- Department of Forest and Conservation Sciences, University of British Columbia, Vancouver, BC, Canada
Vegetation plays an important role in determining soil organic carbon (SOC) stocks, and influences the mechanisms through which SOC is stabilized within the soil. The type of vegetation selected for use in reclamation may therefore influence the accumulation rate and residence time of SOC in these ecosystems. Earlier studies at reclaimed sites in the Alberta Oil Sands demonstrated that reclaimed ecosystems planted with deciduous trees accumulated the most soil organic matter in the top 10 cm of reclamation material, followed by grass sites, while coniferous sites accumulated the least SOM. The objective of this study was to assess differences in SOC stabilization in the upper 10 cm of soil among revegetated deciduous, coniferous and grass ecosystems 20–40 years following reclamation. We compared soil C in unprotected, physically protected, and chemically protected forms among the three reclamation treatments using density flotation to isolate free particulate (unprotected) SOC from the soil sample, and size fractionation to separate the remaining sample into heavy particulate (physically protected) SOC and mineral-associated (chemically protected) SOC. In addition to this analysis, we used NaOCl oxidation to distinguish chemically resistant and chemically oxidizable C stocks. Chemically resistant C was consistent across all vegetation treatments at approximately 25% of total soil C, while the remaining 75% was chemically oxidizable. Total SOC stocks were also not significantly different among vegetation treatments. Deciduous sites had 57.8 Mg ha–1 SOC, grass sites had 52.7 Mg ha–1 SOC, and coniferous sites had 43.7 Mg ha–1 SOC. Two-thirds of total SOC at grass sites was in protected forms, compared to half of total SOC at coniferous sites and one-third of total SOC at deciduous sites (33.6, 22.6, and 15.6 Mg ha–1, respectively). Grass sites had significantly more physically protected SOC than deciduous sites while deciduous sites had more unprotected SOC than grass sites. Our findings indicate that the type of vegetation selected for reclaimed areas has important implications for soil carbon in persistent versus unprotected pools.
Introduction
Most organic carbon (C) in terrestrial ecosystems, approximately 1,400 petagrams, is stored in soil (Scharlemann et al., 2014), and restoration of disturbed ecosystems presents an opportunity to sequester atmospheric CO2 into persistent forms of soil C (Lal et al., 2018). Stocks of SOC reflect the balance between inputs of C (e.g., dead plant material, root and microbial exudates, and soil organism necromass) and losses of C (e.g., microbial respiration, leaching, and erosion). The SOC pool includes diverse compounds that have different residence times and respond differently to environmental factors such as climate or land use. Forms of SOC that have short residence times and respond quickly to changes in climate or land use are referred to as labile or fast-cycling SOC, while forms that have longer residence times and respond more slowly are typically referred to as stable SOC (Soucémarianadin et al., 2018). These forms are not inherently stable; rather they are in a state of “dynamic stability” due to biological, environmental, and physicochemical constraints on decomposition (Dynarski et al., 2020), and so can be better described as persistent or slow-cycling forms.
Slow-cycling SOC pools are created through three main pathways (Lützow et al., 2006). First, some organic matter inputs have complex chemical structures that make them resistant to decomposition and result in long residence times (chemically resistant C). Second, organic matter can become chemically protected by binding to mineral surfaces, making it inaccessible to decomposers and resulting in long residence times (mineral-associated organic matter or MAOM-C). Third, organic particles can become physically protected through incorporation into soil aggregates where they are partially protected from microbial enzymes (heavy particulate organic matter or hPOM-C). Within this physically protected pool, SOC is stored in different size classes of aggregates that offer varying degrees of protection. The strength of the binding agents that hold aggregates together increases as the aggregate size decreases, resulting in microaggregates being associated with older, more persistent SOC while macroaggregates are associated with younger, faster-cycling SOC (Jastrow et al., 1996; Six et al., 2004). Further, the roots and hyphae that bind macroaggregates together are thought to act as nuclei for microaggregate formation within macroaggregates, particularly in soils where organic matter (OM) is an important binding agent (Oades, 1984; Six et al., 2004). Uncomplexed SOC that is not physically or chemically protected from microbes is referred to as the free light fraction, or free particulate organic matter (fPOM-C) and is a fast-cycling C pool. Fast-cycling soil C can promote the production of persistent SOC by stimulating production of microbial biomass, residues and decay products (Kleber et al., 2011; Dungait et al., 2012; Cotrufo et al., 2013).
Vegetation type influences the rate of input, the chemical structure of C compounds entering the soil, and the relative magnitude of the various SOC stabilization pathways. Grasses typically have abundant fine and very fine roots, which are more likely to interact with aggregates and clay minerals than medium or coarse roots, facilitating the generation of chemically and physically protected SOC (Prescott et al., 2019). Grassland establishment on degraded soils increases soil C stocks as much or more than afforestation (Poeplau et al., 2011; Wei et al., 2012), and conversion of grasslands to forests often cause SOC stocks to decline (Guo and Gifford, 2002; Poeplau et al., 2011). Grass ecosystems had greater stocks of chemically protected SOC (Eclesia et al., 2012) and physically protected SOC than nearby forests (Guidi et al., 2014). In forest ecosystems, deciduous trees typically have deeper and more extensive root systems than conifers, which contribute more organic matter to the soil (Finér et al., 2007; Laganière et al., 2010). As a result, broadleaved species tend to have greater C stocks in the mineral soil, whereas coniferous species accumulate more C in the forest floor (Peng et al., 2020). Deciduous tree species are often associated with greater macrofaunal activity than coniferous forests (Józefowska et al., 2016), which mix surface organic material with mineral soil (Frouz et al., 2006). Soil macrofauna also add mucus, which acts as a cementing agent, holding organic and mineral particles together and creating biogenic structures like earthworm casts that contribute to aggregation (Bottinelli et al., 2015). By stimulating microbial growth, earthworm mucus also increases microbial necromass and production of extracellular polymeric substances which promote the creation of micro- and macroaggregates (Angst et al., 2019a).
Reclaimed ecosystems can sequester substantial amounts of soil C if they are managed to promote soil C stabilization (Akala and Lal, 2001). The type of vegetation planted on reclaimed sites (e.g., grasses, broadleaved trees or coniferous trees) can influence rates of soil C sequestration and stabilization. Degraded soils restored to grassland or pasture often accumulate soil C faster than those restored to forest (Akala and Lal, 2001; Wei et al., 2012; Frouz et al., 2013). Reclaimed sites planted with deciduous trees accumulate SOC more rapidly and deeper in the soil profile than sites with coniferous trees (Frouz et al., 2009, 2013; Laganière et al., 2010; Vindušková and Frouz, 2013). At 93 restoration sites summarized by Frouz et al. (2013), mean rates of SOC accumulation were significantly lower in coniferous forests than in grasslands or deciduous forests.
Reclaimed ecosystems provide an opportunity to isolate the effects of vegetation on SOC stabilization pathways while minimizing the influence of confounding factors such as differences in parent material, soil properties and site characteristics that typically determine the distribution of vegetation communities and SOC pools on the landscape (Angst et al., 2018; Wiesmeier et al., 2019). In reclaimed ecosystems in the oil sands region of Alberta, Canada, Anderson et al. (2019) reported the greatest accumulations of soil organic matter (SOM) at reclaimed sites planted with deciduous trees, followed by sites seeded with grasses, while reclaimed sites planted with coniferous trees had the least SOM. This difference in reclaimed vegetation treatments was attributed to greater macrofaunal activity and higher root biomass at deciduous and grass treatments, compared to coniferous treatments. All reclaimed vegetation treatments had substantially more organic matter in the mineral soil than natural sites in the area (Anderson et al., 2019). We sampled the same reclaimed ecosystems dominated by deciduous trees, coniferous trees and grass to determine the proportion of SOC in each vegetation treatment in fast and slow-cycling pools – specifically in uncomplexed, physically protected, chemically protected, and chemically resistant SOC pools. We hypothesized that reclaimed grass sites would have the most physically and chemically protected SOC, and that aggregation would be greater in soils at grass and deciduous sites than at sites with coniferous vegetation.
