- 1Department of Ecosystem Science and Sustainability, Warner College of Natural Resources, Colorado State University, Fort Collins, CO, United States
- 2Smithsonian Tropical Research Institute, Balboa, Panama
- 3CSIR-Forestry Research Institute of Ghana, KNUST, Kumasi, Ghana
- 4Environmental Sciences Division, Climate Change Sciences Institute, Oak Ridge National Laboratory, Oak Ridge, TN, United States
- 5Asian School of the Environment, Nanyang Technological University, Singapore, Singapore
- 6IFREMER, Laboratoire Environnement et Ressources des Pertuis Charentais (LER-PC), La Tremblade, France
- 7School of Geography, Earth, and Environmental Sciences, University of Birmingham, Birmingham, United Kingdom
- 8Cary Institute of Ecosystem Studies, Millbrook, NY, United States
- 9School of Geography, University of Leeds, Leeds, United Kingdom
- 10Department of Natural Sciences, Manchester Metropolitan University, Manchester, United Kingdom
- 11Department of Geography, UCLA, Los Angeles, CA, United States
- 12Department of Biology, Bieler School of Environment, McGill University, Montreal, QC, Canada
- 13Jardín Botánico Francisco Javier Clavijero, Instituto de Ecología, Xalapa, Mexico
- 14Schmid College of Science and Technology, Chapman University, Orange, CA, United States
- 15Joint Institute for Regional Earth System Science and Engineering, University of California, Los Angeles, Los Angeles, CA, United States
- 16Department Biogeochemical Signals, Max-Planck-Institute for Biogeochemistry, Jena, Germany
- 17AMAP (botAnique et Modélisation de l’Architecture des Plantes et des Végétations), Université de Montpellier, CIRAD, CNRS, INRAE, IRD, Montpellier, France
- 18Centre of Microbiology and Environmental Systems Science, University of Vienna, Vienna, Austria
- 19Biodiversity, Macroecology, and Biogeography, Faculty of Forest Sciences and Forest Ecology, University of Göttingen, Göttingen, Germany
- 20Plant Ecology and Ecosystems Research, Albrecht von Haller Institute for Plant Sciences, University of Göttingen, Göttingen, Germany
- 21Coordination of Environmental Dynamics, National Institute of Amazonian Research, Manaus, Brazil
- 22Center of Applied Ecology and Sustainability, Pontificia Universidad Católica de Chile, Santiago, Chile
- 23Institute of Botany, The Czech Academy of Sciences, Prùhonice, Czechia
- 24Department of Ecology and Evolutionary Biology, Brown University, Providence, RI, United States
- 25Department of Ecology and Environmental Science, Umeå University, Umeå, Sweden
- 26Department of Ecology and Evolutionary Biology, University of Tennessee, Knoxville, Knoxville, TN, United States
- 27Department of Plant Biology, Institute of Biology, University of Campinas – UNICAMP, Campinas, Brazil
- 28Department of Plant and Microbial Biology, University of Minnesota, St. Paul, MN, United States
- 29Department of Ecology, Evolution, and Behavior, University of Minnesota, St. Paul, MN, United States
- 30School of Life Sciences Weihenstephan, Technical University of Munich, Freising, Germany
- 31Department of Ecology, Evolution and Environmental Biology, Columbia University, New York, NY, United States
- 32Department of Ecology and Evolutionary Biology, University of Michigan, Ann Arbor, MI, United States
- 33Department of Biological Sciences, Institute of Environment, International Center of Tropical Biodiversity, Florida International University, Miami, FL, United States
- 34Lyon Arboretum, University of Hawaii at Mânoa, Honolulu, HI, United States
Vegetation processes are fundamentally limited by nutrient and water availability, the uptake of which is mediated by plant roots in terrestrial ecosystems. While tropical forests play a central role in global water, carbon, and nutrient cycling, we know very little about tradeoffs and synergies in root traits that respond to resource scarcity. Tropical trees face a unique set of resource limitations, with rock-derived nutrients and moisture seasonality governing many ecosystem functions, and nutrient versus water availability often separated spatially and temporally. Root traits that characterize biomass, depth distributions, production and phenology, morphology, physiology, chemistry, and symbiotic relationships can be predictive of plants’ capacities to access and acquire nutrients and water, with links to aboveground processes like transpiration, wood productivity, and leaf phenology. In this review, we identify an emerging trend in the literature that tropical fine root biomass and production in surface soils are greatest in infertile or sufficiently moist soils. We also identify interesting paradoxes in tropical forest root responses to changing resources that merit further exploration. For example, specific root length, which typically increases under resource scarcity to expand the volume of soil explored, instead can increase with greater base cation availability, both across natural tropical forest gradients and in fertilization experiments. Also, nutrient additions, rather than reducing mycorrhizal colonization of fine roots as might be expected, increased colonization rates under scenarios of water scarcity in some forests. Efforts to include fine root traits and functions in vegetation models have grown more sophisticated over time, yet there is a disconnect between the emphasis in models characterizing nutrient and water uptake rates and carbon costs versus the emphasis in field experiments on measuring root biomass, production, and morphology in response to changes in resource availability. Closer integration of field and modeling efforts could connect mechanistic investigation of fine-root dynamics to ecosystem-scale understanding of nutrient and water cycling, allowing us to better predict tropical forest-climate feedbacks.
Introduction: Tropical Root Acquisition of Nutrients and Water
Tropical forests play a dominant role in regulating global water, nutrient, and carbon (C) cycles (Field et al., 1998; Jobbagy and Jackson, 2000; Cleveland et al., 2011), and fine root biomass, production, and uptake activity mediate all of these biogeochemical cycles. Fine roots in tropical forests are responsible for acquisition of water and nutrients under very different climatic and edaphic conditions compared to temperate forests, where most of our knowledge on root traits and function originates. First, seasonality in tropical forests is typically dictated by changes in rainfall rather than temperature. Second, deep, clay-rich, strongly weathered soils scarce in rock-derived nutrients are dominant in >50% of tropical forests (Holzman, 2008), unlike most temperate forests. Thus, surface soil water availability is often scarce in tropical forests for parts of the year and must be sourced from deeper soil (Davidson et al., 2011), while available nutrients accumulate in the seasonally dry surface soils (Cornejo et al., 1994), creating temporal and spatial separation between water and nutrient availability. Tropical forests rely on fine root adaptations to overcome these resource scarcities to support some of the greatest annual primary productivity rates on Earth (Field et al., 1998). Vegetation models increasingly include the complexity and functionality of water, nitrogen (N), and phosphorus (P) availability in order to predict responses of tropical forests to global change (Fleischer et al., 2019). Nonetheless, in vegetation models, tradeoffs and synergies in root traits for water uptake and nutrient acquisition in tropical forests are largely unknown, or continue to be derived from models based on temperate forest observations. A better understanding of how commonly measured tropical root traits relate to nutrient and water uptake and plant function in tropical forests could help us improve predictive models of forest-climate feedbacks for tropical biomes.
One of the largest challenges facing tropical forests is chronic atmospheric drying and increased severity and frequency of episodic drought as a result of climate change. These drying trends have already been documented in some tropical regions (Aleixo et al., 2019; Powers et al., 2020) and are predicted to be the primary manifestations of climate change for the majority of tropical regions (Kharin et al., 2007; Feng et al., 2013; Magrin et al., 2014; Duffy et al., 2015; Chadwick et al., 2016; Barkhordarian et al., 2019), with likely catastrophic effects for tropical forest persistence and function in some areas (Brodribb et al., 2020). Extreme droughts are predicted to shift tropical plant community assemblages and select for drought tolerant plant functional types, suppress net primary production (NPP), increase plant mortality, and reduce ecosystem C storage (Nepstad et al., 2002, 2007; da-Costa et al., 2010; Doughty et al., 2015; Cusack et al., 2016; Feldpausch et al., 2016; Aguirre-Gutierrez et al., 2019). For example, in areas of the Amazon, dry-adapted species are increasing in abundance (Esquivel-Muelbert et al., 2019). However, the high diversity of plant hydraulic strategies, as well as niche partitioning belowground could help mitigate the negative effects of drought on net water and nutrient uptake at the ecosystem level, as shown in some Amazon forests (Chitra-Tarak et al., 2018; Brum et al., 2019; Janssen et al., 2020; Oliveira et al., 2021). Belowground niche partitioning among plant functional types and taxonomic units is still poorly characterized in most tropical forests, and should be investigated through soil profiles vertically, laterally, and temporally. Understanding the mechanisms and capacity for tropical forests to overcome increasingly scarce resources through diverse root acquisition strategies will help us better predict broad-scale responses of tropical forests to expected climate change.
Nutrient and water acquisition occur primarily through fine roots (roots < 2 mm diameter), particularly by absorptive roots, which are the most distal orders of fine root systems (typically first- to third-order roots) (McCormack et al., 2015). The production, turnover, and physiological activity of fine roots therefore mediate nutrient, water, and carbon exchanges among soils, plants, and the atmosphere (Bardgett et al., 2014). To overcome resource scarcity, plants can dynamically allocate assimilated C to biomass growth above- or belowground, including C allocation to microbial symbionts that assist with resource acquisition (Davidson, 1969; Thornley, 1972; Bloom et al., 1985). For example, when water or nutrients are relatively more limiting to growth than aboveground resources (e.g., light), plants can increase the relative allocation of C to root biomass, increasing root:shoot ratios (Brouwer, 1963; Shipley and Meziane, 2002; Roa-Fuentes et al., 2021). Under these conditions, plants tend to express fine root morphological, physiological, and phenological traits that maximize resource acquisition (Violle et al., 2007).
Many fine root traits assist with multiple functions, such that a single trait can contribute to water and nutrient acquisition (Comas et al., 2013; Freschet et al., 2021). For example, specific root length (SRL, or length per mass), fine root biomass depth distribution, root elongation rate, root branching density, and mycorrhizal colonization intensity are all traits that can adjust to multiple resource scarcities (Kong et al., 2014). Other root traits are associated more specifically with acquisition of only one resource, like root nodulation to host symbiotic N-fixing microbes for N acquisition, root phosphatase production for P acquisition, or hydraulic conductance and cortical thickness for water acquisition (Lambers et al., 2008). Thus, root physiology and symbiotic associations can be more targeted at the acquisition of a particular resource. For fine root traits that are associated with the acquisition of multiple resources, the responses to water versus nutrient scarcity can differ, which might lead to tradeoffs among traits expressed, and spatial and temporal offsets in resource availability versus plant growth. For example, root biomass depth distributions and productivity through the soil profile, which are traits of overall root architecture and phenology, could be concentrated in surface soils for nutrient acquisition, or could shift to deeper soil for water acquisition (at the expense of nutrient acquisition) during dry periods.
Key uncertainties exist surrounding model representations of tropical plant biomass allocation belowground, root depth distributions, and responses to water and nutrient limitation (Fisher et al., 2012; Smithwick et al., 2014; Warren et al., 2015; Fleischer et al., 2019). While recent progress has been made to improve water, nutrient, and C cycling in vegetation and nutrient uptake models (Zhu et al., 2019; Fisher and Koven, 2020), realistic representations of drying and drought effects on belowground C allocation and water sourcing in diverse tropical forests are challenging (Ciais et al., 2013; Todd-Brown et al., 2014; Tang and Riley, 2021). Trait-based modeling, which relies on plant parameters measured in the field, is increasingly being explored as an alternative to empirical parameters to constrain model uncertainty and improve projections (Xu and Trugman, 2021). Since different resource acquisition strategies exist, which presumably have varying costs and benefits for plants, parameterization and representation of tropical forest nutrient and water uptake require empirical, process-based understanding from field research (Zhu et al., 2019; Allen et al., 2020). A recent surge in field experiments and observations of tropical root responses to changing soil resource conditions could help address these modeling needs.
Here, we synthesize the available data on tropical forest root traits linked to nutrient and water acquisition, assess variation across resource gradients, and based on these patterns, make inferences about how fine root traits might respond to predicted changes in resource availability. We first give sections “Methods” and “Background”, and then explore “Fine Root Traits Linked to Nutrient and Water Acquisition in Tropical Forests.” Within this section we review commonly measured fine root traits and dynamics that are linked to water and nutrient acquisition (Figure 1), including: (1) fine root biomass stocks and depth distributions; (2) fine root dynamics, including patterns in production, mortality, turnover, and phenology; (3) fine root morphological traits; (4) fine root physiological traits; (5) fine root symbiotic associations (i.e., “symbiotic traits”), including mycorrhizas and N-fixing microbes. We then assess “Representation of Root Nutrient and Water Acquisition in Terrestrial Vegetation Models,” assessing advances to improve the parameterization and representation of fine root dynamics for nutrient and water acquisition, particularly for tropical forests. Finally, we suggest section “Frontiers in Tropical Root Acquisition Research” for research and data-model integration to improve our understanding of how tropical forest fine roots mediate and respond to changes in resource availability.
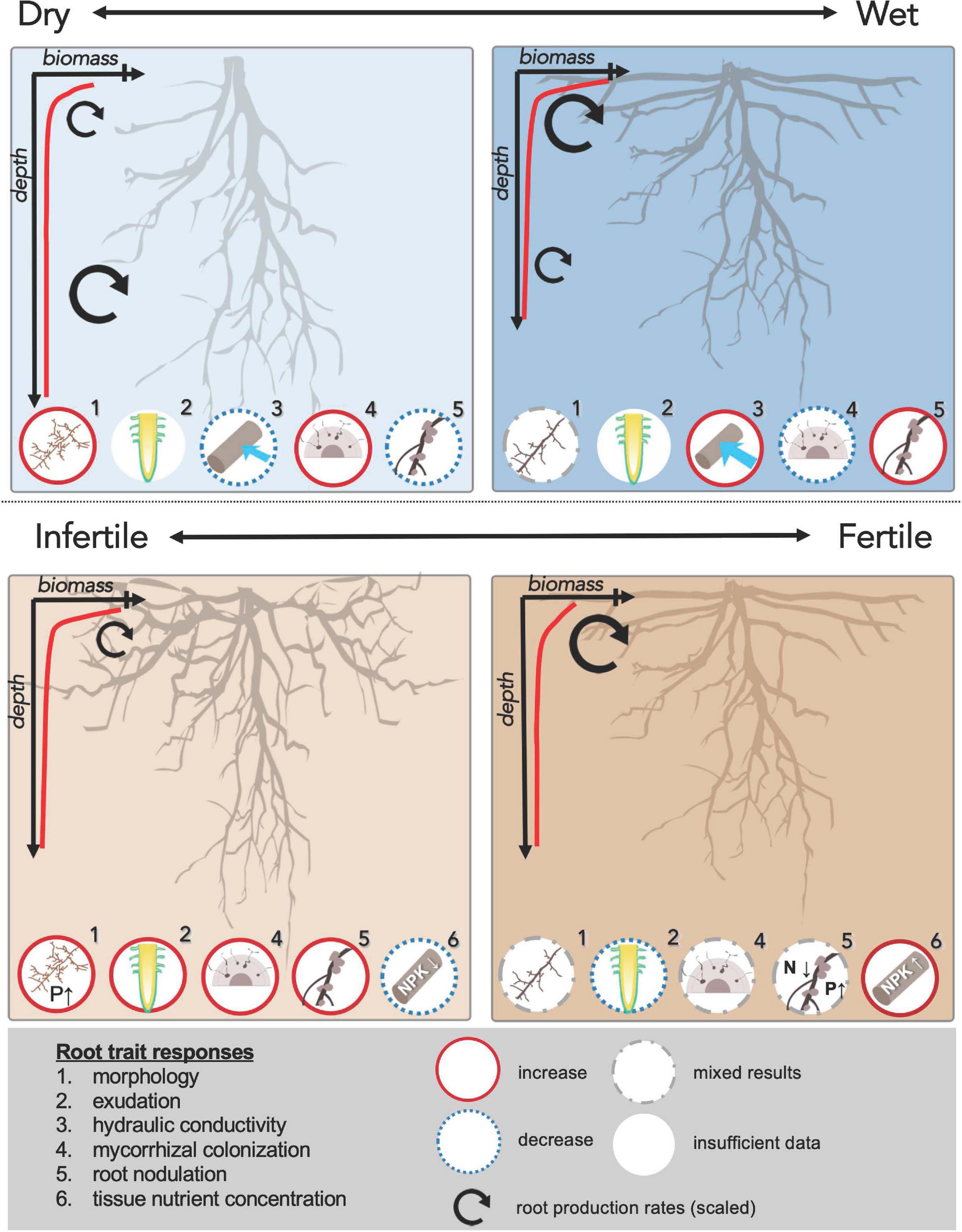
Figure 1. Fine root trait responses to variation in moisture and nutrient availability are shown for tropical forests, following empirical studies in the text. Differences in overall root architecture are shown in each panel, with representative fine root biomass depth distribution curves. Relative differences in fine root production rates are shown as different sized curved arrows for surface soils and subsoils. For example, on the dry-to-wet gradient, wetter sites have shallower root architecture with more surface root biomass, and faster root turnover at the surface but slower root turnover at depth relative to drier sites. Insets show responses of 6 additional fine root traits to the resource conditions, including: (1) root morphology – specific root length per mass (SRL) and/or average diameter; (2) root organic acid and phosphatase enzyme exudates; (3) hydraulic conductance; (4) mycorrhizal colonization rates; (5) root nodulation for N fixing symbionts; (6) fine root tissue content of N, P and K. In the lower panels, circles with N or P and arrows show how increased availability of that nutrient increases or decreases that fine root trait (e.g. increased P increases root SRL in the lower left panel). Missing numbers in some panels are from traits with no relevant data for the gradient shown (e.g., #3 - hydraulic traits - are not shown on the soil fertility gradient). Colored lines around the inset circles indicate: solid line = increase, small dashed line = decrease, mid-dashed line = mixed effects across studies, no line = no data.
Methods
Online search engines, including Web of Science and Scopus, were initially explored in October 2020 using a combination of 118 keywords, yielding 390 results, which were then screened by the authors for relevance (Appendix 1a for initial keywords, Appendix 2a for initial results and screening). These initial results formed the basis of the review. Subsequent literature searches were completed in May 2021, in which we repeated the initial search and then optimized the initial keyword searches by text-mining and keyword co-occurrence networks using the R package “LitSearchR” resulting in a final search that used 118 alternative keywords (Appendix 1b; Grames et al., 2019). These final keywords were generated based on titles, abstracts, and keywords from papers identified in the initial search. These final keywords were adjusted for Boolean operator strings of search engines, structured to include terms related to root traits and nutrient and water cycling in tropical forest ecosystems and exclude agricultural terms. The revised searches in English, Spanish and Portuguese using Web of Science and Scopus found 2,500 potentially relevant articles (Appendix 2b). This set of articles was further screened by requiring that the title or abstract contained the root trait terms emphasized in this study (e.g., see headings of root trait sections below), and that soil nutrients/fertility or water/moisture was also included, and that the article focused on tropical forests. This further screening resulted in 70 potentially relevant articles not already included in the review to that point (Appendix 2c). These additional articles were then assessed by the authors for inclusion in the final manuscript. Nearly all empirical field and modeling studies report results for bulk, community-scale root traits, unless noted in the text that results are at the species-level.
Background: Resource Variation Across Tropical Forests
Variation in Water Availability Across Tropical Forests
Ecosystem moisture conditions vary considerably among tropical forests, setting different baselines for plant adaptations to water scarcity and acquisition strategies (Worden et al., 2021). Tropical forests are often differentiated by spatial variation in mean annual precipitation (MAP) and temporal variation in rainfall seasonality. Moist and wet forests span a range of 2,000 to >8,000 mm/year MAP (Holdridge et al., 1971), with many of these forests having an extended dry season of up to 3 months (FAO, 2012). By contrast, dry forests are distinguished by lower MAP of 500–2,000 mm/y, and one or more longer dry seasons (Murphy and Lugo, 1986). Mangrove forests are dispersed across tropical and subtropical coastlines, encompassing a broad range of MAP and seasonality, and are best delimited by the 20°C winter isotherm of sea-surface temperature (Tomlinson, 1986; Duke et al., 1998; Simard et al., 2019). Evapotranspiration in the tropics is often incorrectly considered a constant, but in fact varies widely and is particularly sensitive to net radiation and cloud cover (Fisher et al., 2009). Thus, there is wide variation of climate seasonality and ecosystem water fluxes within forests of any given MAP (Schwartz et al., 2020).
During dry seasons in tropical forests, surface soil moisture can decline dramatically, although the magnitude of the decline varies substantially depending on background rainfall and soil properties (e.g., clay content), as seen across 15 seasonal forests in Panama along rainfall and soil gradients (Cusack et al., 2019). While plants within communities have adapted to seasonal drying, severe episodic drought is defined as outside the normal range of conditions, and has been identified as a period when the relative extractable soil water is less than the 10% quantile of all average dry season months (Janssen et al., 2020). These droughts can manifest as an extension of the dry season, and/or as chronic reductions in rainfall throughout all or part of the wet season (e.g., due to ENSO events) (Ropelewski and Halpert, 1987), and can reduce deep soil water and lower water tables (Broedel et al., 2017). At the other end of the soil moisture spectrum, some tropical forests in valleys are characterized by seasonal flooding and prolonged anaerobic conditions (Esteban et al., 2021). Most data from tropical forests are from upland sites, but we include available studies from mangroves and flooded forests for completeness.
Variation in Nutrient Availability Across Tropical Forests
More than 50% of tropical forests occur on the two most strongly weathered soil orders in USDA soil taxonomy: Ultisols and Oxisols (Holzman, 2008). In these soils, available P and base cations tend to be scarce due to long-term weathering, leaching, and erosion losses of primary minerals (Walker and Syers, 1976; Crews et al., 1995). Thus, P and base cations tend to be cycled quickly and conservatively, with availability of these nutrients driven by decomposition of organic matter (Vitousek and Sanford, 1986). The remaining tropical forests grow on a wide range of soil types that are less weathered and more fertile in P and bases (e.g., Alfisols, Mollisols), but where N is typically more scarce (Sayer and Banin, 2016; Fujii et al., 2018). Variation among soil types and soil orders in tropical forests can occur at landscape- and regional-scales, such as within the lowland forests of Panama and the Amazon basin, both of which include a broad range of soil orders and corresponding nutrient availabilities (Turner and Engelbrecht, 2011; Cusack et al., 2018; Quesada et al., 2020). Thus, tropical forests across geologically diverse landscapes can encompass a broad range of soil orders and nutrient conditions, as observed in >250-fold variation in soil available P and base cations across lowland Panamanian forests (Turner and Engelbrecht, 2011; Cusack et al., 2018), and the Amazon Basin (Quesada et al., 2020). Vertical distributions of nutrients through the soil profile can also vary, with sharp P and base cation depletion at depth in strongly weathered soils, and more even depth distributions of nutrients in fertile soils (Cusack and Turner, 2020). Among tropical mangrove soils, which are typically marine alluvium, there is substantial variation in texture, nutrient content, and organic matter (Hossain and Nuruddin, 2016). This variation in nutrient availability gives rise to large-scale variation in which nutrients limit or co-limit tropical forest NPP and belowground C allocation among sites, as observed across natural soil gradients in the Amazon Basin, Borneo, Costa Rica, French Guiana, Puerto Rico, Panama, and Peru (Medina and Cuevas, 1989; Beinroth et al., 1996; Higgins et al., 2011; Quesada et al., 2012; Russo et al., 2012, 2013; Fisher et al., 2013, 2020; Hofhansl et al., 2020; Soong et al., 2020), and in nutrient fertilization studies across 48 tropical forest sites (Wright, 2019).
There is also temporal variation in nutrient availability in tropical forests, commonly resulting from patterns in litterfall biomass related to rainfall seasonality. With greater litterfall biomass during dry seasons, nutrients tend to accumulate at the soil surface, and then decline rapidly with the onset of the rainy season as plant and microbial uptake and leaching accelerate (Herrera et al., 1978; Yavitt and Wright, 1996; James et al., 2003; Kunert et al., 2010; Cusack et al., 2019). Tropical mangroves and forests on floodplains also have temporal variation in nutrient availability regulated by inputs from flooding (Koschorreck and Darwich, 2003; Chacón et al., 2008) and upstream nutrient discharges (e.g., from upstream farmland and sewage) (Sanders et al., 2014; Reis et al., 2017; Alongi, 2018).
