- Department of Geography and the Environment, University of North Texas, Denton, TX, United States
Woody ecosystems have a relatively thin but aerially extensive and dynamic layer of bark that, like leaves, regulates material exchange at the interface of air, water, and biota. Through interception, retention, and leaching of materials and interactions with epiphytic communities, bark alters the chemistry and composition of water draining over its surface during precipitation. This mini-review explores different perspectives and approaches to the study of bark and what they reveal about the myriad ways bark surfaces influence the quality of sub-canopy precipitation. Observational studies conducted over the past five decades in the fields of environmental science, ecohydrology, epiphyte ecology, and microbiology demonstrate that bark is an accumulator, transporter, substrate, and reactor. Bark passively accumulates materials from the atmosphere, water, and canopies, and also serves as an active transport surface, exchanging materials laterally and longitudinally. In addition, bark substrates influence epiphyte diversity, composition, and distribution, which, in turn, affect material cycling. Bark surfaces are dynamic over time, changing in response to disturbances (e.g., insect outbreaks, aging, and tree death)—how such changes influence the chemical and elemental composition of throughfall and stemflow merits further study. Moving forward, integration of diverse perspectives and approaches is needed to elucidate the influence of bark surfaces on solute and particulate transport and cycling within woody ecosystems.
Introduction
The outer bark of tree branches and stems (i.e., phellem or rhytidome) constitutes a critical interface between the atmosphere, water, and vegetation that has important implications for the cycling of materials in woody plant-dominated ecosystems. Bark is a passive receptor surface to which materials deposit during precipitation (wet deposition) and via dry deposition. Some fraction of these materials can sorb to or be absorbed by bark surfaces, resulting in retention. Materials also leach from bark surfaces, moving through the bark into external solution that drains to the surface during storms. Growing on and within bark surfaces, epiphytic plants (e.g., mosses, ferns, and bromeliads) intercept, retain, and leach substances (Mendieta-Leiva et al., 2020), while bark-dwelling microorganisms and fauna produce, transform, and decompose materials (Aguirre-von-Wobeser, 2020). Exchanges between epiphytic communities and their substrates create additional pathways for material cycling within and below canopies. Thus, bark surfaces directly and indirectly influence the physical, chemical, and biological characteristics of water flowing through woody canopies (Ponette-González et al., 2020).
Water that drips from leaves, twigs, and branches (throughfall) and that flows down tree stems (stemflow) washes canopy surfaces, integrating deposition, retention, and leaching processes and the outcomes of bark-epiphyte interactions (Decina et al., 2020). As such, understanding the complete network of surfaces—including the non-leafy components—that links the top of the canopy to the soil is critical for a more complete and comprehensive view of how woody plants alter biogeochemical inputs to soils and the potential consequences for ecosystem functions, such as carbon and nutrient cycling (Van Stan et al., 2021a).
Bark exhibits a diverse array of physical and chemical properties that affect the chemistry and composition of waters draining over its surface (Oka et al., 2021). Importantly, bark can comprise a significant proportion of the total plant or ecosystem surface area available for passive interception and active exchange (i.e., uptake and leaching) of materials. Early estimates from temperate deciduous forest indicate that branch and stem bark surface areas combined range from 1.5 to 2.8 m2 per m2, while leaf surface area ranges from 3 to 6 m2 per m2 (Whittaker and Woodwell, 1967). Recent estimates from temperate evergreen coniferous forest dominated by redwood (Sequoia sempervirens) show exceptionally high and nearly equivalent bark and leaf surface areas (Sillett et al., 2019). In other words, as much as 30–50% of the total plant or ecosystem surface area exposed to the atmosphere (and precipitation) is bark. While the relative importance of the bark interface varies spatially due to species- and community-specific differences in outer surface areas, the bark interface varies temporally as well. The ratio of bark to leaf surfaces increases with tree age (Whittaker and Woodwell, 1967), during periods of leaf abscission, and after disturbances (e.g., hurricanes and insect outbreaks) that result in partial or complete canopy defoliation. Bark surfaces are also more temporally persistent than leaves (Van Stan et al., 2021a), meaning that they accumulate and exchange materials continuously over multiple seasons, years, and often over the entirety of a plant’s life.
Bark surfaces are rough, porous, hygroscopic (absorb and retain water), and sorptive (Supplementary Figure 1), characteristics that influence deposition, leaching, and retention and interactions with epiphytes. The sorptive properties of bark and its effectiveness at removing metal ions from aqueous solution has resulted in growing interest in using bark in water and wastewater treatment (Şen et al., 2015). The hygroscopicity of bark is of relevance as it represents a potentially significant component of total bark water storage. In temperate forests, water adsorbed from the atmosphere during dry periods can constitute 10–30% of maximum bark water storage capacity, with values exceeding 60% at humid forest sites (Ilek et al., 2016, 2021). These findings suggest that bark surfaces with lower hygroscopicity will retain more water during storms, increasing water residence time and opportunities for canopy exchange on bark. Surface roughness is another bark property affecting both bark water storage and dry deposition. As is the case with leaves and whole canopies (Rindy et al., 2019), increased roughness enhances particulate capture (Oka et al., 2021). Deposited particulates can wash off bark surfaces or accumulate within porous bark “traps” (Magyar et al., 2021; Supplementary Figure 1). Additionally, some tree species, such as paper birch (Betula papyrifera) and copperwood (Bursera simaruba), undergo periodic exfoliation. Bark shedding releases materials retained on and in bark tissues and leads to renewal of the bark surface. Finally, bark surfaces are diverse in chemical and elemental composition. Species differ in resource allocation to chemical defenses against insects, pests, and pathogens (e.g., Franceschi et al., 2005) and may translocate elements such as manganese to the bark to avoid toxic concentrations in leaves and other tissues (Hauck and Paul, 2005). Taken together, the structural heterogeneity and complex composition of bark give rise to unique associations with flora and fauna that in turn participate in material cycling and alteration of water quality.
