- 1Department of Earth and Environmental Sciences, KU Leuven, Leuven, Belgium
- 2Department of Ecosystem and Landscape Dynamics, Institute for Biodiversity and Ecosystem Dynamics, University of Amsterdam, Amsterdam, Netherlands
- 3Department of Geosciences and Natural Resource Management, University of Copenhagen, Frederiksberg, Denmark
Despite the general agreement that maximizing carbon storage and its persistence in forest soils are top priorities in the context of climate change mitigation, our knowledge on how to steer soil organic carbon (SOC) through forest management remains limited. For some soils, tree species selection based on litter quality has been shown a powerful measure to boost SOC stocks and stability, whereas on other locations similar efforts result in insignificant or even opposite effects. A better understanding of which mechanisms underpin such context-dependency is needed in order to focus and prioritize management efforts for carbon sequestration. Here we discuss the key role of acid buffering mechanisms in belowground ecosystem functioning and how threshold behavior in soil pH mediates tree species effects on carbon cycling. For most forests around the world, the threshold between the exchange buffer and the aluminum buffer around a pH-H2O of 4.5 is of particular relevance. When a shift between these buffer domains occurs, it triggers changes in multiple compartments in the soil, ultimately altering the way carbon is incorporated and transformed. Moreover, the impact of such a shift can be amplified by feedback loops between tree species, soil biota and cation exchange capacity (CEC). Hence, taking into account non-linearities related to acidity will allow more accurate predictions on the size and direction of the effect of litter quality changes on the way soil organic carbon is stored in forest soils. Consequently, this will allow developing more efficient, context-explicit management strategies to optimize SOC stocks and their stability.
Introduction
The potential of forests, and particularly the soils beneath them, in combating climate change is increasingly recognized in science (Bastin et al., 2019), policy (European Commission [EC], 2019), and management (Mayer et al., 2020). Boosting soil organic matter (SOC) in forest soils not only mitigates the continued increase of atmospheric CO2 concentrations, but also provides associated benefits, including increased soil fertility, productivity, water holding capacity and therefore better adaptation in the face of climate change (Tiessen et al., 1994; Minasny et al., 2017). Tree species selection has been identified as a promising avenue to steer carbon cycling by the forester (Prescott and Vesterdal, 2013). A considerable body of literature has addressed the link between overstory tree species and functioning of the forest soil, in terms of productivity, nutrient availability, biological activity and soil carbon cycling (Finzi et al., 1998; Augusto et al., 2002; Reich et al., 2005; Mueller et al., 2015; Schelfhout et al., 2017). However, the reported effects of litter quality on belowground functioning often vary in magnitude or even in direction (Ehrenfeld et al., 2005). Accordingly many papers conclude that litter effects are site- or context-dependent (Eviner and Hawkes, 2008; Kooijman and Martinez-Hernandez, 2009). To reach the full potential that is predicted in terms of boosting soil carbon stocks via forest management, it is therefore crucial to understand what drives this context-dependency (Jandl et al., 2007; Jackson et al., 2017; Solly et al., 2020). Or, as Prescott and Vesterdal, 2013 emphasize, the question is no longer “what is the effect?”, but “under which conditions is this effect happening”?
Context-dependency was anchored in soil carbon research by Schmidt et al. (2011), who argued that carbon persistence in soils is an ecosystem property. Lehmann et al. (2020) moreover highlighted the role of substrate complexity in soil carbon cycles, but the nexus between litter quality, the edaphic context and soil carbon decomposition and stabilization to this date remains poorly explored. Previous studies have already indicated the role of soil pH (Vedy, 1973; Beck et al., 1969; Gurmesa et al., 2013; Solly et al., 2020), texture (Vesterdal and Raulund-Rasmussen, 1998; Angst et al., 2018b; Desie et al., 2020c), cation exchange capacity (CEC) (Rasmussen et al., 2018; Desie et al., 2019), soil type (Kögel-Knabner and Amelung, 2021), and parent material (Heckman et al., 2009; Angst et al., 2018a) on various aspects of soil organic matter dynamics, but integration of these variables into an overall conceptual model remains elusive. Moreover, a complex system approach to ecosystem science (sensu Messier et al., 2013) is needed to recognize tipping points or non-linearity in a system’s behavior. Indeed, non-linearity has been identified as a key feature of ecosystems (Scheffer et al., 2001) and accordingly it is important in the success (or failure) of forest ecosystems as measures for global climate change mitigation.
In this concept letter, we explore the importance of a well-described pedogenic threshold in soil acidity at a pH-H2O of ca. 4.5 and a base saturation (BS) of 30% for soil carbon = processes (BOX1). This threshold is known as the limit between the exchange buffer range (“acidic” or mesotrophic domain) and the aluminum buffer range (“acid” or oligotrophic domain) (Ulrich, 1991). Literature agrees that a shift from the exchange to the aluminum domain can result in multiple shifts in belowground functioning (Ulrich, 1991; Ponge, 2013). In this letter, we discuss how a change in tree species composition can trigger such a shift in forest floor and soil functioning and argue how that must ultimately also affect carbon dynamics in the soil, particularly the quantity, composition and stability of forest soil carbon can be affected. A change in overstory species composition has multiple effects on belowground ecosystem functioning, including a potential change in (leaf/root) litter input quantity and quality (Guo et al., 2005; Mueller et al., 2015), rooting patterns (Spielvogel et al., 2014; Cremer et al., 2016), microclimate (Joly et al., 2017), associated mycorrhiza (Heděnec et al., 2020), and etc. Here, we focus on the impact of leaf litter quality which has been identified as a strong driver of soil pH (van Breemen et al., 1997). Other indirect tree species effects were not in the scope of this concept letter, although remain key for understanding plant-soil interactions. Secondly, soil buffer mechanisms and ecological feedbacks can mitigate or amplify shifts in belowground functioning and thereby explain context dependent litter effects. This would also imply that there is a “window of opportunity” to steer carbon sequestration outcomes via the selection of tree species based on litter quality. We will discuss (i) how leaf litter-induced acidification can cause rapid changes in forest soils; (ii) how these changes in belowground functioning affect processes relevant to carbon cycling; and (iii) how context-dependency leads to a “window of opportunity” for steering carbon cycling through selection of tree species.