Spatial and temporal trends in N availability in tropical forests are shifting with rapidly increasing atmospheric N deposition from human activity (Schwede et al., 2018). The external inputs of biologically available N to tropical forests have the capacity to change nutrient availability, decomposer activity, and root biomass (Matson et al., 1999; Cusack et al., 2011, 2015; Hietz et al., 2011; Liu et al., 2011). Together, variation in MAP, rainfall seasonality, and nutrient availability set different resource baselines upon which climate change and other global change factors like N deposition are occurring.
Fine Root Traits Linked to Nutrient and Water Acquisition in Tropical Forests
Next, we review patterns of tropical forest fine root trait variation across nutrient and moisture availability gradients, which reflect long-term adaptations of plant communities. We also discuss tropical root trait responses to drying and fertilization experiments, which more likely reflect plasticity (i.e., ability to respond to changing conditions) within plant communities. Overall, tropical root traits tend to vary with changing moisture and soil fertility (Table 1 and Figure 1).
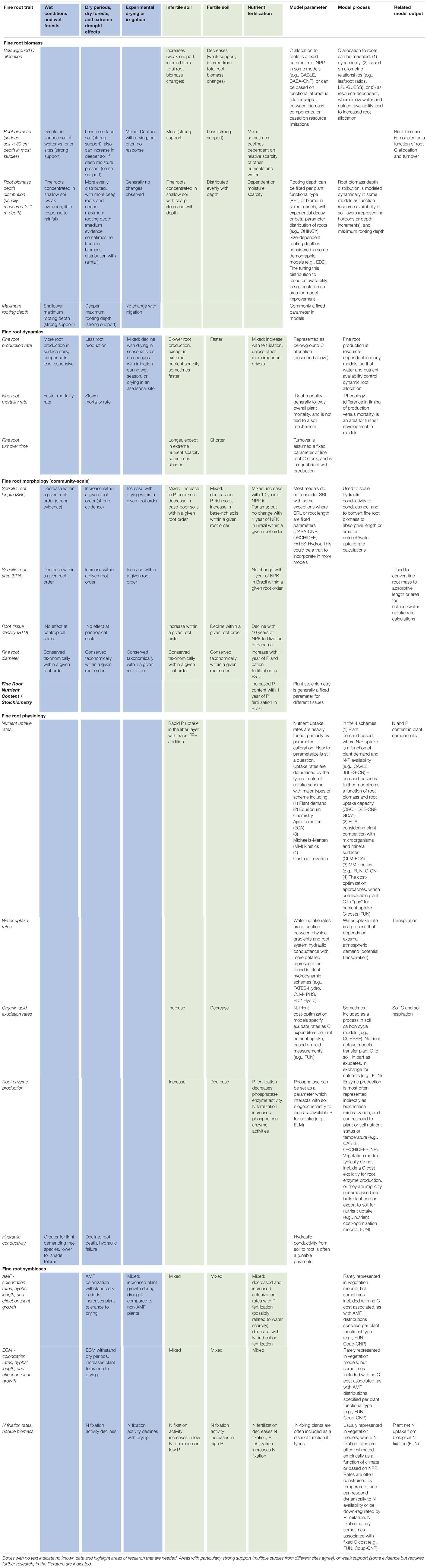
Table 1. Summary of how the different root traits respond to resource variation (blue = water, green = nutrients) based on empirical studies in tropical forests (cited in the text), and summary of the roles of different root traits in vegetation and nutrient uptake models.
Root Biomass Variation and Depth Distributions
Root Biomass: Nutrient Responses
Fine root biomass at the community scale has been by far the most readily available root data across tropical forest root studies (Vogt et al., 1996), particularly for surface soils (e.g., 0–10 cm), as evidenced in the global Fine-Root Ecology Database (FRED) (Iversen et al., 2017, 2021). Thus, patterns of fine root biomass with changes in resource availability are some of the most robust data for drawing insights into tropical forest water and nutrient acquisition strategies, particularly in deeper soils where other root trait data are very scarce. In general, greater P and base cation availability along soil fertility gradients correlate negatively with fine root biomass stocks in surface soils (∼0–30 cm), with this pattern occurring in dry, wet, and mangrove tropical forests (Gower, 1987; Maycock and Congdon, 2000; Ostertag, 2001; Espeleta and Clark, 2007; Castaneda-Moya et al., 2011; Powers and Perez-Aviles, 2013; Cormier et al., 2015; Simard et al., 2019; Huasco et al., 2021). This widespread pattern suggests reduced community-scale belowground biomass allocation when P or base cations are plentiful in tropical forests (Bloom et al., 1985), and can also result from faster turnover rates of fine roots in more fertile soils (Nadelhoffer et al., 1985; Aragao et al., 2009). This inverse relationship also holds for deeper fine-root biomass stocks at 1–2 m depths, as seen for fine roots versus P and base cations across lowland tropical forest soil fertility gradients in Queensland, Costa Rica, and Panama (Cavelier, 1992; Maycock and Congdon, 2000; Espeleta and Clark, 2007; Cusack et al., 2018). There are exceptions to this pattern, particularly in sites where other environmental drivers are stronger drivers of root biomass, such as salinity or hydroperiod in some mangrove forests (Adame et al., 2014, 2017). Other soil factors like texture have also been related to fine root stock variation across tropical forest gradients (Huasco et al., 2021). Still, the inverse relationship is prevalent enough across tropical forests to suggest that soil P and base cations are a dominant driver of fine root biomass stocks.
Fertilization experiments in tropical forests provide more mixed support for the inverse relationship between nutrient availability and C allocation to fine roots, possibly because not all fertilization experiments successfully alleviate nutrient limitation. A full factorial nutrient fertilization experiment with N, P, potassium (K) and micronutrients in a lowland Panamanian forest found that K additions significantly suppressed root biomass stocks in surface soils, suggesting an alleviation of K limitation (Wurzburger and Wright, 2015). The results from this fertilization experiment in Panama were corroborated by biogeographic patterns of increased root biomass stocks with declining soil K across natural soil gradients in the same region (Cusack et al., 2018). In a factorial N and P fertilization experiment in old-growth and secondary forests in Borneo, fine-root biomass also decreased in response to P fertilization in both old-growth and secondary forests (Yokoyama et al., 2017). However, there are also some mixed results. For example, some studies on infertile soils in montane Puerto Rican forests found positive effects of N or N + P additions on fine root biomass (Fetcher et al., 1996; Yaffar and Norby, 2020), while another montane Puerto Rican study found that N fertilization decreased surface fine root biomass stocks for two forest types (Cusack et al., 2011). Also, a meta-analysis of tropical forest fertilization experiments did not find an overall effect of fertilization with N, P and/or base cations on fine root biomass across 48 sites, likely reflecting site-scale variation in nutrient availability, and also possibly because other aspects of plant C allocation belowground were not considered (e.g., root turnover, organic exudates and root respiration) (Wright, 2019). Thus, assessment of fertilization effects on fine root biomass should consider baseline nutrient availability within a site, whether there is sufficient water availability for uptake of added nutrients, and the effects of fertilization on other root traits. Across these fertilization studies, it seems likely that experiments that added a limiting nutrient effectively reduced root biomass stocks, whereas fertilization experiments that added a non-limiting nutrient (relative to local conditions) did not alter root biomass stocks.
Root biomass depth distributions through the soil profile also often correspond to soil nutrient availability. In infertile soils, which have relatively larger root biomass stocks (see above), the majority of the fine root biomass is often concentrated in the surface soils (0–20 cm), whereas more fertile soils can have more even depth distributions of fine root biomass. This pattern was observed across 43 Panamanian forests where extractable K was the best predictor of root depth distributions (Cusack and Turner, 2020). Similarly, studies in rainforests of Borneo, Costa Rica, Jamaica, and Colombia have found that forest stands on acidic and infertile soils poor in P, K, and/or calcium (Ca) have relatively more fine root biomass concentrated in surface soils compared with more fertile and neutral pH soils (Berish, 1982; Gower, 1987; Espeleta and Clark, 2007; Jimenez et al., 2009; Brearley, 2013; Kochsiek et al., 2013). This concentration of root biomass in surface soils likely promotes rapid uptake of nutrients at the soil surface, as observed with 32P additions in an Amazon forest (Stark and Jordan, 1978). Still, a substantial proportion of root biomass can occur at depth even in infertile soils. More than 40% of fine-root biomass and productivity occurred deeper than 30 cm in a P-deficient site in the Brazilian Amazon (Cordeiro et al., 2020), which could be related to water acquisition in these nutrient-poor subsoils.
Root Biomass: Water Responses
In contrast to nutrient availability, soil moisture has been positively correlated with fine root biomass stocks in humid tropical forests. For example, fine root biomass stocks declined during dry periods in Brazilian forests (Metcalfe et al., 2008), and surface root biomass decreased during the dry season in a Brazilian eucalyptus plantation (Jourdan et al., 2008), and during the dry season in a Panamanian forest (Cavelier et al., 1999). In a 7-year Costa Rican study, root growth increased with monthly and annual increases in soil moisture, while the magnitude of the response varied across soil fertility gradients (Espeleta and Clark, 2007). Similarly, surface root biomass was positively correlated with the soil water content of the preceding 30 days in Malaysian forests with little rainfall seasonality (Green et al., 2005). Thus, there is relatively strong evidence that surface root biomass tends to decline during dry periods in tropical forests, which could be accompanied by allocation of root biomass deeper in the soil profile with greater moisture availability.
In contrast to temporal seasonality, there is less indication of variation in root biomass stocks across rainfall gradients. Across a gradient representing a near doubling of rainfall in seasonal lowland forests in Panama from 1,750 to 3,300 mm MAP, there was no relationship between MAP and root biomass stocks to 1 m depth (Cusack et al., 2018), or root depth distributions (Cusack and Turner, 2020), both of which were instead related to soil nutrients (see above). However, in three sites along a drier rainfall gradient in Ghana from 1,200 to 2,050 mm MAP there was a significant increase in root biomass stocks to 30 cm depth with increased rainfall (Ibrahim et al., 2020), which the authors suggest could be related to more acidic soils, larger trees, and reduced fire occurrence in the wetter forest.
Tropical forest rainfall manipulations have shown that fine root biomass sometimes responds to relatively extreme changes in moisture. A 60–80% experimental reduction in rainfall in Indonesia led to a decline in live fine root biomass to 120 cm depth, with the largest decline from 20 to 40 cm depth, and ∼3-fold increase in dead root biomass to 40 cm depth (Moser et al., 2014). In contrast, fine root biomass to 20 cm depth did not change with a 50% reduction in rainfall in southern China, but fine root biomass increased by 20% when rainfall frequency increased (Deng et al., 2018). In a lowland Panamanian forest, 5 years of irrigation during the dry season did not change fine root biomass to 30 cm depth (Yavitt and Wright, 2001). Thus, moisture manipulations in tropical forests sometimes lead to root biomass responses that mimic natural seasonal patterns, but not in all cases. At sites where irrigation did not lead to increased root biomass, root phenology might respond to other climatic cues besides (or in addition to) increased moisture, such as day length. Or, a different resource, like soil nutrients, might limit root biomass responses to increased moisture. In sites where drying did not lead to declines in root biomass, plant communities might have strong root drought tolerance, or root biomass might not change if roots are dormant but do not turn over during dry periods.
Root biomass depth distributions are important in relation to water acquisition during dry seasons and episodic droughts, with deeper roots likely being able to access deep soil water when surface soils are dry. According to a seminal study on global root distributions (Jackson et al., 1996), ∼ 70% of root biomass in tropical deciduous and evergreen forests occurs in the top 30 cm of soil, but this varies according to moisture regime. For example, a dry forest of Puerto Rico had relatively more deep root biomass compared with wetter Puerto Rican forests (Yaffar and Norby, 2020), although rainfall was not a predictor of root biomass depth distributions over a doubling of rainfall in Panama forests, and instead nutrients predicted depth distributions (Cusack and Turner, 2020). Even though a relatively small proportion of root biomass is typically distributed below 30 cm, even in drier tropical forests, deep roots can be disproportionately important for accessing deep soil water during dry periods in tropical forests (Wagner et al., 2011).
Maximum rooting depth is therefore an important aspect of root depth distributions when considering water acquisition. For example, fine roots in highly seasonal evergreen forests in the eastern Amazon reach depths of up to 18 m, and during the dry season, these trees commonly rely on water obtained from soil depths greater than 8 m (Nepstad et al., 1994). Similarly, a study of 62 tree seedling species in the Amazon found deeper root distributions in drier versus wetter forests (Markesteijn and Poorter, 2009). Isotopic δ18O data from xylem water on Barro Colorado Island (BCI) in Panama indicated that a subset of canopy trees accessed groundwater during the dry season (Meinzer et al., 1999; Andrade et al., 2005). In addition to supplying water directly to plant xylem, deep roots can also redistribute water to recharge surface soils during the dry season in a process termed “hydraulic lift,” which has been observed in seasonal Amazonian forests (Oliveira et al., 2005; Markewitz et al., 2010). A synthesis for tropical ecosystems showed that evergreen trees tend to be more deep-rooted in drier forests, but this relationship was not observed for tropical deciduous trees (Oliveira et al., 2021). This same synthesis and another global synthesis found that water table depth is a key factor determining maximum rooting depth within tropical ecosystems (Fan et al., 2017; Oliveira et al., 2021).
Moisture manipulations have generally had no effect on tropical forest root depth distributions and maximum rooting depths. In common garden irrigation experiments in seasonal Panamanian forests, watering for 4 years did not affect the maximum rooting depth of lianas or trees (Smith-Martin et al., 2019), nor did another dry season irrigation experiment with seedlings in Panama (Yavitt and Wright, 2001). Deep roots are important for withstanding drought and experimental drying, however. For example, a 30% throughfall reduction for 3 years in a strongly seasonal Amazon forest in Tapajos (6-month dry season), did not alter evapotranspiration rates, which a hydraulic uptake model attributed to increased water acquisition from 750 to 1,150 cm depths (Markewitz et al., 2010). Then, in years four and five of this experiment, water was no longer accessed from the deep soil, indicating water depletion that coincided with tree mortality in the drought treatment (Nepstad et al., 2007). Thus, while root depth distributions and maximum rooting depth may not be very plastic root traits, these are important characteristics to withstand drought in tropical forests.
While deep-rooted trees are associated with greater water uptake during dry periods in tropical forests, this is not the only plant strategy for surviving drought. Deep-rooted plants have access to relatively stable deep soil water sources and maintain higher transpiration rates during dry periods, but they also tend to be less resistant to embolism, and have been termed “drought avoiders.” This type of drought avoidance can support evergreen trees through dry periods, as observed in seasonal Amazonian forests (Nepstad et al., 1994; Jackson et al., 1995; Davidson et al., 2011). This is in contrast to “drought tolerant” species that have been observed across moisture gradients in the Amazon, which maintain shallow root depth distributions during dry periods, have reduced water uptake, and can also maintain canopy foliage during dry periods (Barros et al., 2019). Forests with long dry seasons in the Amazon have been shown to include a mixture of species with these different strategies (Brum et al., 2019). In tropical dry forests, drought deciduousness, another type of drought avoidance, tends to be the dominant ecological strategy (Oliveira et al., 2021).
Although the majority of our information about tropical roots comes from biomass data, there are some limitations to consider. First, many studies, especially on mature trees, measure changes in absolute fine root biomass rather than root mass fraction (i.e., fine root dry mass per total plant dry mass) along resource gradients. To detect increased plant biomass allocation belowground to roots, studies that measure changes in root biomass should account for aboveground changes in plant biomass, although this is difficult for community-scale studies. Also, root biomass production is only one component of plant belowground C allocation, with root respiration and root organic acid exudates potentially comprising a large proportion of plant belowground C flux (Gill and Jackson, 2000; Phillips et al., 2008). Finally, the relationship between resource acquisition and root biomass is indirect, since root biomass data does not directly indicate water or nutrient uptake activity. These problems create a disconnect between field data and vegetation model approaches, which typically parameterize and represent C allocation belowground dynamically, based on allometric relationships (e.g., leaf:root ratios), or dependent on resources (De Kauwe et al., 2014; Trugman et al., 2019). Plant C allocation belowground is also the main determinant of root biomass and root production in many vegetation models. Thus, field studies should accompany fine root biomass measurements with whole-plant measures when possible, and with more functional root traits, as described below.
Fine Root Dynamics: Production, Turnover Rates, and Phenology
The timespan from fine root production to mortality is termed the turnover rate, and the timing of root flushing to mortality is termed phenology. Globally, fine root production tends to increase with MAT and MAP, and fine root turnover is greatest in tropical forests compared with temperate and boreal forests (Finer et al., 2011). Below, we consider fine root production and turnover rates from different tropical forest studies, and where possible, also the timing of root flushing and mortality relative to shifts in resource availability and seasonality.
Production and Turnover: Nutrient Responses
Many tropical soils are characterized as nutrient-poor (typically poor in P and base cations), and these soils tend to have larger stocks of fine root biomass, slower fine root production rates, and longer root turnover times compared to more fertile tropical soils. For example, faster fine root production rates have been observed in Hawaiian sites with greater P availability across soil gradients (Ostertag, 2001), and also in higher fertility sites across Central Amazonian soil gradients (Aragao et al., 2009). There was a positive relationship of fine root productivity and turnover rate with greater total soil N and K levels in a wet Colombian forest, as well as greater productivity in more fertile surface soils versus less fertile deeper soils (Quinto et al., 2016). Greater root production rates were also observed in more fertile landward mangroves relative to infertile seaward sites in China (Zhang et al., 2021). However, a contrasting pattern has been observed with extreme nutrient scarcity, with faster root turnover rates in extremely P-scarce ecosystems, possibly because P resorption from roots is efficient (relative to N resorption), so turnover allowed for expanded soil exploration by roots with minimal P losses (Laliberte et al., 2015). Similarly, an extremely infertile sandy soil in the Colombian Amazon had greater root productivity rates in surface soils compared with a more fertile loamy soil (Jimenez et al., 2009). Thus, natural soil fertility gradients indicate that a more conservative strategy of slower production and longer turnover times are typical on infertile sites, although extremely infertile sites can have increased productivity and mortality rates to facilitate exploration of greater soil volumes.
Plot-scale nutrient addition experiments had mixed effects on root productivity. Fine root production rates increased after 10 years of K fertilization in a Panamanian forest (Yavitt et al., 2011), and with N fertilization in Brazilian plantations (Jourdan et al., 2008). Similarly, P addition increased fine root productivity by 23% after 1 year in the Central Amazon (Lugli et al., 2021). In tropical mangroves, P addition also significantly increased fine and coarse root production in sites with infrequent tidal inundation and high salinity (McKee et al., 2007; Adame et al., 2014). In contrast, P addition had no effect on root production in a mangrove frequently inundated with water with low salinity (Adame et al., 2017). Thus, fertilization experiments can yield similar results as observed across natural soil fertility gradients, depending on other modulating environmental factors.
Production and Turnover: Water Responses
Root dynamics in tropical forests tend to respond strongly to seasonal moisture cycles, with more root production in surface soils being typical during the wet season (Luizao et al., 2007; Rodtassana and Tanner, 2018; Singha et al., 2020). For example, fine root production was greatest during wet periods in an Amazonian rainforest (0–90 cm depth), and this relationship was strongest in surface soils. Fine root mortality was also greater during rainy periods for the top 0–30 cm of soil, while mortality in deeper layers decreased slightly with increased precipitation (Cordeiro et al., 2020). Similarly, there was less production of surface roots (0–15 cm) during the dry season in a Panamanian forest, followed by root flushing upon re-wetting (Yavitt and Wright, 2001). In dry forests in Thailand, mature rubber trees had the greatest root growth 3 months into the rainy season in the top 2 m of the soil, while root growth below this depth did not respond to increased rainfall (Maeght et al., 2015). Even in wetter tropical forests, moisture commonly limits plant growth and dry periods can quickly suppress root production and activity (Clark et al., 2010; Vasconcelos et al., 2012). Thus, tropical forest tree species seem well-adapted to natural moisture shifts, with increased production and mortality during wetter periods, particularly in surface soils.
Experimental manipulation of soil moisture via precipitation diversion has had more mixed effects on root dynamics. For example, increased moisture inputs via dry season irrigation did not affect root growth in a Panamanian forest (Yavitt and Wright, 2001). Fine root production to 20 cm depth also did not change in response to 2 years of experimental drought in Indonesia (Moser et al., 2014). In contrast, fine root mass, length, and production at 0–30 cm soil depth decreased with 2–4 years of experimental drought in a sandy Oxisol in the Brazilian Amazon (Metcalfe et al., 2008). These contrasting results could be related to different baseline conditions in Indonesian versus Brazilian sites, which had 1,894 mm MAP versus 2,500 mm MAP, no dry season versus a 6-month dry season, and pre-montane versus lowland forests, respectively. In particular, the Brazilian forest is adapted to a long natural dry season, which may have increased the responsiveness of roots to experimental drought. Understanding the plasticity of root systems at different sites in response to drought, either via avoidance (e.g., shifting root production deeper for water uptake), or via tolerance (e.g., dormancy through dry periods), will be crucial for predicting spatial variation in forest responses to climatic drying.
Overall, root phenology appears to respond to the spatial and temporal variations in nutrient availability and seasonality in a particular site, whereas experimental manipulations have had only mixed success at changing fine root production and turnover rates. Thus, environmental cues in addition to moisture likely influence the timing of root production and turnover in many forests. The degree of plasticity in root phenology, both at species and community scales, will be a crucial trait for tropical forests to withstand more intense drought periods. From the limited data, community-scale plasticity in root phenology seems to be far more restricted than might be assumed from patterns across gradients and seasons.
Morphological Root Traits
Fine root morphological traits have been linked to nutrient and water acquisition. For example, plants can explore a larger volume of soil for a given biomass investment with roots that have greater SRL (length per mass), greater specific root area (SRA, area per mass), reduced root tissue density (RTD, mass per volume), greater root branching (e.g., how many first order roots per second order root), and increased root hair density (Eissenstat and Yanai, 1997; Hodge, 2004; Metcalfe et al., 2008). These more acquisitive root traits often present together, as illustrated by some Panamanian tree species (Figure 2), and these traits may vary over soil depth. Root hairs can be especially important in plants with minimal mycorrhizal associations, exploring soil volumes that would not be explored by hairless roots (Bates and Lynch, 2001; Abrahao et al., 2019). Higher SRL, or longer and thinner fine roots, can increase overall absorptive capacity, but this morphology has also been associated with greater respiration costs, faster turnover times, decreased mycorrhizal association, and increased susceptibility to pathogen and herbivore attack (Maherali, 2014; Laliberte et al., 2015; McCormack et al., 2015; Weemstra et al., 2019). Root tissue density is associated with longer turnover times and a negative relationship to nutrient uptake capacity (Eissenstat, 1992; Comas and Eissenstat, 2004), such that low density roots are associated with greater resource acquisition. Cluster roots - clusters of closely spaced short lateral rootlets characteristic of plants in the Proteaceae growing in extremely nutrient-poor habitats - can respond to microsites of resource availability and often produce large amounts of organic acid exudates and phosphatase enzymes (Skene, 2001; Costa et al., 2016).