The role and potential significance of the outer bark in atmosphere-water-vegetation interactions is often examined qualitatively or overlooked in field and modeling studies (Butler et al., 2020; Pace and Grote, 2020). In many fields, leaves still rule. As a result, the processes of deposition, retention, leaching, and washoff are relatively well described for leaves, but not so for bark. This precludes our ability to fully understand how woody plants influence the quality of water transported within (branch bark) and below (stem bark) tree canopies. In this mini-review, I briefly explore diverse perspectives and approaches to the study of bark and what they reveal about the myriad ways bark surfaces influence the chemistry and composition of sub-canopy precipitation.
Perspectives on Bark Are Diverse but Complementary
Bark interactions with the atmosphere, water, and vegetation have been the subject of research in the fields of environmental science, ecohydrology, epiphyte ecology, and microbiology for over five decades (e.g., Staxäng, 1969; Johnsen and Søchting, 1976; Farmer et al., 1991). A review of selected peer-reviewed literature indicates that within these fields bark is conceptualized as: (1) accumulator and biomonitor of atmospheric pollution; (2) transport surface; (3) substrate for epiphytic communities; and (4) reactor.
A Web of Science search for articles published between 1945 and present conducted with the terms “bark” and “biomonitoring” (n = 148), “bark” and “stemflow” (n = 122), and “bark” and “epiphytes” (n = 212) suggests that bark is most often considered in the context of plant ecology. Less than 10% of the articles identified in the “bark” and “epiphytes” group focused on microorganisms and animals. While the number of publications in all research areas has grown steadily since 1976 (Supplementary Figure 2), there are important differences in where, at what scale, and how bark is studied (Figure 1).
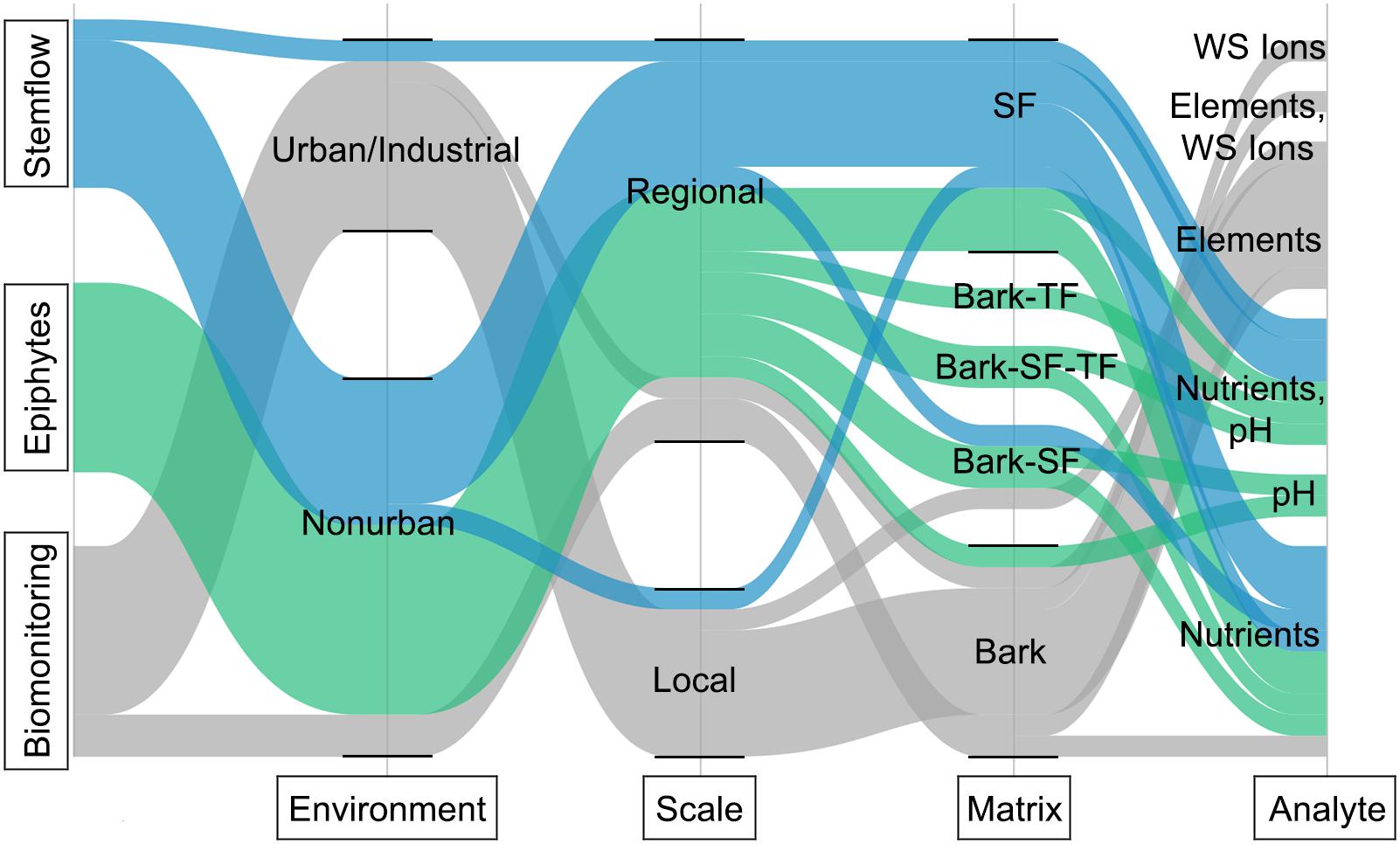
Figure 1. Parallel plot illustrating approaches to the study of bark in three research areas: bark and biomonitoring (n = 10 studies), bark and stemflow (n = 8 studies), and bark and epiphytes (n = 9 studies). The y-axis depicts the three research areas. The width of the parallel lines represents the frequency of studies falling in each category along the x-axis. The x-axis categories include the environment in which the study was conducted (urban/industrial or nonurban), the spatial scale at which the study was conducted (local or regional), the environmental matrix sampled [bark, stemflow (SF), and throughfall (TF)], and the dissolved or particulate analytes. Analytes include water-soluble ions (ws ions), trace and major elements (elements), dissolved and soluble nutrients (nutrients), and pH.