Materials and Methods
Study Sites
The study sites are located in the Athabasca Oil Sand region in the Boreal Mixedwoods region of Alberta, Canada. The area has long, cold winters and short, warm summers. Mean monthly air temperatures range from 17.1°C in July to −17.4°C in January (Environment Canada, 2020). Mean annual precipitation is 418.6 mm and two-thirds of this precipitation falls as rain during the growing season (Environment Canada, 2020). This is an undulating landscape of uplands dominated by aspen (Populus tremuloides Michx.) and white spruce (Picea glauca [Moench] Voss.), with jack pine (Pinus banksiana Lamb.) in well drained areas, and lowlands dominated by black spruce (Picea mariana [Mill.] BSP) and larch (Larix laricina [Du Roi] K. Koch). Upland soils are primarily Eutric Brunisols and Gray Luvisols that have developed on glaciofluvial, glaciolacustrine and glacial till deposits (Soil Classification Working Group, 1998). Organic soils are common in lowlands and consist of decomposed plant remains, primarily sphagnum (Sphagnum spp.), feather mosses (various species) and Labrador tea (Rhododendron). Because of the abundance of organic soils in the Oil Sands disturbance footprint, the salvaged coversoils used in reclamation are typically composed of peat or a mixture of peat and mineral upland soils. Wildfire is the dominant natural disturbance regime in the Boreal mixedwood region (Bergeron et al., 2014).
Fifteen sites were selected in reclaimed portions of oil sands mining leases north of Fort McMurray, AB (56°39′12′′ N, 111°13′24′′ W). Five sites had been planted with coniferous tree species (mostly white spruce), five with deciduous tree species (mostly trembling aspen) and five had been seeded with grasses (primarily Festuca spp. and Bromus inermis [Leyss.]). Forested sites had at least 80% tree cover and grass sites had less than 10% tree cover and less than 20% shrub cover (Anderson et al., 2019). Vegetation composition of the study sites is detailed in Anderson et al. (2019). Briefly, understory cover at deciduous sites consisted of grasses (20%), shrubs (15%), forbs (6%), and mosses (4%), while coniferous site understory was primarily mosses (46%), followed by forbs (11%), shrubs (5%) and very few grasses (1%). Other than planting prescription, the 15 study sites received similar reclamation treatments and had been reclaimed approximately 30 years earlier. All were classified as mesic upland sites and had a peat-mineral mix cover-soil over overburden or secondary materials. Peat-mineral mixes are defined as mineral soils with less than 17% organic carbon, and the peat-to-mineral ratio targeted for reclamation covers ranges from 50:50 to 70:30 (Alberta Environment Water, 2012). Initial SOC content of cover material was not available for the study sites. Cover-soil depths ranged from 10 to 100 cm, average depths and ranges within each vegetation treatment were similar (34 cm on average), and differences in cover-soil depths were not a confounding factor. All sites had a soil texture of either Sandy loam or Loamy sand. Only sites that were reclaimed at least 20 years prior were selected for sampling. At the time of sampling, deciduous and coniferous sites ranged from 21 to 29 years since reclamation, while grass sites ranged from 28 to 41 years.
Soil Sampling
Study sites were sampled in 2013. At each site, one 10-m2 plot was subdivided into 10 1-m2 subplots and three subplots were randomly selected for sampling. At each subplot, a 30-cm deep soil pit was dug and described, including a brief description of organic and mineral horizons. Organic surface horizons were removed and soil structure samples were carefully extracted from the top 10 cm of each subplot using a long-tipped trowel to avoid soil compaction and minimize the crushing of soil aggregates. A subsample of soil homogenized from the three soil pits was put on ice and stored at −18°C until laboratory analyses were performed. All other bulk soil samples were stored at 4°C until they could be further processed. The top 10 cm of mineral soil was selected as vegetation species influences on soils tend to appear sooner in the upper layers. Additionally, Anderson et al. (2019), found differences in SOM contents among these vegetation treatments only in the top 10 cm.
Bulk Density, Root Biomass and Extractable Microbial Biomass Carbon
Bulk density was determined by cutting a 10-cm3 block of soil from each soil pit and carefully extracting all soil from the hole. Field moist samples were then weighed and air-dried at 105°C to determine gravimetric water content. Bulk density was averaged by site and corrected for gravel and coarse fragments using the equation described by Page-Dumroese et al. (1999) to calculate fine bulk density. Root biomass was measured by hand-sorting roots into four size classes: very fine (<1 mm), fine (1–2 mm), medium (2–5 mm) and coarse (>5 mm). Root samples were then washed, dried in a forced-air oven at 60°C, and then weighed. Bulk density results were used to express root biomass in megagrams per hectare. Extractable microbial biomass carbon (eMBC) was measured in fresh soil samples the same day they were sampled using the chloroform-fumigation direct-extraction method from Tate et al. (1988). Extracts were frozen, stored at −20°C, and sent to the Analytical Service Laboratory of the University of Alberta where total organic carbon was measured using a TOC-TN autoanalyzer (Shimadzu, Kyoto, Japan). Bulk density results were used to express extractable eMBC in megagrams per hectare (Mg ha–1). Microbial biomass C was not calculated because a calibration factor (kEC) from natural soils would not be reliable for these peat-rich materials.
Aggregate Size Separation
Field-moist soil blocks were gently broken apart by hand and passed through an 8-mm sieve before air-drying. Subsamples of air-dried soil (50 g) were wet-sieved using the method described in Elliot (1986) and adapted by Six et al. (2000) to separate bulk soil into four aggregate size classes: large macroaggregates (2,000 – 8,000 μm; large), macroaggregates (250 – 2,000 μm; macro), microaggregates (53 – 250 μm; micro) and silt and clay particles (<53 μm; S&C). Briefly, samples were slaked in distilled water for 5 min to disrupt unstable aggregates through the buildup and release of air and pressure, leaving only water-stable aggregates (Laganière et al., 2010). Samples were then sieved through a series of sieves (2,000, 250, and 53 μm), and material that remained on each sieve was collected in pre-weighed aluminum drying pans. Any floating organic material that was left in the 2,000-μm sample was discarded because it is considered too large to be part of the SOC fraction (Six et al., 2002). Samples were dried in a forced-air oven at 60°C and weighed. Individual fraction weights are expressed as a percentage of dry soil weight.
Size-Density Fractionation
Density and size fractionation methods described by Laganière et al. (2011) and adapted from Gregorich et al. (2006) were used to separate soil into uncomplexed (fPOM), physically protected (hPOM), and chemically protected (MAOM) C pools. Briefly, 25-g soil samples, previous sieved through an 8-mm sieve and air-dried, were gently shaken in a 1.7 g cm–3 solution of sodium iodide (NaI) for 20 min and allowed to settle for 48 h. The suspended fPOM was then recovered from the surface of each sample using vacuum-filtration equipment. This recovered light fraction was rinsed several times with a 0.01-M calcium dichloride (CaCl2) solution and distilled water, then oven-dried at 60°C and weighed. Size separation was used to separate the remaining soil into heavy and mineral-associated fractions. The sample was rinsed as described above and centrifuged for 15 min at 2,000 rpm several times. Aggregates were then dispersed in the sample using glass beads and a reciprocal shaker, and the dispersed sample was wet-sieved through a 53-μm sieve. The sample remaining on the sieve (hPOM) and the sample that passed through the sieve (MAOM) were oven-dried at 60°C and weighed. Organic matter content was measured in each size-density fraction using the loss-on-ignition method (Rabenhorst, 1988). The LOI method used in this study was tested using calibrated controls (Masse et al., 2017), and was selected to facilitate comparison between previous work on the same study sites that used the same method. Organic C content was determined using the coefficient 1.724 (Rabenhorst, 1988). Bulk density results were used to express soil C pools in megagrams per hectare (Mg ha–1).
Separating Chemically Oxidizable and Chemically Resistant Carbon Pools Using Oxidation
Soil C was separated into chemically oxidizable and chemically resistant pools using sodium hypochlorite (NaOCl) oxidation (described by Zimmermann et al., 2007). This analysis was used to divide total soil C into an old, more recalcitrant C pool and a younger, more labile C pool. A 25-g sample of air-dried, sieved (2 mm) soil was added to 50 mL of 6% (wt/wt) NaOCl. Samples were oxidized for 16 h at 25°C, then washed with distilled water, dried at 60°C and weighed. The difference between the initial sample weight and the NaOCl-treated sample weight represents the chemically oxidizable organic material. Total C and total N were measured in NaOCl-treated samples and untreated samples by dry combustion using an elemental analyzer (Elementar Vario EL Cube, Hanau, Germany). Changes in mass and C contents before and after oxidation were used to calculate chemically resistant C and chemically oxidizable C, which are expressed as megagrams per hectare. Total N content was used to determine C:N ratios of chemically resistant and chemically oxidizable C pools.
