- 1Japan International Research Center for Agricultural Sciences, Tsukuba, Japan
- 2Forest Department Sarawak, Kuching, Malaysia
- 3Faculty of Agriculture and Marine Science, Kochi University, Nankoku, Japan
Although leaf toughness is an essential plant adaptation to herbivore pressure and environmental stress, the relationships of leaf toughness with leaf anatomy and photosynthetic traits, and its spatial variations within tropical rainforests, remain poorly understood. We measured these traits in 103 tree species belonging to 27 families from the canopy to understory using a canopy crane system in a tropical rainforest in Sarawak, Malaysia. We focused on the leaf anatomical trait of bundle-sheath extensions (BSEs) around the vascular bundle due to their diverse ecophysiological functions. We divided the trees into heterobaric species with BSEs and homobaric species lacking BSEs, to investigate the relationships of leaf toughness with tree height, leaf functional traits such as carbon (C) and nitrogen (N) content, thickness, leaf mass per area (LMA) and the maximum photosynthetic rate (Pmax). Leaf toughness, LMA, thickness and C and N contents increased with height regardless of BSE presence. Heterobaric leaves had greater toughness than homobaric leaves, whereas leaf thickness, LMA and C were similar between the two leaf types throughout the height gradient. We found that standardized toughness per thickness or C was greater in heterobaric species, as BSEs consist mainly of fibrous tissue. Pmax was higher for heterobaric than homobaric leaves in the upper canopy presumably due to the functions of BSEs, including water conductivity, but did not differ with plant type in the lower layers. In other words, heterobaric species efficiently exploit the advantages of tougher leaves and higher Pmax by having BSEs. The increased proportion of heterobaric species, with their tougher leaves and higher Pmax, in the upper canopy is consistent with adaptation to physically stressful conditions in the tropical rainforest canopy, including high herbivore pressure and strong light.
Introduction
Leaf toughness is a key functional trait in plant adaptation to herbivore pressures and physically stressful environmental factors, such as strong wind (Lucas et al., 2000; Onoda et al., 2011). In addition, leaf toughness is related to forest ecosystem function through the decomposition of leaf litter (Pérez-Harguindeguy et al., 2000; Li et al., 2009). Leaf toughness varies by plant form and climatic zone (Onoda et al., 2011), with variations of up to 820-fold between species being reported; this exceeds the variability in other traits such as leaf thickness and leaf mass per area (LMA; 40–50-fold; Onoda et al., 2011). This high variability has major implications for ecological processes and adaptation.
In tropical rainforests, where herbivore pressure is high, leaf toughness is important for maintaining photosynthetic production through protection of the leaves (Coley, 1983; Coley and Barone, 1996; Caldwell et al., 2016). Toughness may vary with spatial location in the forest, as environmental stresses and herbivore pressure vary with tree height. Variations in leaf toughness may be important for tree adaptation to different growth heights (Ribeiro and Basset, 2007; Thomas et al., 2010). Leaf traits such as thickness, LMA, nitrogen (N) content and photosynthetic traits vary with tree height in tropical rainforests, thus supporting acclimation to environmental changes (Kenzo et al., 2006, 2015; Cavaleri et al., 2010; Lloyd et al., 2010; Ichie et al., 2016). For example, increased LMA and reduced leaf size with increasing tree height increase tolerance to drought and high temperature in canopy environments with strong light, high temperature and low water potential (Thomas and Ickes, 1995; Turner, 2001). On the other hand, few studies have explored the variation of toughness across the height gradient in tropical rainforests, and those that have reported conflicting results: several researchers reported higher leaf toughness in canopy compared to juvenile trees in South American tropical rainforests (Dominy et al., 2003; Kitajima and Poorter, 2010), whereas some understory tree species had similar toughness to canopy tree species in Southeast Asia (Turner et al., 1993). Kitajima and Poorter (2010) reported that the relationships between leaf toughness and leaf traits persisted even when phylogenetic constraints were considered and thus the relationship between tree height and toughness might be a general trend regardless of phylogeny. In the forest canopy, leaf toughness may be a major contributor to plant protection due to the high herbivore pressure and physical stress compared with the forest understory (Kato et al., 1995; Turner, 2001; Novotny and Basset, 2005; Yoneyama and Ichie, 2019). Therefore, leaf toughness may change with height, although the pattern of change is unclear due to the difficulty of accessing canopy leaves in forests. In addition, due to the high species richness of tropical rainforests, large variations in leaf toughness among species within a forest are likely.
Although various leaf traits including thickness, LMA, and carbon (C) content are related to toughness, several studies have indicated that internal leaf structure may have the strongest impact on toughness (Lucas et al., 1991; Choong et al., 1992; Turner et al., 2000; Turner, 2001). Although leaf age also affects toughness, the effect stabilizes with maturity (Yoneyama and Ichie, 2019). A worldwide meta-analysis revealed that < 50% of leaf toughness is explained by LMA (mean = 34.3% ± 8.2% based on tear, punch, and shear tests; Onoda et al., 2011). Thus, other factors such as C content and anatomical structure may determine leaf toughness (Onoda et al., 2011). Among leaf anatomical structures, the presence or absence of bundle sheath extensions (BSEs) around leaf vascular bundles may be strongly associated with leaf toughness, as BSEs are comprised of parenchyma or sclerenchyma cells, and are very physically strong (Lucas et al., 1991; Turner, 2001). The leaves of broadleaf trees can be divided into heterobaric leaves containing BSEs and homobaric leaves without BSEs (Figure 1). Due to the absence of chloroplasts in the BSEs of heterobaric leaves, light penetrates the leaf and the vein network is typically clearly visible (Figures 1A,C), whereas it is not visible in homobaric leaves (Figures 1B,D). Moreover, BSEs have strong effects on leaf ecophysiological traits including the photosynthesis rate, N content and water use (Wylie, 1952; Terashima, 1992; Scoffoni et al., 2008; Inoue et al., 2015; Taneda et al., 2016). For example, the presence of BSEs leads to higher photosynthetic rates due to increased leaf water conductivity and light penetration into the leaf blade compared with homobaric leaves lacking BSEs (Karabourniotis et al., 2000, 2021; Nikolopoulos et al., 2002; Scoffoni et al., 2008; Liakoura et al., 2009; Sack and Scoffoni, 2013). On the other hand, greater cell wall thickness is necessary for leaf toughness. Cell walls that are thick and fibrous generally increase the leaf’s internal resistance to CO2 diffusion, leading to greater allocation of N for the construction of cell walls in the leaf, and thus a possible trade-off characterized by reduced photosynthesis (Hikosaka, 2004). The relationship between leaf toughness and photosynthetic ability is not well understood, and photosynthesis in heterobaric species may be less limited by leaf toughness due to the photosynthetically advantageous BSE functions of water conduction and light transmission into the leaf.
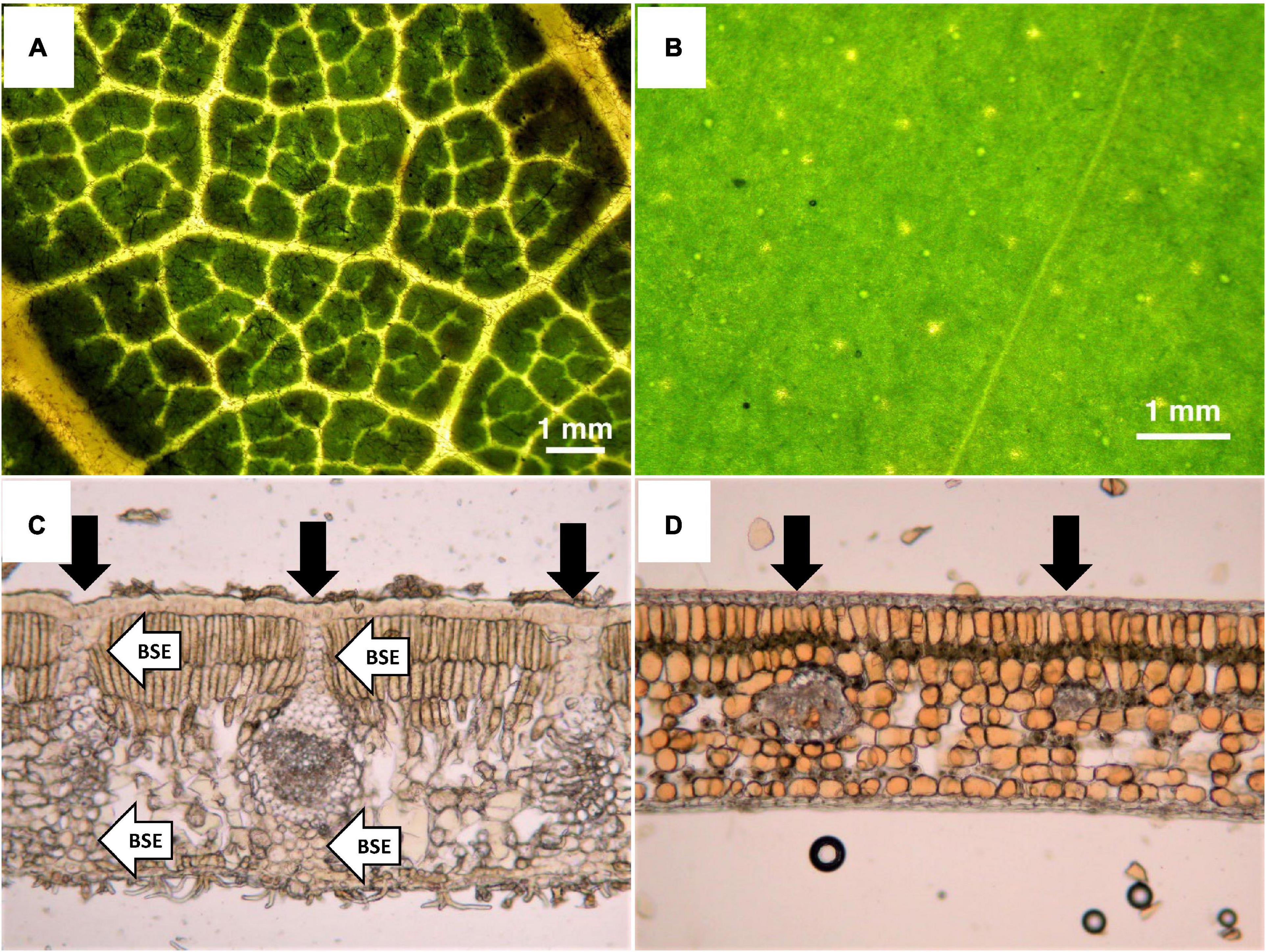
Figure 1. Photographs of leaf surfaces (upper panels) and transverse sections of leaves (lower panels). (A) Heterobaric leaf from the canopy (Dacryodes incurvata), (B) homobaric leaf from the subcanopy (Syzygium griffithii), (C) heterobaric leaf from the canopy (Lithocarpus luteus), and (D) homobaric leaf from the understory (Dimorphocalyx denticulatus). Black arrows in the lower panels indicate the positions of vascular bundles, and white arrows indicate BSEs. Due to the absence of chloroplasts in BSEs, the light penetrates the leaf, and thus the vein network is clearly visible in heterobaric leaves (A), but not in homobaric leaves (B).
The presence or absence of BSEs affects not only physiological functions but also the ecological distribution of plants in tropical rainforests. Specifically, there is clear evidence of niche segregation, with a higher proportion of homobaric species found among forest floor shrubs, and a higher proportion of heterobaric species in high-light environments, such as the canopy and canopy gaps (Kenzo et al., 2007; Boeger et al., 2016). In addition, leaf type does not vary ontogenetically within species (Wylie, 1952). As BSEs lack chloroplasts, the allocation of C to leaves may be greater in heterobaric than homobaric leaves. The presence of BSEs in leaves may be a key trait for predicting leaf toughness and photosynthetic traits, which remain poorly understood. In this study, we focused on heterobaric leaves with BSEs, and homobaric leaves lacking BSEs, to investigate the relationships of leaf toughness with tree height, other leaf traits such as LMA and thickness, and leaf photosynthetic function. We hypothesized that leaf toughness varies with the forest height, that heterobaric leaves have greater leaf toughness (according to C content) than homobaric leaves, and that BSEs may contribute to higher leaf toughness and photosynthesis levels in heterobaric leaves.
Materials and methods
Study site
This study was conducted at the Crane Plot (4 ha; 200 m × 200 m), which is located in a lowland tropical rainforest in Lambir Hills National Park, Sarawak, Malaysia (4°20′N, 113°50′E; 150–250 m a.s.l.). The canopy layer in the stand was approximately 30–40 m, and some emergent trees reached 50 m in height. An 85-m-tall canopy crane with a 75-m-long rotating jib was constructed in the center of this plot to provide three-dimensional access to the canopy from near the forest floor (Kenzo et al., 2015). The study site is in a humid tropical area with no distinct dry season. The average annual precipitation at the study site from 2000 to 2009 was 2,600 mm, and the average annual temperature over that period was 25.8°C (Kume et al., 2011).