Bark biomonitoring often takes place within urban and industrial areas, where atmospheric pollution is a health and environmental concern. Bark is considered advantageous in biomonitoring research given its widespread distribution, accessibility, and capacity to intercept and retain pollutants. Indeed, bark is widely used to map and measure past and present impacts of airborne pollution downwind of point sources (e.g., incinerator and smelter; Cocozza et al., 2016) and near city and heavily trafficked roads (e.g., Catinon et al., 2012). The objective often is to monitor pollution changes over long-time frames or on local scales (e.g., Guéguen et al., 2012)—that is, within kilometers of major emissions sources. Most studies that utilize tree bark as a passive biomonitor determine trace element concentrations in bark tissue, with heavy metals such as lead, copper, cadmium, mercury, and uranium of particular interest due to their toxicity to human populations (e.g., Fujiwara et al., 2011; Chiarantini et al., 2016). For example, Flett et al. (2021) determined uranium concentrations in tree bark on tribal lands in the western United States and found that these were highest along an abandoned mine access road and near a mill where uranium was processed; concentrations decreased with increasing distance from pollution hotspots. The effectiveness of bark relative to other biomonitors such as leaves, lichens, and mosses has also been examined. Comparisons of multiple biomonitors consistently show that pollutant concentrations decrease in the order lichen/moss > bark > leaf surface > leaf wax (e.g., Cucu-Man and Steinnes, 2013).
Insights on how bark alters stemflow material inputs to soils generally derive from measurements conducted in non-urban forests. In this context, stemflow water is generally collected and analyzed for dissolved inorganic nutrients, organic carbon, organic nitrogen, and pH. While bark characteristics influence solute chemistry and fluxes, beyond the role of bark in altering stemflow water volumes, it is unclear how (Levia and Germer, 2015). In ecohydrological research, rarely are concentrations in bark leachate compared to those in stemflow (but see Tucker et al., 2020).
Ecologists and microbiologists frequently investigate bark-epiphyte interactions in non-urban forested environments. For example, in the northeastern United States, United Kingdom, and Sweden, pollution gradient studies underscore the effects of increasing pollutant deposition and acidification on bark substrates and epiphytic lichens and bryophytes along their length (e.g., Schmull et al., 2002; Mitchell et al., 2005; Fritz et al., 2009). In contrast to studies focused on epiphytic vegetation, we are only beginning to understand the role of bark and resident epiphytes as habitat for microorganisms and faunal communities (e.g., Aguirre-von-Wobeser, 2020). To understand the various factors influencing epiphytic microbial, plant, and animal communities, it is not uncommon for researchers to collect samples from diverse environmental matrices, including bark, precipitation (rainfall, throughfall, and stemflow), epiphytic tissue, and in some cases soils, for analysis of nutrients and pollutants (e.g., Farmer et al., 1991). Despite differences in scale, approach, and method, knowledge gained from all research areas elucidates how bark can influence biogeochemical cycling in woody ecosystems now and in the future.
Bark as Accumulator, Transporter, Substrate, and Reactor
Bark as Accumulator
Bark accumulates particulates from the atmosphere and plant canopies (Van Stan et al., 2021b), including organic matter (e.g., pollen and microbes), crustal matter (i.e., dust), and pollutants (e.g., heavy metals). It has been estimated that ∼80% of a tree’s bark surface deposit is organic matter, with the remaining 20% comprising similar amounts of crustal and anthropogenic particulates (Catinon et al., 2009).