When Tree Species Acidify the Soil, That Cascades Through Belowground Functioning
A change in overstory species composition that results in a decrease in litter quality, i.e., either characterized by a decrease in base cation content or an increase in C/N ratio, lignin, and cutin content (Cornwell et al., 2008), can lead to a litter-induced shift between the exchange and aluminum soil process domain and trigger sudden changes in soil geochemistry thereby altering the composition, diversity and functioning of biotic communities (Figure 1).
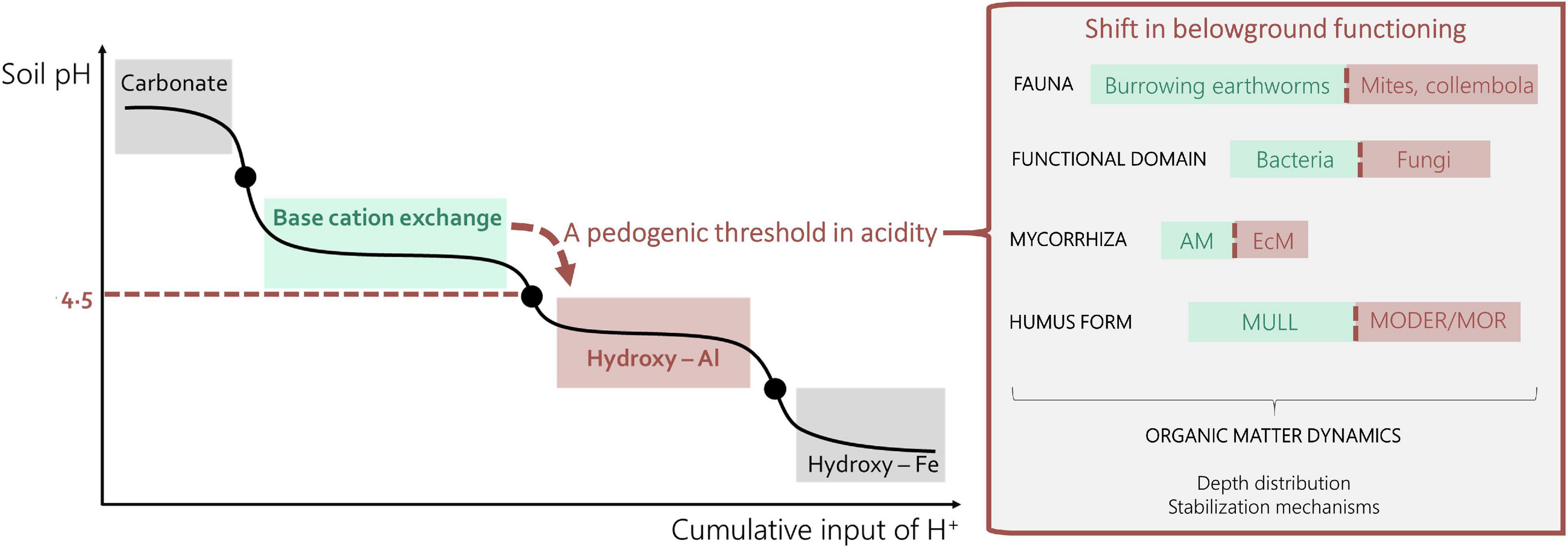
Figure 1. Left: Soil pH-H2O is buffered by several subsequent mechanisms. These soil process domains are interchanged by pedogenic thresholds (BOX1). Right: A shift from the exchange domain to the aluminum domain cascades through belowground functioning and ultimately affects SOC dynamics.
The composition of meso- and macrofaunal communities is one of the first biotic compartments to react. The activity of burrowing macro-fauna such as endogeic and anecic earthworm species is reduced below a pH of ca. 4.5 (De Wandeler et al., 2016; Schelfhout et al., 2017) with the exception of acid tolerant ants and termites (Taylor et al., 2019). Smaller mesofauna such as enchytraeids, collembola and mites become more dominant (Briones, 2014; Korboulewsky et al., 2016), leading to reduced soil bioturbation and a vertical decoupling of organic matter and nutrient cycles in the soil (Muys et al., 1992; Ponge et al., 2010). This provokes a clear spatial disconnection between microbial communities in the litter layer and underlying mineral soil layers: most of the microbial catabolic potential is concentrated in the litter layer when the soil further acidifies (Desie et al., 2019). Not only the location of dominance, but also the species composition of decomposers shifts below a pH of ca. 4.5: fungi become dominant over bacteria (Blagodatskaya and Anderson, 1998; Phillips et al., 2013; Heděnec et al., 2020) and the diversity of bacterial communities decreases (Fierer and Jackson, 2006). This also leads to more pronounced substrate-decomposer interactions (Palozzi and Lindo, 2018), e.g., there is an increased cooperation between plants and nutrient mining symbionts, which are often species specific (Eastwood et al., 2011). Corresponding with aboveground changes in species composition, the mycorrhizal associations may change: Ectomycorrhiza (ECM) are typically more related to tree species with low quality litter and more acidic conditions whereas arbuscular mycorrhiza (AM) depend on saprotrophic microbes for nutrient release and are therefore associated with base cation-rich, fast decaying litter and higher soil pH (that allow bacterial communities to thrive) (Phillips et al., 2013; Heděnec et al., 2020; Peng et al., 2020). Overall, when the soil shifts to the Al buffer domain, ecological strategies focus more on mitigating nutrient limitations (Wardle et al., 2004; Ribbons et al., 2018) mirrored by a more “organic based nutrient economy” instead of a mineral nutrient economy (Lin et al., 2017). Moreover, the aboveground shift in tree species can also correspond with changes in canopy and rooting characteristics that further impact the belowground ecosystem and amplify or mitigate other litter effects (Spielvogel et al., 2014; Joly et al., 2017).