Soil texture, cation exchange capacity, pH and soil inorganic carbon measured at the study sites were reported by Masse et al. (2017). Soil inorganic carbon was found to be negligible at all sites.
Statistical Analyses
Data from the three subplots at each site were averaged to determine mean soil C stocks, aggregate size fractions, root biomass and bulk density. Composite soil samples were used to determine microbial biomass, CEC, exchangeable cations, conductivity and pH. One-way analysis of variance (ANOVA) was used to determine significant differences among vegetation treatments (n = 5). Data that did not meet assumptions of normality were log-transformed to ensure that assumptions were met. Tukey HSD tests were used to compare differences among treatments. A significance threshold of α = 0.05 was used for all statistical analyses, although differences up to α = 0.1 are noted in recognition of the considerable variability associated with the use of actual reclamation treatments in the field. Canonical redundancy analyses (RDA) were used to identify variables that significantly explained variation among vegetation treatments in soil C fractions and aggregate size-classes. The following variables were hypothesized to explain variation in soil C fractions: microaggregates, small macroaggregates, C:N ratios, depth of the organic surface horizon, pH, CEC, biomass of very fine roots, eMBC, understory cover, total cover, and the clay-and-silt fraction. Additionally, very fine roots, total SOC, C:N ratios, pH, CEC, understory cover, eMBC, total cover, Ca, Mg and the clay-and-silt fraction were hypothesized to explain variation in aggregate size-classes. Cover data was not available for two of the sites (one deciduous and one grass), therefore only 13 sites were included in RDA analysis. Significant variables were selected using a forward-selection algorithm: vegan’s ordistep function (Oksanen et al., 2020). The RDA model, axis, and explanatory variables were tested using a permutation test (Bordcard et al., 2011). R software (ver.4.0.3) was used for all analyses (R Core Team, 2020).
Results
The surface organic layer was deepest at deciduous sites and shallowest at coniferous sites (Table 1, p = 0.08). Total root biomass was greater at deciduous sites than at grass sites (p = 0.08), primarily due to the abundance of very fine roots at deciduous sites. More than half of total root biomass at deciduous and grass sites was in the form of very fine roots, while coarse roots made up the biggest portion of root biomass at coniferous sites (40%). Soil bulk density and eMBC were similar among treatments (0.82 ± 0.05 g cm–3 and 0.92 ± 0.08 Mg C ha–1, respectively). Grass sites had a mean soil pH of 7.0, while coniferous and deciduous sites were significantly more acidic (6.5 and 6.4, respectively).

Table 1. Site and soil properties measured in the three vegetation treatments (mean ± standard error).
Large macroaggregates were the most abundant size class of aggregates in soils at coniferous sites whereas macroaggregates were most abundant at deciduous and grassland sites (Figure 1). Differences in aggregate size-classes among treatments were only significant for small size-fractions. Microaggregates represented a greater portion of the soil dry weight at grass sites than at coniferous sites (p = 0.04) or deciduous sites (p = 0.08). The silt-and-clay-sized fraction was also greater at grassland sites than at deciduous (p = 0.01) and coniferous (p = 0.07) sites.
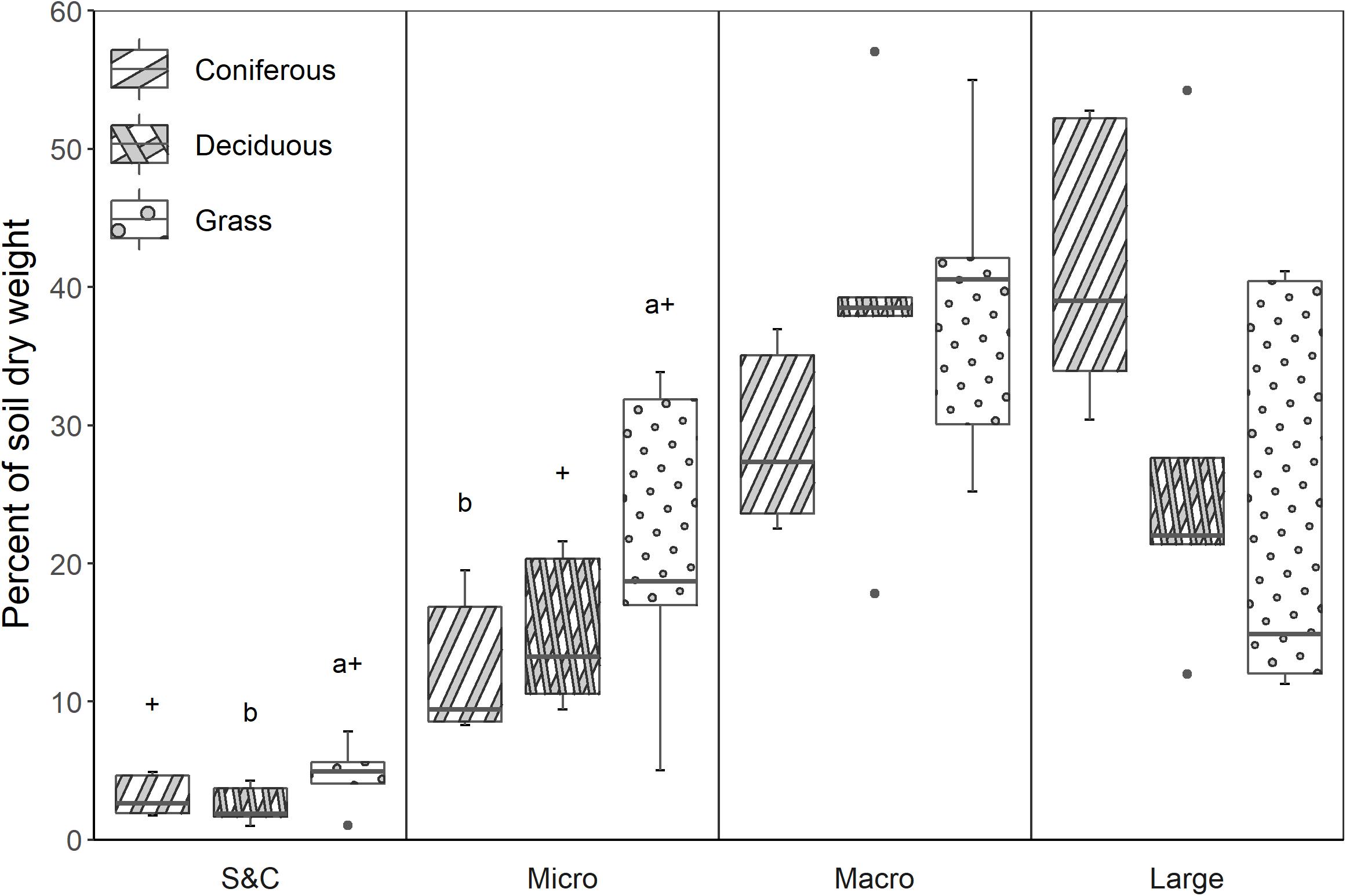
Figure 1. Distribution of aggregate size classes (g aggregate g–1 dry soil) among the three vegetation treatments, as a percentage of the total soil dry weight (S&C = silt-and-clay-sized fraction; Micro = microaggregates; Macro = macroaggregates; Large = large macroaggregates). Significant differences (p ≤ 0.05) among treatments are noted using a letter above the boxplot, and differences in which p ≤ 0.10 are symbolized using +.
Stocks of total SOC were greatest at deciduous sites, followed by grass sites, and then coniferous sites (Table 2). No significant differences in total SOC were found among vegetation treatments, however, SOC was distributed differently among size-density fractions in the different vegetation treatments (Figure 2). At deciduous sites, two-thirds of total SOC was in unprotected forms (fPOM-C), compared to half of total SOC at coniferous sites and one-third of total SOC at grass. Grass sites had a larger hPOM-C pool than coniferous (p = 0.07) or deciduous (p = 0.02) sites. Stocks of MAOM-C were greater at grass sites than deciduous sites (p = 0.09), while coniferous and grass sites were similar. Total stocks of protected SOC were 33.6 Mg ha–1 at grass sites, 22.6 Mg ha–1 at coniferous sites, and 15.6 Mg ha–1 at deciduous sites.