Plant materials and measurements of leaf toughness
We analyzed 135 individuals of 103 species, representing 66 genera and 27 families, and all strata between the understory and canopy trees (Appendices 1, 2). Species composition varied with height. The occurrence of BSEs was determined by microscopy (Kenzo et al., 2007). Although most specimens were collected from mature individuals, we sampled juvenile and mature trees of eight species from four families (Swintonia foxworthyi, Dipterocarpus globosus, Dryobalanops aromatica, Shorea beccariana, Shorea macroptera, Vatica oblongifolia, Mallotus eucastus, and Allantospermum borneense). The presence of BSEs was unaffected by ontogeny. Most measurements were conducted in March 2006 and July 2007. Five mature leaves were collected from the top of the crown of each individual plant, and leaf thickness was measured with a micrometer (CLM1-15QMX; Mitsutoyo, Kawasaki, Japan). Leaf toughness (MPa) was measured using a penetrometer (RX-1; Aikoh Engineering Co., Tokyo, Japan) with a straight metal rod 2 mm in diameter (Kenzo et al., 2012). This method measures the load required for the metal rod to penetrate a leaf blade (Onoda et al., 2011). Toughness was measured five times per leaf, and the average value was used in analyses. Measurements were taken on the leaf blades, excluding the main vein but in most cases including BSEs.
Leaf mass per area, density, and C and N contents
After measurement, a sample of the leaf blade was punched out with a 5-mm diameter punch and dried at 60°C for 3 days to calculate the LMA. Leaf density was calculated by dividing the LMA by the leaf thickness (Niinemets, 2001). The N and C contents of the punched leaf sample were determined using an NC analyzer (Sumigraph NC-900; Shimadzu, Kyoto, Japan).
Leaf photosynthesis
A portable photosynthesis apparatus (LI-6400; Li-Cor, Lincoln, NE, USA) was used to determine the maximum photosynthetic rate (Pmax) in the morning (between 08:00 and 11:00 h), to avoid the midday depression in photosynthesis (Kenzo et al., 2015). The measurement was conducted while the leaf was attached to the plant using a canopy crane system (Kenzo et al., 2015). We used three fully expanded and apparently non-senescent leaves, generally overlapping with the leaves used for toughness measurement. Environmental conditions inside the chamber were controlled to maintain a leaf temperature of 30°C, relative humidity of approximately 60%, CO2 concentration of 360 ppm, and saturation levels of active photosynthetic photon flux density (800 μmol photon m–2 s–1 for understory trees and 1,700 μmol photon m–2 s–1 for mid- and upper-canopy trees, respectively). Notably, a large Pmax dataset was used in our previous study (Kenzo et al., 2015), although we were unable to measure approximately 10 individuals.
Statistical analyses
Scatterplots of leaf toughness against tree height and leaf traits were plotted and linearly fitted, and the significance of the resulting equations was tested through regression analysis. Although we attempted log transformations to analyze relationships such as that between leaf traits and tree height, the regression coefficients (r2) were approximately 10% lower than when we used the normal scale. Thus, we used normally scaled data for all analyses. We used an ordinary latest-squares regression to investigate changes associated with height and leaf traits. Differences in the intercepts of the linear regressions between heterobaric and homobaric leaves associated with tree height or leaf traits were examined using analysis of covariance (ANCOVA, Sokal and Rohlf, 1995).
To characterize tree species and families, despite the small number of individuals, we created lists of species with high and low toughness and used them to compare leaf morphology and habitat. Habitats were classified into three types based on tree height at maturity (Kenzo et al., 2007): understory (<12.5 m), subcanopy (12.5–27.5 m), and canopy (>27.5 m) (Appendix 2). Differences in leaf toughness, LMA, and thickness among 10 families that included three or more species were assessed by ANOVA and post hoc Tukey’s HSD tests. The proportion of heterobaric species in each family was tested using Fisher’s exact test (Sokal and Rohlf, 1995).
The leaf traits related to leaf toughness were identified through stepwise multiple regression (Sokal and Rohlf, 1995). LMA, leaf thickness, leaf density, C content, and the presence of BSEs (heterobaric or homobaric leaf type) were used as explanatory variables. For the presence of BSEs, a dummy variable was used, with a heterobaric leaf represented by 1 and homobaric leaf by 0. In addition to the multiple regression, we conducted a principal component regression (PCR) to explore the relationships between leaf toughness and other leaf traits. Before the PCR, we conducted a principal component analysis (PCA) using leaf thickness, density, LMA, C content, and the presence of BSEs as variables, and calculated a PCA score for each tree. Similar analyses were conducted to identify the variables explaining leaf Pmax using LMA, leaf thickness, density, N content, and the presence of BSEs as explanatory variables. All statistical analyses were performed with SPSS software for Windows (ver. 27.0; IBM Corp., Armonk, NY, USA).
Results
Relationship between leaf toughness and tree height
Leaf toughness increased significantly with tree height, regardless of the presence of BSEs (heterobaric leaves: y = 0.04 + 0.84x, r2 = 0.45, P < 0.0001; homobaric leaves: y = 0.02 + 0.76x, r2 = 0.24, P < 0.0001; Figure 2A). Greater toughness was observed in canopy leaves, although toughness values varied more than fivefold among canopy trees. Most homobaric canopy leaves had toughness values < 2 MPa, whereas several heterobaric leaves had toughness values of 3–4 MPa. Thus, leaf toughness was significantly greater for heterobaric than homobaric leaves, even at the same height (ANCOVA, P < 0.0001). Toughness according to leaf blade thickness increased slightly with tree height in heterobaric species (y = 0.00003 + 0.005x, r2 = 0.12, P < 0.01), although it was nearly constant across tree height in homobaric species (r2 = 0.005, P > 0.05; Figure 2B). The toughness value was significantly higher in species with heterobaric than homobaric leaves (ANCOVA, P < 0.001). The toughness per leaf blade volume of both leaf types increased with tree height in heterobaric leaves (y = 0.06 + 2.4x, r2 = 0.23, P < 0.001; homobaric leaves: P > 0.05), and was significantly higher in heterobaric than homobaric leaves (ANCOVA, P < 0.01; Figure 2C). For both leaf types, the relationship between toughness and leaf C content did not vary with tree height (P > 0.05; Figure 2D), but toughness was significantly higher in heterobaric species (ANCOVA, P < 0.001).
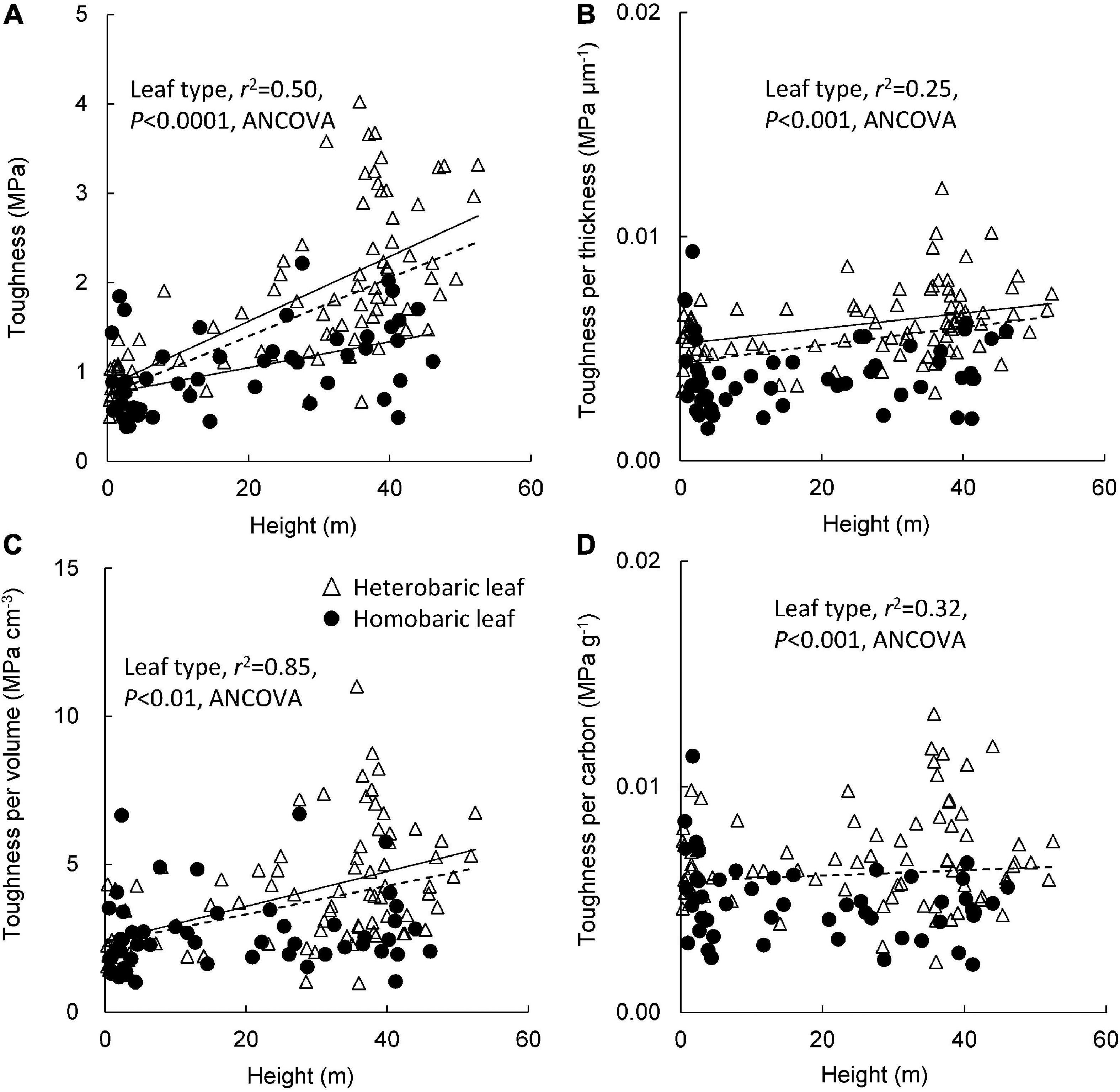
Figure 2. Changes in leaf toughness (A), toughness per leaf blade thickness (B), toughness per leaf blade volume (C), and toughness per carbon (D) with tree height between heterobaric and homobaric leaf trees. Regression lines are: (A) heterobaric leaf, r2 = 0.45, P < 0.0001; homobaric leaf, r2 = 0.24, P < 0.0001; (B) heterobaric leaf, r2 = 0.12, P < 0.01; homobaric leaf, ns; (C) heterobaric leaf, r2 = 0.23, P < 0.001; homobaric leaf, ns; (D) heterobaric and homobaric leaf, ns. “ns” means not significant. Details of the results of ANCOVA appear in Appendix 6. Dotted line means regression line for pooled data for both leaf types.
We observed differences in toughness among ontogenetic stages (Table 1). Leaf toughness of the eight species from which we sampled mature and juvenile trees was 0.98 ± 0.05 MPa for juveniles and 1.93 ± 0.22 MPa for mature trees. The leaves of mature trees were twice as tough, (P < 0.01, t-test; Table 1). The mature leaves of Dipterocarpus globosus and Shorea beccariana were nearly three times tougher than were the juvenile leaves, whereas the mature leaves of Mallotus eucastus were less than 10% tougher than juvenile leaves (Table 1).
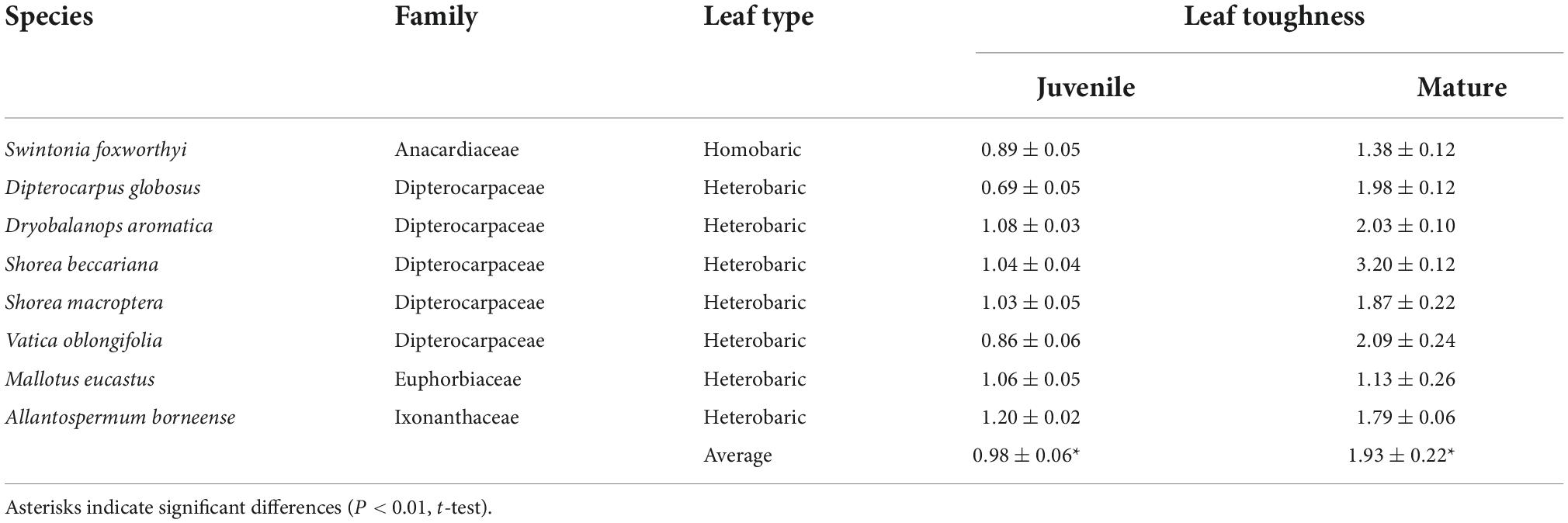
Table 1. Comparison of average leaf toughness (MPa) with standard error between juvenile and mature trees.