Field and laboratory experiments, in some instances coupled with microscopy, demonstrate that particulate accumulation on bark surfaces is a highly complex process varying as a function of meteorological (e.g., rain), bark, and particle factors. In a series of sorption experiments, Su et al. (2013) found that spruce bark has a strong affinity for metals, such as iron, lead, copper, and cadmium, which explains why outer bark surfaces are typically enriched in these metals near pollution sources such as industrial plants and highways (Suzuki, 2006; Catinon et al., 2009). Less is known about the deposition of plant limiting nutrients, such as nitrogen, on tree bark, although bark may reflect broad-scale spatial gradients in N deposition (Boltersdorf et al., 2014). Accumulation of water-soluble ions can also be significant, representing ∼20% of the total particulate mass on bark surfaces (Xu et al., 2019). Proximity and location relative to pollution sources (e.g., downwind or facing) influence the composition of accumulated particles as well as their size. Near industrial pollution sources and roads, bark particulates are frequently <10 μm in diameter (Suzuki, 2006; Tye et al., 2006). In contrast, bark surfaces distant from these sources have been found to accumulate higher proportions of large (10–100 μm in diameter) particulates (Xu et al., 2019). Accumulation is also dependent on location within the bark (e.g., Chiarantini et al., 2016). Suberized cells within the outer bark tissue have been shown to preferentially accumulate elements derived from crustal and anthropogenic pollution sources (Catinon et al., 2011), whereas non-suberized cells are subject to the wear and tear of precipitation. Combining measurements of elemental composition in bark tissue, stemflow, and xylem sap, Catinon et al. (2012) showed that outer bark surface deposits are subject to intense washoff during storms. Taken together, these studies show that bark surface deposits are mixtures of materials whose composition reflects diverse sources and processes.
Bark as Transporter
Water travels over complex bark topography as it moves from the atmosphere to the ground below. Bark physical properties affect the volume and routing of water in ways that matter for material inputs to soil during precipitation (Levia and Herwitz, 2005). Given its effects on stemflow volume and water residence time, bark water storage capacity is of particular relevance.
Bark water storage capacity is significantly greater than that of foliage and can account for as much as 80% of total tree water storage (Herwitz, 1985), albeit the amount of water retained by bark varies considerably within and among species. Rough-barked species typically have higher water storage capacities than smooth-barked species (Levia and Herwitz, 2005) and therefore lower stemflow volumes (Ponette-González et al., 2010). High-resolution (0.1 mm vertical resolution) characterization of tree trunks reveals the influence of bark microrelief on intra- and inter-specific variability in water storage capacity. One study found that a pedunculate oak (Quercus robur) tree with highly ridged and steeply sloped bark could retain >2.5-fold more water than a smooth-barked European beech (Fagus sylvatica) tree of similar size (Van Stan et al., 2016). Bark water storage was recently shown to vary over meters distance along single tree stems (Sioma et al., 2018). In that study, four of five species sampled had higher bark water interception potential at 1.5 m compared to 15 m height due to a larger volume of water-accumulating space. Because bark water storage capacity is positively related to water residence time, higher storage results in increased chemical concentrations as well increased time available for exchange processes across and along the bark surface. Indeed, Oka et al. (2021) found that stemflow concentrations of calcium and potassium (ions easily leached from canopy surfaces; Ponette-González et al., 2016), increased along a gradient from smooth- to rough-barked species presumably as a result of longer water residence times. The research by Sioma et al. (2018) suggests that the lower sections of tree stems, where the downward transport of materials and higher water storage combine, could represent biogeochemical hotspots within tree canopies, providing a more nuanced explanation for high solute fluxes to near-stem soils during rainfall.
Measurements of stemflow (and throughfall) under tree canopies during leaf-on and leaf-off periods or along gradients of tree decay/death also demonstrate how bark surfaces modify water chemistry and composition (e.g., Siegert et al., 2018). In a Belgian oak forest, nitrate and ammonium concentrations were lower in stemflow compared to rainfall during the leaf-off season suggesting net uptake by bark surfaces (André et al., 2008). In an old-growth forest with varying levels of decay, stemflow collected under snags with little decay had higher calcium, potassium, and zinc concentrations compared to stemflow under live trees and snags with advanced decay. The pulse of elements with decay onset was attributed to release from decaying wood; release from deeper layers of bark and wood exposed with decay; or transport from outer wood to bark with stem evaporation (Bade et al., 2015).
Compared to bark effects on the downward flux of materials in stemflow, less is known about how the bark transport surface alters ionic and elemental exchanges horizontally across the bark membrane. Direct water (and nutrient) uptake through the bark may be more prevalent and significant than once thought (Berry et al., in press). Moving in the other direction, stem transpiration can result in calcium and potassium leaching from the xylem and subsequent re-deposition on the bark surface (Catinon et al., 2012). Despite knowledge limitations, bark appears to be a reactive substrate that exerts important controls over the materials transported by draining stormwater.
Bark as Substrate
Tree bark is a substrate whose physical and chemical properties affect epiphytic plant communities directly and indirectly through effects on branchflow and stemflow quality. Numerous studies highlight the importance of bark pH in epiphyte community composition, especially in polluted areas, where nitrogen and sulfur deposition can lead to bark acidification (e.g., Mitchell et al., 2005; Cleavitt et al., 2011). In these landscapes, both ammonium and nitrate have been associated with decreasing bryophyte and lichen cover (Schmull et al., 2002; Mitchell et al., 2005). Bark also represents a source of micronutrients, such as manganese, which is readily leached from bark into stemflow, but that can be toxic to epiphytes when supplied in excess (Hauck and Paul, 2005). Intraspecific changes in bark properties and associated nutrient gradients that occur with tree age represent an additional, though less well studied, control on epiphyte communities. For example, cation leaching from damaged areas on older trees may increase stemflow pH, thereby providing microhabitats for epiphytes of conservation value (Fritz et al., 2009). McGee et al. (2019) demonstrated that bark substrates become enriched in nutrients as tree bark thickness increases with tree size and age, and that enrichment correlates positively with the cover of several mesophytic and calciphilic epiphytes. In turn, epiphytes alter the chemistry and composition of draining waters. The magnitude and extent of chemical alteration is beyond the scope of this mini-review but the subject of an extensive review by Van Stan and Pypker (2015).