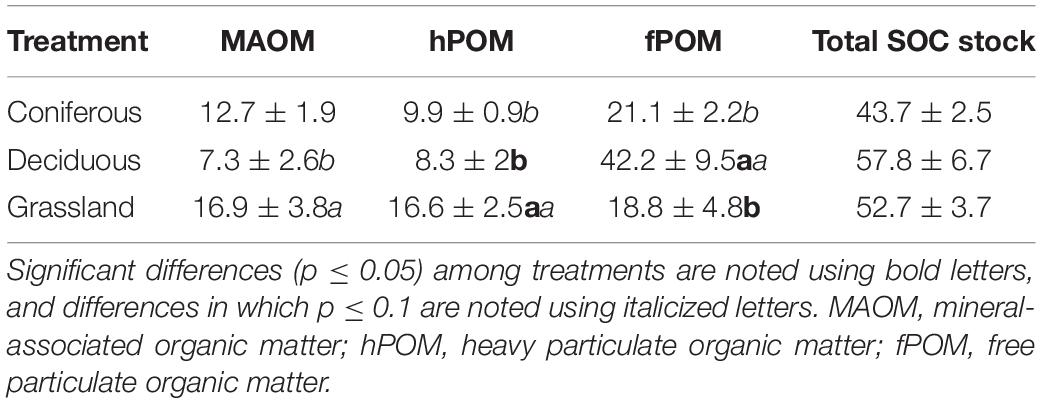
Table 2. Total soil organic C stocks among the three reclaimed vegetation treatments, as well as organic C stored within different size-density fractions in the soil, in Mg C ha–1.
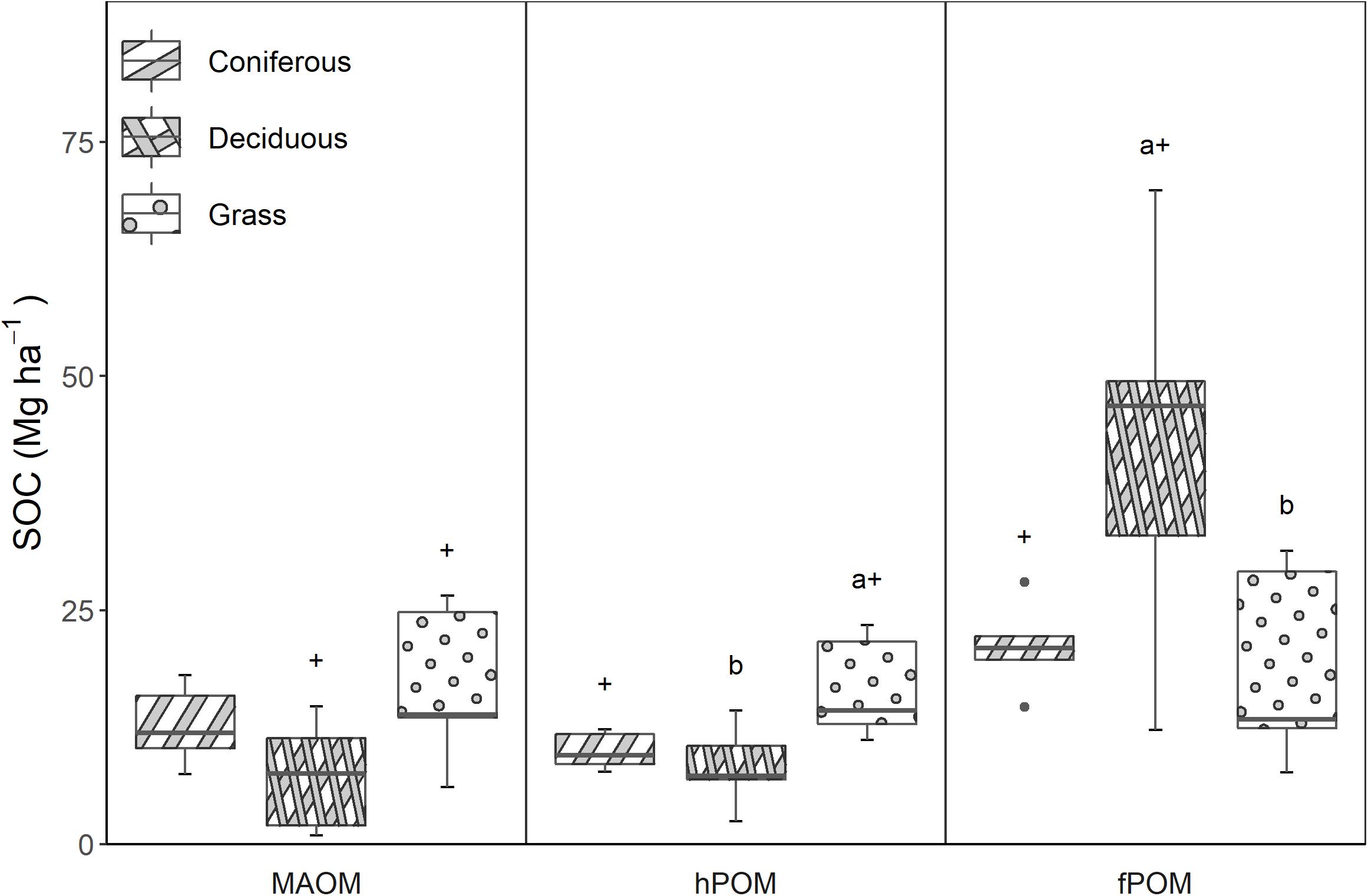
Figure 2. Stocks of soil organic C in MAOM (mineral-associated organic matter), hPOM (heavy particulate organic matter) and fPOM (free particulate organic matter) pools among the three reclaimed vegetation treatments. Significant differences (p ≤ 0.05) among treatments are noted using a letter above the boxplot, and differences in which p ≤ 0.10 are symbolized using +.
Across all vegetation treatments, at least 75% of all soil C was chemically oxidizable, while the remaining 25% was chemically resistant (Figure 3). Treatment means for chemically oxidizable C ranged from 41.7 Mg ha–1 at grass sites to 50.7 Mg ha–1 at deciduous sites, while the chemically resistant pool ranged from 8.22 ha–1 at deciduous sites to 14.23 ha–1 at coniferous sites. No significant differences were observed among the three vegetation treatments.