Variations in leaf toughness among species and families
Few families occurred in both the list of the 20 species with the highest toughness and the list of the 20 species with the lowest toughness (Tables 2, 3). The tougher-leaved species represented 11 families, and the softer-leaved species represented 13 families. Whereas the families Rubiaceae and Sapotaceae occurred in both lists, no genus in these families was common to both lists (Tables 2, 3). Calophyllum sp. had the toughest leaves (>4 Mpa). By contrast, Gomphandra cumingiana had the lowest toughness value (0.38 MPa), less than one-tenth that of Calophyllum sp. LMA of Gomphandra cumingiana was less than 3.2-fold compared with Calophyllum sp. Among the commonly occurring Rubiaceae species, the highest toughness was observed in Mussaendopsis beccariana (3.67 MPa) and the lowest in Pavetta axillaris (0.48 MPa), a 7.8-fold difference. In the Sapotaceae, Palaquium sp. had the highest toughness (2.23 MPa) and Madhuca crassipes had the lowest toughness value (0.66 MPa), a 3.4-fold difference. Eight Dipterocarpaceae were among the species with highest toughness. There was a clear trend in the 20 species with high leaf toughness with respect to habitat and leaf type: 90% were canopy species (mature height > 27.5 m; Kenzo et al., 2007), and 95% were heterobaric species. The only homobaric species on this list was Hydnocarpus pinguis, a sub-canopy species (Table 2). By contrast, 90% of the softer-leaved species had homobaric leaves, 60% were understory species, and 30% were sub-canopy species (Table 3). The proportion of leaf types in different habitats, based on tree height, also exhibited a clear gradient between the understory and the canopy. Most (78%) canopy trees had heterobaric leaves, whereas 93% of understory trees and 67% of sub-canopy trees had homobaric leaves (Appendix 3).
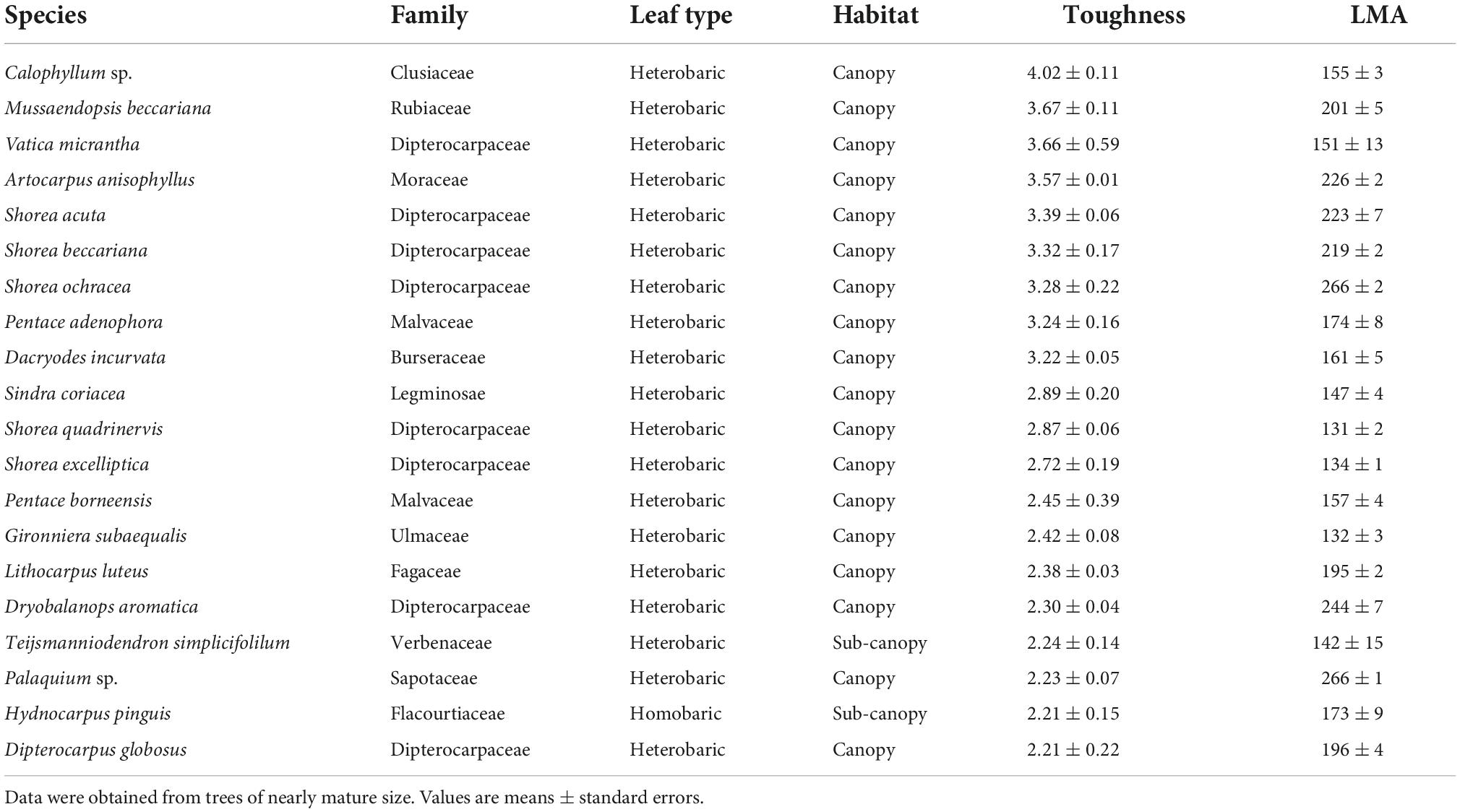
Table 2. The 20 species with the highest leaf toughness with LMA, shown in descending order of average toughness (MPa), and their LMA (g m–2) of three leaves.
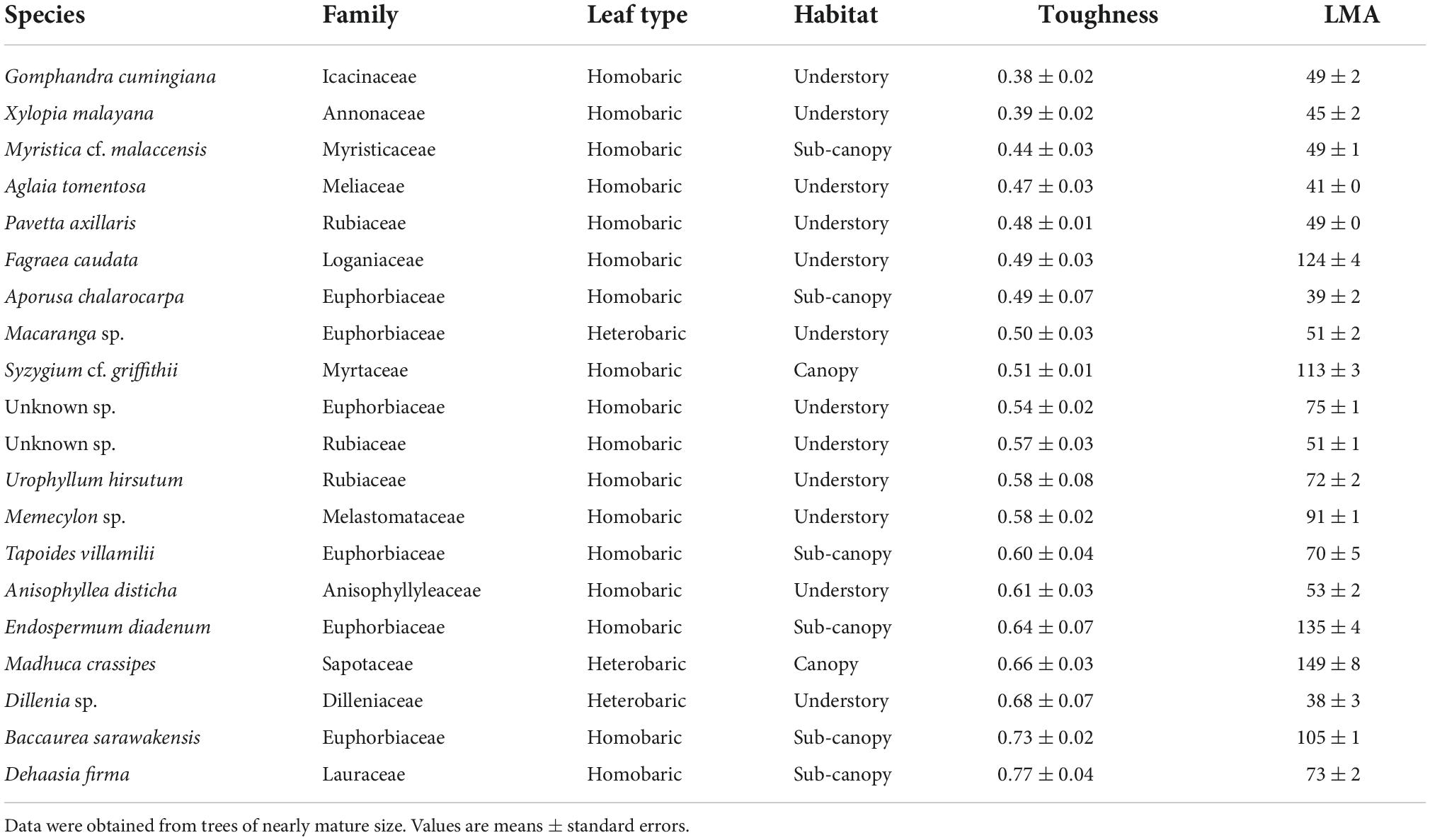
Table 3. The 20 species with the lowest leaf toughness, shown in ascending order of average toughness (MPa), and their LMA (g m–2) of three leaves.
A comparison of the leaf toughness in the 10 families containing at least three species indicated significant differences between families (P < 0.001, ANOVA; Table 4). Dipterocarpaceae species had the highest toughness (2.19 ± 0.12 MPa), more than double that of the family with the lowest toughness (Euphorbiaceae; 0.75 ± 0.07 MPa). The proportions of heterobaric and homobaric species also differed significantly among families (χ2 = 41.1, df = 9, P < 0.0001, Fisher’s exact test). For example, all 22 Dipterocarpaceae species had heterobaric leaves, whereas approximately 78% of species in the Euphorbiaceae had homobaric leaves. Leaf thickness did not differ significantly among families (P > 0.05, ANOVA; Table 4), whereas the LMA differed significantly (P < 0.001, ANOVA; Table 4). The LMA was highest in the Dipterocarpaceae (170.3 ± 7.7 g m–2) and significantly lower in the Annonaceae (90.8 ± 14.6 g m–2), Euphorbiaceae (90.9 ± 9.6 g m–2), and Rubiaceae (93.3 ± 36.3 g m–2).