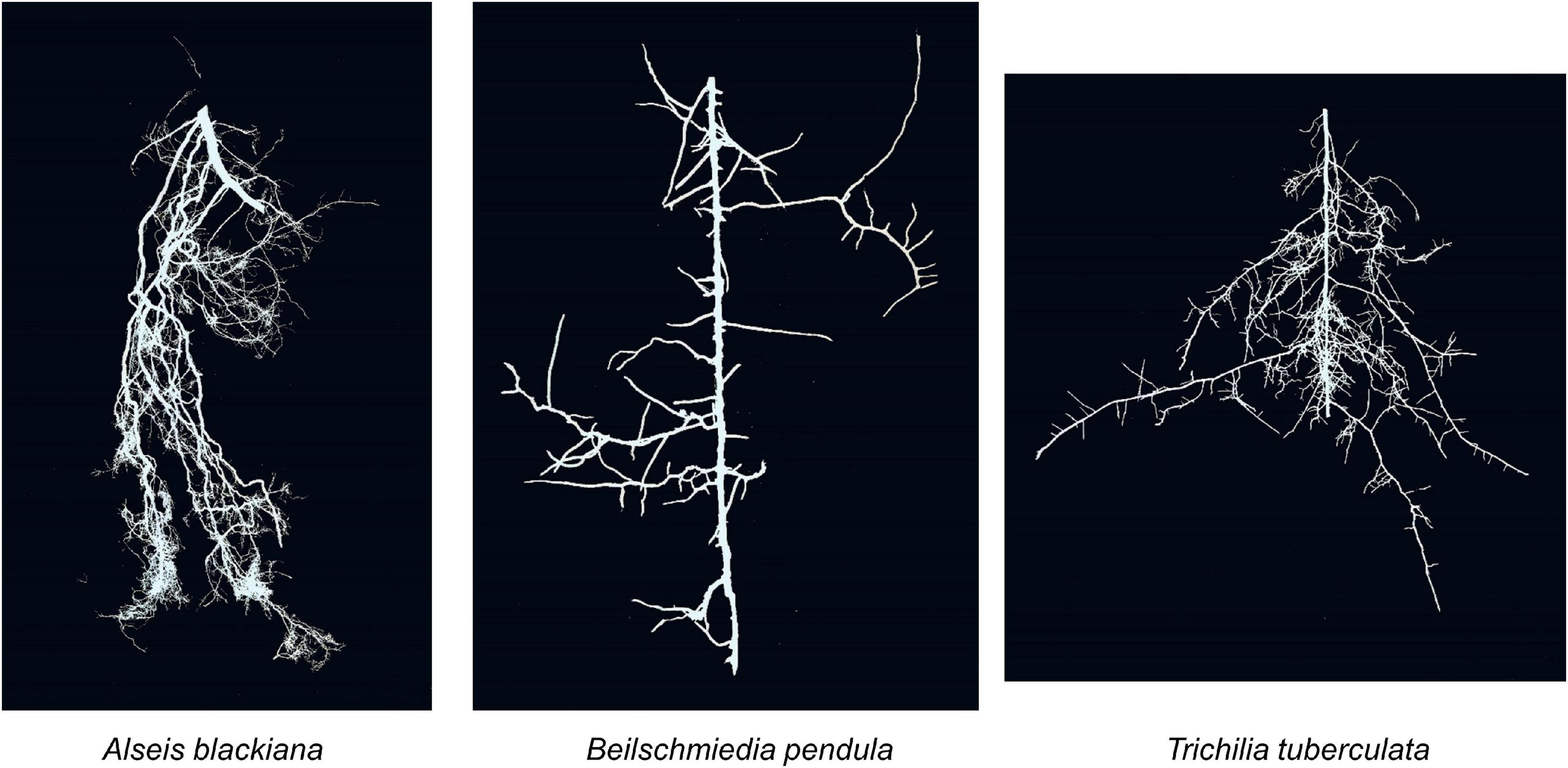
Figure 2. Three contrasting root depth distributions and morphologies are shown for tree species from lowland Panamanian forests. Alseis blackiana Hemsl. (left) provides an example of deep biomass depth distributions, roots with high SRL, and high branching ratios, all of which are associated with water acquisition. Beilschmiedia pendula (Sw.) Hensl. (center) provides an example of thicker diameter roots and lower root branching ratios, generally associated with a more conservative root strategy and longer turnover times. Trichilia tuberculata (Triana & Planch.) C. DC. (left) provides an example of a shallower root depth distribution and high SRL, which can be associated with more fertile soils in tropical sites. Photo credit: S. Bernal and SJ Wright.
While some fine root morphological traits are generally considered to be responsive to changes in resource availability, other morphological traits, like average root diameter per root order, can be taxonomically conserved (McCormack and Iversen, 2019). The distribution of fine root diameters for tropical forests globally is flatter and evenly distributed from 0.1 to 1 mm, compared to a greater abundance of very fine roots <0.5 mm in other biomes (Ma et al., 2018). Thicker diameter has been associated with longer root turnover times, may have improved resource transport within roots, and have been associated with greater arbuscular mycorrhizal fungi (AMF) colonization rates, as observed in a Brazilian forest (Lugli et al., 2020), suggesting that these roots are more dependent on symbiotic relationships for resource acquisition (Hodge, 2004; Liu et al., 2015; McCormack et al., 2015). There appear to be phylogenetic constraints on the extent to which root morphological traits can respond to changing resources, according studies in subtropical China and French Guiana (Kong et al., 2014; Sun et al., 2020; Valverde-Barrantes et al., 2020). Understanding the plasticity of morphological traits, within and among tropical species, is a crucial step for predicting how tropical forest communities will withstand climatic drying and changes in nutrient availability.
The relative tradeoffs represented by these different root morphologies have been described as a multi-dimensional spectrum of plant belowground strategies (Bergmann et al., 2020). In this conceptualization, one axis encompasses the classic tradeoff between fast growth, resource acquisition (indicated by root %N), and rapid turnover (i.e., “grow fast die young”) at one end of the spectrum, versus slow growth, resource conservation (indicated by dense roots), and longer lifespan at the other end (Reich, 2014). A second axis encompasses a spectrum of resource exploration strategies, with thicker diameter roots that have greater mycorrhizal colonization at one end of the spectrum, versus more long, thin roots with high SRL and less mycorrhizal colonization at the other end.
At the biome scale, tropical forests have, on average, root systems with thicker diameter roots, lower SRL, lower N content, and higher RTD than those reported for temperate, boreal or arid ecosystems (Freschet et al., 2017). Following the multidimensional root spectrum hypothesis (Bergmann et al., 2020), these trends suggest that there is an overall selection in tropical areas for long-living root systems that depend largely on mycorrhizal associations for nutrient acquisition. A recent study of variation in fine roots of tropical trees showed that species in dominant tropical families (Moraceae, Fabaceae, and Sapotaceae) have relatively thick diameter, unbranched root systems, which are usually associated with basal angiosperm groups (Valverde-Barrantes et al., 2020). Thicker diameter fine roots in this study were also associated with a set of root anatomical traits, including thick cortical tissue, high cortex:stele ratio, and low root tip abundance, which may also be indicative of increased root colonization by AMF for resource scavenging. This type of study linking anatomical traits with more commonly measured morphological traits are rare in the tropics, and are very useful for corroborating the function of fine root morphologies.
We assessed a subset of 240 tropical plant species (Appendix 3) included in a broader study of root trait syndromes (Valverde-Barrantes et al., 2021), to show that among tropical plants, the strongest fine root morphological tradeoff is between SRL and diameter, which create an axis along which woody and non-woody tropical species separate (Figure 3), with a second axis separating along RTD and root N content. These axes separating fine root morphological traits and nutrient content could be explained by different root architectural anatomies, and in particular differences in cortical versus vascular root tissues (Figure 3). Globally, tropical forest fine woody root N content tends to span a broader range, from ∼0.005 to 0.04% N, compared with other biomes where woody roots tend to have narrower ranges (e.g., ∼0.012 to 0.03% N in temperate forests) (Ma et al., 2018).
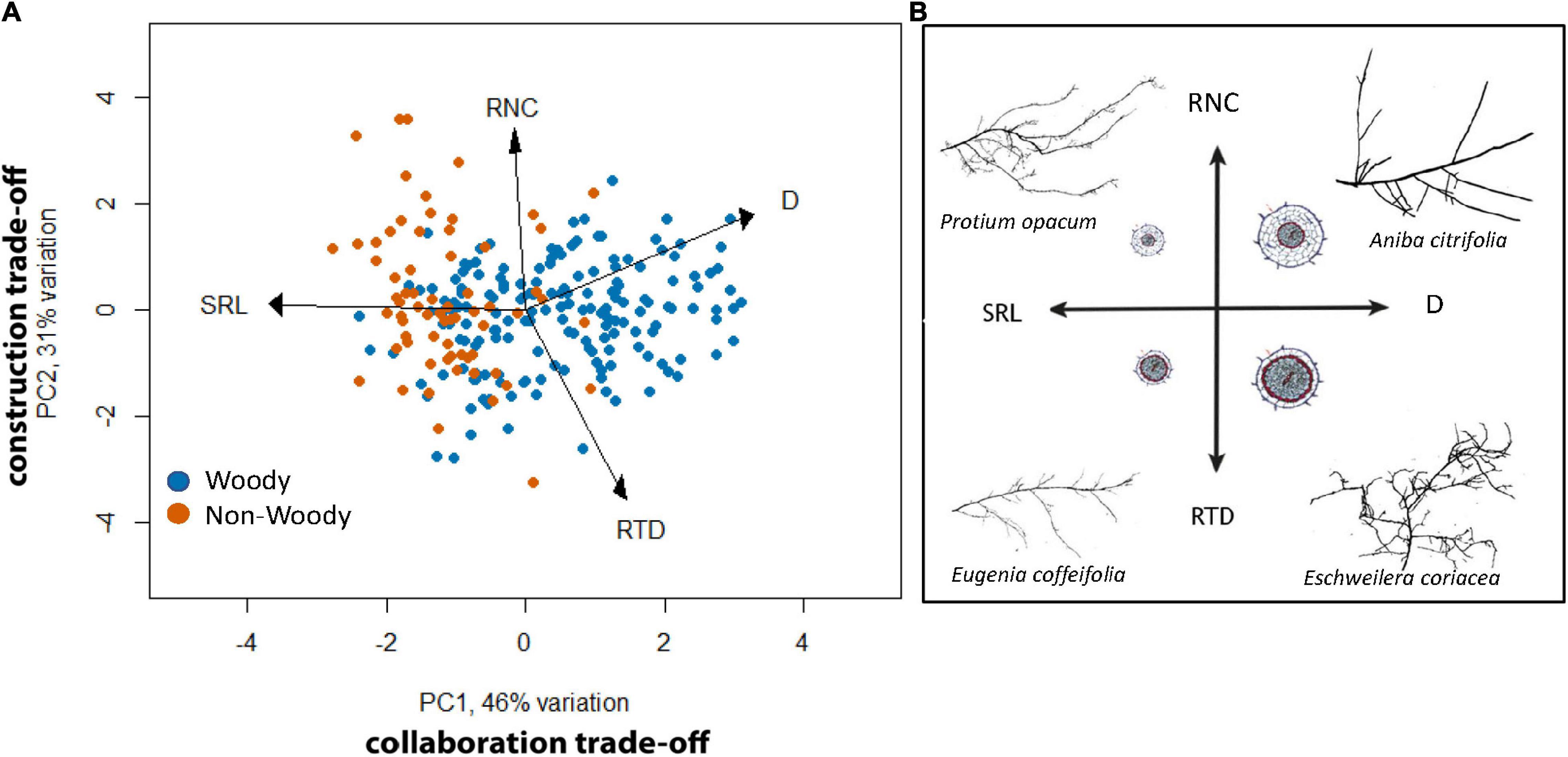
Figure 3. Tradeoffs among fine root morphological traits and root N are shown for 240 tropical woody (blue) and non-woody (orange) species are show, with a conceptual representation of the possible relationship between root morphological/chemical traits and root anatomy. Panel (A) presents a principal component analysis (PCA) ordination of four commonly measured root morphological traits and nutrient content (specific root length, SRL; mean root diameter, D; root N content, RNC; root tissue density, RTD) for 240 tropical plant species. Axis 1 (PC1) explained 46% of the variation in fine root traits, with the strongest loadings by SRL (−3.6) and D (3.2). Axis 2 (PC2) explained 31% of the remaining variation, with the strongest loadings by RTD (−1.3) and RNC (0.04). Panel (B) illustrates the possible variation in anatomical traits that explains the variation in morphological traits and N content. Species with larger cortical tissue (clear areas in the root cross section) likely have greater N content, whereas greater vascular stele (gray areas) likely increase tissue density. Fine root diameter and SRL should therefore be a function of the ratio between cortical and stele tissue. Root systems in panel (B) exemplify the natural variation in tropical species within the conceptual frame. Figure modified from Valverde-Barrantes et al. (2021).
Morphological Traits: Nutrient Responses
The relationships between fine root morphology, particularly SRL, and nutrient availability in different tropical forest studies are mixed. A pan-tropical review recently indicated that SRL was strongly positively related to base saturation, somewhat contradictory to the expectation that SRL increases when nutrients are scarce (Addo-Danso et al., 2020). This same study found that SRL was weakly negatively correlated with inorganic soil P across global tropical forest regions, similar to local-scale gradient studies in Borneo and Brazil where SRL also decreases in sites with greater inorganic P (Metcalfe et al., 2008; Zangaro et al., 2008; Kochsiek et al., 2013). Greater root branching, which can also increase root surface area, was observed in response to P scarcity in Puerto Rico (Yaffar et al., 2021). Thus, root SRL appears to respond differently to P versus base cation availability at landscape and larger biogeographic scales, and the functional link between SRL and the uptake of different nutrients requires more investigation.
Fertilization experiments provide some insight into how a community can respond to changes in in situ nutrient availability. In lowland seasonal forests in Panama, community-scale RTD declined with 10+ years of N, K, and NPK fertilization, and fine root SRL increased up to 50% with addition of K, NP and NPK (Wurzburger and Wright, 2015), indicating that long-term fertilization led to plants with longer, thinner, and less dense roots, typically associated with greater soil exploration, rapid nutrient uptake, and faster turnover. In contrast, 1 year of N, P, and base cation fertilization in the Central Amazon did not alter RTD, SRL, or SRA in newly produced roots from in-growth cores, and only fine root diameter increased with the addition of P and cations separately (Lugli et al., 2021), although it was unclear in this study whether the same species were being observed, or if there was a shift in which species were represented in the cores. Together, these data suggest that most morphological root traits can adapt to changes in nutrient availability, but the change can be gradual in response to fertilization (i.e., more than 1 year). Clearly, more data from tropical fertilization experiments over longer time periods are needed for a better assessment of the plasticity of morphological root traits.
Morphological Traits: Water Responses
Tree species and community-scale fine root morphological traits tend to assemble in predictable ways in relation to soil moisture (Holdaway et al., 2011). Plants adapted to dry conditions often have smaller diameter fine roots and greater SRL relative to wetter sites, increasing the volume of soil explored and decreasing the apoplastic barrier for water entering the xylem (Comas et al., 2013). However, data for root morphology across tropical rainfall gradients are lacking. An experimental drought in seasonal Amazonian forests increased SRA and SRL at the same time that overall root biomass decreased, indicating reduced biomass with longer, thinner roots in drier soils (Metcalfe et al., 2008). Thus, SRL can increase in response to greater moisture, and appears to be a relatively plastic trait in response to drought, although more research on the biogeographic patterns and plasticity of SRL with changes in moisture is needed.
Physiological Root Traits: Nutrient Uptake, Root Enzyme Activities, and Exudation Rates
In general, fine root physiological traits are less studied compared with morphological traits in tropical forests, constituting a key knowledge gap in tropical ecology. Physiological traits directly measure root function, whereas the biomass and morphological traits discussed above are generally surrogates for root physiological functions (Freschet et al., 2021). Fine root nutrient and water uptake rates are physiological traits, as are root exudation rates of nutrient-acquiring enzymes and organic acids, and hydraulic conductance.
There is very little data for nutrient uptake rates in tropical forests aside from uptake of N, which as discussed above is often relatively abundant in tropical soils. As might be expected given the relative abundance of N in tropical forests, the few published N uptake rates for tropical forests indicate much slower uptake compared with other biomes (e.g., 1.6 μgN/g-root/h in tropical forests, versus 17.7 in temperate forests μgN/g-root/h) (Ma et al., 2018). Nutrient uptake rates can be measured directly using a solution-depletion method in the field on in-tact roots (Lucash et al., 2007). Plant nutrient uptake rates can also be directly measured by tracing isotopically labeled nutrients and/or water, using the rare stable isotopes 15N, 2H, and 17O, and the much more logistically challenging radio-isotope 32P (Herrera et al., 1978; Templer et al., 2008).
Fine root exudation of organic acids and nutrient-acquiring enzymes, like phosphatases, increase nutrient availability in the rhizosphere. First, organic acid exudates solubilize inorganic nutrients in general, leading to greater availability (Costa et al., 2016). Second, root extracellular enzymes released into soils hydrolyze ester bonds in organic matter and release inorganic nutrients. Organic acid exudation has been measured primarily in temperate forests and tropical savannas on individual, intact roots immersed in water solutions (Phillips et al., 2008; Abrahao et al., 2014, 2019; Teodoro et al., 2019). Root extracellular enzyme activities are often measured on cut roots in the lab using standard hydrolytic enzyme substrates (Steidinger et al., 2015; Yokoyama et al., 2017), although many more studies have measured bulk soil enzyme activities as an integrated measure of root plus microbial enzyme activities.
For water acquisition, root hydraulic conductance has been used as a direct measure of water uptake capacity. Fine root hydraulic conductance is linked to a plants’ capacity to withstand drought (Comas et al., 2013), and can also aid in passive uptake of nutrients dissolved in soil water (McMurtrie and Nasholm, 2018). The hydraulic efficiency of water uptake can be measured as root hydraulic conductivity, a property of root tissue, or as root hydraulic conductance, a rate (Hunt et al., 1991; Schuldt et al., 2013). Root hydraulic conductivity is comprises radial and axial components, with radial conductivity indicating water flow from soil-to-root, and axial conductivity indicating water flow within root xylem. Root radial hydraulic conductivity is orders of magnitude lower than axial conductivity, and is often the rate limiting step for water acquisition. Radial hydraulic conductivity commonly varies greatly in magnitude both intra- and interspecifically for tropical trees (Tyree et al., 1998; Shimizu et al., 2005).
In general, these physiological traits are targeted at either nutrient or water acquisition, but overlaps and indirect effects among them deserve more investigation.
Physiological Traits: Nutrient Responses
Direct measures of nutrient uptake rates are very rare for tropical forests, and most have focused on plant nutrient preferences, rather than comparing nutrient uptake rates across soil fertility gradients. For example, trees in a montane Puerto Rican forest showed preference for ammonium (NH4+) over nitrate (NO3–) in a study using 15N isotopic tracers (Templer et al., 2008). A sapling study in a montane forest in Ecuador showed that four species preferred NO3– over NH4+, but the two species with the highest growth rates preferred NH4+ (Wittich et al., 2015). In Panama, 15N isotopic tracer additions showed no overall preference among palm and woody tree species for NO3–, NH4+, or glycine (Andersen and Turner, 2013; Andersen et al., 2017), and there was a similar lack of preference among N chemical forms for nine Bornean tree species (Russo et al., 2013). These studies indicate that plant species within the same site vary in N preference, or show no N preference. Addition of trace amounts of 32P in an infertile Amazon forest showed rapid and almost complete uptake of added P in the litter layer of soil (Herrera et al., 1978; Stark and Jordan, 1978), showing conservative cycling of P. An important next step in this line of research will be to compare uptake rates among N, P and base cations within and among tree species, and across sites with different soil fertility. This type of study would indicate the capacity for fine roots to adjust uptake rates in response to changing resources.
Rates of root organic acid exudation generally increase when soil nutrients are scarce (Costa et al., 2016). For example, root exudation rates in Malaysian forests were greater in P-poor soils and used a greater proportion of plant NPP, and exudation rates were lower in a P-rich soil (Aoki et al., 2012). In this study, organic acid exudation rates were positively correlated with fine root surface area, indicating a link between morphology and physiology. Similarly, 18 tree species in a subtropical forest in China had fine root organic exudation rates that were positively associated with other traits indicative of nutrient acquisition (e.g., root N concentration), and exudate rates were negatively associated with root traits indicative of more conservative fine root strategies (e.g., RTD) (Sun et al., 2020). Thus, fine root morphology and nutrient content, which are relatively easy to measure, might be good indicators of root exudation activity, which is much more difficult to quantify extensively.
Fine root phosphatase activities are generally inversely related to soil P availability in tropical forests. Across lowland Panamanian forests, fine root phosphatase activity rates were higher in P-scarce soils and lower in P-rich soils, but there was also high interspecific variation in fine root phosphatase activity in the P-scarce soils, suggesting a range of strategies to tolerate P scarcity (Guilbeault-Mayers et al., 2020). A study in Puerto Rico showed that fine root phosphatase activity was negatively associated with soil extractable inorganic P (Cabugao et al., 2021). Fine root phosphatase activity decreased with P fertilization in a Central Amazonian forest (Lugli et al., 2021), and in old-growth and secondary forests in SE Asia (Yokoyama et al., 2017). Increased phosphatase enzyme activity has also been associated with greater SRL (Cabugao et al., 2021) and fine root P content (Lugli et al., 2021), but the relationship with SRL was observed only at the community level and not at the species level in Puerto Rico (Yaffar et al., 2021). Nevertheless, these results suggest that occurrence of plants with longer, thinner roots (high SRL) can increase resource acquisition in nutrient-poor soils, in this case via enzyme production.
While direct studies of root phosphatase activities in tropical forests are still relatively scarce, a broader set of research using bulk soils indicates that N availability might also influence root phosphatase activities. A global meta-analysis found that P addition suppressed phosphatase activity, but N fertilization increased root phosphatase activity, possibly because of the high N requirement for enzyme production (Marklein and Houlton, 2012). The meta-analysis had very few tropical studies, but similar increases in soil phosphatase enzymes with N fertilization in an upper elevation Puerto Rican forest confirm the trend (Cusack et al., 2010). Also, fertilization in Hawaiian forests showed that P addition reduced root phosphatase enzyme activities in a forest on strongly-weathered, P-scarce soils, while N fertilization increased root phosphatase production (Treseder and Vitousek, 2001; Fujii et al., 2018). More studies of interacting nutrient effects on a broader suite of root enzyme activities are needed.
Physiological Traits: Water Responses
Dry soils with large soil water potential gradients can lead to embolisms in the root xylem vessels, decreasing hydraulic conductance from the soil into the plant. Within the rhizosphere, root dehydration can lead to mechanical failure of cortical cells followed by the shrinkage of roots, promoting a disconnection from soils (Carminati et al., 2009; Cuneo et al., 2016). The capacity for roots to dynamically adjust their water uptake surface area via length growth and mucilage excretions in response to desiccation can minimize the risk of embolism (Carminati and Vetterlein, 2013). Unlike the robust structural and physiological protection plants have against stem hydraulic failure, root mortality, and turnover are common during drought, which can be less costly to plants than investing C in root hydraulic infrastructure.
There are limited field measurements of hydraulic conductance responses to changing moisture availability in tropical regions. In Paleotropical forests, light-demanding, pioneer tree species had more dynamic change in root hydraulic conductance in response to soil water gradients compared with slower growing, late successional species (Shimizu et al., 2005). In Neotropical forests, roots of shade-tolerant species had lower overall hydraulic conductance and were less susceptible to mechanical or hydraulic damage with drying (Tyree et al., 1998). It is unclear whether greater plasticity in root hydraulic conductance of light-demanding species, or the lower but more robust hydraulic conductance of shade-tolerant species, would be more advantageous in drought conditions.
Symbiotic Traits
Mycorrhizal fungal associations are the primary symbiotic root trait related to both nutrient and water uptake. Arbuscular mycorrhizal fungi (AMF) are dominant globally, comprising >72% of associations with plants (Smith and Read, 2008; Brundrett and Tedersoo, 2018). Ectomycorrhizal (ECM) fungal associations are more common in temperate ecosystems (Tedersoo et al., 2010, 2012), but ECM fungi also associate with between 6% (Neotropics) and 19% (Paleotropics) of tropical tree species (Brundrett, 2009; Fukami et al., 2017). There is evidence that 283 plant species from 18 tropical plant families can form ECM associations (Corrales et al., 2018), with ECM fungi commonly dominating in localized areas, comprising up to 75% of tree species symbioses in some tropical forest communities (Lu and Hedin, 2019). Mycorrhizal fungal associations are most commonly measured as the intensity of intraradical colonization, which can be measured for AMF using root staining and microscopic assessment (McGonigle et al., 1990). However, other fungal traits have been proposed to better capture the functional nature of the symbiosis, including: hyphal length, fusions and lifespan, speed of root colonization, formation of vesicles and auxiliary cells, and spore production (van der Heijden and Scheublin, 2007). Molecular techniques to identify specific fungal groups are also increasing in use (McGuire et al., 2012), but the relationship between these molecular studies and nutrient or water uptake rates are limited.