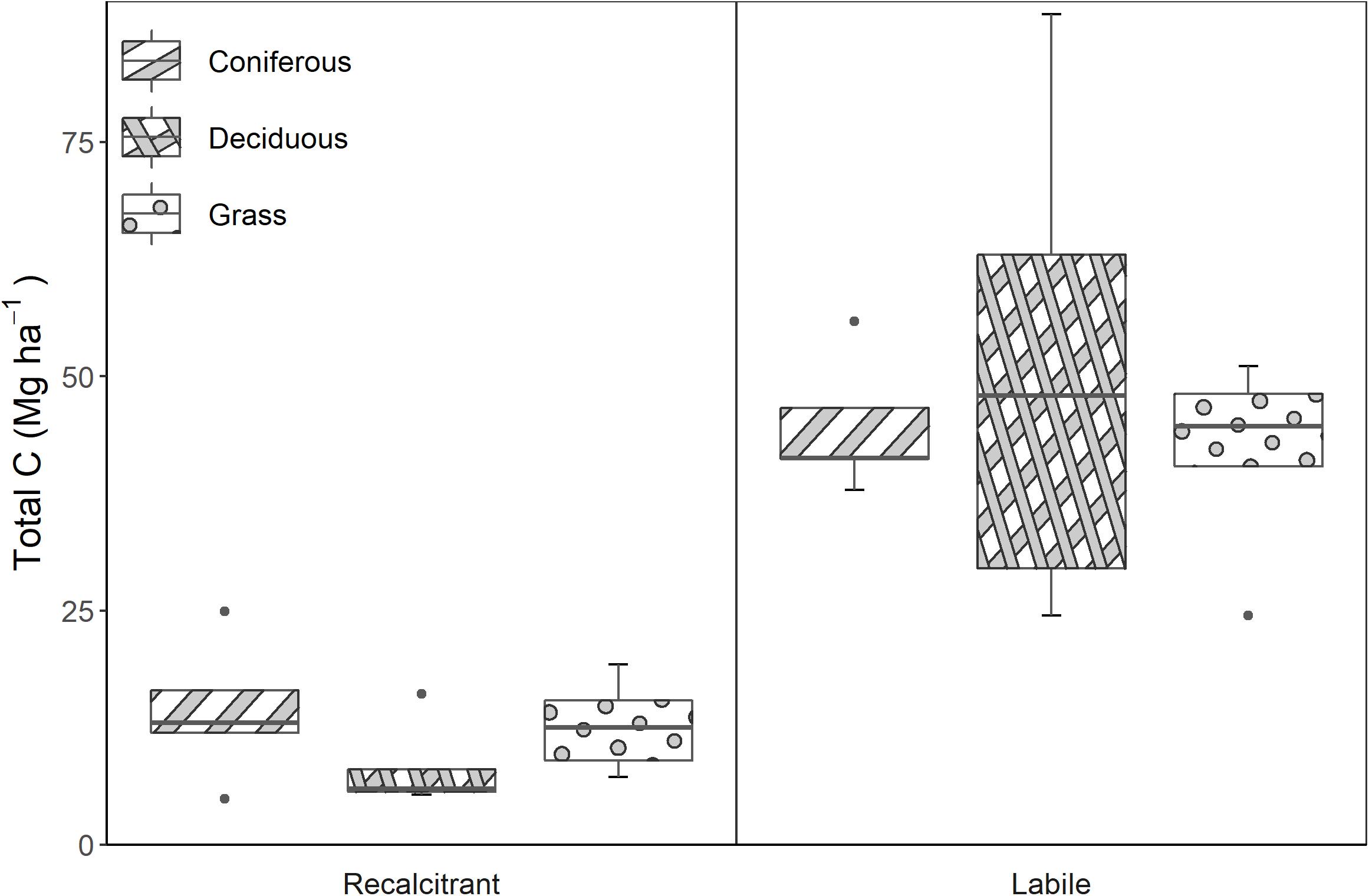
Figure 3. Total C in chemically resistant and chemically oxidizable pools in the three reclaimed vegetation treatments. There were no significant differences among treatments.
Canonical redundancy analysis indicated that 59% of the variation in the distribution of C fractions across the 13 study sites used in this analysis could be explained by environmental variables (Figure 4; F = 6.62, p = 0.001), specifically understory cover (F = 7.77, p ≤ 0.01) and macroaggregates (F = 9.79, p ≤ 0.01). Very fine roots and pH contributed to the explained variation, but were not significant explanatory variables. RDA1 was the only significant axis (F = 16.72, p ≤ 0.01). Macroaggregates explained 30% of the variation and were positively correlated with the uncomplexed C fraction (fPOM) and deciduous sites. Understory cover explained 27% of the variation in C fractions and was positively correlated with protected pools of C (hPOM-C and MAOM-C) and grass sites. The three vegetation treatments overlapped, indicating similarity in C pools across vegetation treatments, although grass and deciduous treatments diverged slightly. Canonical redundancy analysis was also used to explore whether environmental variables could explain the distribution of aggregate size classes, but the model was not significant.
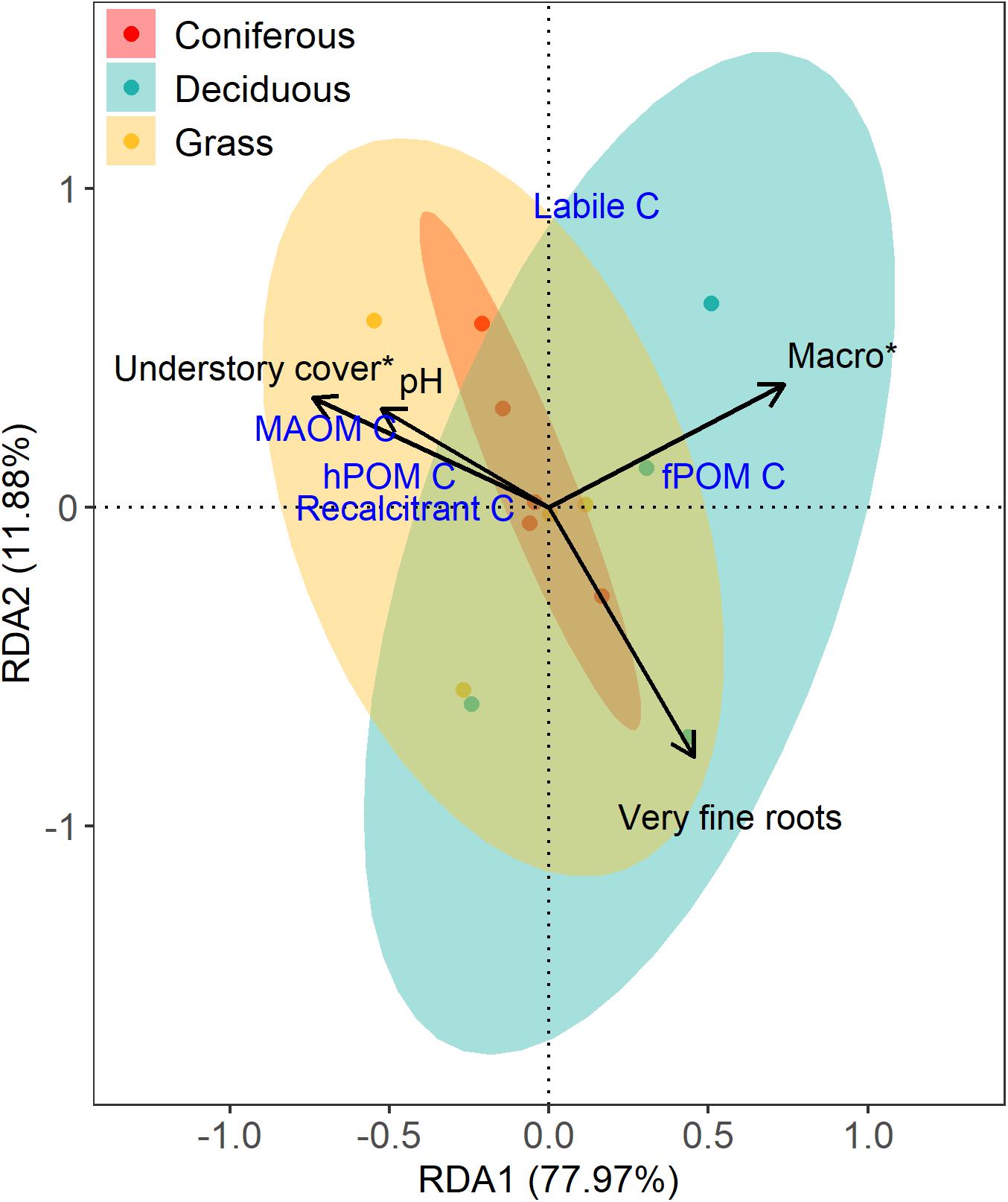
Figure 4. Canonical redundancy analysis (scaling 2) demonstrating the relationships among vegetation and soil properties, and soil C pools in the studied soils. Blue text represents response variables and black text and arrows represent the environmental variables that explained variance in the response variables among study sites. Significant explanatory variables (p ≤ 0.05) are indicated by a star.
Discussion
Consistent with our hypothesis that reclaimed sites with grass would have the most physically protected SOC, grass sites had more physically protected C (hPOM-C) than coniferous or deciduous treatments. These results suggest that soil carbon at grass sites may be more resistant to losses through disturbances such as wildfire than soil carbon at afforested sites. Microaggregates were also greatest in the grass treatment, while macroaggregates and large macroaggregates did not differ among treatments. These results suggest that the greater C in protected pools at grass sites can be attributed to SOC protected within microaggregates. Guidi et al. (2014) also found that the greater SOC in grasslands compared to adjacent afforested areas was primarily due to SOC stored in small aggregates. Accumulation of SOC under grasses is commonly attributed to greater fine roots compared to forest soils, and therefore greater association between root biomass and mineral surfaces (Poeplau et al., 2011; Wei et al., 2012). However, fine and very fine root biomass were similar between vegetation treatments in this study. Very fine roots were also not a significant variable in explaining the variation in SOC pools, suggesting that differences in root biomass among vegetation treatments were not a strong driver of aggregation or SOC stabilization in the top 10 cm. The greater stocks of C in small aggregates at grass sites may be a consequence of the dominance of arbuscular mycorrhizae (AM) in the ecosystems. AM-associated plants, including most grass and herb species, release proteins such as glomalin, which act as binding agents, increasing aggregate stability, and contributing to greater pools of physically and chemically protected SOC (Rillig, 2004; Bedini et al., 2009; Dignac et al., 2017). Boreal forest tree species, both coniferous and deciduous, are dominated by ectomycorrhizal (ECM) fungi (Policelli et al., 2020).