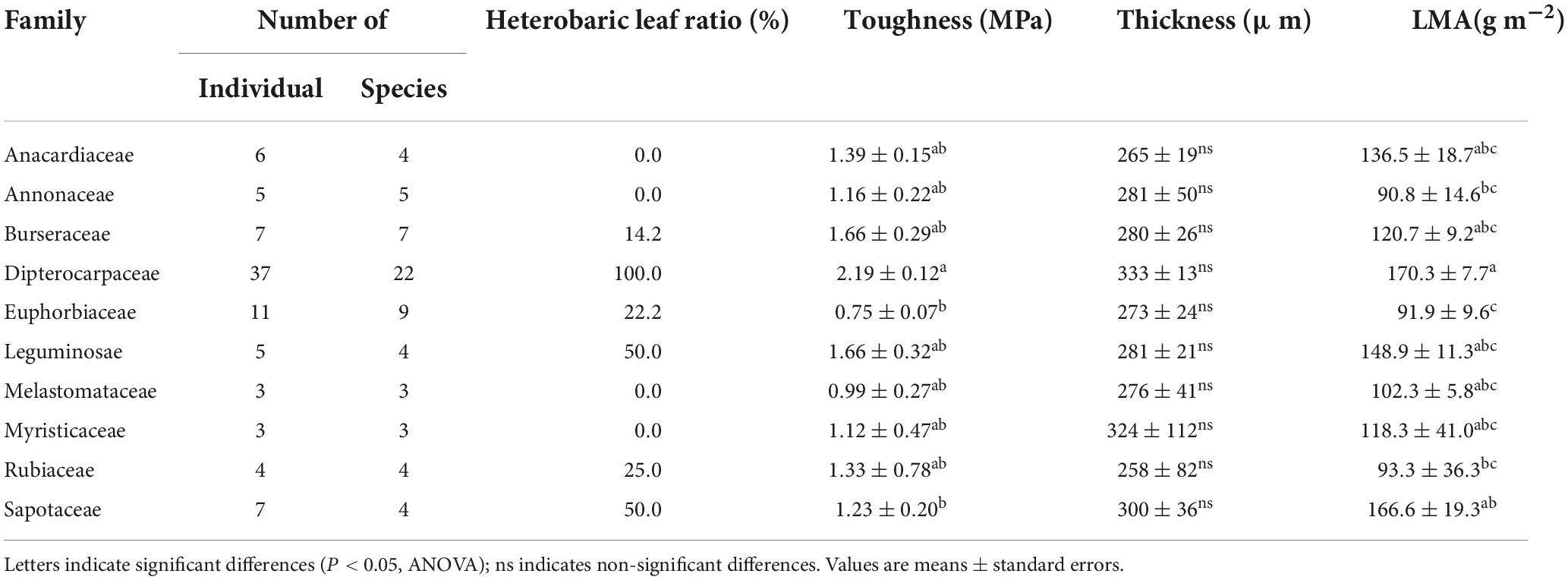
Table 4. The numbers of individuals and species, proportion of heterobaric species, leaf toughness, thickness, and LMA among 10 families.
Relationships between leaf traits and tree height
Leaf blade thickness increased with tree height in both homobaric (y = 3.0 + 215.0x, r2 = 0.24, P < 0.001) and heterobaric species (y = 4.4 + 171.7x, r2 = 0.53, P < 0.0001; Figure 3A). Although large variations of thickness were found among individuals and species, no significant difference was detected between heterobaric and homobaric species (ANCOVA, P > 0.05; Figure 3A). LMA was positively related to tree height, and had higher correlation coefficients than the relationship of tree height with thickness (homobaric species: y = 2.3 + 63.9x, r2 = 0.67; heterobaric species: y = 2.8 + 62.3x, r2 = 0.65; P < 0.0001; Figure 3B). This relationship did not differ significantly between leaf types (ANCOVA, P > 0.05; Figure 3B). Leaf density also increased significantly with tree height in both heterobaric (y = 0.003 + 0.40x, r2 = 0.21, P < 0.01) and homobaric species (y = 0.003 + 0.40x, r2 = 0.33, P < 0.001; Figure 3C). Heterobaric leaves had significantly higher leaf density than homobaric leaves (ANCOVA, P < 0.05; Figure 3C). Leaf C content increased with increasing height in both homobaric (y = 0.10 + 47.6x, r2 = 0.19, P < 0.01) and heterobaric leaves (y = 0.12 + 47.3x, r2 = 0.20, P < 0.01), and no significant difference was detected between leaf types (ANCOVA, P > 0.05; Figure 3D).
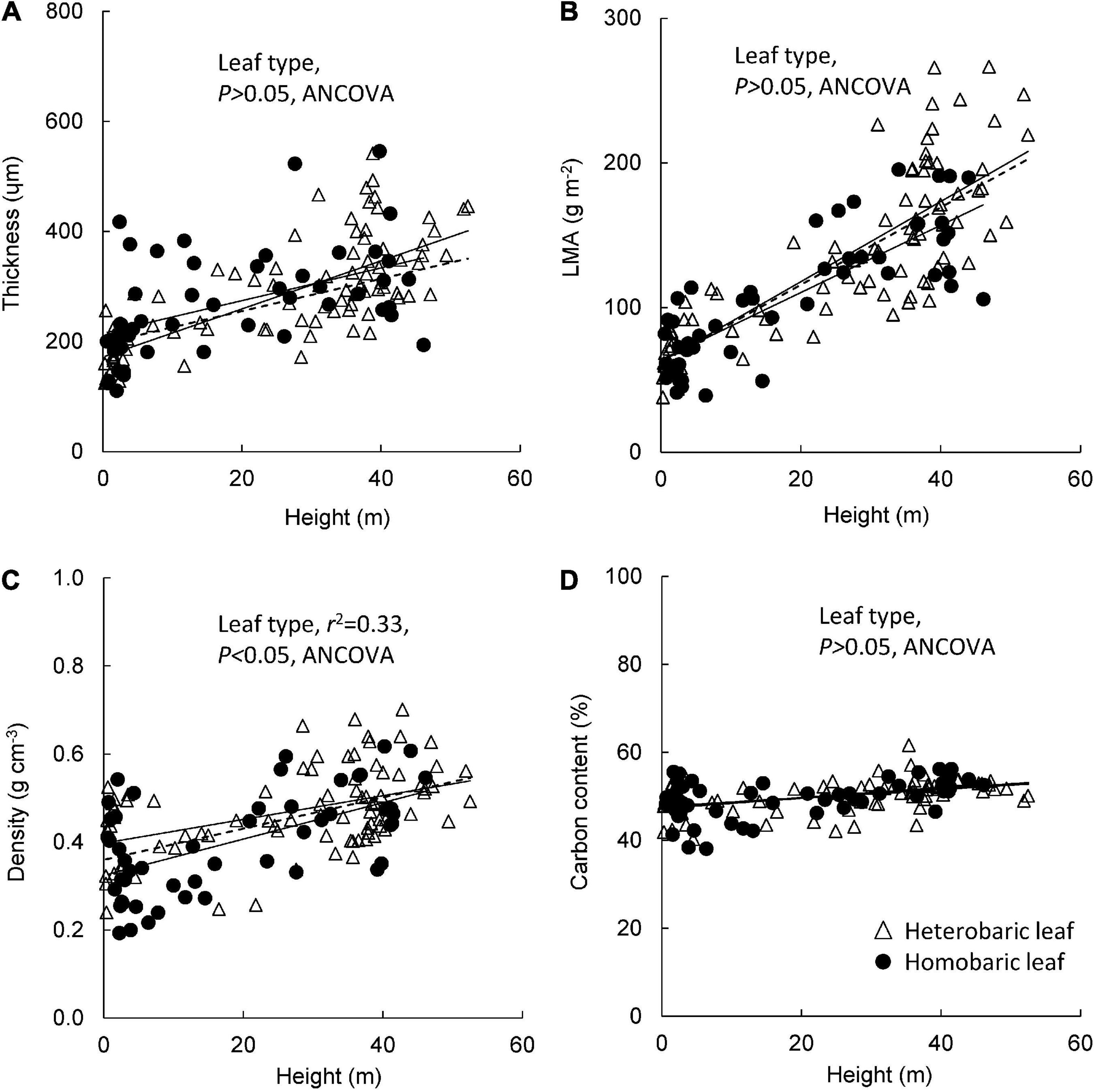
Figure 3. Changes in leaf traits with tree height for heterobaric and homobaric leaves: height in relation to leaf blade thickness (A), LMA (B), leaf density (C), and carbon content (D). Regression lines are as follows: (A) heterobaric leaf, r2 = 0.53, P < 0.0001; homobaric leaf, r2 = 0.24, P < 0.001; (B) heterobaric leaf, r2 = 0.65, P < 0.0001; homobaric leaf, r2 = 0.67, P < 0.0001; (C) heterobaric leaf, r2 = 0.21, P < 0.0001; homobaric leaf, r2 = 0.21, P < 0.0001; (D) heterobaric leaf, r2 = 0.20, P < 0.001; homobaric leaf, r2 = 0.19, P < 0.001. Details of the results of ANCOVA appear in Appendix 7. Dotted line means regression line for pooled data for both leaf types.
Relationships between leaf toughness and leaf traits
Significant correlations were found between leaf toughness and leaf blade thickness in both heterobaric (y = 0.008 + 0.21x, r2 = 0.72, P < 0.001) and homobaric species (y = 0.003 + 0.21x, r2 = 0.37, P < 0.001; Figure 4A). At the same leaf thickness, heterobaric leaves had significantly greater toughness than homobaric leaves (ANCOVA, P < 0.001). A similar trend was found between toughness and LMA in heterobaric (y = 0.011 + 0.27x, r2 = 0.52, P < 0.001) and homobaric species (y = 0.007 + 0.23x, r2 = 0.50, P < 0.001; Figure 4B). Greater toughness was observed in heterobaric than homobaric species, even at the same LMA (ANCOVA, P < 0.001). A positive relationship was found between leaf toughness and leaf density in homobaric species (y = 1.48 + 0.43x, r2 = 0.13, P < 0.01), but this relationship was not significant in heterobaric species (r2 = 0.01, P > 0.05; Figure 4C). Leaf toughness was positively correlated with leaf C content in homobaric species (y = 0.03–0.61x, r2 = 0.10, P < 0.05), but this relationship was not significant for heterobaric species (r2 = 0.02, P > 0.05; Figure 4D). Heterobaric species had tougher leaves than homobaric species at the same leaf density and leaf C content (ANCOVA, P < 0.001; Figures 4C,D).
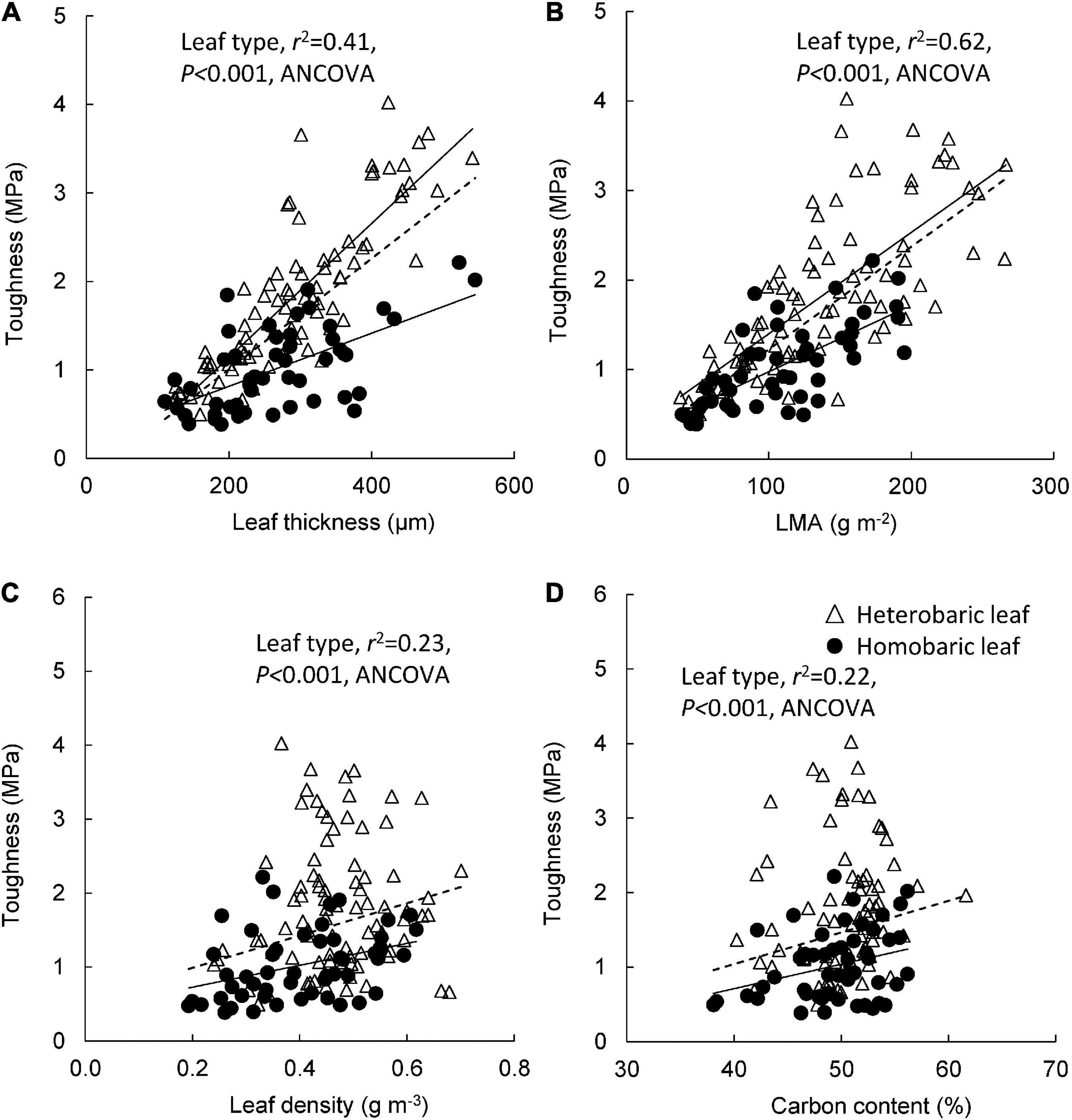
Figure 4. Leaf toughness in relation to leaf blade thickness (A), LMA (B), leaf density (C), and leaf carbon content (D). Regression lines are as follows: (A) heterobaric leaf, r2 = 0.72, P < 0.0001; homobaric leaf, r2 = 0.37, P < 0.0001; (B) heterobaric leaf, r2 = 0.53, P < 0.0001; homobaric leaf, r2 = 0.50, P < 0.0001; (C) heterobaric leaf, ns; homobaric leaf, r2 = 0.13, P < 0.01; (D) heterobaric leaf, ns; homobaric leaf, r2 = 0.09, P < 0.05. Details of the results of ANCOVA appear in Appendix 8. “ns” means not significant. Dotted line means regression line for pooled data for both leaf types.