BOX 1. Pedogenic thresholds in soil acidity.
Acidity has long been recognized as a key factor of belowground forest ecosystem functioning (Ulrich, 1991), linked to differences in the biotic compartment for a range of soils (Ponge, 2003). Moreover, it is one of the best described edaphic properties prone to tipping points in soil geochemistry (Ulrich, 1987) and thereby a good example of non-linearity in belowground ecosystem functioning. Most of the time, a temporary increase in H+ ion production or exogenic input has little or no effect, as these protons are neutralized by a range of soil buffer mechanisms. These include dissolution of carbonates between pH-H2O 8.6 and 6.2, weathering of silicates between pH-H2O 6.2 and 5.0, mainly exchange of base cations between pH-H2O 5.0 and 4.5 and Al-hydroxides below pH-H2O ca. 4.5 (Ulrich, 1991). When a certain buffer is exhausted, pH drops suddenly in response to a small additional increase in acidity. These steep intervals in soil pH response curve are termed pedogenic thresholds (Chadwick and Chorover, 2001). As most forests around the world are characterized by low pH values (Supplementary Figure 1), many of them might be very close to the threshold value of 4.5 (Slessarev et al., 2016). Hence, understanding the effect of overstory species around that threshold is of particular relevance for forest carbon cycles around the globe.
… and Ultimately Affects SOC Dynamics
Both the input of low quality litter and the changed environmental conditions (low pH, high Al, low base cations availability, in addition to microclimatic conditions) change the community of decomposers leading to a slower decomposition pathway and the accumulation of organic matter on the soil surface (Blagodatskaya and Anderson, 1998). Therefore, we argue that this abiotic shift in soil process domain and the following chain in biotic reactions eventually translates in a change in soil carbon dynamics, i.e., a change in the vertical distribution of SOC, its chemical composition and its stability.
The most visible and widely studied result of all above changes in faunal and microbial life in the soil is a change in the forest floor or litter layer. Most studies agree that forest floors become thicker when litter quality decreases (Toutain, 1981). Moreover the litter layer also changes functionally with a shift in soil process domain: when pH drops below 5, moder and mor humus forms become dominant and mull humus forms no longer occur (Ponge et al., 2010), indicating that humus form would already be a good indicator of carbon cycling (Andreetta et al., 2011).
The accumulation on top of the soil profile and the reduced activity of bioturbators leads to a different vertical distribution of carbon within in the soil profile as a consequence of a shift in soil process domain (Vesterdal et al., 2013). In accordance with the accumulation in the forest floor, many studies report an increase in topsoil total carbon stocks with a change to lower litter quality (Vesterdal et al., 2008; Boča et al., 2014; Augusto et al., 2015). For deeper soil layers, the results are less clear, yet some studies report a decrease in subsoil carbon stocks, although more subtle, as bioturbation and organic matter incorporation are inhibited (Oostra et al., 2006; Mareschal et al., 2010; Frouz et al., 2013). Subsoil carbon stocks are probably relatively more influenced by other tree species effects (e.g., rooting patterns and root litter and exudates) and soil texture (vertical migration of DOC) (Rumpel and Kögel-Knabner, 2011).
Moreover, the change in decomposer community causes a change in the chemical composition as lignin is favorably decomposed by a dominantly fungal community (Vancampenhout et al., 2009; Brock et al., 2019). Concurrently, interactions between different types of organic molecules in the soil may also change. Typically, the addition of high-quality litter leads to increased decomposition of low-quality litter in non-acid soils (positive priming), while negative apparent priming is observed in acid systems (the most advantageous food source is processed first) (Heitkötter et al., 2017; Zhou et al., 2021).
Finally, also the stability of stored carbon is altered when the pH drops below ca. 4.5. First of all, the vertical distribution of carbon will affect its persistence as carbon in the forest floor and topsoil layers is more exposed to environmental disturbances, such as increased microbial decomposition because of higher soil temperatures in canopy gaps or direct oxidation by fire (von Lützow et al., 2006; Jandl et al., 2007). Secondly, we argue that with this change in biochemical conditions and the players present in the process, also the stabilization mechanisms within the mineral soil are altered. Microbial activity is inhibited by low pH values and thus there is reduced microbial entombing for SOC stabilization (Cotrufo et al., 2013; Liang et al., 2017; Angst et al., 2021). The absence of burrowing macro- and mesofauna moreover leads to reduced aggregate stabilization and reduced organo-mineral interactions (Briones, 2014), resulting in less carbon stored in mineral associated fractions and more in large particulate organic matter (POM) (Laganière et al., 2011; Angst et al., 2018b; Desie et al., 2019; Giannetta et al., 2019). Chemical recalcitrance and metal-humus complexation become the main SOC stabilization mechanisms at play (Heckman et al., 2009; Clarholm and Skyllberg, 2013). Corresponding with this, Hobbie et al. (2007) reported greater impact of acidic hydrolyzing cations (Al and Fe) on the exchange complex for carbon stabilization in mineral soil layers via cation bridging and flocculation and indirectly by inhibiting microbial activity (due to the low pH and high Al). Hence, organic matter decomposition becomes nutrient driven rather than energy driven (Grime et al., 1988; Camenzind et al., 2018; Wiesmeier et al., 2019). Altogether, these studies illustrate that with a change in soil process domain (when the pH drops below ca. 4.5) the carbon cycle is altered. Although carbon sequestration by accumulation in the forest floor may increase, the mechanisms controlling SOC stabilization shift as well and the stability of the carbon stored in the soil decreases.