We also hypothesized that reclaimed sites with grass would have the most chemically protected SOC (MAOM-C). Although MAOM-C was not significantly different among vegetation treatments, average values were larger at grass sites than deciduous sites. Other studies have reported no measurable change in MAOM-C after 40–50 years, and suggested that ecosystem development on a decadal timeframe is too short to detect changes in this slow-cycling C pool (Lajtha et al., 2014; Angst et al., 2019b).
Contrary to our hypothesis, soils at deciduous sites did not have greater aggregation; instead they had twice as much uncomplexed C (fPOM-C) as coniferous or grass treatments. Our expectation of greater soil aggregation, and therefore greater physically protected C, at sites with deciduous trees was based on common characteristics of deciduous/broadleaved tree species such as rapidly decomposing leaf litter (rich in nutrients, calcium, and soluble compounds) which promotes burrowing earthworms, and AM fungal associates (Reich et al., 2005; Phillips et al., 2013). However, aspen leaf litter does not decompose much faster than spruce needle litter (Prescott et al., 2000, 2004; Jerabkova et al., 2006), and in the boreal mixedwood forests of western Canada, trembling aspen roots are dominated by ECM fungi (Visser et al., 1998). Burrowing earthworms are not abundant, rather the soil macrofauna are detritivores that comminute litter and transform it into fecal pellets with minimal mixing into mineral soil. Intact macrofaunal fecal material was abundant in the forest floor and the upper 10 cm of mineral soil at deciduous sites (Anderson et al., 2019), and would have contributed to the abundance of fPOM at these sites. The greater amount of fPOM-C at deciduous sites can also be attributed to greater litter input in deciduous stands in boreal mixedwood forests (Jerabkova et al., 2006) and the 9-year retention time of needles of spruce (Anderson et al., 2019) which forestalls the development of forest floors in spruce stands.
Understory cover explained 27% of the variation in C pools and was positively correlated with protected pools of C (hPOM-C and MAOM-C) and grass sites. At grass sites, understory cover was often equivalent to total cover and consisted of grasses and to a lesser extent, herbaceous species. Understory vegetation at deciduous sites was dominated by grasses and shrubs whereas mosses dominated on coniferous sites (Anderson et al., 2019). This finding indicates an important role of understory vegetation, particularly grasses, in generating stocks of protected soil C (MAOM-C and hPOM-C) during early phases of restoration in these ecosystems. Deciduous sites had greater grass covers than coniferous sites, but this did not translate into greater protected pools of C at deciduous sites. This can be attributed to grass cover being on average only 20% (2–60% range) at deciduous sites compared with 75% grass cover (44–96% range) at grass sites. We propose that the grass cover at deciduous sites was not great enough to significantly increase protected C pools, and as canopy closure is reached at these sites, grasses will likely be replaced by the natural regeneration of herbaceous species and shrubs that may not have the same effect of generating stocks of protected soil C. As protected forms of SOC are more resistant to loss through disturbances such as wildfire than are unprotected forms of C, inclusion of native grasslands in reclaimed landscapes could assist in long-term soil development and C sequestration.
Although there were some indications of different SOC stabilization pathways among the vegetation treatments, there was also significant overlap in their distributions in the multivariate analyses. This could be attributable to peat from the original peat-mineral mix in reclaimed soils masking smaller changes in the quantity and chemical composition of inputs from the three vegetation treatments. Nuclear magnetic resonance (NMR) spectra indicated that SOM in reclaimed soils under different vegetation treatments was chemically homogeneous, while natural soils in the Oil Sands region were more variable and their chemical compounds were more closely tied to the different vegetation types (Turcotte et al., 2009). These results suggest that the reclaimed ecosystems have not developed to the point where SOC clearly reflect the dominant stabilization processes associated with the type of vegetation planted on the site, as would be expected in natural ecosystems (Crow et al., 2009; Dawud et al., 2016; Angst et al., 2019b).
Conclusion
We compared soil organic C pools at reclaimed sites revegetated with deciduous trees, coniferous trees or grass in the boreal forest. Although total SOC stocks did not differ, the relative stabilization of SOC differed among vegetation treatments. At reclaimed grass sites, most SOC was physically and/or chemically protected, whereas most SOC at reclaimed deciduous sites was in unprotected forms (coniferous sites were intermediate). Our findings indicate that the type of vegetation selected for reclaimed areas has important implications for soil carbon in persistent versus unprotected pools.
Data Availability Statement
The raw data supporting the conclusions of this article will be made available by the authors, without undue reservation.
Author Contributions
Both authors listed have made a substantial, direct and intellectual contribution to the work, and approved it for publication.
Funding
This research was funded by the Natural Sciences and Engineering Research Council (NSERC) of Canada through a Collaborative Research and Development Grant (CRD 401580-10), which was co-financed by the Environmental Reclamation Research Group (ERRG) of the Canadian Oil Sands Network for Research and Development (CONRAD).
Conflict of Interest
The authors declare that the research was conducted in the absence of any commercial or financial relationships that could be construed as a potential conflict of interest.
Publisher’s Note
All claims expressed in this article are solely those of the authors and do not necessarily represent those of their affiliated organizations, or those of the publisher, the editors and the reviewers. Any product that may be evaluated in this article, or claim that may be made by its manufacturer, is not guaranteed or endorsed by the publisher.
Acknowledgments
We thank Jacynthe Masse, Eli Rechtschaffen, Jeff Anderson, Julia Amerongen Maddison, Dru Yates, and Carolyn King for their help in the field and in the lab, as well as participating in many useful discussions about the project; and Jérôme Laganière and Les Lavkulich for providing guidance related to lab methodology.
Supplementary Material
The Supplementary Material for this article can be found online at: https://www.frontiersin.org/articles/10.3389/ffgc.2021.689594/full#supplementary-material
References
Akala, V. A., and Lal, R. (2001). Soil Organic Carbon Pools and Sequestration Rates in Reclaimed Minesoils in Ohio. J. Environ. Qual. 30, 2098–2104. doi: 10.2134/jeq2001.2098
Alberta Environment Water. (2012). Best Management Practices For Conservation Of Reclamation Materials In The Mineable Oil Sands Region Of Alberta. Fort McMurray: Terrestrial Subgroup, Best Management Practices Task Group of the Reclamation Working Group of the Cumulative Environmental Management Association.
Anderson, J., Prescott, C. E., and Grayston, S. J. (2019). Organic matter accumulation in reclaimed soils under spruce, poplar and grass in the Alberta Oil Sands. New For. 50, 307–322. doi: 10.1007/s11056-018-9646-4
Angst, G., Messinger, J., Greiner, M., Häusler, W., Hertel, D., Kirfel, K., et al. (2018). Soil organic carbon stocks in topsoil and subsoil controlled by parent material, carbon input in the rhizosphere, and microbial-derived compounds. Soil Biol. Biochem. 122, 19–30. doi: 10.1016/j.soilbio.2018.03.026
Angst, G., Mueller, C. W., Prater, I., Angst, Š, Frouz, J., Jílková, V., et al. (2019a). Earthworms act as biochemical reactors to convert labile plant compounds into stabilized soil microbial necromass. Commun. Biol. 2, 1–7. doi: 10.1038/s42003-019-0684-z
Angst, G., Mueller, K. E., Eissenstat, D. M., Trumbore, S., Freeman, K. H., Hobbie, S. E., et al. (2019b). Soil organic carbon stability in forests: distinct effects of tree species identity and traits. Glob. Chang. Biol. 25, 1529–1546. doi: 10.1111/gcb.14548
Bedini, S., Pellegrino, E., Avio, L., Pellegrini, S., Bazzoffi, P., Argese, E., et al. (2009). Changes in soil aggregation and glomalin-related soil protein content as affected by the arbuscular mycorrhizal fungal species Glomus mosseae and Glomus intraradices. Soil Biol. Biochem. 41, 1491–1496. doi: 10.1016/j.soilbio.2009.04.005
Bergeron, Y., Chen, H. Y. H., Kenkel, N. C., Leduc, A. L., and Macdonald, S. E. (2014). Boreal mixedwood stand dynamics: ecological processes underlying multiple pathways. For. Chron. 90, 202–213. doi: 10.5558/tfc2014039
Bordcard, D., Gillet, F., and Legendre, P. (2011). Numerical Ecology With R. New York, NY: Springer.