Root nodulation is another symbiotic root trait for hosting N-fixing bacteria, predominantly in leguminous trees (Fabaceae). Generally, this symbiosis can supply substantial quantities of N to plants, and this plant functional type is abundant in many tropical forests (Menge et al., 2017). Plants that establish N-fixing symbioses can also have systematically higher root phosphatase activity than other species, although this differs across species (Nasto et al., 2014, 2017, 2019; Batterman et al., 2018; Soper et al., 2019), and it is unclear whether it results from the high requirement for N in phosphatase production (Houlton et al., 2008) or the alleviation of N limitation by fixation (Batterman et al., 2013a, 2018). Some field and experimental evidence suggests that N fixation can also be associated with improved water use efficiency and tolerance of drought (Adams et al., 2016; McCulloch et al., 2021). Root traits associated with N fixation include nodulation rates, with uptake rates measured using isotopically labeled 15N2 or acetylene reduction (Barron et al., 2009; Batterman et al., 2018). One common method to infer the contribution of N fixation to nutrient uptake is by measuring fine root N content. Many plants associate with both mycorrhizal fungi and symbiotic N-fixing bacteria simultaneously (Brearley et al., 2016), and the direct interactions between these two resource-acquiring symbionts require further study in the tropics.
Symbiotic Traits: Nutrient Responses
The major benefit of the association between plants and AMF is the translocation of inorganic P to the host plant, particularly in infertile soils (Smith and Read, 2008). Root-associated AMF also acquire and translocate other micro- and macronutrients, as observed for some Brazilian trees (Siqueira et al., 1998; Zangaro et al., 2003). Tissue N:P stoichiometry of AMF hyphae has been used in other biomes to assess P versus N uptake by this symbiont, and to indicate fungal growth strategies, such as acquisitive, facilitative, and growth-investing fungi, versus conservative, competitive, and C immobilizing fungi (Powell and Rillig, 2018), and fungal mycelia have more flexible C:N:P stoichiometry than most plant tissues (Camenzind et al., 2021).
The dominant paradigm is that plants invest in mycorrhizal symbioses when nutrients are scarce, and reduce these associations when nutrients are plentiful, which has been observed across many nutrient addition studies globally (Wallenda and Kottke, 1998; Treseder, 2004). Accordingly, P fertilization consistently reduced mycorrhizal colonization rates in Hawaiian forests with P-poor soils (Treseder and Vitousek, 2001). Addition of P and N+P in the Peruvian Amazon decreased fungal hyphal respiration rates, suggesting reduced AMF activity (Fisher et al., 2013). Similarly, a negative relationship between AMF colonization and P availability has been observed in Brazilian savannas (Oliveira et al., 2015). However, an inverse relationship between soil fertility and AMF colonization is not consistently observed across tropical forests. A biogeographic study across Brazil, Costa Rica, Panama, and Peru found that AMF hyphal length was not related to soil nutrient concentrations (Powers et al., 2005). A nutrient fertilization experiment in dry Costa Rican forests also found no effect on AMF colonization (Waring et al., 2019). At mid-elevations in Ecuador, AMF colonization rates increased with added P, and AMF biomass increased with added P and N + P, decreasing only with N fertilization (Camenzind et al., 2016). Similarly, in Panama, added N decreased AMF while added P increased AMF (Wurzburger and Wright, 2015; Sheldrake et al., 2018). Also, cation fertilization, but not P fertilization, increased fine root AMF colonization after 1 year of nutrient manipulation in the Central Amazon (Lugli et al., 2021). These mixed responses of AMF to increased nutrient availability presents something of a paradox. One explanation is that plants invest more in AMF symbioses when one scarce nutrient becomes available but other nutrients are still limiting to plant growth, or water is scarce, or a short term response to increased nutrients. It could also be because many studies examine bulked root samples of a whole community response, which can miss species-specific responses. Also, AMF symbiosis is one of several strategies for plants to acquire P, as suggested by a study of five Puerto Rican canopy tree species where there appeared to be a tradeoff among AMF colonization, root phosphatase enzyme activity, or branching density (Yaffar et al., 2021).
While AMF primarily acquire inorganic nutrients, ECM fungi can acquire nutrients in both organic and inorganic forms (Liu et al., 2018), but remain an understudied symbiont in tropical forests. The abundance of ECM did not vary along a natural P gradient in French Guiana (Soong et al., 2020). A long-term N fertilization experiment in a montane Panamanian forest showed decreased ECM abundance in Oreomunnea mexicana roots and a change in the overall ECM genetic composition (Corrales et al., 2017). In contrast, pot experiments with Dipterocarpaceae seedlings, Shorea macroptera (Turner et al., 1993) and Drybalanops lanceolata (Irino et al., 2004) had increased ECM colonization with NPK fertilization. Another study showed no overall effect of P addition on ECM colonization in two Dipterocarp species, but there were contrasting effects of P on individual ECM taxa (Brearley et al., 2007). Thus, ECM responses to changes in nutrient availability are mixed, similar to AMF responses, and the functional response of mycorrhizae to changes in multiple nutrient and water availability requires more attention.
There is a substantial body of literature suggesting that investment in symbiotic N fixation is regulated by both N and P availability in tropical forests. Experiments demonstrate that tropical N-fixing trees generally utilize a facultative fixation strategy in response to soil N scarcity (Menge et al., 2009), as seen following disturbance and during periods of high net biomass accumulation in forests of Panama, Costa Rica, Congo, and Trinidad (Barron et al., 2011; Batterman et al., 2013a; Sullivan et al., 2014; Bauters et al., 2016; Brookshire et al., 2019; Taylor et al., 2019). This facultative response is characterized by downregulation of root nodule biomass and N fixation rates in response to added N, and upregulation of N fixation rates in response to addition of scarce rock-derived nutrients like P and molybdenum, as observed for canopy trees and seedlings in Panama (Barron et al., 2009; Batterman et al., 2013b; Trierweiler et al., 2018). In a Costa Rican dry forest there were large increases in nodule abundance in response to P fertilization (Waring et al., 2019). In Panamanian forests plant phylogeny is the strongest determinant of N fixation rates, but root nodulation and N fixation rates also increased in tree fall gaps, where soil nutrient availability is greater than in surrounding undisturbed forest (Wurzburger and Hedin, 2016). Across nutrient gradients in Panamanian forests, root nodule biomass was inversely related to soil mineral N availability (Barron et al., 2011), but in a wet Costa Rican forest, N fixation rates have been more strongly related to light availability (Taylor and Menge, 2018; McCulloch and Porder, 2021).
Symbiotic Traits: Water Responses
Both ECM and AMF fungi can access soil meso- and micropores with their hyphae that fine roots with relatively larger diameters cannot access (Allen, 2007). Also, ECM can increase plant water access because fungal hyphae can tolerate lower water potentials and continue to absorb moisture when root hairs cannot (Theodorou, 1978). These advantages might enhance plant drought tolerance, extend the plant growing seasons by several weeks into dry periods (Allen and Allen, 1986; Auge, 2001), and reduce root mortality rates during dry periods (Allen, 2011). Not many studies have assessed how mycorrhizal fungi influence plant species responses to drought in tropical forests. A drying experiment conducted on one species in French Guiana showed that AMF colonized seedlings had larger biomass compared to non-colonized seedlings under water stress (Bereau et al., 2005). However, an experiment conducted in a dry forest in Mexico found no benefits for plants inoculated with AMF under water stress compared to non-inoculated plants (Gavito et al., 2008). The role of AMF and ECM in water acquisition across environmental gradients and with sporadic drought requires further investigation in tropical forests.
The link between N fixation and water acquisition is unclear. On the one hand, nodulation and N fixation rates are sensitive to soil moisture (Serraj et al., 1999). Experimental evidence indicates drought negatively affects root nodule N fixation in Neotropical tropical tree seedlings (McCulloch et al., 2021). Seasonal variability in root nodule N fixation activity has also been observed in tropical dry forests, with reduced activity during dry periods (Gei and Powers, 2013). On the other hand, trees with N fixation symbioses are much more abundant in dry than wet tropical forests (Gei et al., 2018; Steidinger et al., 2019), suggesting that the trait conveys a benefit in water use or acquisition. Indeed, Adams et al. (2016) found trees with N fixation symbioses have higher water use efficiency than non-fixing trees. Thus, while symbiotic N fixation itself can be constrained by water (Table 1), it also appears to benefit trees in water-stressed conditions. Direct links between root nodule N fixation activity and water acquisition require further study.
Overall, the functional role of root symbionts for nutrient and water uptake remains poorly characterized for tropical forests. In particular, the interacting or tradeoff effects of symbioses on water versus nutrient acquisition require further study. Measuring these functional roles in the field, and integrating these processes into ecosystem models are crucial next steps for predicting how tropical forests will respond to global change.
Representation of Root Nutrient and Water Acquisition in Terrestrial Vegetation Models
Process-based models of the terrestrial biosphere project ecosystem responses to changing environmental drivers. The ability of vegetation models to meet plant demands for water and nutrients has direct feedbacks on biomass growth, significantly altering coupling of C, water, and other biogeochemical cycles (Fleischer et al., 2019; Trugman et al., 2019; Meyerholt et al., 2020), and mycorrhizal representation can also influence modeled soil C storage (Sulman et al., 2019). Traditionally, model development typically focuses on functions of either water or nutrients alone. Recent efforts indicate that P scarcity might be linked to tropical forest NPP susceptibility to drought, illustrating complex interactions among soil resources (Goll et al., 2018). Most nutrient-enabled models include C and N, and more are including P, but none to date include base cations (Thornton et al., 2007; Yang et al., 2014). Base cation availability and mobility in soils are distinct from phosphate (PO43–) and other forms of P (Schlesinger and Bernhardt, 2020), so model development to incorporate P cycling is not a good proxy for base cation cycling. Because base cations appear to drive root traits and dynamics in many of the studies reviewed above, base cation cycling should be incorporated into vegetation models to improve simulation of tropical forest belowground dynamics.
Within models, root traits can be a parameter, a functional process, or an outcome – it is important to distinguish how root traits are represented in models in order to integrate them with field research. While improving representation of belowground processes is an area of active development in vegetation models, it lags behind more readily observed aboveground processes (Warren et al., 2015; Fisher et al., 2018). For example, belowground biomass is often an outcome of vegetation models, root depth distribution and hydraulic conductance are often set or adjustable parameters, and biomass allocation to roots can be a fixed parameter or is dynamically controlled by resource availability (Table 1). In the IPCC there have been up to 28 Earth system models, and many of these are linked to vegetation models (e.g., the TRENDY set of models) that include root dynamics (Arora et al., 2020). Typically, maximum rooting depth varies with PFT in vegetation models. For example, in a new version of Lund-Potsdam-Jena managed land vegetation model (LPJmL4.0), rooting depth varies from 0.5 to 18 m, depending on allocation of the PFT, but rooting depth also depends on ecosystem factors (Sakschewski et al., 2021). With dynamic C allocation schemes that respond to resource limitation, simulation of accurate root productivity is likely to improve, as indicated in this new version of LPJmL4.0.
Vegetation Models: Nutrient Uptake
While water uptake processes are dominated by gradient-driven mass fluxes in ecosystems, nutrients are mediated by more complex chemical and biological interactions within the soil. Thus, their incorporation into global models has been more gradual, with some large-scale efforts focusing on C, N, and P (Wang et al., 2010; Yang et al., 2014). Model development first focused on implementation of N cycling and acquisition in vegetation models, mostly based on process understanding and observations from temperate forests (Thornton et al., 2007; Bonan and Levis, 2010; Zaehle and Friend, 2010; Zaehle et al., 2014; Arora et al., 2020), with symbiotic N fixation often represented as an empirical function of evapotranspiration or NPP, rather than as a dynamic response to soil N availability (Wieder et al., 2015), but recent analyses have found this approach to have poor agreement with observations (Davies-Barnard and Friedlingstein, 2020). A more mechanistic representation of biological N fixation as a functional tradeoff in C cost among other uptake pathways is becoming more predominant in Earth system models (ESMs) (Fisher et al., 2010, 2019; Shi et al., 2016; Lawrence et al., 2019). At the same time, efforts to realistically represent P cycling and acquisition in vegetation models are surging, largely to improve representation of tropical forests (Yang et al., 2014). In their inter-model comparison, Fleischer et al. (2019) found that inclusion of P cycle constraints reduced biomass accumulation of a tropical site in response to elevated CO2 by approximately 50% compared to C-only and C–N models. Considerable variability in model output among the CNP models was attributed to the different plant P acquisition and usage schemes utilized by the models, so constraining these processes is paramount. However, lack of process understanding and data for parameterization are hampering progress.
Nutrient acquisition schemes vary across model scale and type, and often are not explicitly tied to root traits or dynamics. In most model schemes, nutrient demand by the plant is determined by: (1) fixed or dynamic stoichiometric ratios for each plant tissue type, and (2) allometric parameters that relate nutrients to C allocation among plant tissues (Thornton et al., 2007; Yang et al., 2014). Stoichiometric ratios can be allowed to vary within bounds, as in CABLE, QUINCY, and ORCHIDEE-CNP, reflecting field observations of plasticity in stoichiometric ratios (Meyerholt and Zaehle, 2018). In other models, stoichiometric ratios of C:N and N:P are fixed (e.g., JULES-CN), or vary without bounds (e.g., O-CN, GDAY) (Zaehle and Friend, 2010; Fleischer et al., 2019). Stoichiometric ratios are important features in vegetation models, because they govern plant demand for nutrients, and should be related to root nutrient uptake, nutrient resorption rates and tissue turnover times.
Within the context of plant demand and soil nutrient supply, root biomass and root uptake capacity additionally determine nutrient uptake rates in some vegetation models, such as ORCHIDEE-CNP and GDAY (Goll et al., 2017). Some models consider Michaelis-Menten kinetics of ammonium and nitrate uptake capacity per root biomass, for example O–CN (Zaehle and Friend, 2010), while others consider N uptake to be a function of plant N demand and cohort partitioning of fine root surface area, for example LPJ-GUESS (Smith et al., 2014). Among these models, none consider the functionality of fine root morphological traits like SRL for N or P uptake. Thus, more extensive data on root functionality, and how this relates to commonly measured root traits and to nutrient availability across tropical forests, could improve nutrient acquisition and allocation representation in vegetation models.
Vegetation models also implement a range of assumptions about competition for soil nutrients among plants, soil microbes, and mineral surfaces, which can modulate P availability in particular in tropical soils, as in CLM-ECA and the Jena Soil Model (JSM) (Zhu et al., 2016; Yu et al., 2020). For P acquisition, most models include the biochemical mineralization of organic P through phosphatase enzyme activity, but functional representation differs across models, with most models using a relatively simple control of plant demand or soil P availability over phosphatase-based mineralization (Fleischer et al., 2019). Yang et al. (2016) identified phosphatase as a critical response variable for vegetation biomass production under low fertility conditions. However, observations of tropical root phosphatase activity are scarce, and functional understanding of controls over phosphatase activity in tropical forests is limited. Some recent field data from Puerto Rico suggest that phosphatase activity in the rhizosphere and on root surfaces are more closely related to root P content and soil P availability than are bulk soil enzyme activities (Cabugao et al., 2021), so direct measures of enzyme activities on roots are needed. In most of these models, production of phosphatase enzymes and other investments in nutrient acquisition come at no extra C costs to plants. Approaches in models that focus more specifically on nutrient uptake determine N and P uptake rates as a balance of dynamically-varying C costs among different uptake pathways, including AMF and ECM symbioses, direct root uptake, leaf retranslocation, and biological N fixation, with pathways varying relative to demand for plant growth and maintenance, such as in the Fixation and Uptake of Nutrients (FUN and FUN-P) nutrient uptake models (Fisher et al., 2010; Brzostek et al., 2014; Allen et al., 2020), which can be coupled to vegetation models and embedded in land surface models to improve nutrient uptake process-scale representation (Shi et al., 2016). The relationship of root nutrient uptake rate with other more commonly measured root traits, like morphological traits, production, and mortality, would be useful for improving nutrient uptake representation for tropical forests in vegetation models.
Vegetation Models: Water Uptake
Earth system models have classically represented water uptake as a function of atmospheric demand and root biomass, with water uptake constrained under dry soil conditions by a simple linear function (Feddes et al., 1976). While computationally inexpensive, this approach has been shown to underestimate water uptake under water-limited or drought conditions (Feddes et al., 2001). More recently, there has been a push for ESMs to incorporate more mechanistic plant hydraulics schemes (rather than empirical functions), which capture the plasticity of plant ecohydrology under drying conditions (e.g., hydraulic redistribution, compensatory uptake) (Eller et al., 2020), and can feedback to modify local climate, as indicated when Amazon hydraulic redistribution was incorporated into an atmospheric circulation model (Lee et al., 2005).
Root-mediated water uptake in larger land surface and climate models has evolved from purely zero-dimensional empirical functions that represent water uptake as a function of rooting depth, to one- and two-dimensional formulations which account for the vertical distribution of root biomass within the soil (Warren et al., 2015; Fatichi et al., 2016). Early implementations were biased toward the early onset of water limitation to plants, unable to fully resolve field observations under drying conditions (Feddes et al., 2001). Additional functions, or “correction factors,” were later added to account for hydraulic redistribution and compensatory uptake, processes observationally shown to mediate plant water stress (Oliveira et al., 2005; Stahl et al., 2013), but these functions lack connection with measurable root traits (Feddes and Roats, 2004).
Recent work has demonstrated the importance of improving representation of fine roots to model ecosystem and regional-scale hydraulics (Fatichi et al., 2016; Xu and Trugman, 2021). Yet, aside from vertical root biomass and root depth distributions, approaches in terrestrial vegetation models are still largely decoupled from the measured root traits and dynamics reviewed above. These models typically rely on static model parameters inferred to match site-specific conditions and observed water fluxes. Recent advances in root representation in models include the addition of plant hydrodynamics modules to larger vegetation models, aimed at increasing the fidelity of feedbacks between water acquisition and plant growth (Christoffersen et al., 2016; Xu et al., 2016; Kennedy et al., 2019). Similar to finer scale models, water uptake is driven by water potential gradients within the soil-plant-atmosphere continuum in these modules, resolving processes like hydraulic redistribution and compensatory uptake. In these modules, which are relatively good at modeling plant mortality, important drivers of water uptake include: vertical root depth distribution, and hydraulic resistance between soil and the rhizosphere (typically measured by root hydraulic conductance) (Christoffersen et al., 2016; Kennedy et al., 2019). Plant hydrodynamics implemented within CLM5 by Kennedy et al. (2019) quantified soil to root conductance as a function of root hydraulic conductivity and root surface area per unit ground area, so fine root SRL can play a role in these models. In FATES-Hydro (previously published as TFS v.1-Hydro; Christoffersen et al., 2016), the rhizosphere was distributed as shells layered around roots in the soil column, with soil to root hydraulic conductance scaled as a function of root biomass depth distribution, defined as an exponential decay function, as well as SRL and root hydraulic conductivity. Both approaches incorporate measurable root characteristics to improve representation of water uptake and effects on plant NPP, however, the paucity of tropical forest field observations has led to inference of parameters using more readily available aboveground proxies, with whole root conductance designated as a fraction of modeled aboveground biomass or resistance.
Beyond plant hydrodynamics models, which incorporate the entire soil-plant-atmospheric continuum, there are approaches which focus on resolving more detailed and mechanistic root structure and function with the computational scalability needed for larger scale simulations. These smaller scale models incorporate root morphology and physiology, with spatiotemporal patterns of water uptake emerging as a result of interactions between the physical soil environment and root biology (Doussan et al., 2006). These models capture spatial and temporal shifts in plant water sourcing driven by individual and competitive interactions among plants within the system (Manoli et al., 2014; Agee et al., 2021). A modeling study using 3 years of seasonal drought data from the Amazon suggested that deep roots with niche partitioning adaptations, which source water from different depths in the soil during different times of year, explained observational resilience to seasonal drought and vulnerability to severe episodic drought (Ivanov et al., 2012). This study added to previous representations by generating multiple root biomass depth distributions for different tree size classes, creating “root niche segregation” profiles, which are more closely aligned with site observations than earlier models without multiple root depth profiles.
Linking these smaller scale models to larger scale function, Couvreur et al. (2012) developed a hybrid micro-/macroscale scheme which reduces root system architecture and hydraulic traits (e.g., radial and axial root hydraulic conductivity) at the individual scale to develop scalable macroscale parameters which maintain important hydraulic information. This approach was implemented in HYDRUS (Baram et al., 2016; Cai et al., 2018), and CLM models (Sulis et al., 2019). However, these fine-scale approaches require large amounts of data to parameterize and validate model outcomes, constituting a significant challenge to scaling beyond a few individuals or a local region (Javaux et al., 2013). Linking small-scale root traits and root function representation into these larger scale models remains a frontier for accurate representation of root water uptake, particularly for biomes like tropical forests with scarce data.
Notably, fine root morphology is one of the more common sets of root traits used in hydraulically-enabled vegetation models. For example, SRL is used for converting fine root biomass values into total fine root length, which represents the surface area for resource uptake, and soil-root hydraulic resistance (Xu and Trugman, 2021). Thus, clarifying the functional relationships between fine root morphology and resource acquisition could be utilized within existing model frameworks to improve representation of tropical forests.
Frontiers in Tropical Root Acquisition Research
Following the review of available literature above, we suggest research avenues that would be useful to better understand and model tropical root nutrient and water acquisition across tropical forests under global change scenarios of resource change.
What Are the Key Root Traits for Inferring Ecosystem Function in Tropical Forests?
Most of the vegetation modeling efforts to better represent nutrient uptake do not include root traits or function, and there remains a gulf between the data field ecologists collect and the parameters that drive models. The assumption that plant investments in different nutrient and water acquisition mechanisms depends on a C cost-benefit tradeoff. This assumption is currently not widely used in vegetation models, but C costs of resource uptake are increasingly considered (Fisher et al., 2010, 2018). In the field, determining the C cost per unit of nutrient or water acquired and how this varies along nutrient and moisture gradients is extremely difficult. However, studies reviewed here have already made advances in determining how plants are investing their C belowground (e.g., root length construction, phosphatase, organic acids exudation, and mycorrhizal associations at the community scale). These assessments are very helpful to constrain C investments in nutrient and water acquisition in vegetation models. Complementary studies in controlled settings could further assess the C cost of different resource acquisition strategies across soil fertility and moisture levels.
Also, key root traits related to nutrient uptake rate might not be the traits we commonly measure in the field, such as SRL and root N (Freschet et al., 2021), and root traits important in tropical forests might not be the same as the key root traits in temperate ecosystems. In particular, P is commonly a limiting nutrient in tropical forests, while N is generally limiting in temperate forests. The importance of accurately modeling P was demonstrated in an assessment of uncertainty among models for predicting Amazon forest biomass response to increased atmospheric CO2 across 14 vegetation models. Among models that included the C, N, and P cycles, uncertainty in biomass C response to increased atmospheric CO2 was attributed primarily to the representation of different P use and P acquisition strategies among models, which are challenging to constrain due to the lack of data (Fleischer et al., 2019). For example, plant strategies for P uptake a very different from those for N uptake (e.g., root exudates and AMF association for P versus ECM and N fixing bacteria association for N) (Weemstra et al., 2016; Freschet et al., 2021), so models designed for forests with soil N scarcity cannot easily be used to simulate dynamics in tropical forests with P scarcity. The tropical field studies reviewed here indicate that fine root biomass can decline when soil P and base cations are plentiful, and that rock-derived nutrients, particularly P and K, drive root biomass stocks and depth distributions across tropical forests (Table 1; note that results from fertilization studies were more mixed). Thus, fine root biomass measures should be paired with measures of P and cation availability, cycling, and root uptake to increase process-level understanding that can be incorporated into model development.
Tradeoffs in morphological and physiological traits should be paired with more detailed studies in root anatomy in order to separate short-term functional changes from potentially longer-term structural changes. Changes in root morphology, architecture and physiology are ultimately regulated by the relative areal investment between cortical and stele (vascular) tissue, a trait scarcely included in root ecology studies, but which could be related to variation in fine root SRL, RTD, diameter, and N content (Figure 3). The fact that roots of tropical species showed relatively thicker, low SRL, and denser roots compared to other ecosystems suggests a greater dependency on mycorrhizal associations in this biome (Freschet et al., 2017; Wang et al., 2018), although there is a wide range of variation in tropical root morphological and architectural traits (Ma et al., 2018; Shen et al., 2019). This suggests that species can vary their allocation to cortical tissues according to local nutrient availability, as a way to decrease C investment in symbiotic associations and increase root surface area (Kong et al., 2021). In relation to water uptake, Eucalyptus roots in Brazil can present fewer xylem vessels but with larger diameter at depths greater than 10 m compared to shallow roots (Germon et al., 2020), which suggests that changes in anatomical traits in deeper soil layers might increase hydraulic efficiency for that species. Studies of grasses in Japan indicate that proportional allocation to different root tissues (e.g., stele, cortex, xylem, or aerenchyma) is one response to moisture gradients (Yamauchi et al., 2021). However, there is a lack of studies assessing root anatomical traits along nutrient or moisture gradients in tropical areas.