Bark as Reactor
Compared to research on interactions between bark and epiphytic vegetation, we are only beginning to understand the role of bark and resident epiphytes as habitat for microorganisms and faunal communities (e.g., Aguirre-von-Wobeser, 2020). Bark contains high levels of microbial diversity (Lambais et al., 2014), a diversity that is often intermediate between, and distinctive in composition from, adjacent leaves and soils (Leff et al., 2015). Bark microbial communities also have been shown to vary by season (Beck et al., 2014) and spatially along bark surfaces (Leff et al., 2015). For instance, Leff et al. (2015) found higher microbial diversity near the interior and on the underside of branches. Such variations in diversity and composition are attributable to micro-environmental differences in UV radiation exposure, water, nutrient and carbon availability, as well as the presence of antimicrobial compounds (e.g., Magyar et al., 2021).
What functions do bark-dwelling microbes and fauna perform? Increasing evidence highlights the important roles bark bacterial communities play in the cycling of carbon and nitrogen (Jeffrey et al., 2021). Abundant photosynthetic genes identified in microbes sampled from bark tissues indicate the potential for bacterial primary production on bark surfaces that could help sustain heterotrophic bacteria (Aguirre-von-Wobeser, 2020). In turn, heterotrophic bacterial and fungal communities associated with decaying wood and bark (Martins et al., 2013) decompose complex carbon compounds. The potential for methane consumption by bark-dwelling methane-oxidizing bacteria was recently demonstrated by Jeffrey et al. (2021), illustrating yet another pathway by which bark bacterial communities influence carbon cycling. Some bacteria and lichens also add nitrogen to canopies through nitrogen fixation (Aguirre-von-Wobeser, 2020). Although less well studied, faunal communities within bark epiphytes (e.g., foliose lichens) and on bark surfaces make up part of a complex and rich bark food web that remains poorly understood (Anderson, 2014; Asplund et al., 2018). In sum, although nascent, this research points to the potential for significant transfers of microorganismal and faunal biomass via throughfall and stemflow to the ground below (Guidone et al., 2021; Magyar et al., 2021) along with important fluxes of dissolved and suspended materials.
Future Directions
Bark is a passive accumulator, an active transport surface, a substrate, and a reactor. Although bark has been shown to accumulate considerable amounts of particulates, the contribution of bark to total nutrient and pollutant loading has not been quantified. Further, we know little about exchanges of both water, nutrients, and pollutants across tree branch and stem surfaces. In the future, changes in atmospheric composition, precipitation, and disturbance regimes will alter what is deposited to bark surfaces, as well as woody plant species composition and species’ expression of bark. The latter may occur via changes in the age structure of stands or intraspecific variation in bark structure and chemistry. In sum, the interaction of bark and stormy conditions may represent a critical influence on the accumulation, exchange and transport of elements between atmosphere, water, and vegetation.
Author Contributions
AGP-G conceived the mini-review, reviewed the literature, created the figures, and wrote the manuscript.
Funding
Partial funding for manuscript publication fees was provided through a Frontiers fee support grant.
Conflict of Interest
The author declares that the research was conducted in the absence of any commercial or financial relationships that could be construed as a potential conflict of interest.
Publisher’s Note
All claims expressed in this article are solely those of the authors and do not necessarily represent those of their affiliated organizations, or those of the publisher, the editors and the reviewers. Any product that may be evaluated in this article, or claim that may be made by its manufacturer, is not guaranteed or endorsed by the publisher.
Acknowledgments
I would like to acknowledge John Van Stan II for constructive comments on two versions of this manuscript and my three hound dogs for inspiring me with every bark.
Supplementary Material
The Supplementary Material for this article can be found online at: https://www.frontiersin.org/articles/10.3389/ffgc.2021.716557/full#supplementary-material
References
Aguirre-von-Wobeser, E. (2020). Functional metagenomics of bark microbial communities from avocado trees (Persea americana Mill.) reveals potential for bacterial primary productivity. bioRxiv [Preprint] doi: 10.1101/2020.09.05.284570
Anderson, O. R. (2014). Microbial communities associated with tree bark foliose lichens: a perspective on their microecology. J. Eukaryot. Microbiol. 61, 364–370. doi: 10.1111/jeu.12116
André, F., Jonard, M., and Ponette, Q. (2008). Effects of biological and meteorological factors on stemflow chemistry within a temperate mixed oak–beech stand. Sci. Total Environ. 393, 72–83. doi: 10.1016/j.scitotenv.2007.12.002
Asplund, J., Strandin, O. V., and Gauslaa, Y. (2018). Gastropod grazing of epiphytic lichen-dominated communities depends on tree species. Basic Appl. Ecol. 32, 96–102. doi: 10.1016/j.baae.2018.07.007
Bade, C., Jacob, M., Leuschner, C., and Hauck, M. (2015). Chemical properties of decaying wood in an old-growth spruce forest and effects on soil chemistry. Biogeochemistry 122, 1–13. doi: 10.1007/s10533-014-0015-x
Beck, A., Peršoh, D., and Rambold, G. (2014). First evidence for seasonal fluctuations in lichen-and bark-colonising fungal communities. Folia Microbiol. 59, 155–157. doi: 10.1007/s12223-013-0278-y
Berry, Z. C., Ávila-Lovera, E., De Guzman, M. E., O’Keefe, K., and Emery, N. C. (in press). Beneath the bark: assessing woody stem water and carbon fluxes and its prevalence across climates and the woody plant phylogeny. Front. For. Glob. Chang.
