
Tai, X., Mackay, D. S., Anderegg, W. R. L., Sperry, J. S., and Brooks, P. D. (2017). Plant hydraulics improves and topography mediates prediction of aspen mortality in Southwestern USA. New Phytol. 213, 113–127. doi: 10.1111/nph.14098
Tan, Z. H., Wu, Z. X., Hughes, A. C., Schaefer, D., Zeng, J., Lan, G. Y., et al. (2017). On the ratio of intercellular to ambient CO2 (ci/ca) derived from ecosystem flux. Int. J. Biometeorol. 61, 2059–2071. doi: 10.1007/s00484-017-1403-4
Tardieu, F., and Simonneau, T. (1998). Variability among species of stomatal control under fluctuating soil water status and evaporative demand: Modelling isohydric and anisohydric behaviours. J. Exp. Bot. 49, 419–432. doi: 10.1093/jxb/49.Special_Issue.419
Tomasella, M., Petrussa, E., Petruzzellis, F., Nardini, A., and Casolo, V. (2020). The possible role of non-structural carbohydrates in the regulation of tree hydraulics. Int. J. Mol. Sci. 21:144. doi: 10.3390/ijms21010144
Trifilò, P., Kiorapostolou, N., Petruzzellis, F., Vitti, S., Petit, G., lo Gullo, M. A., et al. (2019). Hydraulic recovery from xylem embolism in excised branches of twelve woody species: Relationships with parenchyma cells and non-structural carbohydrates. Plant Physiol. Biochem. 139, 513–520. doi: 10.1016/j.plaphy.2019.04.013
Turner, N. C. (1988). Measurement of plant water status by the pressure chamber technique. Irrig. Sci. 9, 289–308. doi: 10.1007/BF00296704
Walker, A. P., Quaife, T., van Bodegom, P. M., de Kauwe, M. G., Keenan, T. F., Joiner, J., et al. (2017). The impact of alternative trait-scaling hypotheses for the maximum photosynthetic carboxylation rate (VCmax) on global gross primary production. New Phytol. 215, 1370–1386. doi: 10.1111/nph.14623
Wang, R., Chen, J. M., Luo, X., Black, A., and Arain, A. (2019). Seasonality of leaf area index and photosynthetic capacity for better estimation of carbon and water fluxes in evergreen conifer forests. Agric. For. Meteorol. 279:107708. doi: 10.1016/j.agrformet.2019.107708
Wheeler, E. A., Baas, P., and Rodgers, S. (2007). Variations in dicot wood anatomy: A global analysis based on the InsideWood database. IAWA J. 28, 229–258. doi: 10.1163/22941932-90001638
White, M. A., Thornton, P. E., Running, S. W., and Nemani, R. R. (2000). Parameterization and sensitivity analysis of the BIOME–BGC terrestrial ecosystem model: Net primary production controls. Earth Interact. 4, 1–85. doi: 10.1175/1087-3562(2000)004<0003:PASAOT>2.0.CO;2
Wilson, P. J., Thompson, K., and Hodgson, J. G. (1999). Specific leaf area and leaf dry matter content as alternative predictors of plant strategies. New Phytol. 143, 155–162. doi: 10.1046/j.1469-8137.1999.00427.x
Woodruff, D. R., and Meinzer, F. C. (2011). “Size-dependent changes in biophysical control of tree growth: The role of turgor,” in Size- and age-related changes in tree structure and function tree physiology, Vol. 4, eds F. Meinzer, B. Lachenbruch, and T. Dawson (Berlin: Springer Science + Business Media B.V), 363–384. doi: 10.1007/978-94-007-1242-3_14
Wright, I. J., Reich, P. B., Westoby, M., Ackerly, D. D., Baruch, Z., Bongers, F., et al. (2004). The worldwide leaf economics spectrum. Nature 428, 821–827. doi: 10.1038/nature02403
Yoshimura, K., Saiki, S.-T., Yazaki, K., Ogasa, M. Y., Shirai, M., Nakano, T., et al. (2016). The dynamics of carbon stored in xylem sapwood to drought-induced hydraulic stress in mature trees. Sci. Rep. 6:24513. doi: 10.1038/srep24513
Zhang, T., Niinemets, Ü., Sheffield, J., and Lichstein, J. W. (2018). Shifts in tree functional composition amplify the response of forest biomass to climate. Nature 556, 99–102. doi: 10.1038/nature26152
Keywords: gas exchange, canopy measurements, specific leaf area, temperate forest, tree physiology
Citation: Bryant K, Fredericksen B, Hudiburg T and Rosenthal D (2023) Physiological strategies for handling summer water stress differ among co-existing species and between juvenile and mature trees. Front. For. Glob. Change 5:1018789. doi: 10.3389/ffgc.2022.1018789
Received: 13 August 2022; Accepted: 06 December 2022;
Published: 04 January 2023.
Edited by:
Alana R. O. Chin, ETH Zürich, SwitzerlandReviewed by:
Thomas Kolb, Northern Arizona University, United StatesCopyright © 2023 Bryant, Fredericksen, Hudiburg and Rosenthal. This is an open-access article distributed under the terms of the Creative Commons Attribution License (CC BY). The use, distribution or reproduction in other forums is permitted, provided the original author(s) and the copyright owner(s) are credited and that the original publication in this journal is cited, in accordance with accepted academic practice. No use, distribution or reproduction is permitted which does not comply with these terms.
*Correspondence: Kelsey Bryant, ✉ a2Vsc2V5YnJ5YW50QHVpZGFoby5lZHU=
Disclaimer: All claims expressed in this article are solely those of the authors and do not necessarily represent those of their affiliated organizations, or those of the publisher, the editors and the reviewers. Any product that may be evaluated in this article or claim that may be made by its manufacturer is not guaranteed or endorsed by the publisher.
Research integrity at Frontiers
Learn more about the work of our research integrity team to safeguard the quality of each article we publish.