To identify key traits related to tropical forest function, and to address the recurring theme of general lack of field data for tropical forests, we suggest an assessment of the spatial patterns in community-scale traits across tropical bioregions and continents, and comparison with temperate patterns. Standardized root traits and metrics across studies would greatly help in assessment of the tropics as a whole. For example, multiple depths should be assessed for fine root biomass and morphological measures like diameter across studies, so that depth distribution differences can be assessed. These types of comparable and large-scale biogeographic study would help us understand whether a particular trait/response is a genuine pantropical forest pattern, versus a regional or forest type-specific process.
What Are Tropical Root Trait Tradeoffs and Synergies for Nutrient and Water Acquisition?
Key questions remain about tradeoffs and synergies among root traits involved in nutrient and water uptake across tropical forests. In assessing tradeoffs, studies should cover the full resource spectrum, low to high soil fertility and low to high water availability, to assess the full niche space (Silvertown et al., 2015). Many of the above studies investigate how morphological or physiological traits of tropical plants respond to drought, but far fewer investigate the response to flooding, thus omitting the high end of the water availability spectrum, i.e., flooded forests, peat swamp forests, etc. Greater investigation into tradeoffs and synergies among root traits could offer great mechanistic insight to strategies employed by tropical trees. For example, research on the timing and extent of root enzyme activities and/or root exudation, and water uptake, would also inform questions about root trait tradeoffs and synergies for nutrient and water uptake.
To assess these questions, we suggest coordinated studies and manipulation experiments that directly assess water versus nutrient uptake at the community level. For example, natural experiments across biogeographic gradients where rainfall and soil nutrient availability vary could be used more to examine key root traits related to water and nutrient uptake would be useful. Alternatively, large-scale nutrient and rainfall manipulation experiments could address responses to water limitation with changes in nutrient availability. The bulk of the evidence in this review comes from gradient studies and fertilization experiments, which are correlative. To understand functional tradeoffs and synergies among root traits, we also suggest greater emphasis on nutrient tracing experiments with isotopically-labeled nutrient additions to provide greater mechanistic information.
What Are the Cross-Scale Patterns of Root Trait Plasticity in Tropical Forests Undergoing Changing Water and Nutrient Availability?
The plasticity of root depth distribution in response to episodic drought, both at species and community scales, is likely to be crucial for survival in a future drier climate. Some tropical tree species appear to have limited capacity to shift root depth distribution in response to sudden moisture changes, as was shown in a stand-level irrigation experiment in Panama (Yavitt and Wright, 2001). A study in French Guiana using a dual isotope labeling approach found that half of the 65 trees from 47 species studied accessed deep soil water below 100 cm depth during dry periods, and nine individuals had great plasticity in their depth of water uptake, taking advantage of surface water and nutrients during rain events, and quickly switching to deep water stores during dry conditions (Stahl et al., 2013).
We suggest investigating the plasticity of root depth distributions and productivity in response to changes in water availability over seasonal shifts in tropical forests, as well as in response to chronic drying (i.e., incremental reductions in annual rainfall), and increased intensity and frequency of extended drought. For example, studies could assess intraspecific variation in root trait plasticity across multiple species, and investigate the ability of different species to respond to changes in water and/or nutrient availability. One approach could be sampling root traits for individuals at the centers and edges of species’ environmental distribution, and sampling rare species with distinct strategies. This approach could help us identify the range (the extremes) of the tropical belowground trait space.
Should Vegetation Models Represent Plasticity and Sensitivity of Individual Plants or Community-Level Trends for Water and Nutrient Acquisition?
A major question facing vegetation modelers seeking to accurately represent tropical forests is how to incorporate empirical process understanding at the species- or community-level, and use that to create an intermediate scale that aggregates responses of individual plants as functional strategies that can compete with one another within the ecosystem. Thus, functional strategies would be defined to represent the diversity of competitive strategies. Defining these functional types is a challenge, and one reason why many models have explored using belowground economic spectrum analogies. However, belowground empirical studies have demonstrated that simple economic spectrums similar to leaf economic spectrums are inadequate to describe root system tradeoffs (Bergmann et al., 2020). Appropriate groupings for root traits and functions would reproduce plant responses to resource gradients of water and nutrients in tropical forests. These groupings could then be used to forecast plant water, C, and nutrient dynamics under changing climate, as well as shifts in forest composition. A better representation of belowground functional strategy groups within diverse tropical forests would facilitate linkages to aboveground traits and processes. Because of the diversity of tropical forests, characterizing emergent trends in communities over seasonal timescales in the field is likely the most attainable and useful approach to link with modeling endeavors.
Conclusion
Fine roots represent the primary site of water and nutrient uptake in tropical forest trees. Because water and nutrients directly constrain plant growth, the traits and functions of fine roots are centrally important to tropical forest growth, nutrient cycling, and responses to climatic changes. This review assessed root traits through the lenses of biomass, depth distributions, phenology, morphology, physiology, and symbiosis, and modeling efforts to represent tropical root traits at small to large scales. We identified emerging trends in the literature that tropical fine root biomass and production in surface soils are greatest under nutrient scarcity or water abundance. We also found that SRL, which is typically thought to increase under resource scarcity to expand the volume of soil explored, instead increased with greater base cation availability both across natural gradients and in fertilization experiments. The combined literature indicates that the response of the microbial-root symbiosis to changing nutrient levels across tropical forest gradients depends on baseline nutrient concentrations and relative nutrient or water limitation to plant and fungal production. For example, nutrient additions, rather than reducing mycorrhizal colonization of fine roots as might be expected, increased colonization rates under scenarios of water scarcity in some sites.
Efforts to model the traits and functions of fine roots have grown more sophisticated over time, yet there is a disconnect between the emphasis in models on characterizing nutrient and water uptake rates and C costs, and the strong emphasis in field experiments on measuring root biomass, production, and morphology in response to changes in resource availability. Empirical understanding of how water and nutrients, particularly base cations, influence root depth distributions, SRL, physiological root traits, and microbe-root symbioses across tropical forests is still limited, and yet the recent surge in tropical root trait studies provides enough evidence for process-based representation in vegetation models.
We highlight several frontiers for research on tropical forest root nutrient and water uptake: (1) identifying key root traits for predicting ecosystem function and aboveground linkages, (2) connecting commonly measured root morphological traits with physiological and anatomical traits such as hydraulic conductance and nutrient uptake rates for which they currently often serve as proxies, (3) elucidating fine root trait tradeoffs and synergies with microbial symbionts for nutrient and water acquisition, and (4) investigating the extent and scales of plasticity in root trait responses to environmental changes. Closer integration of field and modeling efforts could connect mechanistic investigation of fine-root dynamics to ecosystem-scale understanding of nutrient and water cycling, allowing us to better predict tropical forest-climate feedbacks.
Orcid
Daniela Francis Cusack
Shalom D. Addo-Danso
Elizabeth A. Agee
Kelly M. Andersen
Marie Arnaud
Sarah A. Batterman
Francis Q. Brearley
Amanda L. Cordeiro
Caroline Dallstream
Milton H. Diaz-Toribio
Lee H. Dietterich
Joshua B. Fisher
Katrin Fleischer
Claire Fortunel
Lucia Fuchslueger
Nathaly R. Guerrero-Ramírez
Martyna M. Kotowska
Laynara Figueiredo Lugli
César Marín
Lindsay A. McCulloch
Jean-Luc Maeght
Dan Metcalfe
Richard J. Norby
Rafael S. Oliveira
Jennifer S. Powers
Tatiana Reichert
Stuart W. Smith
Fiona M. Soper
Laura Toro
María N. Umaña
Oscar Valverde-Barrantes
Monique Weemstra
Leland K. Werden
Michelle Wong
Cynthia L. Wright
Stuart Joseph Wright
Daniela Yaffar
Author Contributions
All authors helped conceive of write, and edit the article, and all approved the submitted version.
Conflict of Interest
The authors declare that the research was conducted in the absence of any commercial or financial relationships that could be construed as a potential conflict of interest.
Publisher’s Note
All claims expressed in this article are solely those of the authors and do not necessarily represent those of their affiliated organizations, or those of the publisher, the editors and the reviewers. Any product that may be evaluated in this article, or claim that may be made by its manufacturer, is not guaranteed or endorsed by the publisher.
Funding
The U.S. Department of Energy Office of Biological and Environmental Research (DOE-BER), Terrestrial Ecosystem Science program supported this research under Early Career Award Number DESC0015898 to DFC, and DE-SC0008317 and DE-SC0016188 to JF and the NGEE-Tropics program support for EA, CW, and DY. JF contributed in part at the Jet Propulsion Laboratory, California Institute of Technology, under contract with the National Aeronautics and Space Administration, California Institute of Technology. NG-R was supported by the Dorothea Schl zer Postdoctoral Programme of the Georg-August-Universit t Goettingen. The Royal Society Leverhulme Africa Postdoctoral Fellowships. Grant No. LAF\R1\180025 provided funding to SA-D. Amazon FACE/CAPES grant 88887.154643/2017-00 provided support for LL. The US National Science Foundation (DEB-2016678) funded MU. European Union Horizon 2020 under the Mari Skłodovska-Curie grant agreement (No. 847693, REWIRE) provided funding to LF. Data storage and some synthesis activities were supported as part of the Next Generation Ecosystem Experiments – Tropics, funded by DOE-BER. National Science Foundation Research Coordination Grant INCyTE: DEB-1754126 to investigate nutrient cycling in terrestrial ecosystems. United Kingdom Natural Environment Research Council (NE/M019497/1, NE/S009663/1) and The Leverhulme Trust supported SAB.
Acknowledgments
A New Phytologist Workshop supported the collaboration of the TropiRoot group that produced this review. N. E. Binte Rahman and M. Y. Lee assisted with initial literature searches.
Supplementary Material
The Supplementary Material for this article can be found online at: https://www.frontiersin.org/articles/10.3389/ffgc.2021.704469/full#supplementary-material
References
Abrahao, A., Costa, P. D., Lambers, H., Andrade, S. A. L., Sawaya, A., Ryan, M. H., et al. (2019). Soil types select for plants with matching nutrient-acquisition and -use traits in hyperdiverse and severely nutrient-impoverished campos rupestres and cerrado in Central Brazil. J. Ecol. 107, 1302–1316. doi: 10.1111/1365-2745.13111
Abrahao, A., Lambers, H., Sawaya, A., Mazzafera, P., and Oliveira, R. S. (2014). Convergence of a specialized root trait in plants from nutrient-impoverished soils: phosphorus-acquisition strategy in a nonmycorrhizal cactus. Oecologia 176, 345–355. doi: 10.1007/s00442-014-3033-4
Adame, F., Teutli, C., Santini, N. S., Caamal, J. P., Zaldivar-Jimenez, A., Hernandez, R., et al. (2014). Root biomass and production of mangroves surrounding a karstic oligotrophic coastal lagoon. Wetlands 34, 479–488. doi: 10.1007/s13157-014-0514-5
Adame, M. F., Cherian, S., Reef, R., and Stewart-Koster, B. (2017). Mangrove root biomass and the uncertainty of belowground carbon estimations. For. Ecol. Manag. 403, 52–60. doi: 10.1016/j.foreco.2017.08.016
Adams, M. A., Turnbull, T. L., Sprent, J. I., and Buchmann, N. (2016). Legumes are different: leaf nitrogen, photosynthesis, and water use efficiency. Proc. Natl. Acad. Sci. U.S.A. 113, 4098–4103. doi: 10.1073/pnas.1523936113
Addo-Danso, S. D., Defrenne, C. E., McCormack, M. L., Ostonen, I., Addo-Danso, A., Foli, E. G., et al. (2020). Fine-root morphological trait variation in tropical forest ecosystems: an evidence synthesis. Plant Ecol. 221, 1–13. doi: 10.1007/s11258-019-00986-1
Agee, E., He, L., Bisht, G., Couvreur, V., Shahbaz, P., Meunier, F., et al. (2021). Root lateral interactions drive water uptake patterns under water limitation. Adv. Water Resour. 151:103896. doi: 10.1016/j.advwatres.2021.103896
Aguirre-Gutierrez, J., Oliveras, I., Rifai, S., Fauset, S., Adu-Bredu, S., Affum-Baffoe, K., et al. (2019). Drier tropical forests are susceptible to functional changes in response to a long-term drought. Ecol. Lett. 22, 855–865. doi: 10.1111/ele.13243
Aleixo, I., Norris, D., Hemerik, L., Barbosa, A., Prata, E., Costa, F., et al. (2019). Amazonian rainforest tree mortality driven by climate and functional traits. Nat. Clim. Change 9, 384–388. doi: 10.1038/s41558-019-0458-0
Allen, E. B., and Allen, M. F. (1986). Water relations of xeric grasses in the field – interactions of mycorrhizas and competition. New Phytol. 104, 559–571. doi: 10.1111/j.1469-8137.1986.tb00656.x
Allen, K., Fisher, J. B., Phillips, R. P., Powers, J. S., and Brzostek, E. R. (2020). Modeling the carbon cost of plant nitrogen and phosphorus uptake across temperate and tropical forests. Front. For. Glob. Change 3:12. doi: 10.3389/ffgc.2020.00043
Allen, M. F. (2007). Mycorrhizal fungi: highways for water and nutrients in arid soils. Vadose Zone J. 6, 291–297. doi: 10.2136/vzj2006.0068
Allen, M. F. (2011). Linking water and nutrients through the vadose zone: a fungal interface between the soil and plant systems. J. Arid Land 3, 155–163. doi: 10.3724/sp.J.1227.2011.00155
Alongi, D. M. (2018). Impact of global change on nutrient dynamics in mangrove forests. Forests 9:596. doi: 10.3390/f9100596
Andersen, K. M., and Turner, B. L. (2013). Preferences or plasticity in nitrogen acquisition by understorey palms in a tropical montane forest. J. Ecol. 101, 819–825. doi: 10.1111/1365-2745.12070
Andersen, K. M., Mayor, J. R., and Turner, B. L. (2017). Plasticity in nitrogen uptake among plant species with contrasting nutrient acquisition strategies in a tropical forest. Ecology 98, 1388–1398. doi: 10.1002/ecy.1793
Andrade, J. L., Meinzer, F. C., Goldstein, G., and Schnitzer, S. A. (2005). Water uptake and transport in lianas and co-occurring trees of a seasonally dry tropical forest. Trees Struct. Funct. 19, 282–289. doi: 10.1007/s00468-004-0388-x
Aoki, M., Fujii, K., and Kitayama, K. (2012). Environmental control of root exudation of low-molecular weight organic acids in tropical rainforests. Ecosystems 15, 1194–1203. doi: 10.1007/s10021-012-9575-6
Aragao, L., Malhi, Y., Metcalfe, D. B., Silva-Espejo, J. E., Jimenez, E., Navarrete, D., et al. (2009). Above- and below-ground net primary productivity across ten Amazonian forests on contrasting soils. Biogeosciences 6, 2759–2778. doi: 10.5194/bg-6-2759-2009
Arora, V. K., Katavouta, A., Williams, R. G., Jones, C. D., Brovkin, V., Friedlingstein, P., et al. (2020). Carbon-concentration and carbon-climate feedbacks in CMIP6 models and their comparison to CMIP5 models. Biogeosciences 17, 4173–4222. doi: 10.5194/bg-17-4173-2020
Auge, R. M. (2001). Water relations, drought and vesicular-arbuscular mycorrhizal symbiosis. Mycorrhiza 11, 3–42. doi: 10.1007/s005720100097
Baram, S., Couvreur, V., Harter, T., Read, M., Brown, P. H., Kandelous, M., et al. (2016). Estimating nitrate leaching to groundwater from orchards: comparing crop nitrogen excess, deep vadose zone data-driven estimates, and HYDRUS modeling. Vadose Zone J. 15, 1–13. doi: 10.2136/vzj2016.07.0061
Bardgett, R., Mommer, L., and Vries, F. D. (2014). Going underground: root traits as drivers of ecosystem processes. Trends Ecol. Evol. 29, 692–699.
Barkhordarian, A., Saatchi, S. S., Behrangi, A., Loikith, P. C., and Mechoso, C. R. (2019). A recent systematic increase in vapor pressure deficit over tropical South America. Sci. Rep. 9:12. doi: 10.1038/s41598-019-51857-8
Barron, A. R., Purves, D. W., and Hedin, L. O. (2011). Facultative nitrogen fixation by canopy legumes in a lowland tropical forest. Oecologia 165, 511–520. doi: 10.1007/s00442-010-1838-3
Barron, A. R., Wurzburger, N., Bellenger, J. P., Wright, S. J., Kraepiel, A. M. L., and Hedin, L. O. (2009). Molybdenum limitation of asymbiotic nitrogen fixation in tropical forest soils. Nat. Geosci. 2, 42–45.
Barros, F. D., Bittencourt, P. R. L., Brum, M., Restrepo-Coupe, N., Pereira, L., Teodoro, G. S., et al. (2019). Hydraulic traits explain differential responses of Amazonian forests to the 2015 El Nino-induced drought. New Phytol. 223, 1253–1266. doi: 10.1111/nph.15909
Bates, T. R., and Lynch, J. P. (2001). Root hairs confer a competitive advantage under low phosphorus availability. Plant Soil 236, 243–250. doi: 10.1023/a:1012791706800
Batterman, S. A., Hall, J. S., Turner, B. L., Hedin, L. O., Walter, J. K. L., Sheldon, P., et al. (2018). Phosphatase activity and nitrogen fixation reflect species differences, not nutrient trading or nutrient balance, across tropical rainforest trees. Ecol. Lett. 21, 1486–1495. doi: 10.1111/ele.13129
Batterman, S. A., Hedin, L. O., Breugel, M. V., Ransijn, J., Craven, D., and Hall, J. (2013a). Key role of symbiotic dinitrogen fixation in tropical forest secondary succession. Nature 502, 224–227.
Batterman, S. A., Wurzburger, N., and Hedin, L. O. (2013b). Nitrogen and phosphorus interact to control tropical symbiotic N-2 fixation: a test in Inga punctata. J. Ecol. 101, 1400–1408. doi: 10.1111/1365-2745.12138
Bauters, M., Mapenzi, N., Kearsley, E., Vanlauwe, B., and Boeckx, P. (2016). Facultative nitrogen fixation by legumes in the central Congo basin is downregulated during late successional stages. Biotropica 48, 281–284. doi: 10.1111/btp.12312
Beinroth, F. H., Vázquez, M. A., Snyder, V. A., Reich, P. F., and Pérez Alegria, L. R. (1996). Factors Controlling Carbon Sequestration in Tropical Soils: A Case Study of Puerto Rico. San Juan: USDA Natural Resources Conservation Service World Soil Resources and Caribbean Area Office, 35.
Bereau, M., Bonal, D., Louisanna, E., and Garbaye, J. (2005). Do mycorrhizas improve tropical tree seedling performance under water stress and low light conditions? A case study with Dicorynia guianensis (Caesalpiniaceae). J. Trop. Ecol. 21, 375–381. doi: 10.1017/s0266467405002348
Bergmann, J., Weigelt, A., van Der Plas, F., Laughlin, D. C., Kuyper, T. W., Guerrero-Ramirez, N. R., et al. (2020). The fungal collaboration gradient dominates the root economics space in plants. Sci. Adv. 6:eaba3756. doi: 10.1126/sciadv.aba3756
Berish, C. W. (1982). Root biomass and surface area in three successional tropical forests. Can. J. For. Res. Revue Can. Recherche For. 12, 699–704. doi: 10.1139/x82-104
Bloom, A. J., Chapin, F. S., and Mooney, H. A. (1985). Resource limitation in plants – an economic analogy. Annu. Rev. Ecol. Syst. 16, 363–392. doi: 10.1146/annurev.es.16.110185.002051
Bonan, G. B., and Levis, S. (2010). Quantifying carbon-nitrogen feedbacks in the Community Land Model (CLM4). Geophys. Res. Lett. 37:L07401. doi: 10.1029/2010gl042430
Brearley, F. Q. (2013). Nitrogen stable isotopes indicate differences in nitrogen cycling between two contrasting Jamaican montane forests. Plant Soil 367, 465–476. doi: 10.1007/s11104-012-1469-z
Brearley, F. Q., Elliott, D. R., Iribar, A., and Sen, R. (2016). Arbuscular mycorrhizal community structure on co-existing tropical legume trees in French Guiana. Plant Soil 403, 253–265. doi: 10.1007/s11104-016-2818-0
Brearley, F. Q., Scholes, J. D., Press, M. C., and Palfner, G. (2007). How does light and phosphorus fertilisation affect the growth and ectomycorrhizal community of two contrasting dipterocarp species? Plant Ecol. 192, 237–249. doi: 10.1007/s11258-007-9325-6
Brodribb, T. J., Powers, J., Cochard, H., and Choat, B. (2020). Hanging by a thread? Forests and drought. Science 368, 261–266. doi: 10.1126/science.aat7631
Broedel, E., Tomasella, J., Cândido, L. A., and Von Randow, C. (2017). Deep soil water dynamics in an undisturbed primary forest in central Amazonia: differences between normal years and the 2005 drought. Hydrol. Processes 31, 1749–1759.
Brookshire, E. N. J., Wurzburger, N., Currey, B., Menge, D. N. L., Oatham, M. P., and Roberts, C. (2019). Symbiotic N fixation is sufficient to support net aboveground biomass accumulation in a humid tropical forest. Sci. Rep. 9:7571. doi: 10.1038/s41598-019-43962-5
Brouwer, R. (1963). Some physiological aspects of the influence of growth factors in the root medium on growth and dry matter productio. Med. Inst Bioo Scheikundig Onderz Landbouwgewassen 212-219, 211–230.