Still, the impact of a pedogenic threshold in terms of total carbon stocks is less clear: studies reporting differences in total carbon over the entire soil profile are inconsistent (Mayer et al., 2020). This can partly be explained by the longer timespan needed to alter total carbon stocks: decomposer communities react fast to changing conditions thereby more quickly resulting in changes in the forest floor and stabilization mechanisms, whereas in terms of total carbon stocks, legacies can mask the effect of changed conditions for a relative long time. That could also explain why the impact of litter quality on mineral soil carbon stocks is more pronounced in recently reclaimed mining sites, where legacy effects are small (Frouz et al., 2013). Also, carbon saturation and/or differences in root litter carbon input (that are relatively more pronounced in the mineral soil) may explain the absence of clear trends in total mineral soil carbon stocks per soil process domain. Finally, only few studies follow and integrate the continuum from forest floors to deep mineral soil layers (Thomas et al., 2021).
Incorporating the impact of acid buffering explicitly into soil carbon research could provide more insight in the mechanisms controlling organic matter cycles, including the effect on total mineral carbon stocks, and how to steer it by management. Along that line, Schmidt et al. (2011) emphasized that the way carbon is processed (and its persistence) does not merely depend on intrinsic properties of the input (e.g., the litter quality), but is to a great extent determined by the surrounding environment, i.e., it can be considered an ecosystem property. This corroborates findings of Duchaufour (1990) and Raulund-Rasmussen and Vejre (1995) that already stressed the important influence of the environment and edaphic factors on carbon cycling. In forest ecosystems in particular, the pervasive role of acidity is long recognized (Beck et al., 1969; Ulrich, 1991) and broadly applicable (Figure 1; Supplementary Figure 1; Slessarev et al., 2016). However, based on existing literature, it is hard to evaluate effects of tree species on soil carbon relative to the soil process domain they create, as few studies provide data on texture, soil pH and base saturation per tree species (or per reported effect). Also, the myriad of methods and setups to evaluate tree species effects makes meta-analysis challenging. Future studies of tree species effects on SOC should clearly describe and parameterize the context (including the dominating soil process domain) and evaluate tree species effects relative to this context. In that regard, reporting the soil type as a resulting structure of the processes at play might once again prove valuable (De Vos et al., 2015; Kögel-Knabner and Amelung, 2021). Nevertheless, it remains a challenge to find set-ups, e.g., multisite common garden experiments, that allow to evaluate tree species and context independently (Vesterdal et al., 2013).
A Window of Opportunity for Management
If and when a change in litter quality can induce the above described shift in soil process domain and the corresponding cascade in belowground functioning, depends on intrinsic soil properties and is simultaneously mitigated by mechanisms of mineral and biological nature (Ulrich, 1987; Ponge, 2013). Combined, these mechanisms underpin the context-dependency of litter effects on carbon cycling.
First, we discuss how the mechanism of mineral nature causes context-dependency and leads to a window of opportunity for management. As indicated by Slessarev et al. (2016), all climatic zones that are suitable for forest growth, typically characterized by a precipitation surplus that induces leaching of nutrients, move naturally toward the threshold between the exchange and the aluminum domain (Figure 1 and Supplementary Figure 1). In this range, the shape of soil response curves to increased acidity essentially depend on two factors: (i) the total amount of negative charge on the exchange complex (i.e., CEC), and (ii) the relative proportion of basic cations (Ca2+, Mg2+, K+, or Na+) on that exchange complex that have already been replaced by H+ or Al3+ (i.e., the base saturation or, inverted, the exchangeable acidity) (Ulrich, 1991; Blume et al., 2016).
In soils with a very low CEC, e.g., extremely sandy soils low in organic matter or carbonates, there is very little exchange buffer capacity and pH will not exhibit strong threshold behavior because of the variable charge, which is dependent on the acid strength of specific organic matter functional groups (Schwertmann et al., 1987). The response curve therefore approaches an inverse logarithmic relation (Figure 2A). In these forest soils the potential to alter carbon processing via tree species selection is rather limited. Note that in this concept we assume CEC to remain constant over the entire soil profile. In practice, however, there are situations where CEC and BS vary with depth, which results in more complex results; e.g., in acidified topsoil with low CEC (Figure 2A), tree species can still induce a shift if base cations can be taken up from deeper unaffected layers. This added complexity when integrating depth was not included in our concept, however, it remains key in the understanding of belowground functioning and carbon cycles (Rumpel and Kögel-Knabner, 2011).