We compared four models to identify the variables driving leaf toughness. We developed three models using stepwise multiple regression and calculated Akaike’s information criterion (AIC) for each one (Table 5). Model 1 included only the LMA as an explanatory variable and had the largest AIC value. Model 2 included the LMA and the presence of BSEs as explanatory variables, and Model 3, which had the lowest AIC value (Table 5), included the LMA, leaf thickness, and the presence of BSEs. All three multiple regressions indicated that leaf toughness correlated positively with the LMA, leaf thickness, and presence of BSEs (P < 0.0001; Table 5). Although the AIC value for Model 4 (PCR) was higher than those for Models 2 and 3, PC axes 1 and 2 also significantly explained leaf toughness (Table 5). Axis 1 corresponded to dense leaves (high LMA and leaf density, PCA1; Appendix 4), which correlated positively with toughness. By contrast, axis 2 corresponded to positively with thin leaves, as it was related negatively to toughness. Similar to the multiple regression, these results indicate that higher toughness was associated with leaves that are dense, thick, or have high LMAs.
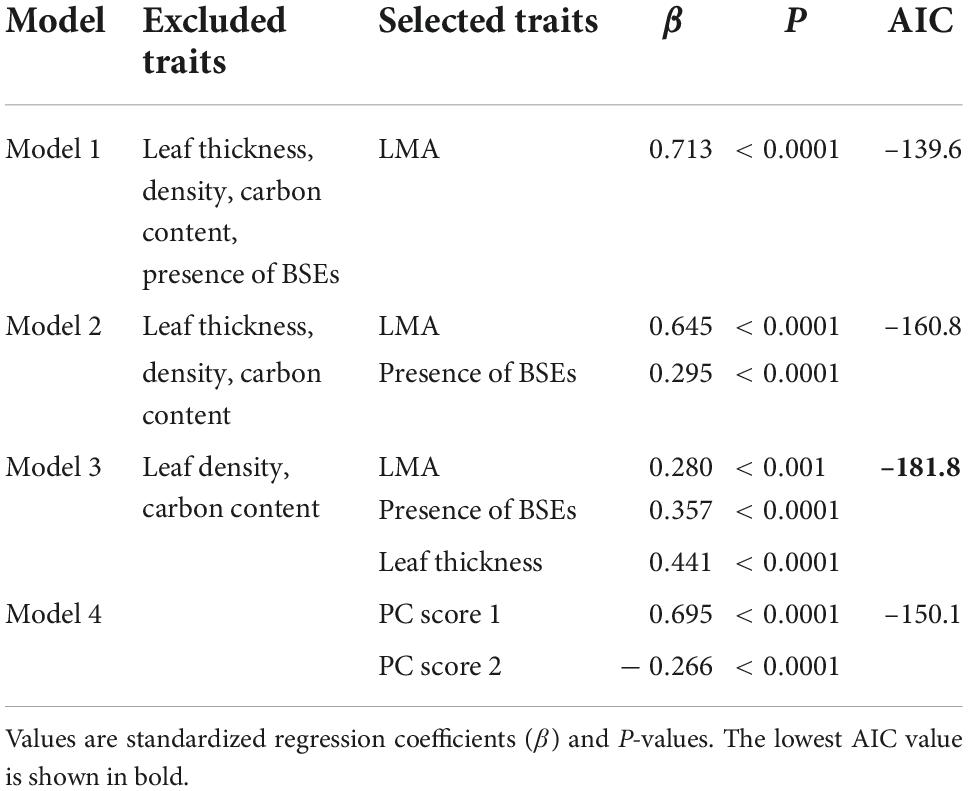
Table 5. Comparison of models including different trait combinations to explain the variation in leaf toughness.
Leaf photosynthesis rate and N content in relation to tree height and toughness
Pmax increased with tree height regardless of leaf type (homobaric species: y = 0.20 + 2.99x, r2 = 0.84; heterobaric species: y = 0.24 + 2.71x, r2 = 0.77; P < 0.0001; Figure 5A). Although similar relationships with tree height were found in homobaric and heterobaric species (P > 0.05, ANCOVA), upper canopy trees with heterobaric leaves (tree height > 27.5 m; Kenzo et al., 2007) had significantly higher Pmax values (n = 48, 11.9 ± 0.4 μmol m–2 s–1) than homobaric species (n = 17, 10.4 ± 0.4 μmol m–2 s–1, P < 0.05, ANOVA). We also assessed interspecific and between-family differences in heterobaric species among the upper canopy trees. Four of the families (Burseraceae, Dipterocarpaceae, Leguminosae, and Sapotaceae) did not differ significantly (P > 0.05, ANOVA; Table 6). By contrast, we observed significant interspecific differences among four species of Dipterocarpaceae (P < 0.05, ANOVA; Table 7). In particular, the Pmax of Shorea beccariana was approximately 1.5 times higher than those of S. acuta and Dipterocarpus globosus. Leaf N content also increased significantly with tree height (homobaric species: y = 0.04 + 0.93x, r2 = 0.71; heterobaric species: y = 0.04 + 0.84x, r2 = 0.77; P < 0.0001), although the difference between leaf types was not significant (P > 0.05, ANCOVA; Figure 5B). A positive correlation was found between Pmax and N in both heterobaric (y = 4.62–0.12x, r2 = 0.77, P < 0.0001) and homobaric (y = 3.84 + 0.26x, r2 = 0.73, P < 0.0001) species (Figure 5C). Pmax was higher in heterobaric than homobaric leaves at the same N content (P < 0.05, ANCOVA; Figure 5C).
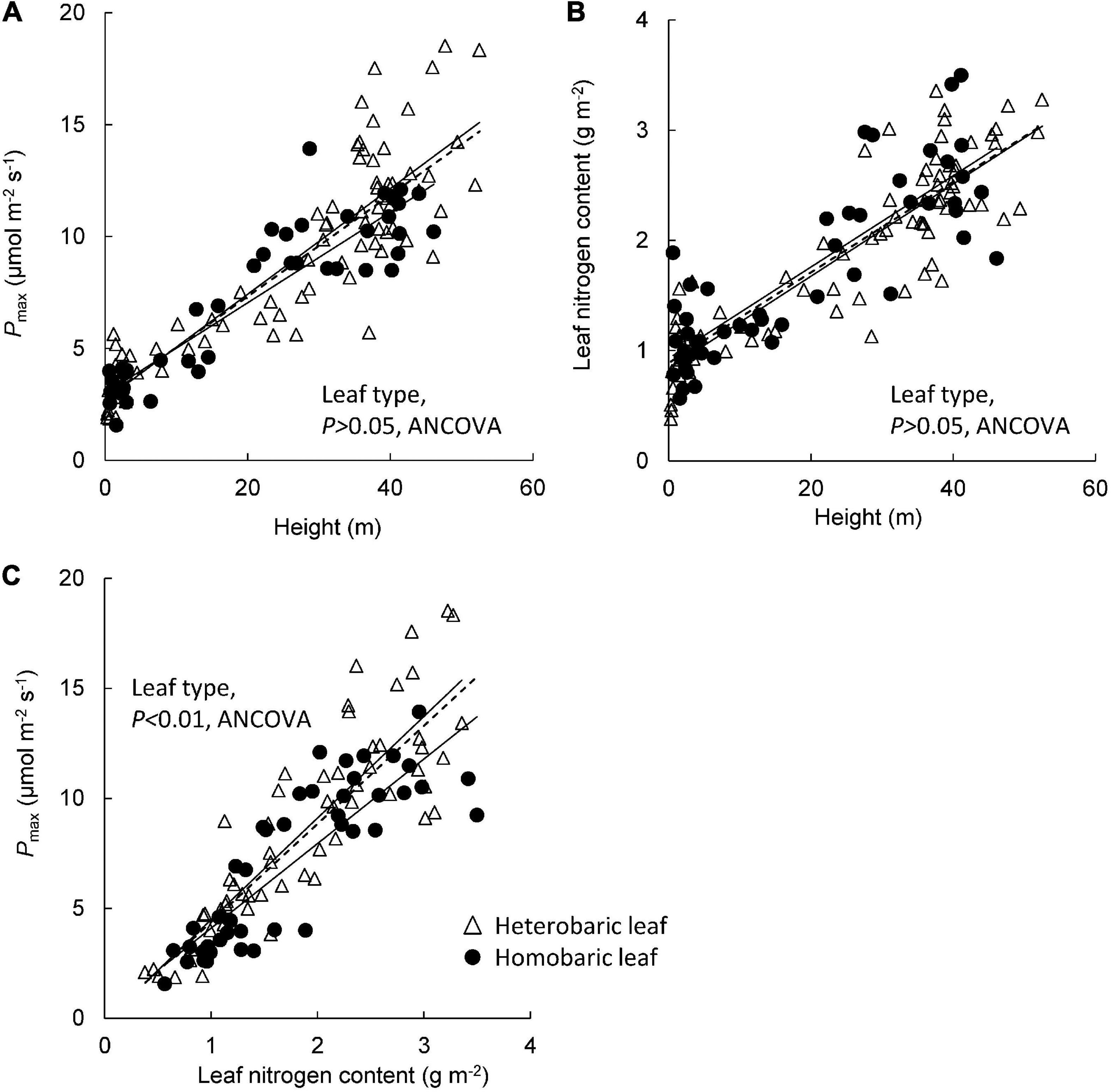
Figure 5. Relationships of tree height with Pmax (A) and leaf nitrogen content (B), and of Pmax with leaf nitrogen content (C). Regression lines are as follows: (A) heterobaric leaf, r2 = 0.77, P < 0.0001; homobaric leaf, r2 = 0.84, P < 0.0001; (B) heterobaric leaf, r2 = 0.77, P < 0.0001; homobaric leaf, r2 = 0.71, P < 0.0001; (C) heterobaric leaf, r2 = 0.77, P < 0.0001; homobaric leaf, r2 = 0.73, P < 0.0001. Details of the results of ANCOVA appear in Appendix 9. Dotted line means regression line for pooled data for both leaf types.
Pmax increased with leaf toughness regardless of leaf type, although the r2 value for heterobaric species was approximately twice that for homobaric species (homobaric species: y = 3.13 + 3.69x, r2 = 0.18; heterobaric species: y = 2.95 + 3.74x, r2 = 0.36; P < 0.01; Figure 6A). Although the ranges of leaf toughness and Pmax were 1.5–2.0 times greater for heterobaric species, the slope and intercept of the regression were similar among leaf types (ANCOVA, P > 0.05; Figure 6A). Leaf N content also increased with leaf toughness (homobaric species: y = 0.87 + 0.80x, r2 = 0.28; heterobaric species: y = 0.63 + 0.81x, r2 = 0.50; P < 0.01; Figure 6B). Homobaric species showed higher N contents than heterobaric species at the same toughness value (ANCOVA, P < 0.01; Figure 6B).