Brum, M., Vadeboncoeur, M. A., Ivanov, V., Asbjornsen, H., Saleska, S., Alves, L. F., et al. (2019). Hydrological niche segregation defines forest structure and drought tolerance strategies in a seasonal Amazon forest. J. Ecol. 107, 318–333. doi: 10.1111/1365-2745.13022
Brundrett, M. C. (2009). Mycorrhizal associations and other means of nutrition of vascular plants: understanding the global diversity of host plants by resolving conflicting information and developing reliable means of diagnosis. Plant Soil 320, 37–77. doi: 10.1007/s11104-008-9877-9
Brundrett, M. C., and Tedersoo, L. (2018). Evolutionary history of mycorrhizal symbioses and global host plant diversity. New Phytol. 220, 1108–1115. doi: 10.1111/nph.14976
Brzostek, E. R., Fisher, J. B., and Phillips, R. P. (2014). Modeling the carbon cost of plant nitrogen acquisition: mycorrhizal trade-offs and multipath resistance uptake improve predictions of retranslocation. J. Geophys. Res. Biogeosci. 119, 1684–1697. doi: 10.1002/2014jg002660
Cabugao, K. G., Yaffar, D., Stenson, N., Childs, J., Phillips, J., Mayes, M. A., et al. (2021). Bringing function to structure: root-soil interactions shaping phosphatase activity throughout a soil profile in Puerto Rico. Ecol. Evol. 11, 1150–1164. doi: 10.1002/ece3.7036
Cai, G., Vanderborght, J., Couvreur, V., Mboh, C. M., and Vereecken, H. (2018). Parameterization of root water uptake models considering dynamic root distributions and water uptake compensation. Vadose Zone J. 17, 1–21. doi: 10.2136/vzj2016.12.0125
Camenzind, T., Homeier, J., Dietrich, K., Hempel, S., Hertel, D., Krohn, A., et al. (2016). Opposing effects of nitrogen versus phosphorus additions on mycorrhizal fungal abundance along an elevational gradient in tropical montane forests. Soil Biol. Biochem. 94, 37–47. doi: 10.1016/j.soilbio.2015.11.011
Camenzind, T., Philipp Grenz, K., Lehmann, J., and Rillig, M. C. (2021). Soil fungal mycelia have unexpectedly flexible stoichiometric C:N and C:P ratios. Ecol. Lett. 24, 208–218. doi: 10.1111/ele.13632
Carminati, A., and Vetterlein, D. (2013). Plasticity of rhizosphere hydraulic properties as a key for efficient utilization of scarce resources. Ann. Bot. 112, 277–290. doi: 10.1093/aob/mcs262
Carminati, A., Vetterlein, D., Weller, U., Vogel, H. J., and Oswald, S. E. (2009). When roots lose contact. Vadose Zone J. 8, 805–809. doi: 10.2136/vzj2008.0147
Castaneda-Moya, E., Twilley, R. R., Rivera-Monroy, V. H., Marx, B. D., Coronado-Molina, C., and Ewe, S. M. L. (2011). Patterns of root dynamics in mangrove forests along environmental gradients in the florida coastal everglades, USA. Ecosystems 14, 1178–1195. doi: 10.1007/s10021-011-9473-3
Cavelier, J. (1992). Fine root biomass and soil properties in a semideciduous and a lower montane rainforest in Panama. Plant Soil 142, 187–201. doi: 10.1007/bf00010965
Cavelier, J., Wright, S. J., and Santamaria, J. (1999). Effects of irrigation on litterfall, fine root biomass and production in a semideciduous lowland forest in Panama. Plant Soil 211, 207–213. doi: 10.1023/a:1004686204235
Chacón, N., Dezzeo, N., Rangel, M., and Flores, S. (2008). Seasonal changes in soil phosphorus dynamics and root mass along a flooded tropical forest gradient in the lower Orinoco river, Venezuela. Biogeochemistry 87, 157–168. doi: 10.1007/s10533-007-9174-3
Chadwick, R., Good, P., Martin, G., and Rowell, D. P. (2016). Large rainfall changes consistently projected over substantial areas of tropical land. Nat. Clim. Change 6, 177–181. doi: 10.1038/nclimate2805
Chitra-Tarak, R., Ruiz, L., Dattaraja, H. S., Kumar, M. S. M., Riotte, J., Suresh, H. S., et al. (2018). The roots of the drought: hydrology and water uptake strategies mediate forest-wide demographic response to precipitation. J. Ecol. 106, 1495–1507. doi: 10.1111/1365-2745.12925
Christoffersen, B. O., Gloor, M., Fauset, S., Fyllas, N. M., Galbraith, D. R., Baker, T. R., et al. (2016). Linking hydraulic traits to tropical forest function in a size-structured and trait-driven model (TFS v.1-Hydro). Geosci. Model Dev. 9, 4227–4255. doi: 10.5194/gmd-9-4227-2016
Ciais, P., Sabine, C., Govindasamy, B., Bopp, L., Brovkin, V., Canadell, J., et al. (2013). “Chapter 6: carbon and other biogeochemical cycles,” in Climate Change 2013 The Physical Science Basis, eds T. Stocker, D. Qin, and G.-K. Platner (Cambridge: Cambridge U. Press).
Clark, D. B., Clark, D. A., and Oberbauer, S. F. (2010). Annual wood production in a tropical rain forest in NE Costa Rica linked to climatic variation but not to increasing CO2. Glob. Change Biol. 16, 747–759. doi: 10.1111/j.1365-2486.2009.02004.x
Cleveland, C. C., Townsend, A. R., Taylor, P., Alvarez-Clare, S., Bustamante, M. M. C., Chuyong, G., et al. (2011). Relationships among net primary productivity, nutrients and climate in tropical rain forest: a pan-tropical analysis. Ecol. Lett. 14, 939–947. doi: 10.1111/j.1461-0248.2011.01658.x
Comas, L. H., and Eissenstat, D. M. (2004). Linking fine root traits to maximum potential growth rate among 11 mature temperate tree species. Funct. Ecol. 18, 388–397. doi: 10.1111/j.0269-8463.2004.00835.x
Comas, L. H., Becker, S. R., Cruz, V. V., Byrne, P. F., and Dierig, D. A. (2013). Root traits contributing to plant productivity under drought. Front. Plant Sci. 4:16. doi: 10.3389/fpls.2013.00442
Cordeiro, A., Norby, R., Andersen, K., Valverde-Barrantes, O., Fuchslueger, L., Oblitas, E., et al. (2020). Fine-root dynamics vary with soil depth and precipitation in a low-nutrient tropical forest in the Central Amazonia. Plant Environ. Interact. 1, 3–16. doi: 10.1002/pei3.10010
Cormier, N., Twilley, R. R., Ewel, K. C., and Krauss, K. W. (2015). Fine root productivity varies along nitrogen and phosphorus gradients in high-rainfall mangrove forests of Micronesia. Hydrobiologia 750, 69–87. doi: 10.1007/s10750-015-2178-4
Cornejo, F. H., Varela, A., and Wright, S. J. (1994). Tropical forest litter decomposition under seasonal drought – nutrient release, fungi and bacteria. Oikos 70, 183–190. doi: 10.2307/3545629
Corrales, A., Henkel, T. W., and Smith, M. E. (2018). Ectomycorrhizal associations in the tropics - biogeography, diversity patterns and ecosystem roles. New Phytol. 220, 1076–1091. doi: 10.1111/nph.15151
Corrales, A., Turner, B. L., Tedersoo, L., Anslan, S., and Dalling, J. W. (2017). Nitrogen addition alters ectomycorrhizal fungal communities and soil enzyme activities in a tropical montane forest. Fungal Ecol. 27, 14–23. doi: 10.1016/j.funeco.2017.02.004
Costa, P. D., Abrahao, A., Viani, R. A. G., Brancalion, P. H. S., Lambers, H., Sawaya, A., et al. (2016). Cluster-root formation and carboxylate release in Euplassa cantareirae (Proteaceae) from a neotropical biodiversity hotspot. Plant Soil 403, 267–275. doi: 10.1007/s11104-015-2630-2
Couvreur, V., Vanderborght, J., and Javaux, M. (2012). A simple three-dimensional macroscopic root water uptake model based on the hydraulic architecture approach. Hydrol. Earth Syst. Sci. 16, 2957–2971. doi: 10.5194/hess-16-2957-2012
Crews, T. E., Kitayama, K., Fownes, J. H., Riley, R. H., Herbert, D. A., Muellerdombois, D., et al. (1995). Changes in soil-phosphorus fractions and ecosystem dynamics across a long chronosequence in Hawaii. Ecology 76, 1407–1424. doi: 10.2307/1938144
Cuneo, I. F., Knipfer, T., Brodersen, C. R., and McElrone, A. J. (2016). Mechanical failure of fine root cortical cells initiates plant hydraulic decline during drought. Plant Physiol. 172, 1669–1678. doi: 10.1104/pp.16.00923
Cusack, D. F., and Turner, B. L. (2020). Fine root and soil organic carbon depth distributions are inversely related across fertility and rainfall gradients in lowland tropical forests. Ecosystems 24, 1075–1092. doi: 10.1007/s10021-020-00569-6
Cusack, D. F., Lee, J., McCleery, T., and LeCroy, C. (2015). Exotic grasses and nitrate enrichment alter soil carbon cycling along an urban-rural tropical forest gradient. Glob. Change Biol. 21, 4481–4496.
Cusack, D. F., Silver, W. L., Torn, M. S., and McDowell, W. H. (2011). Effects of nitrogen additions on above- and belowground carbon dynamics in two tropical forests. Biogeochemistry 104, 203–225.
Cusack, D. F., Torn, M. S., McDowell, W. H., and Silver, W. L. (2010). The response of heterotrophic activity and carbon cycling to nitrogen additions and warming in two tropical soils. Glob. Change Biol. 16, 2555–2572.
Cusack, D., Ashdown, D., Dietterich, L., Neupane, A., Ciochina, M., and Turner, B. (2019). Seasonal changes in soil respiration linked to soil moisture and phosphorus availability along a tropical rainfall gradient. Biogeochemistry 145, 235–254.
Cusack, D., Karpman, J., Ashdown, D., Cao, Q., Ciochina, M., Halterman, S., et al. (2016). Global change effects on humid tropical forests: evidence for biogeochemical and biodiversity shifts at an ecosystem scale. Rev. Geophys. 54, 523–610. doi: 10.1002/2015RG000510
Cusack, D., Markesteijn, L., Condit, R., Lewis, O., and Turner, B. (2018). Soil carbon stocks in tropical forests of Panama regulated by base cation effects on fine roots. Biogeochemistry 137, 253–266. doi: 10.1007/s10533-017-0416-8
da-Costa, A. C. L., Galbraith, D., Almeida, S., Portela, B. T. T., Costa, M. D., Silva, J. D., et al. (2010). Effect of seven years of experimental drought on vegetation dynamics and biomass storage of an eastern Amazonian rainforest. New Phytol. 187, 579–591.
Davidson, E., Lefebvre, P. A., Brando, P. M., Ray, D. M., Trumbore, S. E., Solorzano, L. A., et al. (2011). Carbon inputs and water uptake in deep soils of an eastern Amazon Forest. For. Sci. 57, 51–58.
Davidson, R. L. (1969). Effect of root/leaf temperature differentials on root/shoot rations in some pasture grasses and clover. Ann. Bot. 33, 561–569.
Davies-Barnard, T., and Friedlingstein, P. (2020). The global distribution of biological nitrogen fixation in terrestrial natural ecosystems. Glob. Biogeochem. Cycles 34:e2019GB006387. doi: 10.1029/2019gb006387
De Kauwe, M. G., Medlyn, B. E., Zaehle, S., Walker, A. P., Dietze, M. C., Wang, Y.-P., et al. (2014). Where does the carbon go? A model-data intercomparison of vegetation carbon allocation and turnover processes at two temperate forest free-air CO2 enrichment sites. New Phytol. 203, 883–899. doi: 10.1111/nph.12847
Deng, Q., Zhang, D., Han, X., Chu, G., Zhang, Q., and Hui, D. (2018). Changing rainfall frequency rather than drought rapidly alters annual soil respiration in a tropical forest. Soil Biol. Biochem. 121, 8–15. doi: 10.1016/j.soilbio.2018.02.023
Doughty, C., Metcalfe, D., Girardin, C., Amézquita, F. F., Cabrera, D. G., Huasco, W. H., et al. (2015). Drought impact on forest carbon dynamics and fluxes in Amazonia. Nature 519, 78–82.
Doussan, C., Pierret, A., Garrigues, E., and Pages, L. (2006). Water uptake by plant roots: II – Modelling of water transfer in the soil root-system with explicit account of flow within the root system – Comparison with experiments. Plant Soil 283, 99–117. doi: 10.1007/s11104-004-7904-z
Duffy, P. B., Brando, P., Asner, G. P., and Field, C. B. (2015). Projections of future meteorological drought and wet periods in the Amazon. Proc. Natl. Acad. Sci. U.S.A. 112, 13172–13177. doi: 10.1073/pnas.1421010112
Duke, N. C., Ball, M. C., and Ellison, J. C. (1998). Factors influencing biodiversity and distributional gradients in mangroves. Glob. Ecol. Biogeogr. Lett. 7, 27–47.
Eissenstat, D. M. (1992). Costs and benefits of constructing roots of small diameter. J. Plant Nutr. 15, 763–782. doi: 10.1080/01904169209364361
Eissenstat, D. M., and Yanai, R. D. (1997). “The ecology of root lifespan,” in Advances in Ecological Research, eds M. Begon and A. H. Fitter (London: Academic Press Ltd-Elsevier Science Ltd).
Eller, C. B., Rowland, L., Mencuccini, M., Rosas, T., Williams, K., Harper, A., et al. (2020). Stomatal optimization based on xylem hydraulics (SOX) improves land surface model simulation of vegetation responses to climate. New Phytol. 226, 1622–1637. doi: 10.1111/nph.16419
Espeleta, J. F., and Clark, D. A. (2007). Multi-scale variation in fine-root biomass in a tropical rain forest: a seven-year study. Ecol. Monogra. 77:377–404. doi: 10.1890/06-1257.1
Esquivel-Muelbert, A., Baker, T. R., Dexter, K. G., Lewis, S. L., Brienen, R. J. W., Feldpausch, T. R., et al. (2019). Compositional response of Amazon forests to climate change. Glob. Change Biol. 25, 39–56. doi: 10.1111/gcb.14413
Esteban, E. J. L., Castilho, C. V., Melgaço, K. L., and Costa, F. R. C. (2021). The other side of droughts: wet extremes and topography as buffers of negative drought effects in an Amazonian forest. New Phytol. 229, 1995–2006.
Fan, Y., Miguez-Macho, G., Jobbagy, E. G., Jackson, R. B., and Otero-Casal, C. (2017). Hydrologic regulation of plant rooting depth. Proc. Natl. Acad. Sci. U.S.A. 114, 10572–10577. doi: 10.1073/pnas.1712381114
FAO (2012). “Global ecological zones for FAO forest reporting: 2010 update,” in Proceedings of the Forest Resources Assessment Working Paper (Rome: Food and Agriculture Organication of the United Nations).
Fatichi, S., Pappas, C., and Ivanov, V. Y. (2016). Modeling plant-water interactions: an ecohydrological overview from the cell to the global scale. Wiley Interdisciplinary Rev. Water 3, 327–368. doi: 10.1002/wat2.1125
Feddes, R. A., and Roats, P. A. C. (2004). “Parameterizing the soil-water-plant root system,” in Unsaturated-Zone Modeling: Progress, Challenges and Applications, eds R. A. Feddes, G. H. DeRooij, and J. C. VanDam (Dordrecht: Springer), 95–141.
Feddes, R. A., Hoff, H., Bruen, M., Dawson, T., de Rosnay, P., Dirmeyer, O., et al. (2001). Modeling root water uptake in hydrological and climate models. Bull. Am. Meteorol. Soc. 82, 2797–2809.
Feddes, R. A., Kowalik, P., Kolinskamalinka, K., and Zaradny, H. (1976). Simulation of field water uptake by plants using a soil water dependent root extraction function. J. Hydrol. 31, 13–26. doi: 10.1016/0022-1694(76)90017-2
Feldpausch, T. R., Phillips, O. L., Brienen, R. J. W., Gloor, E., Lloyd, J., Lopez-Gonzalez, G., et al. (2016). Amazon forest response to repeated droughts. Glob. Biogeochem. Cycles 30, 964–982. doi: 10.1002/2015gb005133
Feng, X., Porporato, A., and Rodriguez-Iturbe, I. (2013). Changes in rainfall seasonality in the tropics. Nat. Clim. Change 3, 811–815. doi: 10.1038/nclimate1907
Fetcher, N., Haines, B. L., Cordero, R. A., Lodge, D. J., Walker, L. R., Fernandez, D. S., et al. (1996). Responses of tropical plants to nutrients and light on a landslide in Puerto Rico. J. Ecol. 84, 331–341. doi: 10.2307/2261196
Field, C. B., Behrenfeld, M. J., Randerson, J. T., and Falkowski, P. (1998). Primary production of the biosphere: integrating terrestrial and oceanic components. Science 281, 237–240. doi: 10.1126/science.281.5374.237
Finer, L., Ohashi, M., Noguchi, K., and Hirano, Y. (2011). Fine root production and turnover in forest ecosystems in relation to stand and environmental characteristics. For. Ecol. Manag. 262, 2008–2023. doi: 10.1016/j.foreco.2011.08.042
Fisher, J. B., Badgley, G., and Blyth, E. (2012). Global nutrient limitation in terrestrial vegetation. Glob. Biogeochem. Cycles 26:GB3007.
Fisher, J. B., Malhi, Y., Bonal, D., Da Rocha, H. R., De Araujo, A. C., Gamo, M., et al. (2009). The land-atmosphere water flux in the tropics. Glob. Change Biol. 15, 2694–2714. doi: 10.1111/j.1365-2486.2008.01813.x
Fisher, J. B., Malhi, Y., Torres, I. C., Metcalfe, D. B., van de Weg, M., Meir, P., et al. (2013). Nutrient limitation in rainforests and cloud forests along a 3000 m elevation gradient in the Peruvian Andes. Oecologia 172, 889–902.
Fisher, J. B., Sitch, S., Malhi, Y., Fisher, R. A., Huntingford, C., and Tan, S.-Y. (2010). Carbon cost of plant nitrogen acquisition: a mechanistic, globally applicable model of plant nitrogen uptake, retranslocation, and fixation. Glob. Biogeochem. Cycles 24:GB1014. doi: 10.1029/2009gb003621
Fisher, J., Perakalapudi, N., Turner, B., Schimel, D., and Cusack, D. (2020). Competing effects of soil fertility and toxicity on tropical greening. Sci. Rep. 10:6725.
Fisher, R. A., and Koven, C. D. (2020). Perspectives on the future of land surface models and the challenges of representing complex terrestrial systems. J. Adv. Model. Earth Syst. 12:24. doi: 10.1029/2018ms001453
Fisher, R. A., Koven, C. D., Anderegg, W. R. L., Christoffersen, B. O., Dietze, M. C., Farrior, C. E., et al. (2018). Vegetation demographics in earth system models: a review of progress and priorities. Glob. Change Biol. 24, 35–54. doi: 10.1111/gcb.13910
Fisher, R. A., Wieder, W. R., Sanderson, B. M., Koven, C. D., Oleson, K. W., Xu, C., et al. (2019). Parametric controls on vegetation responses to biogeochemical forcing in the CLM5. J. Adv. Model. Earth Syst. 11, 2879–2895. doi: 10.1029/2019ms001609
Fleischer, K., Rammig, A., De Kauwe, M. G., Walker, A. P., Domingues, T. F., Fuchslueger, L., et al. (2019). Amazon forest response to CO2 fertilization dependent on plant phosphorus acquisition. Nat. Geosci. 12, 736–741. doi: 10.1038/s41561-019-0404-9
Freschet, G. T., Roumet, C., Comas, L. H., Weemstra, M., Bengough, A. G., Rewald, B., et al. (2021). Root traits as drivers of plant and ecosystem functioning: current understanding, pitfalls and future research needs. New Phytol. 232, 1123–1158. doi: 10.1111/nph.17072
Freschet, G. T., Valverde-Barrantes, O. J., Tucker, C. M., Craine, J. M., McCormack, M. L., Violle, C., et al. (2017). Climate, soil and plant functional types as drivers of global fine-root trait variation. J. Ecol. 105, 1182–1196. doi: 10.1111/1365-2745.12769
Fujii, K., Shibata, M., Kitajima, K., Ichie, T., Kitayama, K., and Turner, B. L. (2018). Plant-soil interactions maintain biodiversity and functions of tropical forest ecosystems. Ecol. Res. 33, 149–160.
Fukami, T., Nakajima, M., Fortunel, C., Fine, P. V. A., Baraloto, C., Russo, S. E., et al. (2017). Geographical variation in community divergence: insights from tropical forest monodominance by ectomycorrhizal trees. Am. Nat. 190, S105–S122. doi: 10.1086/692439
Gavito, M. E., Perez-Castillo, D., Gonzalez-Monterrubio, C. F., Vieyra-Hernandez, T., and Martinez-Trujillo, M. (2008). High compatibility between arbuscular mycorrhizal fungal communities and seedlings of different land use types in a tropical dry ecosystem. Mycorrhiza 19, 47–60. doi: 10.1007/s00572-008-0203-4
Gei, M. G., and Powers, J. S. (2013). Do legumes and non-legumes tree species affect soil properties in unmanaged forests and plantations in Costa Rican dry forests? Soil Biol. Biochem. 57, 264–272. doi: 10.1016/j.soilbio.2012.09.013
Gei, M., Rozendaal, D. M. A., Poorter, L., Bongers, F., Sprents, J. I., Garner, M. D., et al. (2018). Legume abundance along successional and rainfall gradients in Neotropical forests. Nat. Ecol. Evol. 2, 1104–1111. doi: 10.1038/s41559-018-0559-6
Germon, A., Laclau, J. P., Robin, A., and Jourdan, C. (2020). Tamm review: deep fine roots in forest ecosystems: why dig deeper? For. Ecol. Manag. 466:16. doi: 10.1016/j.foreco.2020.118135
Gill, R. A., and Jackson, R. B. (2000). Global patterns of root turnover for terrestrial ecosystems. New Phytol. 147, 13–31.
Goll, D. S., Joetzjer, E., Huang, M., and Ciais, P. (2018). Low phosphorus availability decreases susceptibility of tropical primary productivity to droughts. Geophys. Res. Lett. 45, 8231–8240. doi: 10.1029/2018gl077736
Goll, D. S., Vuichard, N., Maignan, F., Jornet-Puig, A., Sardans, J., Violette, A., et al. (2017). A representation of the phosphorus cycle for ORCHIDEE (revision 4520). Geosci. Model Dev. 10, 3745–3770. doi: 10.5194/gmd-10-3745-2017
Gower, S. T. (1987). Relations between mineral nutrient availability and fine root biomass in two Costa Rican tropical wet forests – a hypothesis. Biotropica 19, 171–175. doi: 10.2307/2388741
Grames, E. M., Stillman, A. N., Tingley, M. W., and Elphick, C. S. (2019). An automated approach to identifying search terms for systematic reviews using keyword co-occurrence networks. Methods Ecol. Evol. 10, 1645–1654.
Green, I. J., Dawson, L. A., Proctor, J., Duff, E. I., and Elston, D. A. (2005). Fine root dynamics in a tropical rain forest is influenced by rainfall. Plant Soil 276, 23–32. doi: 10.1007/s11104-004-0331-3
Guilbeault-Mayers, X., Turner, B. L., and Laliberte, E. (2020). Greater root phosphatase activity of tropical trees at low phosphorus despite strong variation among species. Ecology 101:9. doi: 10.1002/ecy.3090
Herrera, R., Merida, T., Stark, N., and Jordan, C. F. (1978). Direct phosphorus transfer from leaf litter to roots. Naturwissenschaften 65, 208–209. doi: 10.1007/bf00450594
Hietz, P., Turner, B. L., Wanek, W., Richter, A., Nock, C. A., and Wright, S. J. (2011). Long-term change in the nitrogen cycle of tropical forests. Science 334, 664–666. doi: 10.1126/science.1211979
Higgins, M. A., Ruokolainen, K., Tuomisto, H., Llerena, N., Cardenas, G., Phillips, O. L., et al. (2011). Geological control of floristic composition in Amazonian forests. J. Biogeogr. 38, 2136–2149.
Hodge, A. (2004). The plastic plant: root responses to heterogeneous supplies of nutrients. New Phytol. 162, 9–24. doi: 10.1111/j.1469-8137.2004.01015.x
Hofhansl, F., Chacón-Madrigal, E., Fuchslueger, L., Jenking, D., Morera-Beita, A., Plutzar, C., et al. (2020). Climatic and edaphic controls over tropical forest diversity and vegetation carbon storage. Sci. Rep. 10:5066.
Holdaway, R. J., Richardson, S. J., Dickie, I. A., Peltzer, D. A., and Coomes, D. A. (2011). Species- and community-level patterns in fine root traits along a 120,000-year soil chronosequence in temperate rain forest. J. Ecol. 99, 954–963. doi: 10.1111/j.1365-2745.2011.01821.x
Holdridge, L., Grenke, W., Hatheway, W., Liang, T., and Tosi, J. (1971). Forest Environments in Tropical Life Zones. New York, NY: Pergamon Press.
Hossain, M., and Nuruddin, A. (2016). Soil and mangrove: a review. J. Environ. Sci. Technil. 9, 198–207.
Houlton, B., Wang, Y., Vitousek, P., and Field, C. (2008). A unifying framework for dinitrogen fixation in the terrestrial biosphere. Nature 454, 327–331.