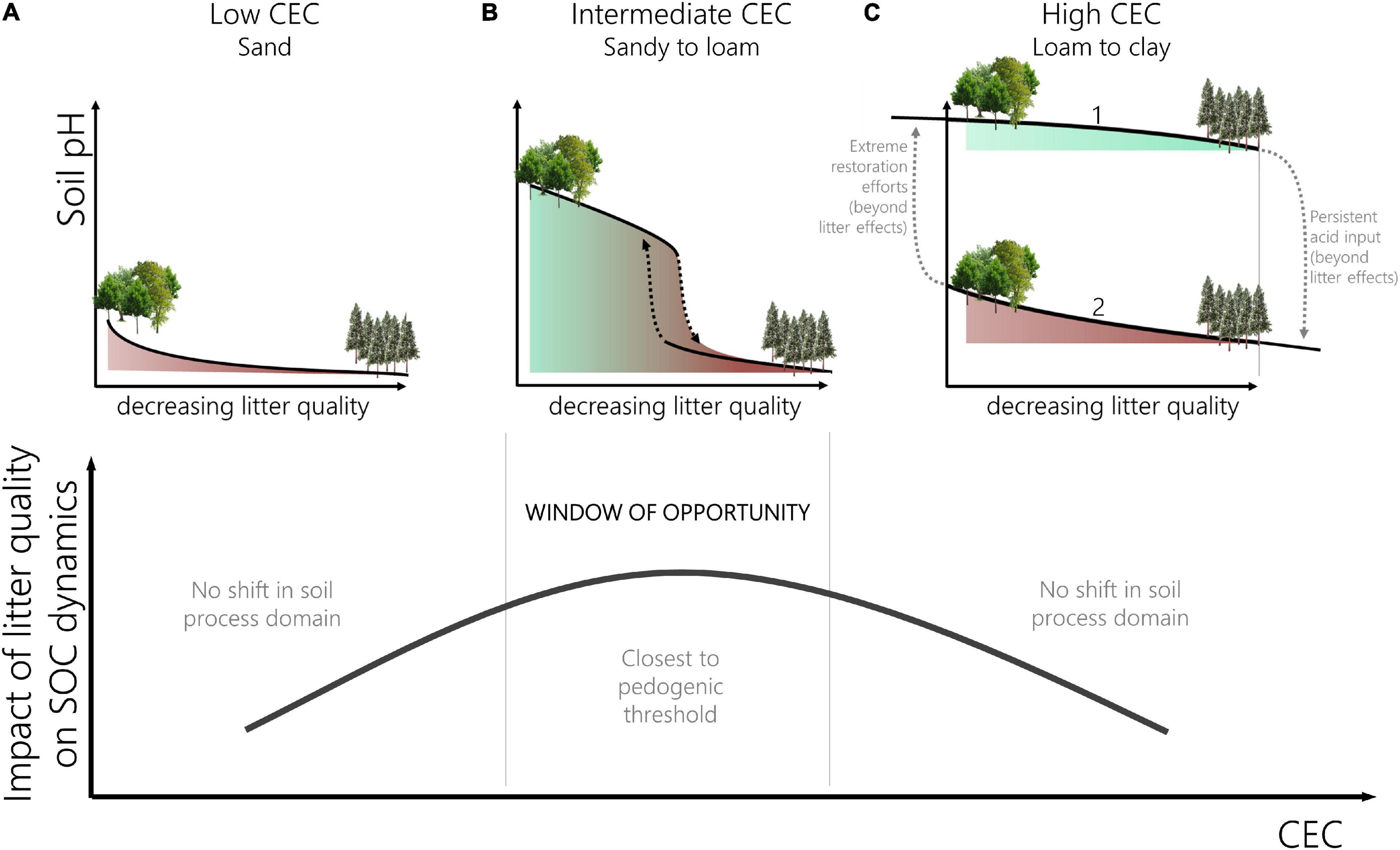
Figure 2. Top: the response curve of soil pH to a change in litter quality (e.g., the input of acidity) differs depending on the context [here cation exchange capacity (CEC)]. The response can be reverse-exponential (A), show threshold behavior (B) or indicate two alternative stable states (C). A distinction can be made for the latter: soils with high CEC and high base saturation (BS) (C1) remain in the base exchange domain upon a decrease in litter quality whereas soils with high CEC and low BS (C2) remain in the aluminum domain even with rich litter input. Note that the CEC is assumed constant over the entire profile whereas in practice variability in CEC with depth can occur, which would lead to increased complexity. Bottom: the closer to a pedogenic threshold the higher the impact of tree species litter quality on SOC dynamics indicating a window of opportunity where management efforts to optimize carbon sequestration are most efficient.
If the CEC buffer is larger, the replacement of basic cations by H+ or Al3+ will initially keep the pH almost constant upon addition of low quality litter (via tree species selection). When most of the base cations have been replaced (typically when the base saturation drops below 30%), the curve shows a clear threshold behavior, i.e., when the ecosystem shifts from the exchange domain to the aluminum domain (Figure 2B). In these zones the choice for a certain tree species can have high consequences in terms of acidity and carbon cycling.
Finally, if the CEC is very high (this limit was set at 24meq/100 g clay for agricultural soils (Driessen et al., 2001)), the response curve can fold back upon itself (sensu Scheffer et al., 2001; Figure 2C). This is when Al3+ saturation will induce increasing hysteresis as a consequence of the stronger sorption of Al on the CEC (Bolt and Bruggenwert, 1978). In this last case, the impact of changing litter quality – solely – is insufficient to shift the soil process domain and affect carbon processes. A high CEC is therefore a mitigating property as long as the exchange buffer capacity has not been exhausted, but will become an amplifying factor once the system has tipped to the acid domain (Verstraeten et al., 2018; Desie et al., 2019). Translated to practice, Figure 2C illustrates that in some cases (soils with high CEC) it is crucial to avoid a shift in soil process domain and management efforts should focus on avoiding degradation because once acidified the potential to affect functioning (and carbon cycling) by litter quality alone is low. Simultaneously, management measures that aim to restore acidified soils or drastically alter the way carbon is processed should focus on soils with intermediate CEC’s, as there the impact is largest (see window of opportunity below).