Boltersdorf, S. H., Pesch, R., and Werner, W. (2014). Comparative use of lichens, mosses and tree bark to evaluate nitrogen deposition in Germany. Environ. Pollut. 189, 43–53. doi: 10.1016/j.envpol.2014.02.017
Butler, E. E., Chen, M., Ricciuto, D., Flores-Moreno, H., Wythers, K. R., Kattge, J., et al. (2020). Seeing the canopy for the branches: Improved within canopy scaling of leaf nitrogen. J. Adv. Model. Earth Syst. 12:e2020MS002237.
Catinon, M., Ayrault, S., Boudouma, O., Asta, J., Tissut, M., and Ravanel, P. (2012). Atmospheric element deposit on tree barks: the opposite effects of rain and transpiration. Ecol. Indic. 14, 170–177. doi: 10.1016/j.ecolind.2011.07.013
Catinon, M., Ayrault, S., Clocchiatti, R., Boudouma, O., Asta, J., Tissut, M., et al. (2009). The anthropogenic atmospheric elements fraction: a new interpretation of elemental deposits on tree barks. Atmos. Environ. 43, 1124–1130. doi: 10.1016/j.atmosenv.2008.11.004
Catinon, M., Ayrault, S., Spadini, L., Boudouma, O., Asta, J., Tissut, M., et al. (2011). Tree bark suber-included particles: a long-term accumulation site for elements of atmospheric origin. Atmos. Environ. 45, 1102–1109. doi: 10.1016/j.atmosenv.2010.11.038
Chiarantini, L., Rimondi, V., Benvenuti, M., Beutel, M. W., Costagliola, P., Gonnelli, C., et al. (2016). Black pine (Pinus nigra) barks as biomonitors of airborne mercury pollution. Sci. Total Environ. 569, 105–113. doi: 10.1016/j.scitotenv.2016.06.029
Cleavitt, N. L., Ewing, H. A., Weathers, K. C., and Lindsey, A. M. (2011). Acidic atmospheric deposition interacts with tree type and impacts the cryptogamic epiphytes in Acadia National Park Maine, USA. Bryologist 114, 570–582. doi: 10.1639/0007-2745-114.3.570
Cocozza, C., Ravera, S., Cherubini, P., Lombardi, F., Marchetti, M., and Tognetti, R. (2016). Integrated biomonitoring of airborne pollutants over space and time using tree rings, bark, leaves and epiphytic lichens. Urban For. Urban Green. 17, 177–191. doi: 10.1016/j.ufug.2016.04.008
Cucu-Man, S.-M., and Steinnes, E. (2013). Analysis of selected biomonitors to evaluate the suitability for their complementary use in monitoring trace element atmospheric deposition. Environ. Monit. Assess. 185, 7775–7791. doi: 10.1007/s10661-013-3135-1
Decina, S. M., Ponette-González, A. G., and Rindy, J. E. (2020). “Urban tree canopy effects on water quality via inputs to the urban ground surface,” in Forest-Water Interactions, eds D. Levia, D. Carlyle-Moses, S. Iida, B. Michalzik, K. Nanko, and A. Tischer (Chem: Springer), 433–457. doi: 10.1007/978-3-030-26086-6_18
Farmer, A. M., Bates, J. W., and Bell, J. N. B. (1991). Seasonal variations in acidic pollutant inputs and their effects on the chemistry of stemflow, bark and epiphyte tissues in three oak∗ woodlands in NW Britain. New Phytol. 118, 441–451. doi: 10.1111/j.1469-8137.1991.tb00026.x
Flett, L., McLeod, C. L., McCarty, J. L., Shaulis, B. J., Fain, J. J., and Krekeler, M. P. S. (2021). Monitoring uranium mine pollution on native american lands: insights from tree bark particulate matter on the spokane reservation, Washington, USA. Environ. Res. 194:110619. doi: 10.1016/j.envres.2020.110619
Franceschi, V. R., Krokene, P., Christiansen, E., and Krekling, T. (2005). Anatomical and chemical defenses of conifer bark against bark beetles and other pests. New Phytol. 167, 353–376. doi: 10.1111/j.1469-8137.2005.01436.x
Fritz, Ö, Brunet, J., and Caldiz, M. (2009). Interacting effects of tree characteristics on the occurrence of rare epiphytes in a Swedish beech forest area. Bryologist 112, 488–505. doi: 10.1639/0007-2745-112.3.488
Fujiwara, F. G., Gómez, D. R., Dawidowski, L., Perelman, P., and Faggi, A. (2011). Metals associated with airborne particulate matter in road dust and tree bark collected in a megacity (Buenos Aires, Argentina). Ecol. Indic. 11, 240–247. doi: 10.1016/j.ecolind.2010.04.007
Guéguen, F., Stille, P., Geagea, M. L., and Boutin, R. (2012). Atmospheric pollution in an urban environment by tree bark biomonitoring–Part I: Trace element analysis. Chemosphere 86, 1013–1019. doi: 10.1016/j.chemosphere.2011.11.040
Guidone, M., Gordon, D. A., and Van Stan, J. T. (2021). Living particulate fluxes in throughfall and stemflow during a pollen event. Biogeochemistry 153, 323–330. doi: 10.1007/s10533-021-00787-7
Hauck, M., and Paul, A. (2005). Manganese as a site factor for epiphytic lichens. Lichenol. 37, 409–423. doi: 10.1017/s0024282905014933
Herwitz, S. R. (1985). Interception storage capacities of tropical rainforest canopy trees. J. Hydrol. 77, 237–252. doi: 10.1016/0022-1694(85)90209-4
Ilek, A., Kucza, J., and Morkisz, K. (2016). Hygroscopicity of the bark of selected forest tree species. iForest-Biogeosciences For. 10:220. doi: 10.3832/ifor1979-009
Ilek, A., Siegert, C. M., and Wade, A. (2021). Hygroscopic contributions to bark water storage and controls exerted by internal bark structure over water vapor absorption. Trees 35, 831–843. doi: 10.1007/s00468-021-02084-0
Jeffrey, L. C., Maher, D. T., Chiri, E., Leung, P. M., Nauer, P. A., Arndt, S. K., et al. (2021). Bark-dwelling methanotrophic bacteria decrease methane emissions from trees. Nat. Commun. 12:2127.