Huasco, W. H., Riutta, T., Girardin, C. A. J., Pacha, F. H., Vilca, B. L. P., Moore, S., et al. (2021). Fine root dynamics across pantropical rainforest ecosystems. Glob. Change Biol. 27, 3657–3680. doi: 10.1111/gcb.15677
Hunt, E. R., Running, S. W., and Federer, C. A. (1991). Extrapolating plant water flow resistances and capacitances to regional scales. Agric. For. Meteorol. 54, 169–195. doi: 10.1016/0168-1923(91)90005-b
Ibrahim, F., Adu-Bredu, S., Addo-Danso, S. D., Duah-Gyamfi, A., Manu, E. A., and Malhi, Y. (2020). Patterns and controls on fine-root dynamics along a rainfall gradient in Ghana. Trees Struct. Funct. 34, 917–929. doi: 10.1007/s00468-020-01970-3
Irino, K. O., Iba, Y., Ishizuka, S., Kenzo, T., Ripot, S., Kendawang, J. J., et al. (2004). Effects of controlled-release fertilizer on growth and ectomycorrhizal colonization of pot-grown seedlings of the dipterocarp Dryobalanops lanceolata in a tropical nursery. Soil Sci. Plant Nutr. 50, 747–753. doi: 10.1080/00380768.2004.10408531
Ivanov, V. Y., Hutyra, L. R., Wofsy, S. C., Munger, J. W., Saleska, S. R., De Oliveira, R. C., et al. (2012). Root niche separation can explain avoidance of seasonal drought stress and vulnerability of overstory trees to extended drought in a mature Amazonian forest. Water Resour. Res. 48:W12507. doi: 10.1029/2012wr011972
Iversen, C. M., McCormack, M. L., Powell, A. S., Blackwood, C. B., Freschet, G. T., Kattge, J., et al. (2017). A global fine-root ecology database to address below-ground challenges in plant ecology. New Phytol. 215, 15–26. doi: 10.1111/nph.14486
Iversen, C., McCormack, M., Baer, J., Powell, A., Chen, W., Collins, C., et al. (2021). in Fine-Root Ecology Database (FRED): A Global Collection of Root Trait Data with Coincident Site, Vegetation, Edaphic, and Climatic Data, Version 3, ed. T. S. Oak Ridge (Oak Ridge, TEN: U.S. Department of Energy).
Jackson, P. C., Cavelier, J., Goldstein, G., Meinzer, F. C., and Holbrook, N. M. (1995). Partitioning of water resources among plants of a lowland tropical forest. Oecologia 101, 197–203. doi: 10.1007/bf00317284
Jackson, R. B., Canadell, J., Ehleringer, J. R., Mooney, H. A., Sala, O. E., and Schulze, E. D. (1996). A global analysis of root distributions for terrestrial biomes. Oecologia 108, 389–411. doi: 10.1007/bf00333714
James, S. A., Meinzer, F. C., Goldstein, G., Woodruff, D., Jones, T., Restom, T., et al. (2003). Axial and radial water transport and internal water storage in tropical forest canopy trees. Oecologia 134, 37–45. doi: 10.1007/s00442-002-1080-8
Janssen, T., Fleischer, K., Luyssaert, S., Naudts, K., and Dolman, H. (2020). Drought resistance increases from the individual to the ecosystem level in highly diverse Neotropical rainforest: a meta-analysis of leaf, tree and ecosystem responses to drought. Biogeosciences 17, 2621–2645. doi: 10.5194/bg-17-2621-2020
Javaux, M., Couvreur, V., Vanderborght, J., and Vereecken, H. (2013). Root water uptake: from three-dimensional biophysical processes to macroscopic modeling approaches. Vadose Zone J. 12, 1–16. doi: 10.2136/vzj2013.02.0042
Jimenez, E. M., Moreno, F. H., Penuela, M. C., Patino, S., and Lloyd, J. (2009). Fine root dynamics for forests on contrasting soils in the Colombian Amazon. Biogeosciences 6, 2809–2827. doi: 10.5194/bg-6-2809-2009
Jobbagy, E. G., and Jackson, R. B. (2000). The vertical distribution of soil organic carbon and its relation to climate and vegetation. Ecol. Appl. 10, 423–436.
Jourdan, C., Silva, E. V., Goncalves, J. L. M., Ranger, J., Moreira, R. M., and Laclau, J. P. (2008). Fine root production and turnover in Brazilian Eucalyptus plantations under contrasting nitrogen fertilization regimes. For. Ecol. Manag. 256, 396–404. doi: 10.1016/j.foreco.2008.04.034
Kennedy, D., Swenson, S., Oleson, K. W., Lawrence, D. M., Fisher, R., Lola da Costa, A. C., et al. (2019). Implementing plant hydraulics in the community land model, version 5. J. Adv. Model. Earth Syst. 11, 485–513. doi: 10.1029/2018ms001500
Kharin, V. V., Zwiers, F. W., Zhang, X., and Hegerl, G. C. (2007). Changes in temperature and precipitation extremes in the IPCC ensemble of global coupled model simulations. J. Clim. 20, 1419–1444.
Kochsiek, A., Tan, S., and Russo, S. E. (2013). Fine root dynamics in relation to nutrients in oligotrophic Bornean rain forest soils. Plant Ecol. 214, 869–882. doi: 10.1007/s11258-013-0215-9
Kong, D. L., Wang, J. J., Valverde-Barrantes, O. J., and Kardol, P. (2021). A framework to assess the carbon supply-consumption balance in plant roots. New Phytol. 229, 659–664. doi: 10.1111/nph.16807
Kong, D., Ma, C., Zhang, Q., Li, L., Chen, X., Zeng, H., et al. (2014). Leading dimensions in absorptive root trait variation across 96 subtropical forest species. New Phytol. 203, 863–872. doi: 10.1111/nph.12842
Koschorreck, M., and Darwich, A. (2003). Nitrogen dynamics in seasonally flooded soils in the Amazon floodplain. Wetlands Ecol. Manag. 11, 317–330. doi: 10.1023/b:Wetl.0000005536.39074.72
Kunert, N., Schwendenmann, L., and Holscher, D. (2010). Seasonal dynamics of tree sap flux and water use in nine species in Panamanian forest plantations. Agric. For. Meteorol. 150, 411–419. doi: 10.1016/j.agrformet.2010.01.006
Laliberte, E., Lambers, H., Burgess, T. I., and Joseph Wright, S. (2015). Phosphorus limitation, soil-borne pathogens and the coexistence of plant species in hyperdiverse forests and shrublands. New Phytol. 206, 507–521. doi: 10.1111/nph.13203
Lambers, H., Raven, J. A., Shaver, G. R., and Smith, S. E. (2008). Plant nutrient-acquisition strategies change with soil age. Trends Ecol. Evol. 23, 95–103. doi: 10.1016/j.tree.2007.10.008
Lawrence, D. M., Fisher, R. A., Koven, C. D., Oleson, K. W., Swenson, S. C., Bonan, G., et al. (2019). The community land model version 5: description of new features, benchmarking, and impact of forcing uncertainty. J. Adv. Model. Earth Syst. 11, 4245–4287. doi: 10.1029/2018ms001583
Lee, J., Oliveira, R., Dawson, T., and Fung, I. (2005). Root functioning modifies seasonal climate. Proc. Natl. Acad. Sci. U.S.A. 102, 17576–17581.
Liu, T., Sheng, M., Wang, C. Y., Chen, H., Li, Z., and Tang, M. (2015). Impact of arbuscular mycorrhizal fungi on the growth, water status, and photosynthesis of hybrid poplar under drought stress and recovery. Photosynthetica 53, 250–258. doi: 10.1007/s11099-015-0100-y
Liu, X. J., Duan, L., Mo, J. M., Du, E. Z., Shen, J. L., Lu, X. K., et al. (2011). Nitrogen deposition and its ecological impact in China: an overview. Environ. Pollut. 159, 2251–2264. doi: 10.1016/j.envpol.2010.08.002
Liu, X., Burslem, D. F. R. P., Taylor, J. D., Taylor, A. F. S., Khoo, E., Majalap-Lee, N., et al. (2018). Partitioning of soil phosphorus among arbuscular and ectomycorrhizal trees in tropical and subtropical forests. Ecol. Lett. 21, 713–723. doi: 10.1111/ele.12939
Lu, M. Z., and Hedin, L. O. (2019). Global plant-symbiont organization and emergence of biogeochemical cycles resolved by evolution-based trait modelling. Nat. Ecol. Evol. 3, 239–250. doi: 10.1038/s41559-018-0759-0
Lucash, M. S., Eissenstat, D. M., Joslin, J. D., and Mcfarlane, K. J. (2007). Estimating nutrient uptake by mature tree roots under field conditions: challenges and opportunities. Trees Struc. Funct. 21, 593–603. doi: 10.1007/s00468-007-0160-0
Lugli, L. F., Andersen, K. M., Aragao, L., Cordeiro, A. L., Cunha, H. K. V., Fuchslueger, L., et al. (2020). Multiple phosphorus acquisition strategies adopted by fine roots in low-fertility soils in Central Amazonia. Plant Soil 450, 49–63. doi: 10.1007/s11104-019-03963-9
Lugli, L. F., Rosa, J. S., Andersen, K. M., Di Ponzio, R., Almeida, R. V., Pires, M., et al. (2021). Rapid responses of root traits and productivity to phosphorus and cation additions in a tropical lowland forest in Amazonia. New Phytol. 230, 116–128. doi: 10.1111/nph.17154
Luizao, R. C. C., Luizao, F. J., and Proctor, J. (2007). Fine root growth and nutrient release in decomposing leaf litter in three contrasting vegetation types in central Amazonia. Plant Ecol. 192, 225–236. doi: 10.1007/s11258-007-9307-8
Ma, Z. Q., Guo, D. L., Xu, X. L., Lu, M. Z., Bardgett, R. D., Eissenstat, D. M., et al. (2018). Evolutionary history resolves global organization of root functional traits. Nature 555, 94–97. doi: 10.1038/nature25783
Maeght, J.-L., Gonkhamdee, S., Clement, C., Ayutthaya, S. I. N., Stokes, A., and Pierret, A. (2015). Seasonal patterns of fine root production and turnover in a mature rubber tree (Hevea brasiliensis Mull. Arg.) stand- differentiation with soil depth and implications for soil carbon stocks. Front. Plant Sci. 6:1022. doi: 10.3389/fpls.2015.01022
Magrin, G. O., Marengo, J. A., Boulanger, J.-P., Buckeridge, M. S., Castellanos, E., Poveda, G., et al. (2014). “Central and south america,” in Climate Change 2014: Impacts, Adaptation, and Vulnerability. Part B: Regional Aspects. Contribution of Working Group II to the Fifth Assessment Report on the Intergovernmental Panel on Climate Change, eds V. R. Barros, C. B. Field, D. J. Dokken, M. D. Mastrandrea, K. J. Mach, T. E. Bilir, et al. (New York, NY: Cambridge University Press), 1499–1566.
Maherali, H. (2014). Is there an association between root architecture and mycorrhizal growth response? New Phytol. 204, 192–200. doi: 10.1111/nph.12927
Manoli, G., Bonetti, S., Domec, J.-C., Putti, M., Katul, G., and Marani, M. (2014). Tree root systems competing for soil moisture in a 3D soil-plant model. Adv. Water Resour. 66, 32–42. doi: 10.1016/j.advwatres.2014.01.006
Markesteijn, L., and Poorter, L. (2009). Seedling root morphology and biomass allocation of 62 tropical tree species in relation to drought- and shade-tolerance. J. Ecol. 97, 311–325. doi: 10.1111/j.1365-2745.2008.01466.x
Markewitz, D., Devine, S., Davidson, E. A., Brando, P., and Nepstad, D. C. (2010). Soil moisture depletion under simulated drought in the Amazon: impacts on deep root uptake. New Phytol. 187, 592–607. doi: 10.1111/j.1469-8137.2010.03391.x
Marklein, A. R., and Houlton, B. Z. (2012). Nitrogen inputs accelerate phosphorus cycling rates across a wide variety of terrestrial ecosystems. New Phytol. 193, 696–704. doi: 10.1111/j.1469-8137.2011.03967.x
Matson, P. A., McDowell, W. H., Townsend, A. R., and Vitousek, P. M. (1999). The globalization of N deposition: ecosystem consequences in tropical environments. Biogeochemistry 46, 67–83.
Maycock, C. R., and Congdon, R. A. (2000). Fine root biomass and soil N and P in north Queensland rain forests. Biotropica 32, 185–190. doi: 10.1111/j.1744-7429.2000.tb00460.x
McCormack, M. L., and Iversen, C. M. (2019). Physical and functional constraints on viable belowground acquisition strategies. Front. Plant Sci. 10:1215. doi: 10.3389/fpls.2019.01215
McCormack, M. L., Dickie, I. A., Eissenstat, D. M., Fahey, T. J., Fernandez, C. W., Guo, D. L., et al. (2015). Redefining fine roots improves understanding of below-ground contributions to terrestrial biosphere processes. New Phytol. 207, 505–518. doi: 10.1111/nph.13363
McCulloch, L. A., and Porder, S. (2021). Light fuels while nitrogen suppresses symbiotic nitrogen fixation hotspots in Neotropical canopy gap seedlings. New Phytol. 231, 1734–1745. doi: 10.1111/nph.17519
McCulloch, L. A., Piotto, D., and Porder, S. (2021). Drought and soil nutrients effects on symbiotic nitrogen fixation in seedlings from eight Neotropical legume species. Biotropica 53, 703–713. doi: 10.1111/btp.12911
McGonigle, T. P., Miller, M. H., Evans, D. G., Fairchild, G. L., and Swan, J. A. (1990). A new method which gives an objective measure of colonization of roots by vesicular arbuscular mycorrhizal fungi. New Phytol. 115, 495–501. doi: 10.1111/j.1469-8137.1990.tb00476.x
McGuire, K. L., Fierer, N., Bateman, C., Treseder, K. K., and Turner, B. L. (2012). Fungal community composition in neotropical rain forests: the influence of tree diversity and precipitation. Microb. Ecol. 63, 804–812. doi: 10.1007/s00248-011-9973-x
McKee, K. L., Cahoon, D. R., and Feller, I. C. (2007). Caribbean mangroves adjust to rising sea level through biotic controls on change in soil elevation. Glob. Ecol. Biogeogr. 16, 545–556. doi: 10.1111/j.1466-8238.2007.00317.x
McMurtrie, R. E., and Nasholm, T. (2018). Quantifying the contribution of mass flow to nitrogen acquisition by an individual plant root. New Phytol. 218, 119–130. doi: 10.1111/nph.14927
Medina, E., and Cuevas, E. (1989). “Patterns of nutrient accumulation and release in amazonian forests of the Upper Rio Negro basin,” in Mineral Nutrients In Tropical Forest And Savanna Ecosystems, ed. J. Proctor (Oxford: Blackwell Scientific Publications), 217–240.
Meinzer, F. C., Andrade, J. L., Goldstein, G., Holbrook, N. M., Cavelier, J., and Wright, S. J. (1999). Partitioning of soil water among canopy trees in a seasonally dry tropical forest. Oecologia 121, 293–301. doi: 10.1007/s004420050931
Menge, D. N. L., Batterman, S. A., Hedin, L. O., Liao, W. Y., Pacala, S. W., and Taylor, B. N. (2017). Why are nitrogen-fixing trees rare at higher compared to lower latitudes? Ecology 98, 3127–3140. doi: 10.1002/ecy.2034
Menge, D. N. L., Levin, S. A., and Hedin, L. O. (2009). Facultative versus obligate nitrogen fixation strategies and their ecosystem consequences. Am. Nat. 174, 465–477. doi: 10.1086/605377
Metcalfe, D. B., Meir, P., Aragao, L., da Costa, A. C. L., Braga, A. P., Goncalves, P. H. L., et al. (2008). The effects of water availability on root growth and morphology in an Amazon rainforest. Plant Soil 311, 189–199. doi: 10.1007/s11104-008-9670-9
Meyerholt, J., and Zaehle, S. (2018). Controls of terrestrial ecosystem nitrogen loss on simulated productivity responses to elevated CO2. Biogeosciences 15, 5677–5698. doi: 10.5194/bg-15-5677-2018
Meyerholt, J., Sickel, K., and Zaehle, S. (2020). Ensemble projections elucidate effects of uncertainty in terrestrial nitrogen limitation on future carbon uptake. Glob. Change Biol. 26, 3978–3996. doi: 10.1111/gcb.15114
Moser, G., Schuldt, B., Hertel, D., Horna, V., Coners, H., Barus, H., et al. (2014). Replicated throughfall exclusion experiment in an Indonesian perhumid rainforest: wood production, litter fall and fine root growth under simulated drought. Glob. Change Biol. 20, 1481–1497. doi: 10.1111/gcb.12424
Murphy, P. G., and Lugo, A. E. (1986). Ecology of tropical dry forest. Annu. Rev. Ecol. Syst. 17, 67–88. doi: 10.1146/annurev.es.17.110186.000435
Nadelhoffer, K. J., Aber, J. D., and Melillo, J. M. (1985). Fine roots, net primary production and soil nitrogen availability – a new hypothesis. Ecology 66, 1377–1390. doi: 10.2307/1939190
Nasto, M. K., Alvarez-Clare, S., Lekberg, Y., Sullivan, B. W., Townsend, A. R., and Cleveland, C. C. (2014). Interactions among nitrogen fixation and soil phosphorus acquisition strategies in lowland tropical rain forests. Ecol. Lett. 17, 1282–1289. doi: 10.1111/ele.12335
Nasto, M. K., Osborne, B. B., Lekberg, Y., Asner, G. P., Balzotti, C. S., Porder, S., et al. (2017). Nutrient acquisition, soil phosphorus partitioning and competition among trees in a lowland tropical rain forest. New Phytol. 214, 1506–1517. doi: 10.1111/nph.14494
Nasto, M. K., Winter, K., Turner, B. L., and Cleveland, C. C. (2019). Nutrient acquisition strategies augment growth in tropical N-2-fixing trees in nutrient-poor soil and under elevated CO2. Ecology 100:12. doi: 10.1002/ecy.2646
Nepstad, D. C., Decarvalho, C. R., Davidson, E. A., Jipp, P. H., Lefebvre, P. A., Negreiros, G. H., et al. (1994). The role of deep roots in the hydrological and carbon cycles of amazonian forests and pastures. Nature 372, 666–669.
Nepstad, D. C., Moutinho, P., Dias, M. B., Davidson, E., Cardinot, G., Markewitz, D., et al. (2002). The effects of partial throughfall exclusion on canopy processes, aboveground production, and biogeochemistry of an Amazon forest. J. Geophys. Res. Atmos. 107, LBA53–1–LBA53–18.
Nepstad, D. C., Tohver, I. M., Ray, D., Moutinho, P., and Cardinot, G. (2007). Mortality of large trees and lianas following experimental drought in an amazon forest. Ecology 88, 2259–2269.
Oliveira, R. S., Dawson, T. E., Burgess, S. S. O., and Nepstad, D. C. (2005). Hydraulic redistribution in three Amazonian trees. Oecologia 145, 354–363. doi: 10.1007/s00442-005-0108-2
Oliveira, R. S., Eller, C. B., Barros, F. D., Hirota, M., Brum, M., and Bittencourt, P. (2021). Linking plant hydraulics and the fast-slow continuum to understand resilience to drought in tropical ecosystems. New Phytol. 230, 904–923. doi: 10.1111/nph.17266
Oliveira, R. S., Galvao, H. C., de Campos, M. C. R., Eller, C. B., Pearse, S. J., and Lambers, H. (2015). Mineral nutrition of campos rupestres plant species on contrasting nutrient-impoverished soil types. New Phytol. 205, 1183–1194. doi: 10.1111/nph.13175
Ostertag, R. (2001). Effects of nitrogen and phosphorus availability on fine-root dynamics in Hawaiian montane forests. Ecology 82, 485–499.
Phillips, R. P., Erlitz, Y., Bier, R., and Bernhardt, E. S. (2008). New approach for capturing soluble root exudates in forest soils. Funct. Ecol. 22, 990–999. doi: 10.1111/j.1365-2435.2008.01495.x
Powell, J. R., and Rillig, M. C. (2018). Biodiversity of arbuscular mycorrhizal fungi and ecosystem function. New Phytol. 220, 1059–1075. doi: 10.1111/nph.15119
Powers, J. S., and Perez-Aviles, D. (2013). Edaphic factors are a more important control on surface fine roots than stand age in secondary tropical dry forests. Biotropica 45, 1–9. doi: 10.1111/j.1744-7429.2012.00881.x
Powers, J. S., Treseder, K. K., and Lerdau, M. T. (2005). Fine roots, arbuscular mycorrhizal hyphae and soil nutrients in four neotropical rain forests: patterns across large geographic distances. New Phytol. 165, 913–921. doi: 10.1111/j.1469-8137.2004.01279.x
Powers, J. S., Vargas, G. G., Brodribb, T. J., Schwartz, N. B., Perez-Aviles, D., Smith-Martin, C. M., et al. (2020). A catastrophic tropical drought kills hydraulically vulnerable tree species. Glob. Change Biol. 26, 3122–3133. doi: 10.1111/gcb.15037
Quesada, C. A., Paz, C., Mendoza, E. O., Phillips, O. L., Saiz, G., and Lloyd, J. (2020). Variations in soil chemical and physical properties explain basin-wide Amazon forest soil carbon concentrations. Soil 6, 53–88. doi: 10.5194/soil-6-53-2020
Quesada, C. A., Phillips, O. L., Schwarz, M., Czimczik, C. I., Baker, T. R., Patino, S., et al. (2012). Basin-wide variations in Amazon forest structure and function are mediated by both soils and climate. Biogeosciences 9, 2203–2246. doi: 10.5194/bg-9-2203-2012
Quinto, H., Caicedo, H., Perez, M. T., and Moreno, F. (2016). Fine root dynamics and its relationship with soil fertility in tropical rainforests of Choco. Rev. Biol. Trop. 64, 1709–1719.
Reich, P. B. (2014). The world-wide ‘fast-slow’ plant economics spectrum: a traits manifesto. J. Ecol. 102, 275–301. doi: 10.1111/1365-2745.12211
Reis, C. R. G., Nardoto, G. B., and Oliveira, R. S. (2017). Global overview on nitrogen dynamics in mangroves and consequences of increasing nitrogen availability for these systems. Plant Soil 410, 1–19. doi: 10.1007/s11104-016-3123-7
Roa-Fuentes, L., Campo, J., and Parra-Tabla, V. (2021). Plant biomass allocation across a precipitation gradient: an approach to seasonally dry tropical forest at yucatán, Mexico. Ecosystems 15, 1234–1244.
Rodtassana, C., and Tanner, E. V. J. (2018). Litter removal in a tropical rain forest reduces fine root biomass and production but litter addition has few effects. Ecology 99, 735–742. doi: 10.1002/ecy.2143
Ropelewski, C. F., and Halpert, M. S. (1987). Global and regional scale precipitation patterns associated with the El Nino Southern Oscillation. Monthly Weather Rev. 115, 1606–1626.
Russo, S. E., Kochsiek, A., Olney, J., Thompson, L., Miller, A. E., and Tan, S. (2013). Nitrogen uptake strategies of edaphically specialized Bornean tree species. Plant Ecol. 214, 1405–1416. doi: 10.1007/s11258-013-0260-4
Russo, S. E., Zhang, L., and Tan, S. (2012). Covariation between understorey light environments and soil resources in Bornean mixed dipterocarp rain forest. J. Trop. Ecol. 28, 33–44. doi: 10.1017/s0266467411000538
Sakschewski, B., von Bloh, W., Drueke, M., Soerensson, A. A., Ruscica, R., Langerwisch, F., et al. (2021). Variable tree rooting strategies are key for modelling the distribution, productivity and evapotranspiration of tropical evergreen forests. Biogeosciences 18, 4091–4116. doi: 10.5194/bg-18-4091-2021
Sanders, C. J., Eyre, B. D., Santos, I. R., Machado, W., Luiz-Silva, W., Smoak, J. M., et al. (2014). Elevated rates of organic carbon, nitrogen, and phosphorus accumulation in a highly impacted mangrove wetland. Geophys. Res. Lett. 41, 2475–2480. doi: 10.1002/2014gl059789
Sayer, E., and Banin, L. (2016). “Tree nutrient status and nutrient cycling in tropical forest—lessons from fertilization experiments,” in Tropical Tree Physiology: Adaptations and Responses in a 23 Changing Environment, eds G. Goldstein and S. Santiago (Cambridge: Springer International Publishing), 275–297.
Schlesinger, W., and Bernhardt, E. (2020). Biogeochemistry: An Analysis of Global Change. Cambridge, MA: Academic Press.