The potential of exchange capacity to drive context-dependent litter effects has been evidenced in previous research, often proxied by clay context (Angst et al., 2018b) or geology (Heckman et al., 2009). For example, Vesterdal and Raulund-Rasmussen (1998) already suggest larger impact of tree species effects on sandy soils, and also, van Oijen et al. (2005); Ribbons et al. (2018), Verstraeten et al. (2018), report more pronounced tree species effects in intermediate sites. Contrary, in sites that are extremely poor the impact of admixing rich litter (and thus tree species litter quality) remains limited (Desie et al., 2020c). This explains another dimension of context-dependent litter effects: the closer a system is to a pedogenic threshold, the more likely the shift will occur, the greater impact external drivers will have and multiple changes are triggered by only a small change in environmental forcing, e.g., a change in litter quality. In intermediately buffered soils, litter quality, hence, has most impact on the ecosystem state, whereas in soils with high contents of free carbonates the impact of litter quality is limited. This can also be seen in the presence of humus forms (Andreetta et al., 2016): in extremely poor sandy soils even under high quality litter tree species only moder and mor humus forms are found (Gurmesa et al., 2013), whereas on very Ca rich soils, we can even find mulls under oak (Andreetta et al., 2011). In between, there is a zone where tree species ultimately determine the dominant humus form, and the full range can be found: mull, moder, mor (Desie et al., 2020b). In these zones, the impact of litter quality on soil carbon sequestration would also be largest. We therefore argue that there is a window of opportunity where the impact of tree species selection is highest: depending on the edaphic setting, a shift in litter quality as represented by a shift in tree species can be a useful tool for the manager to restore a certain state (Desie et al., 2020c) but may lead to a very undesired situation if a shift to a lower state is induced (Desie et al., 2019). This knowledge can help harmonize management efforts and allows to develop more efficient, context-explicit management strategies to optimize SOC stocks and their stability.
Secondly, there are mechanisms of biological nature that can counteract a shift in soil process domain (Ulrich, 1987; Ponge, 2013). Ponge (2013) argues that humus forms can be seen as ecosystem strategies (e.g., mull the “dissipative pathway” versus mor the “conservative pathway”) acting as basins of attraction to which ecosystems evolve and where plant-soil interactions (including the presence and functionality of the present biotic communities) maintain and even reinforce the state. An example of such a mechanism is the positive feedback loop between burrowing earthworms, humus form and soil pH that maintains the system in its present state: by promoting litter turnover rate, thereby increasing topsoil pH and creating suitable living conditions for themselves, burrowing earthworms promote their own abundance and activity (Desie et al., 2020a). However, as this feedback loop can reinforce litter effects, it can also amplify a negative (or positive) spiral when aboveground litter input changes and the mitigating mechanism again becomes the amplifying mechanism. The impact of such feedbacks is most important at intermediate pH values as this is where small changes induced by a certain species can differentially affect other species and trigger faster changes (Figure 2; Ehrenfeld et al., 2005). For example, the above discussed feedback loop is only active below a pH of 5, underpinning another driver of context-dependent litter effects.
These complex response curves imply that a change in litter quality may or may not have an immediate and strong effect on soil acidity and SOC dynamics: depending on the CEC, the remaining base saturation and the present biotic communities, the increase in acidifying litter may take shorter or longer to push a system over the threshold and alter the way carbon is processed. In the case of an alternative stable states curve (high CEC with high hysteresis, Figure 2C), legacy effects come into play: e.g., reducing the acid load and boosting the base cation input by planting rich litter species will not suffice to remove the large quantities of Al sorbed to the CEC (Desie et al., 2020c) and a shift in belowground carbon processing is not made. Acidity response curves may therefore be an interesting lens to build a conceptual framework for better understanding context-dependency in litter effects on belowground organic matter cycling in forest soils.
Conclusion
In this concept letter, we highlight how threshold behavior in soil acid buffering can drive context-dependency of litter effects on belowground ecosystem functioning to shape organic matter dynamics. Acidity is a key driver of forest ecosystem functioning, with clear non-linear relations between buffer domains. A shift in soil process domain in between the exchange and aluminum buffer domain (around a pH of 4.5), has pervasive impact on multiple compartments of the belowground ecosystem (e.g., abiotic conditions and soil biota communities) and will also change carbon dynamics. Particularly the quantity of carbon stored in the litter layer, the vertical distribution of SOC and its stability respond to a shift in soil buffer domain. The impact on total carbon stocks and SOC persistence will require more research. We suggest that studies of vegetation effects on organic matter dynamics should identify and explicitly discuss the context of the reported effects and parameterize it, if possible, based on key soil properties (e.g., soil texture, CEC, base saturation, exchangeable aluminum in addition to soil pH). Moreover, using acidity as the “lense” to study vegetation effects, i.e., the relative evaluation of soil process domain vs. tree species effects, would provide much needed insight into soil the CEC buffer is lar carbon dynamics. Finally, as a plus, the non-linearity associated with acidity also implies that we can target management efforts to where they will be most effective, i.e., in the window of opportunity where tree species selection has most impact on belowground functioning. This knowledge can help harmonize management efforts to optimize SOC stocks and their stability.
Data Availability Statement
The original contributions presented in the study are included in the article/Supplementary Material, further inquiries can be directed to the corresponding author/s.