Johnsen, I., and Søchting, U. (1976). Distribution of cryptogamic epiphytes in a Danish city in relation to air pollution and bark properties. Bryologist 79, 86–92. doi: 10.2307/3241873
Lambais, M. R., Lucheta, A. R., and Crowley, D. E. (2014). Bacterial community assemblages associated with the phyllosphere, dermosphere, and rhizosphere of tree species of the Atlantic forest are host taxon dependent. Microb. Ecol. 68, 567–574. doi: 10.1007/s00248-014-0433-2
Leff, J. W., Del Tredici, P., Friedman, W. E., and Fierer, N. (2015). Spatial structuring of bacterial communities within individual G inkgo biloba trees. Environ. Microbiol. 17, 2352–2361. doi: 10.1111/1462-2920.12695
Levia, D. F., and Germer, S. (2015). A review of stemflow generation dynamics and stemflow-environment interactions in forests and shrublands. Rev. Geophys. 53, 673–714. doi: 10.1002/2015rg000479
Levia, D. F., and Herwitz, S. R. (2005). Interspecific variation of bark water storage capacity of three deciduous tree species in relation to stemflow yield and solute flux to forest soils. Catena 64, 117–137. doi: 10.1016/j.catena.2005.08.001
Magyar, D., Van Stan, J. T., and Sridhar, K. R. (2021). Hypothesis and theory: fungal spores in stemflow and potential bark sources. Front. For. Glob. Chang. 4, 1–18.
Martins, G., Lauga, B., Miot-Sertier, C., Mercier, A., Lonvaud, A., Soulas, M.-L., et al. (2013). Characterization of epiphytic bacterial communities from grapes, leaves, bark and soil of grapevine plants grown, and their relations. PLoS One 8:e73013. doi: 10.1371/journal.pone.0073013
McGee, G. G., Cardon, M. E., and Kiernan, D. H. (2019). Variation in Acer saccharum Marshall (sugar maple) bark and stemflow characteristics: implications for epiphytic bryophyte communities. Northeast. Nat. 26, 214–235. doi: 10.1656/045.026.0118
Mendieta-Leiva, G., Porada, P., and Bader, M. Y. (2020). “Interactions of epiphytes with precipitation partitioning,” in Precipitation Partitioning by Vegetation, eds J. T. Van Stan, E. Gutmann, and J. Friesen (Cham: Springer), 133–146. doi: 10.1007/978-3-030-29702-2_9
Mitchell, R. J., Truscot, A. M., Leith, I. D., Cape, J. N., Van Dijk, N., Tang, Y. S., et al. (2005). A study of the epiphytic communities of Atlantic oak woods along an atmospheric nitrogen deposition gradient. J. Ecol. 93, 482–492. doi: 10.1111/j.1365-2745.2005.00967.x
Oka, A., Takahashi, J., Endoh, Y., and Seino, T. (2021). Bark effects on stemflow chemistry in a Japanese temperate forest I. The role of bark surface morphology. Front. For. Glob. Chang. 4:654375. doi: 10.3389/ffgc.2021.654375
Pace, R., and Grote, R. (2020). Deposition and resuspension mechanisms into and from tree canopies: a study modeling particle removal of conifers and broadleaves in different cities. Front. For. Glob. Chang. 3:26. doi: 10.3389/ffgc.2020.00026
Ponette-González, A. G., Ewing, H. A., and Weathers, K. C. (2016). “Interactions between precipitation and vegetation canopies,” in A Biogeoscience Approach to Ecosystems, eds E. A. Johnson and Y. Martin (New York, NY: Cambridge University Press), 215–253. doi: 10.1017/cbo9781107110632.009
Ponette-González, A. G., Van Stan, J. T. II, and Magyar, D. (2020). “Things seen and unseen in throughfall and stemflow,” in Precipitation Partitioning by Vegetation, eds J. T. Van Stan, II, E. Gutmann, and J. Friesen (Cham: Springer), 71–88. doi: 10.1007/978-3-030-29702-2_5
Ponette-González, A. G., Weathers, K. C., and Curran, L. M. (2010). Water inputs across a tropical montane landscape in Veracruz, Mexico: synergistic effects of land cover, rain and fog seasonality, and interannual precipitation variability. Glob. Chang. Biol. 16, 946–963. doi: 10.1111/j.1365-2486.2009.01985.x
Rindy, J. E., Ponette-González, A. G., Barrett, T. E., Sheesley, R. J., and Weathers, K. C. (2019). Urban trees are sinks for soot: elemental carbon accumulation by two widespread oak species. Environ. Sci. Technol. 53, 10092–10101. doi: 10.1021/acs.est.9b02844
Schmull, M., Hauck, M., Vann, D. R., Johnson, A. H., and Runge, M. (2002). Site factors determining epiphytic lichen distribution in a dieback-affected spruce-fir forest on Whiteface Mountain, New York: stemflow chemistry. Can. J. Bot. 80, 1131–1140. doi: 10.1139/b02-106
Şen, A., Pereira, H., Olivella, M. A., and Villaescusa, I. (2015). Heavy metals removal in aqueous environments using bark as a biosorbent. Int. J. Environ. Sci. Technol. 12, 391–404. doi: 10.1007/s13762-014-0525-z
Siegert, C. M., Renninger, H. J., Sasith Karunarathna, A. A., Riggins, J. J., Clay, N. A., Tang, J. D., et al. (2018). “Biogeochemical hotspots around bark-beetle killed trees,” in Proceedings of the 19th Biennial Southern Silvicultural Research Conference, Washington, DC.