Bottinelli, N., Jouquet, P., Capowiez, Y., Podwojewski, P., Grimaldi, M., and Peng, X. (2015). Why is the influence of soil macrofauna on soil structure only considered by soil ecologists? Soil Tillage Res. 146, 118–124. doi: 10.1016/j.still.2014.01.007
Cotrufo, M. F., Wallenstein, M. D., Boot, C. M., Denef, K., and Paul, E. (2013). The Microbial Efficiency-Matrix Stabilization (MEMS) framework integrates plant litter decomposition with soil organic matter stabilization: do labile plant inputs form stable soil organic matter? Glob. Chang. Biol. 19, 988–995. doi: 10.1111/gcb.12113
Crow, S. E., Lajtha, K., Filley, T. R., Swanston, C. W., Bowden, R. D., and Caldwell, B. A. (2009). Sources of plant-derived carbon and stability of organic matter in soil: implications for global change. Glob. Chang. Biol. 15, 2003–2019. doi: 10.1111/j.1365-2486.2009.01850.x
Dawud, S. M., Raulund-Rasmussen, K., Domisch, T., Finér, L., Jaroszewicz, B., and Vesterdal, L. (2016). Is Tree Species Diversity or Species Identity the More Important Driver of Soil Carbon Stocks, C/N Ratio, and pH? Ecosystems 19, 645–660. doi: 10.1007/s10021-016-9958-1
Dignac, M. F., Derrien, D., Barré, P., Barot, S., Cécillon, L., Chenu, C., et al. (2017). Increasing soil carbon storage: mechanisms, effects of agricultural practices and proxies. A review. Agron. Sustain. Dev. 37:14. doi: 10.1007/s13593-017-0421-2
Dungait, J. A. J., Hopkins, D. W., Gregory, A. S., and Whitmore, A. P. (2012). Soil organic matter turnover is governed by accessibility not recalcitrance. Glob. Chang. Biol. 18, 1781–1796. doi: 10.1111/j.1365-2486.2012.02665.x
Dynarski, K. A., Bossio, D. A., and Scow, K. M. (2020). Dynamic Stability of Soil Carbon: reassessing the “Permanence” of Soil Carbon Sequestration. Front. Environ. Sci. 8:514701. doi: 10.3389/fenvs.2020.514701
Eclesia, R. P., Jobbagy, E. G., Jackson, R. B., Biganzoli, F., and Piñeiro, G. (2012). Shifts in soil organic carbon for plantation and pasture establishment in native forests and grasslands of South America. Glob. Chang. Biol. 18, 3237–3251. doi: 10.1111/j.1365-2486.2012.02761.x
Elliot, E. (1986). Aggregate structure and carbon, nitrogen, and phosphorus in native and cultivated soils. Soil Sci. Soc. Am. J. 50, 627–633.
Environment Canada (2020). Canadian Climate Normals or Averages, 1981-2010, for Fort McMurray, Alberta. Available online at: www.climate.weather.gc.ca (accessed December 20, 2020).
Finér, L., Helmisaari, H. S., Lõhmus, K., Majdi, H., Brunner, I., Børja, I., et al. (2007). Variation in fine root biomass of three European tree species: beech (Fagus sylvatica L.), Norway spruce (Picea abies L. Karst.), and Scots pine (Pinus sylvestris L.). Plant Biosyst. 141, 394–405. doi: 10.1080/11263500701625897
Frouz, J., Dvorščík, P., Vindušková, O., and Cienciala, E. (2013). “Plant Production, Carbon Accumulation and Soil Chemistry at Post-Mining Sites,” in Soil Biota and Ecosystem Development in Post Mining Sites, ed. J. Frouz (Boca Raton: CRC Press), 89–103. doi: 10.1201/b15502-7
Frouz, J., Elhottová, D., Kuráž, V., and Šourková, M. (2006). Effects of soil macrofauna on other soil biota and soil formation in reclaimed and unreclaimed post mining sites: results of a field microcosm experiment. Appl. Soil Ecol. 33, 308–320. doi: 10.1016/j.apsoil.2005.11.001
Frouz, J., Pižl, V., Cienciala, E., and Kalčík, J. (2009). Carbon storage in post-mining forest soil, the role of tree biomass and soil bioturbation. Biogeochemistry 94, 111–121. doi: 10.1007/s10533-009-9313-0
Gregorich, E. G., Beare, M. H., McKim, U. F., and Skjemstad, J. O. (2006). Chemical and biological characteristics of physically uncomplexed organic matter. Soil Sci. Soc. Am. J. 70, 975–985. doi: 10.2136/sssaj2005.0116
Guidi, C., Magid, J., Rodeghiero, M., Gianelle, D., and Vesterdal, L. (2014). Effects of forest expansion on mountain grassland: changes within soil organic carbon fractions. Plant Soil 385, 373–387. doi: 10.1007/s11104-014-2315-2
Guo, L. B., and Gifford, R. M. (2002). Soil carbon stocks and land use change: a meta analysis. Glob. Chang. Biol. 8, 345–360. doi: 10.1046/j.1354-1013.2002.00486.x
Jastrow, J. D., Boutton, T. W., and Miller, R. M. (1996). Division s-3-soil biology & biochemistry. Soil Sci. Am. J. 60, 801–807.
Jerabkova, L., Prescott, C. E., and Kishchuk, B. E. (2006). Nitrogen availability in soil and forest floor of contrasting types of boreal mixedwood forests. Can. J. For. Res. 36, 112–122. doi: 10.1139/x05-220
Józefowska, A., Woś, B., and Pietrzykowski, M. (2016). Tree species and soil substrate effects on soil biota during early soil forming stages at afforested mine sites. Appl. Soil Ecol. 102, 70–79. doi: 10.1016/j.apsoil.2016.02.012
Kleber, M., Nico, P. S., Plante, A., Filley, T., Kramer, M., Swanston, C., et al. (2011). Old and stable soil organic matter is not necessarily chemically recalcitrant: implications for modeling concepts and temperature sensitivity. Glob. Chang. Biol. 17, 1097–1107. doi: 10.1111/j.1365-2486.2010.02278.x
Laganière, J., Angers, D. A., and Paré, D. (2010). Carbon accumulation in agricultural soils after afforestation: a meta-analysis. Glob. Chang. Biol. 16, 439–453. doi: 10.1111/j.1365-2486.2009.01930.x
Laganière, J., Angers, D. A., Paré, D., Bergeron, Y., and Chen, H. Y. H. (2011). Black spruce soils accumulate more uncomplexed organic matter than aspen soils. Soil Sci. Soc. Am. J. 75, 1125–1132. doi: 10.2136/sssaj2010.0275
Lajtha, K., Townsend, K. L., Kramer, M. G., Swanston, C., Bowden, R. D., and Nadelhoffer, K. (2014). Changes to particulate versus mineral-associated soil carbon after 50 years of litter manipulation in forest and prairie experimental ecosystems. Biogeochemistry 119, 341–360. doi: 10.1007/s10533-014-9970-5
Lal, R., Smith, P., Jungkunst, H. F., Mitsch, W. J., Lehmann, J., Ramachandran Nair, P. K., et al. (2018). The carbon sequestration potential of terrestrial ecosystems. J. Soil Water Conserv. 73, 145A–152A. doi: 10.2489/jswc.73.6.145A
Lützow, M. V., Kögel-Knabner, I., Ekschmitt, K., Matzner, E., Guggenberger, G., Marschner, B., et al. (2006). Stabilization of organic matter in temperate soils: mechanisms and their relevance under different soil conditions - A review. Eur. J. Soil Sci. 57, 426–445. doi: 10.1111/j.1365-2389.2006.00809.x
Masse, J., Prescott, C. E., Renaut, S., Terrat, Y., and Grayston, S. J. (2017). Plant community and nitrogen deposition as drivers of alpha and beta diversities of prokaryotes in reconstructed oil sand soils and natural boreal forest soils. Appl. Environ. Microbiol. 83:e03319-16. doi: 10.1128/AEM.03319-16
Oades, J. M. (1984). Soil organic matter and structural stability: mechanisms and implications for management. Plant Soil 76, 319–337. doi: 10.1007/BF02205590
Oksanen, J., Guillaume Blanchet, F., Friendly, M., Kindt, R., Legendre, P., McGlinn, D., et al. (2020). vegan: Community Ecology Package.