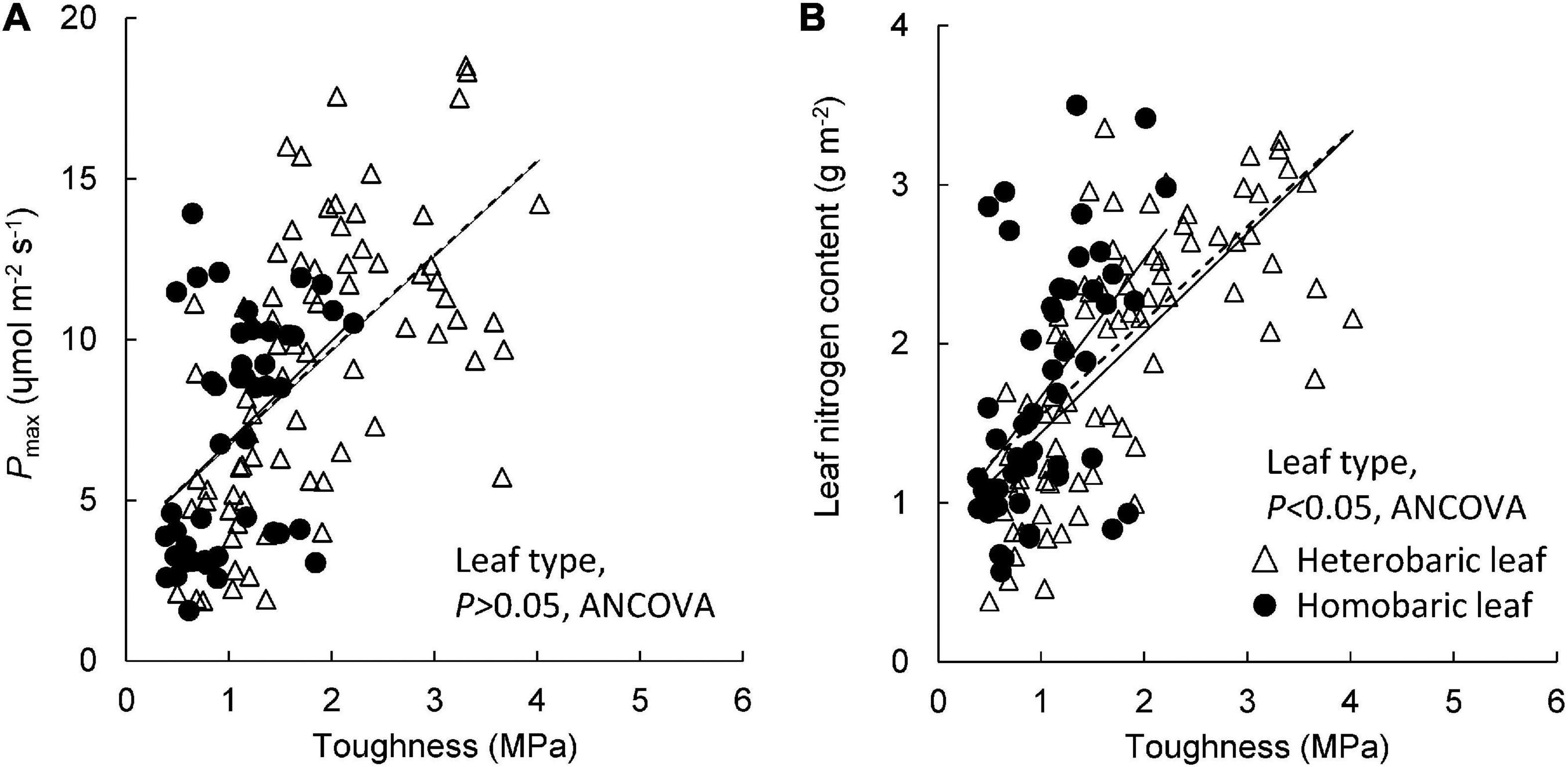
Figure 6. Leaf toughness in relation to Pmax (A) and leaf nitrogen content (B). Regression lines are as follows: (A) heterobaric leaf, r2 = 0.36, P < 0.0001; homobaric leaf, r2 = 0.18, P < 0.01; (B) heterobaric leaf, r2 = 0.50, P < 0.0001; homobaric leaf, r2 = 0.28, P < 0.0001. Details of the results of ANCOVA appear in Appendix 10. Dotted line means regression line for pooled data for both leaf types.
The drivers of leaf Pmax were inferred using multiple regression, and included the leaf N content alone (Model 1), leaf N content and LMA (Model 2), and leaf N content, LMA, and the presence of BSEs (Model 3; Table 8). These variables correlated positively with Pmax in all models. By contrast, only PC score 1 correlated positively with Pmax in Model 4, which was obtained using PCR. The first PCA axis was associated strongly with the LMA, leaf N content, and toughness, suggesting that these traits are associated strongly with higher Pmax (Appendix 5). Among the four models, Model 3 had the lowest AIC value (Table 8).
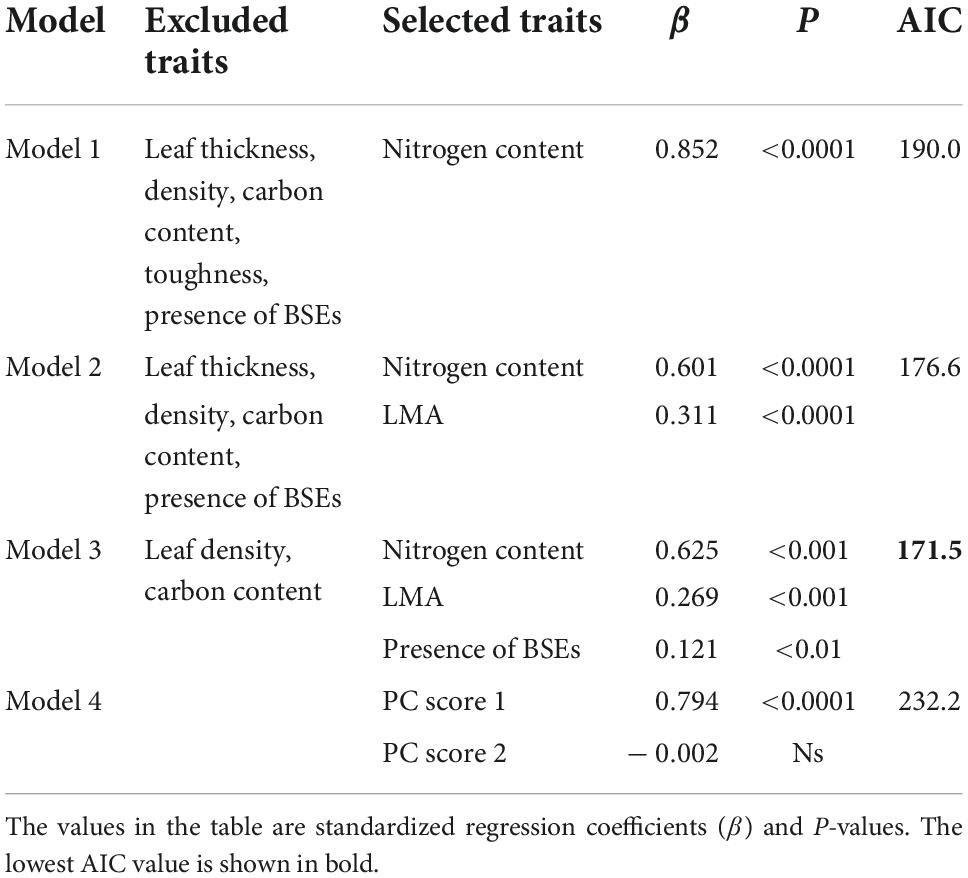
Table 8. Comparison of models including different trait combinations to explain the variation in leaf Pmax.
Discussion
Leaf toughness among species and families
Leaf toughness varied more than 10-fold, though that of LMA was less than 3.2-fold between the species with the lowest (Gomphandra cumingiana) and highest (Calophyllum sp.) values, consistent with other reports that leaf toughness is a highly variable trait (Onoda et al., 2011). Species in the genus Calophyllum have been reported to have tougher leaves than do co-occurring species in other regions, such as Singapore and Cambodia (Lucas and Pereira, 1990; Kenzo et al., 2012), and the strong fibers in BSEs contribute to their toughness (Lucas et al., 1991). Although additional research is required, toughness is partly constrained by phylogeny (Kitajima and Poorter, 2010). Among the studied leaf traits, leaf toughness was highly variable among families. For example, leaves of Dipterocarpaceae species were three times tougher than those of Euphorbiaceae species, whereas leaf thickness did not differ between these families, and differences in the LMA were less than twofold. Species in the Dipterocarpaceae family had the toughest leaves and highest LMA, which may contribute to their dominance in the canopy with higher physical stress, drought and herbivore attack condition.
Vertical variations in leaf toughness and leaf morphological traits
Increasing leaf toughness with tree height was observed in both leaf types, and may be an adaptation to the high levels of herbivore pressure and physical stresses, such as strong winds, in the forest canopy (Dominy et al., 2003; Yoneyama and Ichie, 2019). In accordance with our prediction, heterobaric species had tougher leaves than homobaric species, although large variations were observed according to tree height. Tougher heterobaric leaves indicate that fibrous BSEs may strengthen the leaf blade, as higher toughness in association with BSEs has been reported in several tropical rainforest tree species (Lucas et al., 1991, 1995; Choong et al., 1992; Choong, 1996). We also found that leaf toughness may be more sensitive to height in heterobaric than homobaric species, which may indicate that heterobaric leaves can achieve high plasticity in terms of toughness through alterations of the density of BSEs. In addition, we observed a positive relationship between tree height and leaf toughness, standardized by leaf thickness, in heterobaric, but not homobaric, species. This finding indicates that the toughness of homobaric leaves depends on leaf thickness, whereas the toughness of heterobaric leaves is influenced by the structure of the BSEs, independent of thickness. Denser BSEs in the canopy compared with the forest floor have been reported in several trees, including the species studied here (Wylie, 1952; Kenzo et al., 2007; Lynch et al., 2012), and the presence of denser BSEs in canopy leaves is consistent with the observed increase in leaf toughness with tree height and the increment of toughness, independent of leaf thickness. However, it should be noted that tree species differed along the height gradient in our study. For example, many of the softer-leaved species were understory and sub-canopy trees that grow only in the forest interior and had homobaric leaves. Such species were common in the Euphorbiaceae and Rubiaceae families. By contrast, canopy species, such as the Dipterocarpaceae, had tougher leaves and were predominantly heterobaric. Even within the same family, homobaric understory trees had softer leaves, whereas heterobaric canopy trees had tougher leaves, with a 7.8-fold difference observed among Rubiaceae species. In other words, although species composition varies with height, there is a continuous shift in leaf toughness with tree height, which may indicate convergence based on height-related changes in environmental conditions and herbivory at the community level. Furthermore, similar changes in leaf traits between juveniles and mature trees, with increased toughness and LMA observed in leaves in mature trees, indicate that this convergence is important for survival and environmental adaptation in tropical rainforests.
In this study, the thicker and higher-LMA leaves observed in both the heterobaric and homobaric species in the upper canopy protect against heat and drought conditions in the forest canopy, consistent with previous studies (Niinemets, 2007; Leigh et al., 2012; Kenzo et al., 2015; Ichie et al., 2019). Furthermore, we found that the increases in thickness and LMA contributed to increased leaf toughness with tree height. These results are supported by a previous meta-analysis showing positive correlations of leaf toughness with LMA and thickness (Onoda et al., 2011). However, when the results were examined by leaf type, heterobaric species had tougher leaves than homobaric species with the same leaf thickness, LMA and density values. The meta-analysis showed that LMA alone explained only 50% of leaf toughness, suggesting that anatomical characteristics of the leaf, among others, are also related to toughness (Onoda et al., 2011). Our approach to explaining leaf toughness confirms this, as the AIC value for the model based only on the LMA was higher than those for models that included other leaf traits, such as the presence of BSEs. In accordance with our findings, that analysis of the correlation between leaf type and other factors demonstrates the importance of leaf anatomical structure to leaf toughness, particularly the presence or absence of BSEs in tropical forest trees. Leaf toughness showed a positive correlation with fiber mass (Kitajima et al., 2012, 2016), and heterobaric leaves likely have more fiber mass than homobaric leaves due to the presence of BSEs, which are fiber-rich structures (Wylie, 1951, 1952; Terashima, 1992; Sack and Scoffoni, 2013). Although quantifying the amount of fiber in leaves in the field is difficult, homobaric and heterobaric leaves can be readily distinguished in field observations, and may serve as a simple indicator of leaf toughness. In terms of leaf C resource allocation, heterobaric species more efficiently increase their toughness compared with homobaric species, where greater leaf toughness was observed in heterobaric than homobaric leaves at the same C content. It is not clear why the C content is similar across leaf types, although C may be distributed unevenly within leaves. In other words, BSEs may be high in C whereas other tissues, such as leaf mesophyll cells, may be lower in C, thus offsetting the differences between leaf types at the whole leaf scale.
In addition to the greater toughness of heterobaric species protecting against herbivory and physical stress, tougher leaves are also likely to have greater drought tolerance due to their lower leaf water potential, which could prevent cell collapse. Upper canopy leaves must reduce their water potential to absorb water from the soil (Scholander et al., 1965; Meinzer et al., 2001), and thus the leaf water potential of canopy and emergent trees reaching 40 m in height was generally < –2.0 MPa at our study site (Hiromi et al., 2012; Inoue et al., 2015; Kenzo et al., 2015). Lower midday water potential was observed in leaves with denser BSEs in temperate trees, consistent with our prediction (Kawai et al., 2017). In various forest ecosystems, the proportion of heterobaric species to homobaric species increases with tree height (McClendon, 1992; Boeger et al., 2016; Kenzo et al., 2016). For example, in the studied tropical rainforest, 78% of canopy trees have heterobaric leaves, whereas 93% of understory trees and 67% of sub-canopy trees have homobaric leaves (Appendix 3). Heterobaric leaves are advantageous and may increase as a proportion of total leaves in the upper canopy due to their higher photosynthetic and water conductivity capacities, as well as their greater toughness relative to homobaric leaves. In other words, BSEs increase the plasticity of traits related to leaf structure and function in accordance with irradiance levels and tree height (Barbosa et al., 2019).