Schuldt, B., Leuschner, C., Brock, N., and Horna, V. (2013). Changes in wood density, wood anatomy and hydraulic properties of the xylem along the root-to-shoot flow path in tropical rainforest trees. Tree Physiol. 33, 161–174. doi: 10.1093/treephys/tps122
Schwartz, N. B., Lintner, B. R., Feng, X., and Powers, J. S. (2020). Beyond MAP: A guide to dimensions of rainfall variability for tropical ecology. Biotropica 52, 1319–1332. doi: 10.1111/btp.12830
Schwede, D. B., Simpson, D., Tan, J. N., Fu, J. S., Dentener, F., Du, E. Z., et al. (2018). Spatial variation of modelled total, dry and wet nitrogen deposition to forests at global scale. Environ. Pollut. 243, 1287–1301. doi: 10.1016/j.envpol.2018.09.084
Serraj, R., Sinclair, T. R., and Purcell, L. C. (1999). Symbiotic N-2 fixation response to drought. J. Exp. Bot 50, 143–155. doi: 10.1093/jexbot/50.331.143
Sheldrake, M., Rosenstock, N. P., Mangan, S., Revillini, D., Sayer, E. J., Olsson, P. A., et al. (2018). Responses of arbuscular mycorrhizal fungi to long-term inorganic and organic nutrient addition in a lowland tropical forest. ISME J. 12, 2433–2445. doi: 10.1038/s41396-018-0189-7
Shen, Y., Gilbert, G. S., Li, W. B., Fang, M., Lu, H. P., and Yu, S. X. (2019). Linking aboveground traits to root traits and local environment: implications of the plant economics spectrum. Front. Plant Sci. 10:12. doi: 10.3389/fpls.2019.01412
Shi, M., Fisher, J., Brozostek, E., and Phillips, R. (2016). Carbon cost of plant nitrogen acquisition: global carbon cycle impact from an improved plant nitrogen cycle in the Community Land Model. Glob. Change Biol. 22, 1299–1314.
Shimizu, M., Ishida, A., and Hogetsu, T. (2005). Root hydraulic conductivity and whole-plant water balance in tropical saplings following a shade-to-sun transfer. Oecologia 143, 189–197. doi: 10.1007/s00442-004-1797-7
Shipley, B., and Meziane, D. (2002). The balanced-growth hypothesis and the allometry of leaf and root biomass allocation. Funct. Ecol. 16, 326–331. doi: 10.1046/j.1365-2435.2002.00626.x
Silvertown, J., Araya, Y., and Gowing, D. (2015). Hydrological niches in terrestrial plant communities: a review. J. Ecol. 103, 93–108. doi: 10.1111/1365-2745.12332
Simard, M., Fatoyinbo, L., Smetanka, C., Rivera-Monroy, V. H., Castañeda-Moya, E., Thomas, N., et al. (2019). Mangrove canopy height globally related to precipitation, temperature and cyclone frequency. Nat. Geosci. 12, 40–45.
Singha, D., Brearley, F. Q., and Tripathi, S. K. (2020). Fine root and soil nitrogen dynamics during stand development following shifting agriculture in Northeast India. Forests 11:12. doi: 10.3390/f11121236
Siqueira, J. O., Carneiro, M. A. C., Curi, N., Rosado, S. C. S., and Davide, A. C. (1998). Mycorrhizal colonization and mycotrophic growth of native woody species as related to successional groups in Southeastern Brazil. For. Ecol. Manag. 107, 241–252. doi: 10.1016/s0378-1127(97)00336-8
Skene, K. R. (2001). Cluster roots: model experimental tools for key biological problems. J. Exp. Bot. 52, 479–485.
Smith, B., Warlind, D., Arneth, A., Hickler, T., Leadley, P., Siltberg, J., et al. (2014). Implications of incorporating N cycling and N limitations on primary production in an individual-based dynamic vegetation model. Biogeosciences 11, 2027–2054. doi: 10.5194/bg-11-2027-2014
Smith-Martin, C. M., Bastos, C. L., Lopez, O. R., Powers, J. S., and Schnitzer, S. A. (2019). Effects of dry-season irrigation on leaf physiology and biomass allocation in tropical lianas and trees. Ecology 100:e02827. doi: 10.1002/ecy.2827
Smithwick, E. A. H., Lucash, M. S., McCormack, M. L., and Sivandran, G. (2014). Improving the representation of roots in terrestrial models. Ecol. Model. 291, 193–204. doi: 10.1016/j.ecolmodel.2014.07.023
Soong, J. L., Janssens, I., Grau, O., Margalef, O., Stahl, C., Langenhove, L. V., et al. (2020). Soil properties explain tree growth and mortality, but not biomass, across phosphorus-depleted tropical forests. Sci. Rep. 10:2302.
Soper, F. M., Nasto, M. K., Osborne, B. B., and Cleveland, C. C. (2019). Nitrogen fixation and foliar nitrogen do not predict phosphorus acquisition strategies in tropical trees. J. Ecol. 107, 118–126. doi: 10.1111/1365-2745.13044
Stahl, C., Herault, B., Rossi, V., Burban, B., Brechet, C., and Bonal, D. (2013). Depth of soil water uptake by tropical rainforest trees during dry periods: does tree dimension matter? Oecologia 173, 1191–1201. doi: 10.1007/s00442-013-2724-6
Stark, N., and Jordan, C. F. (1978). Nutrient retention in the root mat of an Amazonian rain forest. Ecology 59, 434–437.
Steidinger, B. S., Crowther, T. W., Liang, J., Van Nuland, M. E., Werner, G. D. A., Reich, P. B., et al. (2019). Climatic controls of decomposition drive the global biogeography of forest-tree symbioses. Nature 569, 404–408. doi: 10.1038/s41586-019-1128-0
Steidinger, B. S., Turner, B. L., Corrales, A., and Dalling, J. W. (2015). Variability in potential to exploit different soil organic phosphorus compounds among tropical montane tree species. Funct. Ecol. 29, 121–130. doi: 10.1111/1365-2435.12325
Sulis, M., Couvreur, V., Keune, J., Cai, G. C., Trebs, I., Junk, J., et al. (2019). Incorporating a root water uptake model based on the hydraulic architecture approach in terrestrial systems simulations. Agric. For. Meteorol. 269, 28–45. doi: 10.1016/j.agrformet.2019.01.034
Sullivan, B. W., Smith, W. K., Townsend, A. R., Nasto, M. K., Reed, S. C., Chazdon, R. L., et al. (2014). Spatially robust estimates of biological nitrogen (N) fixation imply substantial human alteration of the tropical N cycle. Proc. Natl. Acad. Sci. U.S.A. 111, 8101–8106. doi: 10.1073/pnas.1320646111
Sulman, B. N., Shevliakova, E., Brzostek, E. R., Kivlin, S. N., Malyshev, S., Menge, D. N. L., et al. (2019). Diverse mycorrhizal associations enhance terrestrial C storage in a global model. Glob. Biogeochem. Cycles 33, 501–523. doi: 10.1029/2018gb005973
Sun, L., Ataka, M., Han, M., Han, Y., Gan, D., Xu, T., et al. (2020). Root exudation as a major competitive fine-root functional trait of 18 coexisting species in a subtropical forest. New Phytol. 229, 259–271. doi: 10.1111/nph.16865
Tang, J. Y., and Riley, W. J. (2021). On the modeling paradigm of plant root nutrient acquisition. Plant Soil 459, 441–451. doi: 10.1007/s11104-020-04798-5
Taylor, B. N., and Menge, D. N. L. (2018). Light regulates tropical symbiotic nitrogen fixation more strongly than soil nitrogen. Nat. Plants 4, 655–661. doi: 10.1038/s41477-018-0231-9
Taylor, B. N., Chazdon, R. L., and Menge, D. N. L. (2019). Successional dynamics of nitrogen fixation and forest growth in regenerating Costa Rican rainforests. Ecology 100:e02637. doi: 10.1002/ecy.2637
Tedersoo, L., Bahram, M., Toots, M., Diedhiou, A. G., Henkel, T. W., Kjoller, R., et al. (2012). Towards global patterns in the diversity and community structure of ectomycorrhizal fungi. Mol. Ecol. 21, 4160–4170. doi: 10.1111/j.1365-294X.2012.05602.x
Tedersoo, L., May, T. W., and Smith, M. E. (2010). Ectomycorrhizal lifestyle in fungi: global diversity, distribution, and evolution of phylogenetic lineages. Mycorrhiza 20, 217–263. doi: 10.1007/s00572-009-0274-x
Templer, P. H., Silver, W. L., Pett-Ridge, J., DeAngelis, K. M., and Firestone, M. K. (2008). Plant and microbial controls on nitrogen retention and loss in a humid tropical forest. Ecology 89, 3030–3040. doi: 10.1890/07-1631.1
Teodoro, G. S., Lambers, H., Nascimento, D. L., Costa, P. D., Flores-Borges, D. N. A., Abrahao, A., et al. (2019). Specialized roots of Velloziaceae weather quartzite rock while mobilizing phosphorus using carboxylates. Funct. Ecol. 33, 762–773. doi: 10.1111/1365-2435.13324
Theodorou, C. (1978). Soil moisture and mycorrhizal association of Pinus radiata (D Don). Soil Biol. Biochem. 10, 33–37. doi: 10.1016/0038-0717(78)90007-x
Thornley, J. H. (1972). Balanced quantitative model for root-shoot ratios in vegetative plants. Ann. Bot. 36, 431–441. doi: 10.1093/oxfordjournals.aob.a084602
Thornton, P. E., Lamarque, J. F., Rosenbloom, N. A., and Mahowald, N. M. (2007). Influence of carbon-nitrogen cycle coupling on land model response to CO2 fertilization and climate variability. Glob. Biogeochem. Cycles 21:15. doi: 10.1029/2006gb002868
Todd-Brown, K. E. O., Randerson, J. T., Hopkins, F., Arora, V., Hajima, T., Jones, C., et al. (2014). Changes in soil organic carbon storage predicted by Earth system models during the 21st century. Biogeosciences 11, 2341–2356. doi: 10.5194/bg-11-2341-2014
Treseder, K. K. (2004). A meta-analysis of mycorrhizal responses to nitrogen, phosphorus, and atmospheric CO2 in field studies. New Phytol. 164, 347–355. doi: 10.1111/j.1469-8137.2004.01159.x
Treseder, K. K., and Vitousek, P. M. (2001). Effects of soil nutrient availability on investment in acquisition of N and P in Hawaiian rain forests. Ecology 82, 946–954. doi: 10.1890/0012-96582001082
Trierweiler, A. M., Winter, K., and Hedin, L. O. (2018). Rising CO2 accelerates phosphorus and molybdenum limitation of N-2-fixation in young tropical trees. Plant Soil 429, 363–373. doi: 10.1007/s11104-018-3685-7
Trugman, A. T., Anderegg, L. D. L., Sperry, J. S., Wang, Y., Venturas, M., and Anderegg, W. R. L. (2019). Leveraging plant hydraulics to yield predictive and dynamic plant leaf allocation in vegetation models with climate change. Glob. Change Biol. 25, 4008–4021. doi: 10.1111/gcb.14814
Turner, B. L., and Engelbrecht, B. M. J. (2011). Soil organic phosphorus in lowland tropical rain forests. Biogeochemistry 103, 297–315. doi: 10.1007/s10533-010-9466-x
Turner, I. M., Brown, N. D., and Newton, A. C. (1993). The effect of fertilizer application on Dipterocarp seedling growth and mycorrhizal infection. For. Ecol. Manag. 57, 329–337. doi: 10.1016/0378-1127(93)90180-u
Tyree, M. T., Velez, V., and Dalling, J. W. (1998). Growth dynamics of root and shoot hydraulic conductance in seedlings of five neotropical tree species: scaling to show possible adaptation to differing light regimes. Oecologia 114, 293–298. doi: 10.1007/s004420050450
Valverde-Barrantes, O. J., Authier, L., Schimann, H., and Baraloto, C. (2021). Root anatomy helps to reconcile observed root trait syndromes in tropical tree species. Am. J. Bot. 108, 744–755. doi: 10.1002/ajb2.1659
Valverde-Barrantes, O., Baraloto, C., Schimann, H., and Authier, L. (2020). Root Anatomy Helps to Reconcile Observed Root Trait Syndromes in Tropical Tree Species – Data Set. Dryad. doi: 10.5061/DRYAD.5069P5068CZ5916
van der Heijden, M. G. A., and Scheublin, T. R. (2007). Functional traits in mycorrhizal ecology: their use for predicting the impact of arbuscular mycorrhizal fungal communities on plant growth and ecosystem functioning. New Phytol. 174, 244–250. doi: 10.1111/j.1469-8137.2007.02041.x
Vasconcelos, S. S., Zarin, D. J., Araujo, M. M., and Miranda, I. D. (2012). Aboveground net primary productivity in tropical forest regrowth increases following wetter dry-seasons. For. Ecol. Manag. 276, 82–87. doi: 10.1016/j.foreco.2012.03.034
Violle, C., Navas, M.-L., Vile, D., Kazakou, E., Fortunel, C., Hummel, I., et al. (2007). Let the concept of trait be functional! Oikos 116, 882–892. doi: 10.1111/j.2007.0030-1299.15559.x
Vitousek, P. M., and Sanford, R. L. (1986). Nutrient cycling in moist tropical forest. Annu. Rev. Ecol. Syst. 17, 137–167.
Vogt, K. A., Vogt, D. J., Palmiotto, P. A., Boon, P., Ohara, J., and Asbjornsen, H. (1996). Review of root dynamics in forest ecosystems grouped by climate, climatic forest type and species. Plant Soil 187, 159–219.
Wagner, F., Herault, B., Stahl, C., Bonal, D., and Rossi, V. (2011). Modeling water availability for trees in tropical forests. Agric. For. Meteorol. 151, 1202–1213. doi: 10.1016/j.agrformet.2011.04.012
Wallenda, T., and Kottke, I. (1998). Nitrogen deposition and ectomycorrhizas. New Phytol. 139, 169–187. doi: 10.1046/j.1469-8137.1998.00176.x
Wang, C. G., Chen, Z., Yin, H., Guo, W., Cao, Y., Wang, G. J., et al. (2018). The responses of forest fine root biomass/necromass ratio to environmental factors depend on mycorrhizal type and latitudinal region. J. Geophys. Res. Biogeosci. 123, 1769–1788. doi: 10.1029/2017jg004308
Wang, Y. P., Law, R. M., and Pak, B. (2010). A global model of carbon, nitrogen and phosphorus cycles for the terrestrial biosphere. Biogeosciences 7, 2261–2282. doi: 10.5194/bg-7-2261-2010
Waring, B. G., Perez-Aviles, D., Murray, J. G., and Powers, J. S. (2019). Plant community responses to stand-level nutrient fertilization in a secondary tropical dry forest. Ecology 100:12. doi: 10.1002/ecy.2691
Warren, J. M., Hanson, P. J., Iversen, C. M., Kumar, J., Walker, A. P., and Wullschleger, S. D. (2015). Root structural and functional dynamics in terrestrial biosphere models – evaluation and recommendations. New Phytol. 205, 59–78. doi: 10.1111/nph.13034
Weemstra, M., Kiorapostolou, N., Van Ruijven, J., Mommer, L., De Vries, J., and Sterck, F. (2019). The role of fine-root mass, specific root length and lifespan in tree performance: a whole-tree exploration. Dryad 34, 575–585. doi: 10.5061/DRYAD.931ZCRJG3
Weemstra, M., Mommer, L., Visser, E. J. W., van Ruijven, J., Kuyper, T. W., Mohren, G. M. J., et al. (2016). Towards a multidimensional root trait framework: a tree root review. New Phytol. 211, 1159–1169. doi: 10.1111/nph.14003
Wieder, W. R., Cleveland, C., Lawrence, D., and Bonan, G. B. (2015). Effects of model structural uncertainty on carbon cycle projections: biological nitrogen fixation as a case study. Environ. Res. Lett. 10:044016. doi: 10.1088/1748-9326/10/4/044016
Wittich, B., Homeier, J., and Leuschner, C. (2015). Ammonium, nitrate and glycine uptake of six Ecuadorian tropical montane forest tree species: an in situ pot experiment with saplings. J. Trop. Ecol. 31, 139–152. doi: 10.1017/s0266467414000650
Worden, J., Saatchi, S., Keller, M., Bloom, A. A., Liu, J., Parazoo, N., et al. (2021). Satellite observations of the tropical terrestrial carbon balance and interactions with the water cycle during the 21st century. Rev. Geophys. 59, 1–38.
Wright, S. J. (2019). Plant responses to nutrient addition experiments conducted in tropical forests. Ecol. Monogr. 89:18. doi: 10.1002/ecm.1382
Wurzburger, N., and Hedin, L. O. (2016). Taxonomic identity determines N-2 fixation by canopy trees across lowland tropical forests. Ecol. Lett. 19, 62–70. doi: 10.1111/ele.12543
Wurzburger, N., and Wright, S. J. (2015). Fine-root responses to fertilization reveal multiple nutrient limitation in a lowland tropical forest. Ecology 96, 2137–2146. doi: 10.1890/14-1362.1
Xu, X. T., and Trugman, A. T. (2021). Trait-based modeling of terrestrial ecosystems: advances and challenges under global change. Curr. Clim. Change Rep. 7, 1–13. doi: 10.1007/s40641-020-00168-6
Xu, X., Medvigy, D., Powers, J. S., Becknell, J. M., and Guan, K. (2016). Diversity in plant hydraulic traits explains seasonal and inter-annual variations of vegetation dynamics in seasonally dry tropical forests. New Phytol. 212, 80–95. doi: 10.1111/nph.14009
Yaffar, D., and Norby, R. J. (2020). A historical and comparative review of 50 years of root data collection in Puerto RicoPalabras Clave. Biotropica 52, 563–576. doi: 10.1111/btp.12771
Yaffar, D., Defrenne, C., Cabugao, K., Kivlin, S., Childs, J., Carvajal, N., et al. (2021). Trade-offs in phosphorus acquisition strategies of five common tree species in a tropical forest of Puerto Rico. Front. For. Global Change 4:698191. doi: 10.3389/ffgc.2021.698191
Yamauchi, T., Pedersen, O., Nakazono, M., and Tsutsumi, N. (2021). Key root traits of Poaceae for adaptation to soil water gradients. New Phytol. 229:8. doi: 10.1111/nph.17093
Yang, X., Thornton, P. E., Ricciuto, D. M., and Hoffman, F. M. (2016). Phosphorus feedbacks constraining tropical ecosystem responses to changes in atmospheric CO2 and climate. Geophys. Res. Lett. 43, 7205–7214. doi: 10.1002/2016gl069241
Yang, X., Thornton, P. E., Ricciuto, D. M., and Post, W. M. (2014). The role of phosphorus dynamics in tropical forests - a modeling study using CLM-CNP. Biogeosciences 11, 1667–1681. doi: 10.5194/bg-11-1667-2014
Yavitt, J. B., and Wright, S. J. (1996). Temporal patterns of soil nutrients in a Panamanian moist forest revealed by ion-exchange resin and experimental irrigation. Plant Soil 183, 117–129. doi: 10.1007/bf02185571
Yavitt, J. B., and Wright, S. J. (2001). Drought and irrigation effects on fine root dynamics in a tropical moist forest Panama. Biotropica 33, 421–434. doi: 10.1111/j.1744-7429.2001.tb00196.x
Yavitt, J. B., Harms, K. E., Garcia, M. N., Mirabello, M. J., and Wright, S. J. (2011). Soil fertility and fine root dynamics in response to 4 years of nutrient (N, P, K) fertilization in a lowland tropical moist forest, Panama. Austral Ecol. 36, 433–445. doi: 10.1111/j.1442-9993.2010.02157.x
Yokoyama, D., Imai, N., and Kitayama, K. (2017). Effects of nitrogen and phosphorus fertilization on the activities of four different classes of fine-root and soil phosphatases in Bornean tropical rain forests. Plant Soil 416, 463–476. doi: 10.1007/s11104-017-3225-x
Yu, L., Ahrens, B., Wutzler, T., Schrumpf, M., and Zaehle, S. (2020). Jena Soil Model (JSM v1.0; revision 1934): a microbial soil organic carbon model integrated with nitrogen and phosphorus processes. Geosci. Model Dev. 13, 783–803. doi: 10.5194/gmd-13-783-2020
Zaehle, S., and Friend, A. D. (2010). Carbon and nitrogen cycle dynamics in the O-CN land surface model: 1. Model description, site-scale evaluation, and sensitivity to parameter estimates. Glob. Biogeochem. Cycles 24:GB1005. doi: 10.1029/2009gb003521
Zaehle, S., Medlyn, B. E., De Kauwe, M. G., Walker, A. P., Dietze, M. C., Hickler, T., et al. (2014). Evaluation of 11 terrestrial carbon-nitrogen cycle models against observations from two temperate Free-Air CO2 Enrichment studies. New Phytol. 202, 803–822. doi: 10.1111/nph.12697
Zangaro, W., de Assis, R. L., Rostirola, L. V., de Souza, P. B., Goncalves, M. C., Andrade, G., et al. (2008). Changes in arbuscular mycorrhizal associations and fine root traits in sites under different plant successional phases in southern Brazil. Mycorrhiza 19, 37–45. doi: 10.1007/s00572-008-0202-5
Zangaro, W., Nisizaki, S. M. A., Domingos, J. C. B., and Nakano, E. M. (2003). Mycorrhizal response and successional status in 80 woody species from south Brazil. J. Trop. Ecol. 19, 315–324. doi: 10.1017/s0266467403003341
Zhang, Y., Xiao, L., Guan, D., Chen, Y., Motelica-Heino, M., Peng, Y., et al. (2021). The role of mangrove fine root production and decomposition on soil organic carbon component ratios. Ecol. Indic. 125:10 7525.
Zhu, Q., Riley, W. J., Tang, J. Y., Collier, N., Hoffman, F. M., Yang, X. J., et al. (2019). Representing nitrogen, phosphorus, and carbon interactions in the E3SM land model: development and global benchmarking. J. Adv. Model. Earth Syst. 11, 2238–2258. doi: 10.1029/2018ms001571
Keywords: fertility, drought, phosphorus, base cations, uptake, resource limitation, tropical forest, vegetation models
Citation: Cusack DF, Addo-Danso SD, Agee EA, Andersen KM, Arnaud M, Batterman SA, Brearley FQ, Ciochina MI, Cordeiro AL, Dallstream C, Diaz-Toribio MH, Dietterich LH, Fisher JB, Fleischer K, Fortunel C, Fuchslueger L, Guerrero-Ramírez NR, Kotowska MM, Lugli LF, Marín C, McCulloch LA, Maeght J-L, Metcalfe D, Norby RJ, Oliveira RS, Powers JS, Reichert T, Smith SW, Smith-Martin CM, Soper FM, Toro L, Umaña MN, Valverde-Barrantes O, Weemstra M, Werden LK, Wong M, Wright CL, Wright SJ and Yaffar D (2021) Tradeoffs and Synergies in Tropical Forest Root Traits and Dynamics for Nutrient and Water Acquisition: Field and Modeling Advances. Front. For. Glob. Change 4:704469. doi: 10.3389/ffgc.2021.704469
Received: 03 May 2021; Accepted: 05 October 2021;
Published: 02 December 2021.
Edited by:
Oriol Grau, Ecological and Forestry Applications Research Center (CREAF), SpainReviewed by:
Alan Feest, University of Bristol, United KingdomZachary E. Kayler, University of Idaho, United States
Junwei Luan, International Center for Bamboo and Rattan, China
Copyright © 2021 Cusack, Addo-Danso, Agee, Andersen, Arnaud, Batterman, Brearley, Ciochina, Cordeiro, Dallstream, Diaz-Toribio, Dietterich, Fisher, Fleischer, Fortunel, Fuchslueger, Guerrero-Ramírez, Kotowska, Lugli, Marín, McCulloch, Maeght, Metcalfe, Norby, Oliveira, Powers, Reichert, Smith, Smith-Martin, Soper, Toro, Umaña, Valverde-Barrantes, Weemstra, Werden, Wong, Wright, Wright and Yaffar. This is an open-access article distributed under the terms of the Creative Commons Attribution License (CC BY). The use, distribution or reproduction in other forums is permitted, provided the original author(s) and the copyright owner(s) are credited and that the original publication in this journal is cited, in accordance with accepted academic practice. No use, distribution or reproduction is permitted which does not comply with these terms.
*Correspondence: Daniela Francis Cusack, ZGFuaWVsYS5jdXNhY2tAY29sb3N0YXRlLmVkdQ==