Sillett, S. C., Van Pelt, R., Carroll, A. L., Campbell-Spickler, J., Coonen, E. J., and Iberle, B. (2019). Allometric equations for Sequoia sempervirens in forests of different ages. For. Ecol. Manage. 433, 349–363. doi: 10.1016/j.foreco.2018.11.016
Sioma, A., Socha, J., and Klamerus-Iwan, A. (2018). A new method for characterizing bark microrelief using 3D vision systems. Forests 9:30. doi: 10.3390/f9010030
Staxäng, B. (1969). Acidification of bark of some deciduous trees. Oikos 20, 224–230. doi: 10.2307/3543190
Su, P., Granholm, K., Pranovich, A., Harju, L., Holmbom, B., and Ivaska, A. (2013). Sorption of metal ions from aqueous solution to spruce bark. Wood Sci. Technol. 47, 1083–1097. doi: 10.1007/s00226-013-0562-7
Suzuki, K. (2006). Characterisation of airborne particulates and associated trace metals deposited on tree bark by ICP-OES, ICP-MS, SEM-EDX and laser ablation ICP-MS. Atmos. Environ. 40, 2626–2634. doi: 10.1016/j.atmosenv.2005.12.022
Tucker, A., Levia, D. F., Katul, G. G., Nanko, K., and Rossi, L. F. (2020). A network model for stemflow solute transport. Appl. Math. Model. 88, 266–282. doi: 10.1016/j.apm.2020.06.047
Tye, A. M., Hodgkinson, E. S., and Rawlins, B. G. (2006). Microscopic and chemical studies of metal particulates in tree bark and attic dust: evidence for historical atmospheric smelter emissions, Humberside, UK. J. Environ. Monit. 8, 904–912. doi: 10.1039/b605729b
Van Stan, J. T. II, and Pypker, T. G. (2015). A review and evaluation of forest canopy epiphyte roles in the partitioning and chemical alteration of precipitation. Sci. Total Environ. 536, 813–824. doi: 10.1016/j.scitotenv.2015.07.134
Van Stan, J. T., Dymond, S. F., and Klamerus-Iwan, A. (2021a). Bark-water interactions across ecosystem states and fluxes. Front. For. Glob. Chang. 4:28.
Van Stan, J. T., Lewis, E. S., Hildebrandt, A., Rebmann, C., and Friesen, J. (2016). Impact of interacting bark structure and rainfall conditions on stemflow variability in a temperate beech-oak forest, central Germany. Hydrol. Sci. J. 61, 2071–2083. doi: 10.1080/02626667.2015.1083104
Van Stan, J. T., Ponette-González, A. G., Swanson, T., and Weathers, K. C. (2021b). Throughfall and stemflow are major hydrologic highways for particulate traffic through tree canopies. Front. Ecol. Environ (in press). doi: 10.1002/fee.2360
Whittaker, R. H., and Woodwell, G. M. (1967). Surface area relations of woody plants and forest communities. Am. J. Bot. 54, 931–939. doi: 10.1002/j.1537-2197.1967.tb10717.x
Keywords: biomonitoring, epiphytes, forests, microorganisms, stemflow, throughfall, woody ecosystems
Citation: Ponette-González AG (2021) Accumulator, Transporter, Substrate, and Reactor: Multidimensional Perspectives and Approaches to the Study of Bark. Front. For. Glob. Change 4:716557. doi: 10.3389/ffgc.2021.716557
Received: 28 May 2021; Accepted: 16 July 2021;
Published: 05 August 2021.
Edited by:
Anna Klamerus-Iwan, University of Agriculture in Krakow, PolandReviewed by:
Beate Michalzik, Friedrich Schiller University Jena, GermanyDawid Kupka, University of Agriculture in Krakow, Poland
Bernard Okoński, Poznań University of Life Sciences, Poland
Copyright © 2021 Ponette-González. This is an open-access article distributed under the terms of the Creative Commons Attribution License (CC BY). The use, distribution or reproduction in other forums is permitted, provided the original author(s) and the copyright owner(s) are credited and that the original publication in this journal is cited, in accordance with accepted academic practice. No use, distribution or reproduction is permitted which does not comply with these terms.
*Correspondence: Alexandra G. Ponette-González, YWxleGFuZHJhLnBvbmV0dGVAdW50LmVkdQ==