Author Contributions
ED, BM, and KV designed the concept. All authors contributed critically to the final manuscript
Conflict of Interest
The authors declare that the research was conducted in the absence of any commercial or financial relationships that could be construed as a potential conflict of interest.
Supplementary Material
The Supplementary Material for this article can be found online at: https://www.frontiersin.org/articles/10.3389/ffgc.2021.679813/full#supplementary-material
Supplementary Figure 1 | Left: world forest cover (FAO, 2010). Right: World soil pH map (IGBP-DIS Soils Data Task).
References
Andreetta, A., Cecchini, G., Bonifacio, E., Comolli, R., Vingiani, S., and Carnicelli, S. (2016). Tree or soil? Factors influencing humus form differentiation in Italian forests. Geoderma 264, 195–204. doi: 10.1016/j.geoderma.2015.11.002
Andreetta, A., Ciampalini, R., Moretti, P., Vingiani, S., Poggio, G., Matteucci, G., et al. (2011). Forest humus forms as potential indicators of soil carbon storage in Mediterranean environments. Biol. Fertil. Soils 47, 31–40. doi: 10.1007/s00374-010-0499-z
Angst, G., Messinger, J., Greiner, M., Häusler, W., Hertel, D., Kirfel, K., et al. (2018a). Soil organic carbon stocks in topsoil and subsoil controlled by parent material, carbon input in the rhizosphere, and microbial-derived compounds. Soil Biol. Biochem. 122, 19–30. doi: 10.1016/j.soilbio.2018.03.026
Angst, G., Mueller, K. E., Eissenstat, D. M., Trumbore, S., Freeman, K. H., Hobbie, S. E., et al. (2018b). Soil organic carbon stability in forests: distinct effects of tree species identity and traits. Glob. Chang. Biol. 25, 1529–1546. doi: 10.1111/gcb.14548
Angst, G., Mueller, K. E., Nierop, K. G. J., and Simpson, M. J. (2021). Plant- or microbial-derived? A review on the molecular composition of stabilized soil organic matter. Soil Biol. Biochem. 156:108189. doi: 10.1016/j.soilbio.2021.108189
Augusto, L., De Schrijver, A., Vesterdal, L., Smolander, A., Prescott, C., and Ranger, J. (2015). Influences of evergreen gymnosperm and deciduous angiosperm tree species on the functioning of temperate and boreal forests. Biol. Rev. 90, 444–466. doi: 10.1111/brv.12119
Augusto, L., Ranger, J., Binkley, D., and Rothe, A. (2002). Impact of several common tree species of European temperate forests on soil fertility. Ann. For. Sci. 59, 233–253. doi: 10.1051/forest:2002020
Bastin, J.-F., Finegold, Y., Garcia, C., Mollicone, D., Rezende, M., Routh, D., et al. (2019). The global tree restoration potential. Science 365, 76–79. doi: 10.1126/science.aax0848
Beck, G., Dommergues, Y., and Van den Driessche, R. (1969). L’effet litière: II. Étude expérimentale du pouvoir inhibiteur des composés hydrosolubles des feuilles et des litières forestières vis-à-vis de la microflore tellurique. Oecol. Plant. 4, 237–266.
Blagodatskaya, E. V., and Anderson, T.-H. (1998). Interactive effects of pH and substrate quality on the fungal-to-bacterial ratio and qCO2 of microbial communities in forest soils. Soil Biol. Biochem. 30, 1269–1274.
Blume, H.-P., Brümmer, G. W., Fleige, H., Horn, R., Kandeler, E., Kögel-Knabner, I., et al. (2016). “Chemical properties and processes,” in Scheffer/SchachtschabelSoil Science, ed. H.-P. Blume (Berlin: Springer), 123–174.
Boča, A., Van Miegroet, H., and Gruselle, M.-C. (2014). Forest overstory effect on soil organic carbon storage: a meta-analysis. Soil Sci. Soc. Am. J. 78, S35–S47. doi: 10.2136/sssaj2013.08.0332nafsc
Bolt, G. H., and Bruggenwert, M. G. M. (1978). Soil Chemistry. Part A: Basic Elements, Second. Edn. Amsterdam: Elsevier Scientific Publishing Company.