Page-Dumroese, D. S., Brown, R. E., Jurgensen, M. F., and Mroz, G. D. (1999). Comparison of methods for determining bulk densities of rocky forest soils. Soil Sci. Soc. Am. J. 63, 379–383. doi: 10.2136/sssaj1999.03615995006300020016x
Peng, Y., Schmidt, I. K., Zheng, H., Hedìnec, P., Bachega, L. R., Yue, K., et al. (2020). Tree species effects on topsoil carbon stock and concentration are mediated by tree species type, mycorrhizal association, and N-fixing ability at the global scale. For. Ecol. Manag. 478:118510. doi: 10.1016/j.foreco.2020.118510
Phillips, R. P., Brzostek, E., and Midgley, M. G. (2013). The mycorrhizal-associated nutrient economy: a new framework for predicting carbon–nutrient couplings in temperate forests. New Phytol. 199, 41–51. doi: 10.1111/nph.12221
Poeplau, C., Don, A., Vesterdal, L., Leifeld, J., Van Wesemael, B., Schumacher, J., et al. (2011). Temporal dynamics of soil organic carbon after land-use change in the temperate zone - carbon response functions as a model approach. Glob. Chang. Biol. 17, 2415–2427. doi: 10.1111/j.1365-2486.2011.02408.x
Policelli, N., Horton, T. R., Hudon, A. T., Patterson, T. R., and Bhatnagar, J. M. (2020). Back to Roots: the Role of Ectomycorrhizal Fungi in Boreal and Temperate Forest Restoration. Front. For. Glob. Chang. 3:97. doi: 10.3389/ffgc.2020.00097
Prescott, C., Frouz, J., Grayston, S. J., Quideau, S. A., and Straker, J. (2019). “Chapter 13: Rehabilitating forest soils after disturbance,” in Global Change and Forest Soils: cultivating stewardship of a finite natural resource, eds M. Busse, C. P. Giardina, D. M. Morris, and D. S. Page-Dumroese (Amsterdam: Elsevier Inc), 544.
Prescott, C. E., Vesterdal, L., Preston, C. M., and Simard, S. W. (2004). Influence of initial chemistry on decomposition of foliar litter in contrasting forest types in British Columbia. Can. J. For. Res. 34, 1714–1729. doi: 10.1139/X04-040
Prescott, C. E., Zabek, L. M., Staley, C. L., and Kabzems, R. (2000). Decomposition of broadleaf and needle litter in forests of British columbia: influences of litter type, forest type, and litter mixtures. Can. J. For. Res. 30, 1742–1750. doi: 10.1139/x00-097
R Core Team (2020). R: A Language and Environment for Statistical Computing. Vienna: R foundation for Statistical Computing.
Rabenhorst, M. C. (1988). Determination of organic and carbonate carbon in calcareous soils using dry combustion. Soil Sci. Soc. Am. J. 52, 965–968. doi: 10.2136/sssaj1988.03615995005200040012x
Reich, P. B., Oleksyn, J., Modrzynski, J., Mrozinski, P., Hobbie, S. E., Eissenstat, D. M., et al. (2005). Linking litter calcium, earthworms and soil properties: a common garden test with 14 tree species. Ecol. Lett. 8, 811–818. doi: 10.1111/j.1461-0248.2005.00779.x
Rillig, M. C. (2004). Arbuscular mycorrhizae, glomalin, and soil aggregation. Can. J. Soil Sci. 84, 355–363. doi: 10.4141/S04-003
Scharlemann, J. P. W., Tanner, E. V. J., Hiederer, R., and Kapos, V. (2014). Global soil carbon: understanding and managing the largest terrestrial carbon pool. Carbon Manag. 5, 81–91. doi: 10.4155/cmt.13.77
Six, J., Bossuyt, H., Degryze, S., and Denef, K. (2004). A history of research on the link between (micro)aggregates, soil biota, and soil organic matter dynamics. Soil Tillage Res. 79, 7–31. doi: 10.1016/j.still.2004.03.008
Six, J., Feller, C., Denef, K., Ogle, S., de Moraes, J., and Albrecht, A. (2002). Soil organic matter, biota and aggregation in temperate and tropical soils – effects of no-tillage. Agronomie 22, 755–775. doi: 10.1051/agro
Six, J., Paustian, K., Elliott, E. T., and Combrink, C. (2000). Soil structure and organic matter I. Distribution of aggregate-size classes and aggregate-associated carbon. Soil Sci. Soc. Am. J. 64, 681–689. doi: 10.2136/sssaj2000.642681x
Soil Classification Working Group (1998). The Canadian System of Soil Classification, 3rd Edn. Ottawa, ON: NRC Research Press.
Soucémarianadin, L. N., Cécillon, L., Guenet, B., Chenu, C., Baudin, F., Nicolas, M., et al. (2018). Environmental factors controlling soil organic carbon stability in French forest soils. Plant Soil 426, 267–286. doi: 10.1007/s11104-018-3613-x
Tate, K. R., Ross, D. J., and Feltham, C. W. (1988). A direct extraction method to estimate soil microbial c: effects of experimental variables and some different calibration procedures. Soil Biol. Biochem. 20, 329–335. doi: 10.1016/0038-0717(88)90013-2
Turcotte, I., Quideau, S. A., and Oh, S. W. (2009). Organic matter quality in reclaimed boreal forest soils following oil sands mining. Org. Geochem. 40, 510–519. doi: 10.1016/j.orggeochem.2009.01.003
Vindušková, O., and Frouz, J. (2013). Soil carbon accumulation after open-cast coal and oil shale mining in Northern Hemisphere: a quantitative review. Environ. Earth Sci. 69, 1685–1698. doi: 10.1007/s12665-012-2004-5
Visser, S., Maynard, D., and Danielson, R. M. (1998). Response of ecto- and arbuscular mycorrhizal fungi to clear-cutting and the application of chipped aspen wood in a mixedwood site in Alberta, Canada. Appl. Soil Ecol. 7, 257–269. doi: 10.1016/S0929-1393(97)00060-7
Wei, J., Cheng, J., Li, W., and Liu, W. (2012). Comparing the effect of naturally restored forest and grassland on carbon sequestration and its vertical distribution in the Chinese Loess Plateau. PLoS One 7:e40123. doi: 10.1371/journal.pone.0040123
Wiesmeier, M., Urbanski, L., Hobley, E., Lang, B., von Lützow, M., Marin-Spiotta, E., et al. (2019). Soil organic carbon storage as a key function of soils - A review of drivers and indicators at various scales. Geoderma 333, 149–162. doi: 10.1016/j.geoderma.2018.07.026
Keywords: soil organic carbon, reclamation, aggregates, Populus, MAOM, Alberta oil sands, hPOM, fPOM
Citation: Nickels MCL and Prescott CE (2021) Soil Carbon Stabilization Under Coniferous, Deciduous and Grass Vegetation in Post-mining Reclaimed Ecosystems. Front. For. Glob. Change 4:689594. doi: 10.3389/ffgc.2021.689594
Received: 01 April 2021; Accepted: 24 August 2021;
Published: 16 September 2021.
Edited by:
Sandra Spielvogel, Christian-Albrecht University of Kiel, GermanyReviewed by:
Antra Boca, Latvia University of Agriculture, LatviaMichaela Anna Dippold, Georg August University of Göttingen, Germany
Copyright © 2021 Nickels and Prescott. This is an open-access article distributed under the terms of the Creative Commons Attribution License (CC BY). The use, distribution or reproduction in other forums is permitted, provided the original author(s) and the copyright owner(s) are credited and that the original publication in this journal is cited, in accordance with accepted academic practice. No use, distribution or reproduction is permitted which does not comply with these terms.
*Correspondence: Meghan C. L. Nickels, bGFpZGxhdy5tZWdoYW5AZ21haWwuY29t
†These authors have contributed equally to this work