Leaf toughness in relation to photosynthetic capacity and nitrogen content
In forest canopy environments, leaves can receive strong light; consequently, leaves with higher photosynthetic rates have an advantage due to their higher rates of C fixation (Ellsworth and Reich, 1993; Koike et al., 2001; Kenzo et al., 2006; Niinemets et al., 2015). Heterobaric species generally have higher photosynthesis rates than homobaric leaves in strong light environments, driven by higher water conductivity and increased light penetration into the inner leaf through BSEs (Scoffoni et al., 2008; Buckley et al., 2011; Zsögön et al., 2015; Karabourniotis et al., 2021). The Pmax of forest canopy tree species, including those found at our study site, is higher for heterobaric than homobaric species (Liakoura et al., 2009; Inoue et al., 2015). In the present study, trees above the forest canopy (taller than 27.5 m) with heterobaric leaves also had higher Pmax values than those with homobaric leaves, although the Pmax of trees below the forest canopy differed little between leaf types. Although there were few family-level differences in Pmax among canopy species with heterobaric leaves, significant interspecific differences among the four Dipterocarpaceae species were observed. These differences in Pmax may be due to differences in BSEs amount, and other leaf traits, such as the N content and LMA (Kenzo et al., 2004; Inoue et al., 2015). Conversely, smaller differences in Pmax among families may be due to the small number of species per family and the high variability of Pmax. This possibility should be reassessed in future studies including more species.
On the other hand, tougher leaves have increased internal resistance to CO2 diffusion, which limits photosynthesis, i.e., increased toughness due to thick cell walls may lower the photosynthesis rate (Hikosaka, 2004; Niinemets et al., 2005; Morison et al., 2007; Niinemets, 2007; Terashima et al., 2011). In heterobaric species, leaf toughness had a strong positive correlation with photosynthesis, whereas for homobaric species the correlation was weaker. This suggests that BSEs in heterobaric leaves support a higher photosynthesis rate by increasing water transport and light penetration, and simultaneously make leaves tougher due to their fibrous tissue. Thus, heterobaric leaves with BSEs exhibit high plasticity not only for toughness but also for Pmax, consistent with the hypothesis that BSEs function as a hub promoting the plasticity of various physiological properties, as demonstrated previously (Barbosa et al., 2019). In other words, BSEs may mitigate the trade-off between plant growth and defense against herbivory (Züst and Agrawal, 2017).
Increased photosynthesis requires investment of N in photosynthetic enzymes, such as ribulose-1,5-bisphosphate (RuBP), in the leaves (Evans, 1989; Evans and Poorter, 2001). In this study, leaf N content increased with tree height for both homobaric and heterobaric leaves, and a positive correlation between photosynthesis and N was found for both leaf types. In the model analysis of leaf traits related to Pmax, the contribution of the leaf N content was significant, although the LMA and the presence of BSEs also enhanced photosynthesis. These correlations between photosynthesis and N hold true across a broad range of plants, including tropical trees (Evans, 1989; Ellsworth and Reich, 1993; Reich et al., 1994; Hikosaka, 2004; Kenzo et al., 2012, 2015; Ellsworth et al., 2022). Interestingly, the intercept of the photosynthesis–N relationship was higher in heterobaric species, indicating that heterobaric species had greater photosynthetic N use efficiency (PNUE, leaf photosynthetic rate per N) than homobaric species. The higher PNUE of heterobaric leaves may be attributable to the contribution of BSEs to high Pmax. Future studies of the intracellular allocation of N between heterobaric and homobaric leaves may further elucidate differences in N usage between leaf types (Hikosaka, 2004).
Although canopy leaves may have a high risk of herbivore damage due to their high N content (Coley and Barone, 1996; Yoneyama and Ichie, 2019), positive correlations between leaf N and toughness were found for both heterobaric and homobaric leaves in this study, indicating that leaves with higher N are more resistant to herbivore attack. Herbivore defense is comprised of physical and chemical defenses, and BSEs contribute to physical defense by increasing leaf toughness (Coley and Barone, 1996; Züst and Agrawal, 2017). Homobaric leaves had higher N levels than heterobaric leaves of the same toughness, which put them at greater risk of herbivory (Coley, 1983; Yoneyama and Ichie, 2019). Homobaric leaves may have stronger chemical defenses against herbivory, which could be elucidated in detail in future research by quantifying tannins, phenols and alkaloids (Züst and Agrawal, 2017).
Conclusion
As hypothesized, leaf toughness varied significantly with tree height, indicating that heterobaric leaves are tougher than homobaric leaves. Based on our modeling approach, leaf toughness cannot be explained by the LMA alone, as reported in previous studies; rather, it is influenced by a combination of other leaf functional traits, particularly the occurrence of BSEs. The C contents of both leaf types were similar, which did not support our hypothesis; in other words, heterobaric leaves increased their toughness more efficiently in terms of C allocation. Furthermore, the higher Pmax values of the canopy leaves of heterobaric species suggests that BSEs promote toughness and photosynthesis, and also increase the proportion of heterobaric leaves in the canopy environments of tropical rainforests, which are characterized by high light, physical stress, and herbivore pressure.
Data availability statement
The original contributions presented in the study are included in the article/Supplementary material, further inquiries can be directed to the corresponding author.
Author contributions
TK and TI measured the leaf traits, interpreted the results, and wrote the manuscript. MM conducted site preparation. All authors contributed to the article and approved the submitted version.
Funding
This research was partly supported by a grant from the Core Research for Environmental Science and Technology program of the Japan Science and Technology Corporation (JST), by a Grant-in-Aid for scientific research (Nos. 24688017, 20K06153, and 21H05316) from the Ministry of Education, Science and Culture and by a JST/JICA-SATREPS (PUBS).
Acknowledgments
We are grateful to the Forest Department, Sarawak and Sarawak Forestry Corporation and Forestry and Forest Products Research Institute for their kind support of this study.
Conflict of interest
The authors declare that the research was conducted in the absence of any commercial or financial relationships that could be construed as a potential conflict of interest.
Publisher’s note
All claims expressed in this article are solely those of the authors and do not necessarily represent those of their affiliated organizations, or those of the publisher, the editors and the reviewers. Any product that may be evaluated in this article, or claim that may be made by its manufacturer, is not guaranteed or endorsed by the publisher.
Supplementary material
The Supplementary Material for this article can be found online at: https://www.frontiersin.org/articles/10.3389/ffgc.2022.1002472/full#supplementary-material
References
Barbosa, M. A. M., Chitwood, D. H., Azevedo, A. A., Araújo, W. L., Ribeiro, D. M., Peres, L. E., et al. (2019). Bundle sheath extensions affect leaf structural and physiological plasticity in response to irradiance. Plant Cell Environ. 42, 1575–1589. doi: 10.1111/pce.13495
Boeger, M. R. T., Silva, M. M., Nogueira, G., Alvarenga, A., and Pereto, S. S. (2016). Occurrence of homobaric and heterobaric leaves in two forest types of southern Brazil. Acta Bot. Brasilica 30, 304–312. doi: 10.1590/0102-33062016abb0064
Buckley, T. N., Sack, L., and Gilbert, M. E. (2011). The role of bundle sheath extensions and life form in stomatal responses to leaf water status. Plant Physiol. 156, 962–973. doi: 10.1104/pp.111.175638
Caldwell, E., Read, J., and Sanson, G. D. (2016). Which leaf mechanical traits correlate with insect herbivory among feeding guilds? Ann. Bot. 117, 349–361. doi: 10.1093/aob/mcv178
Cavaleri, M. A., Oberbauer, S. F., Clark, D. B., Clark, D. A., and Ryan, M. G. (2010). Height is more important than light in determining leaf morphology in a tropical forest. Ecology 91, 1730–1739. doi: 10.1890/09-1326.1
Choong, M. F. (1996). What makes a leaf tough and how this affects the pattern of Castanopsis fissa leaf consumption by caterpillars. Funct. Ecol. 10, 668–674. doi: 10.2307/2390178
Choong, M. F., Lucas, P. W., Ong, J. S. Y., Pereira, B., Tan, H. T. W., and Turner, I. M. (1992). Leaf fracture toughness and sclerophylly: Their correlations and ecological implications. New Phytol. 121, 597–610. doi: 10.1111/j.1469-8137.1992.tb01131.x
Coley, P. D. (1983). Herbivory and defensive characteristics of tree species in a lowland rain forest. Ecol. Monogr. 53, 209–233. doi: 10.2307/1942495
Coley, P. D., and Barone, J. A. (1996). Herbivory and plant defenses in tropical forests. Annu. Rev. Ecol. Syst. 27, 305–335. doi: 10.1146/annurev.ecolsys.27.1.305
Dominy, N. J., Lucas, P. W., and Wright, S. J. (2003). Mechanics and chemistry of rain forest leaves: Canopy and understorey compared. J. Exp. Bot. 54, 2007–2014. doi: 10.1093/jxb/erg224
Ellsworth, D. S., and Reich, P. B. (1993). Canopy structure and vertical patterns of photosynthesis and related leaf traits in a deciduous forest. Oecologia 96, 169–178. doi: 10.1007/BF00317729
Ellsworth, D. S., Crous, K. Y., De Kauwe, M. G., Verryckt, L. T., Goll, D., Zaehle, S., et al. (2022). Convergence in phosphorus constraints to photosynthesis in forests around the world. Nat. Commun. 13, 1–12. doi: 10.1038/s41467-022-32545-0
Evans, J. R. (1989). Photosynthesis and nitrogen relationships in leaves of C3 plants. Oecologia 78, 9–19. doi: 10.1007/BF00377192
Evans, J., and Poorter, H. (2001). Photosynthetic acclimation of plants to growth irradiance: The relative importance of specific leaf area and nitrogen partitioning in maximizing carbon gain. Plant Cell Environ. 24, 755–767. doi: 10.1046/j.1365-3040.2001.00724.x
Hikosaka, K. (2004). Interspecific difference in the photosynthesis–nitrogen relationship: Patterns, physiological causes, and ecological importance. J. Plant Res. 117, 481–494. doi: 10.1007/s10265-004-0174-2
Hiromi, T., Ichie, T., Kenzo, T., and Ninomiya, I. (2012). Interspecific variation in leaf water use associated with drought tolerance in four emergent dipterocarp species of a tropical rain forest in Borneo. J. For. Res. 17, 369–377. doi: 10.1007/s10310-011-0303-4
Ichie, T., Inoue, Y., Takahashi, N., Kamiya, K., and Kenzo, T. (2016). Ecological distribution of leaf stomata and trichomes among tree species in a Malaysian lowland tropical rain forest. J. Plant Res. 129, 625–635. doi: 10.1007/s10265-016-0795-2
Ichie, T., Yoneyama, A., Hashimoto, T., Tanaka-Oda, A., Kusin, K., and Kenzo, T. (2019). Drainage effects on leaf traits of trees in tropical peat swamp forests in Central Kalimantan. Indonesia. Tropics 28, 1–11. doi: 10.3759/tropics.MS18-12
Inoue, Y., Kenzo, T., Tanaka-Oda, A., Yoneyama, A., and Ichie, T. (2015). Leaf water use in heterobaric and homobaric leafed canopy tree species in a Malaysian tropical rain forest. Photosynthetica 53, 177–186. doi: 10.1007/s11099-015-0105-6
Karabourniotis, G., Bornman, J. F., and Nikolopoulos, D. (2000). A possible optical role of the bundle sheath extensions of the heterobaric leaves of Vitis vinifera and Quercus coccifera. Plant Cell Environ. 23, 423–430. doi: 10.1046/j.1365-3040.2000.00558.x
Karabourniotis, G., Liakopoulos, G., Bresta, P., and Nikolopoulos, D. (2021). The optical properties of leaf structural elements and their contribution to photosynthetic performance and photoprotection. Plants 10:1455. doi: 10.3390/plants10071455
Kato, M., Inoue, T., Hamid, A. A., Nagamitsu, T., Merdek, M. B., Nona, A. R., et al. (1995). Seasonality and vertical structure of light-attracted insect communities in a dipterocarp forest in Sarawak. Popul. Ecol. 37, 59–79. doi: 10.1007/BF02515762
Kawai, K., Miyoshi, R., and Okada, N. (2017). Bundle sheath extensions are linked to water relations but not to mechanical and structural properties of leaves. Trees 31, 1–11. doi: 10.1007/s00468-017-1540-8
Kenzo, T., Ichie, T., Watanabe, Y., and Hiromi, T. (2007). Ecological distribution of homobaric and heterobaric leaves in tree species of Malaysian lowland tropical rainforest. Am. J. Bot. 94, 764–775. doi: 10.3732/ajb.94.5.764
Kenzo, T., Ichie, T., Watanabe, Y., Yoneda, R., Ninomiya, I., and Koike, T. (2006). Changes in photosynthesis and leaf characteristics with tree height in five dipterocarp species in a tropical rain forest. Tree Physiol. 26, 865–873. doi: 10.1093/treephys/26.7.865
Kenzo, T., Ichie, T., Yoneda, R., Kitahashi, Y., Watanabe, Y., Ninomiya, I., et al. (2004). Interspecific variation of photosynthesis and leaf characteristics in canopy trees of five species of Dipterocarpaceae in a tropical rain forest. Tree Physiol. 24, 1187–1192. doi: 10.1093/treephys/24.10.1187
Kenzo, T., Inoue, Y., Yoshimura, M., Yamashita, M., Tanaka-Oda, A., and Ichie, T. (2015). Height-related changes in leaf photosynthetic traits in diverse Bornean tropical rain forest trees. Oecologia 177, 191–202. doi: 10.1007/s00442-014-3126-0
Kenzo, T., Tanaka-Oda, A., Mastuura, Y., and Hinzman, L. D. (2016). Morphological and physicochemical traits of leaves of different life-forms of various broadleaf woody plants in interior Alaska. Can. J. For. Res. 46, 1475–1482. doi: 10.1139/cjfr-2015-0417
Kenzo, T., Yoneda, R., Sano, M., Araki, M., Shimizu, A., Tanaka-Oda, A., et al. (2012). Variations in leaf photosynthetic and morphological traits with tree height in various tree species in a Cambodian tropical dry evergreen forest. JARQ 46, 167–180. doi: 10.6090/jarq.46.167
Kitajima, K., and Poorter, L. (2010). Tissue-level leaf toughness, but not lamina thickness, predicts sapling leaf lifespan and shade tolerance of tropical tree species. New Phytol. 186, 708–721. doi: 10.1111/j.1469-8137.2010.03212.x
Kitajima, K., Llorens, A. M., Stefanescu, C., Timchenko, M. V., Lucas, P. W., and Wright, S. J. (2012). How cellulose-based leaf toughness and lamina density contribute to long leaf lifespans of shade-tolerant species. New Phytol. 195, 640–652. doi: 10.1111/j.1469-8137.2012.04203.x
Kitajima, K., Wright, S. J., and Westbrook, J. W. (2016). Leaf cellulose density as the key determinant of inter-and intra-specific variation in leaf fracture toughness in a species-rich tropical forest. Interface Focus 6:20150100. doi: 10.1098/rsfs.2015.0100
Koike, T., Kitao, M., Maruyama, Y., Mori, S., and Lei, T. T. (2001). Leaf morphology and photosynthetic adjustments among deciduous broad-leaved trees within the vertical canopy profile. Tree Physiol. 21, 951–958. doi: 10.1093/treephys/21.12-13.951
Kume, T., Tanaka, N., Kuraji, K., Komatsu, H., Yoshifuji, N., Saitoh, T. M., et al. (2011). Ten-year evapotranspiration estimates in a Bornean tropical rainforest. Agric. For. Meteorol. 151, 1183–1192. doi: 10.1016/j.agrformet.2011.04.005
Leigh, A., Sevanto, S., Ball, M. C., Close, J. D., Ellsworth, D. S., Knight, C. A., et al. (2012). Do thick leaves avoid thermal damage in critically low wind speeds? New Phytol. 194, 477–487. doi: 10.1111/j.1469-8137.2012.04058.x
Li, A. O., Ng, L. C., and Dudgeon, D. (2009). Effects of leaf toughness and nitrogen content on litter breakdown and macroinvertebrates in a tropical stream. Aqua. Sci. 71, 80–93. doi: 10.1007/s00027-008-8117-y
Liakoura, V., Fotelli, M. N., Rennenberg, H., and Karabourniotis, G. (2009). Should structure–function relations be considered separately for homobaric vs. heterobaric leaves? Am. J. Bot. 96, 612–619. doi: 10.3732/ajb.0800166
Lloyd, J., Patiño, S., Paiva, R. Q., Nardoto, G. B., Quesada, C. A., Santos, A. J. B., et al. (2010). Optimisation of photosynthetic carbon gain and within-canopy gradients of associated foliar traits for Amazon forest trees. Biogeosciences 7, 1833–1859. doi: 10.5194/bg-7-1833-2010
Lucas, P. W., and Pereira, B. (1990). Estimation of the fracture toughness of leaves. Funct. Ecol. 4, 819–822. doi: 10.2307/2389448
Lucas, P. W., Choong, M. F., Tan, H. T. W., Turner, I. M., and Berrick, A. J. (1991). The fracture toughness of the leaf of the dicotyledon Calophyllum inophyllum L.(Guttiferae). Philos. Trans. Royal Soc. Lond. Series B. Biol. Sci. 334, 95–106. doi: 10.1098/rstb.1991.0099
Lucas, P. W., Darvell, B. W., Lee, P. K. D., Yuen, T. D. B., and Choong, M. F. (1995). The toughness of plant-cell walls. Philos. Trans. Royal Soc. B. 348, 363–372. doi: 10.1098/RSTB.1995.0074
Lucas, P. W., Turner, I. M., Dominy, N. J., and Yamashita, N. (2000). Mechanical defences to herbivory. Ann. Bot. 86, 913–920. doi: 10.1006/anbo.2000.1261
Lynch, D. J., McInerney, F. A., Kouwenberg, L. L., and Gonzalez-Meler, M. A. (2012). Plasticity in bundle sheath extensions of heterobaric leaves. Am. J. Bot. 99, 1197–1206. doi: 10.3732/ajb.1100525
McClendon, J. H. (1992). Photographic survey of the occurrence of bundle-sheath extensions in deciduous dicots. Plant Physiol. 99, 1677–1679. doi: 10.1104/pp.99.4.1677
Meinzer, F. C., Clearwater, M. J., and Goldstein, G. (2001). Water transport in trees: Current perspectives, new insights and some controversies. Environ. Exp. Bot. 45, 239–262. doi: 10.1016/S0098-8472(01)00074-0
Morison, J. I., Lawson, T., and Cornic, G. (2007). Lateral CO2 diffusion inside dicotyledonous leaves can be substantial: Quantification in different light intensities. Plant Physiol. 145, 680–690. doi: 10.1104/pp.107.107318
Niinemets, Ü (2001). Global-scale climatic controls of leaf dry mass per area, density, and thickness in trees and shrubs. Ecology 82, 453–469. doi: 10.1890/0012-96582001082[0453:GSCCOL]2.0.CO;2
Niinemets, Ü, Keenan, T. F., and Hallik, L. (2015). A worldwide analysis of within-canopy variations in leaf structural, chemical and physiological traits across plant functional types. New Phytol. 205, 973–993. doi: 10.1111/nph.13096
Niinemets, U. (2007). Photosynthesis and resource distribution through plant canopies. Plant Cell Environ. 30, 1052–1071. doi: 10.1111/j.1365-3040.2007.01683.x
Niinemets, ÜL. O., Cescatti, A., Rodeghiero, M., and Tosens, T. (2005). Leaf internal diffusion conductance limits photosynthesis more strongly in older leaves of Mediterranean evergreen broad-leaved species. Plant Cell Environ. 28, 1552–1566. doi: 10.1111/j.1365-3040.2005.01392.x
Nikolopoulos, D., Liakopoulos, G., Drossopoulos, I., and Karabourniotis, G. (2002). The relationship between anatomy and photosynthetic performance of heterobaric leaves. Plant Physiol. 129, 235–243. doi: 10.1104/pp.010943
Novotny, V., and Basset, Y. (2005). Host specificity of insect herbivores in tropical forests. Proc. Royal Soc. B. 272, 1083–1090. doi: 10.1098/rspb.2004.3023
Onoda, Y., Westoby, M., Adler, P. B., Choong, A. M., Clissold, F. J., Cornelissen, J. H., et al. (2011). Global patterns of leaf mechanical properties. Ecol. Lett. 14, 301–312. doi: 10.1111/j.1461-0248.2010.01582.x
Pérez-Harguindeguy, N., Díaz, S., Cornelissen, J. H., Vendramini, F., Cabido, M., and Castellanos, A. (2000). Chemistry and toughness predict leaf litter decomposition rates over a wide spectrum of functional types and taxa in central Argentina. Plant Soil 218, 21–30. doi: 10.1023/A:1014981715532
Reich, P. B., Walters, M. B., Ellsworth, D. S., and Uhl, C. (1994). Photosynthesis-nitrogen relations in Amazonian tree species. Oecologia 97, 62–72. doi: 10.1007/BF00317909
Ribeiro, S. P., and Basset, Y. (2007). Gall-forming and free-feeding herbivory along vertical gradients in a lowland tropical rainforest: The importance of leaf sclerophylly. Ecography 30, 663–672. doi: 10.1111/j.2007.0906-7590.05083.x
Sack, L., and Scoffoni, C. (2013). Leaf venation: Structure, function, development, evolution, ecology and applications in the past, present and future. New Phytol. 198, 983–1000. doi: 10.1111/nph.12253
Scholander, P. F., Hammel, H. T., Bradstreet, E. D., and Hemmingsen, E. A. (1965). Sap pressure in vascular plants. Negative hydrostatic pressure can be measured in plants. Science 148, 339–346. doi: 10.1126/SCIENCE.148.3668.339
Scoffoni, C., Pou, A., Aasamaa, K., and Sack, L. (2008). The rapid light response of leaf hydraulic conductance: New evidence from two experimental methods. Plant Cell Environ. 31, 1803–1812. doi: 10.1111/j.1365-3040.2008.01884.x
Sokal, R. R., and Rohlf, F. J. (1995). Biometry: The principles and practice of statistics in biological research, 3rd Edn. New York: W.H. Freeman and Co.
Taneda, H., Kandel, D. R., Ishida, A., and Ikeda, H. (2016). Altitudinal changes in leaf hydraulic conductance across five Rhododendron species in eastern Nepal. Tree Physiol. 36, 1272–1282. doi: 10.1093/treephys/tpw058
Terashima, I. (1992). Anatomy of non-uniform leaf photosynthesis. Photosynth. Res. 31, 195–212. doi: 10.1007/BF00035537
Terashima, I., Hanba, Y. T., Tholen, D., and Niinemets, Ü (2011). Leaf functional anatomy in relation to photosynthesis. Plant Physiol. 155, 108–116. doi: 10.1104/pp.110.165472
Thomas, S. C., and Ickes, K. (1995). Ontogenetic changes in leaf size in Malaysian rain forest trees. Biotropica 27, 427–434. doi: 10.2307/2388954
Thomas, S. C., Sztaba, A. J., and Smith, S. M. (2010). Herbivory patterns in mature sugar maple: Variation with vertical canopy strata and tree ontogeny. Ecol. Entomol. 35, 1–8. doi: 10.1111/j.1365-2311.2009.01133.x
Turner, I. M. (2001). The ecology of trees in the tropical rain forest. Cambridge: Cambridge University Press. doi: 10.1017/CBO9780511542206
Turner, I. M., Choong, M. F., Tan, H. T. W., and Lucas, P. W. (1993). How tough are sclerophylls? Ann. Bot. 71, 343–345. doi: 10.1006/anbo.1993.1043
Turner, I. M., Lucas, P. W., Becker, P., Wong, S. C., Yong, J. W. H., Choong, M. F., et al. (2000). Tree leaf form in Brunei: A heath forest and a mixed dipterocarp forest compared 1. Biotropica 32, 53–61. doi: 10.1111/j.1744-7429.2000.tb00447.x
Wylie, R. B. (1951). Principles of foliar organization shown by sun-shade leaves from ten species of deciduous dicotyledonous trees. Am. J. Bot. 38, 355–361. doi: 10.1002/j.1537-2197.1951.tb14834.x
Wylie, R. B. (1952). The bundle sheath extension in leaves of dicotyledons. Am. J. Bot. 39, 645–651. doi: 10.1002/J.1537-2197.1952.TB13080.X
Yoneyama, A., and Ichie, T. (2019). Relationship between leaf flushing phenology and defensive traits of canopy trees of five dipterocarp species in a tropical rain forest. Tropics 27, 67–79. doi: 10.3759/tropics.MS18-13
Zsögön, A., Alves Negrini, A. C., Peres, L. E. P., Nguyen, H. T., and Ball, M. C. (2015). A mutation that eliminates bundle sheath extensions reduces leaf hydraulic conductance, stomatal conductance and assimilation rates in tomato (Solanum lycopersicum). New Phytol. 205, 618–626. doi: 10.1111/nph.13084
Keywords: carbon content, heterobaric leaf, homobaric leaf, leaf functional traits, nitrogen
Citation: Kenzo T, Mohamad M and Ichie T (2022) Leaf toughness increases with tree height and is associated with internal leaf structure and photosynthetic traits in a tropical rain forest. Front. For. Glob. Change 5:1002472. doi: 10.3389/ffgc.2022.1002472
Received: 25 July 2022; Accepted: 25 October 2022;
Published: 09 November 2022.
Edited by:
Alexander Shenkin, University of Oxford, United KingdomReviewed by:
Gerardo Avalos, University of Costa Rica, Costa RicaWakana Azuma, Kobe University, Japan
Copyright © 2022 Kenzo, Mohamad and Ichie. This is an open-access article distributed under the terms of the Creative Commons Attribution License (CC BY). The use, distribution or reproduction in other forums is permitted, provided the original author(s) and the copyright owner(s) are credited and that the original publication in this journal is cited, in accordance with accepted academic practice. No use, distribution or reproduction is permitted which does not comply with these terms.
*Correspondence: Tanaka Kenzo, bW9uYUBhZmZyYy5nby5qcA==