Briones, M. J. I. (2014). Soil fauna and soil functions: a jigsaw puzzle. Front. Environ. Sci. 2:7. doi: 10.3389/fenvs.2014.00007
Brock, O., Kooijman, A., Nierop, K. G. J., Muys, B., Vancampenhout, K., and Jansen, B. (2019). Disentangling the effects of parent material and litter input chemistry on molecular soil organic matter composition in converted forests in Western Europe. Org. Geochem. 134, 66–76. doi: 10.1016/J.ORGGEOCHEM.2019.05.006
Camenzind, T., Hättenschwiler, S., Treseder, K. K., Lehmann, A., and Rillig, M. C. (2018). Nutrient limitation of soil microbial processes in tropical forests. Ecol. Monogr. 88, 4–21. doi: 10.1002/ecm.1279
Chadwick, O. A., and Chorover, J. (2001). The chemistry of pedogenic thresholds. Geoderma 100, 321–353. doi: 10.1016/S0016-7061(01)00027-1
Clarholm, M., and Skyllberg, U. (2013). Translocation of metals by trees and fungi regulates pH, soil organic matter turnover and nitrogen availability in acidic forest soils. Soil Biol. Biochem. 63, 142–153. doi: 10.1016/j.soilbio.2013.03.019
Cornwell, W. K., Cornelissen, J. H. C., Amatangelo, K., Dorrepaal, E., Eviner, V. T., Godoy, O., et al. (2008). Plant species traits are the predominant control on litter decomposition rates within biomes worldwide. Ecol. Lett. 11, 1065–1071. doi: 10.1111/j.1461-0248.2008.01219.x
Cotrufo, M. F., Wallenstein, M. D., Boot, C. M., Denef, K., and Paul, E. (2013). The Microbial Efficiency-Matrix Stabilization (MEMS) framework integrates plant litter decomposition with soil organic matter stabilization: do labile plant inputs form stable soil organic matter? Glob. Chang. Biol. 19, 988–995. doi: 10.1111/gcb.12113
Cremer, M., Kern, N. V., and Prietzel, J. (2016). Forest ecology and management soil organic carbon and nitrogen stocks under pure and mixed stands of European beech, Douglas fir and Norway spruce. For. Ecol. Manag. 367, 30–40. doi: 10.1016/j.foreco.2016.02.020
De Vos, B., Cools, N., Ilvesniemi, H., Vesterdal, L., Vanguelova, E., and Carnicelli, S. (2015). Benchmark values for forest soil carbon stocks in Europe: results from a large scale forest soil survey. Geoderma 25, 33–46. doi: 10.1016/j.geoderma.2015.03.008
De Wandeler, H., Sousa-Silva, R., Ampoorter, E., Bruelheide, H., Carnol, M., Dawud, S. M., et al. (2016). Drivers of earthworm incidence and abundance across European forests. Soil Biol. Biochem. 99, 167–178. doi: 10.1016/j.soilbio.2016.05.003
Desie, E., Van Meerbeek, K., De Wandeler, H., Bruelheide, H., Domisch, T., Jaroszewicz, B., et al. (2020a). Positive feedback loop between earthworms, humus form and soil pH reinforces earthworm abundance in European forests. Funct. Ecol. 34, 2598–2610. doi: 10.1111/1365-2435.13668
Desie, E., Vancampenhout, K., Heyens, K., Hlava, J., Verheyen, K., and Muys, B. (2019). Forest conversion to conifers induces a regime shift in soil process domain affecting carbon stability. Soil Biol. Biochem. 136, 107540. doi: 10.1016/j.soilbio.2019.107540
Desie, E., Vancampenhout, K., Nyssen, B., van den Berg, L., Weijters, M., van Duinen, G.-J., et al. (2020b). Litter quality and the law of the most limiting: opportunities for restoring nutrient cycles in acidified forest soils. Sci. Tot. Environ. 699:134383. doi: 10.1016/J.SCITOTENV.2019.134383
Desie, E., Vancampenhout, K., van den Berg, L., Nyssen, B., Weijters, M., den Ouden, J., et al. (2020c). Litter share and clay content determine soil restoration effects of rich litter tree species in forests on acidified sandy soils. For. Ecol. Manag. 474:118377. doi: 10.1016/j.foreco.2020.118377
Driessen, P. M., Deckers, J., Spaargaren, O., and Nachtergaele, F. (2001). Lecture notes on the major soils of the world. Rome: Food and Agriculture Organization of the United Nations.
Duchaufour, P. (1990). La formation et l’évolution des complexes organo-minéraux dans les sols et leur rôle dans la pédogénèse. Sci. Du Sol 28, 273–284.
Eastwood, D. C., Floudas, D., Binder, M., Majcherczyk, A., Schneider, P., Aerts, A., et al. (2011). The plant cell wall-decomposing machinery underlies the functional diversity of forest fungi. Science 333, 762–765. doi: 10.1126/science.1205411
Ehrenfeld, J. G., Ravit, B., and Elgersma, K. (2005). Feedback in the plant-soil system. Annu. Rev. Environ. Resourc. 30, 75–115. doi: 10.1146/annurev.energy.30.050504.144212
Eviner, V. T., and Hawkes, C. V. (2008). Embracing variability in the application of plant-soil interactions to the restoration of communities and ecosystems. Restor. Ecol. 16, 713–729. doi: 10.1111/j.1526-100X.2008.00482.x
Fierer, N., and Jackson, R. B. (2006). The diversity and biogeography of soil bacterial communities. Proc. Natl. Acad. Sci. U.S.A. 103, 626–631. doi: 10.1073/pnas.0507535103
Finzi, A. C., Canham, C. D., and Van Breemen, N. (1998). Canopy tree-soil interactions within temperate forests: species effects on pH and cations. Ecol. Appl. 8, 447–454.
Frouz, J., Livečková, M., Albrechtová, J., Chroňáková, A., Cajthaml, T., Pižl, V., et al. (2013). Is the effect of trees on soil properties mediated by soil fauna? A case study from post-mining sites. For. Ecol. Manag. 309, 87–95. doi: 10.1016/j.foreco.2013.02.013
FAO (2006). Global Forest Resources Assessment 2005, Main Report. Progress Towards Sustainable Forest Management. FAO Forestry Paper 147. Rome: FAO.
Giannetta, B., Plaza, C., Zaccone, C., Vischetti, C., and Rovira, P. (2019). Ecosystem type effects on the stabilization of organic matter in soils: combining size fractionation with sequential chemical extractions. Geoderma 353, 423–434. doi: 10.1016/j.geoderma.2019.07.009
Guo, L. B., Halliday, M. J., Siakimotu, S. J. M., and Gifford, R. M. (2005). Fine root production and litter input: its effects on soil carbon. Plant Soil 272, 1–10. doi: 10.1007/s11104-004-3611-z
Gurmesa, G. A., Schmidt, I. K., Gundersen, P., and Vesterdal, L. (2013). Soil carbon accumulation and nitrogen retention traits of four tree species grown in common gardens. For. Ecol. Manag. 309, 47–57. doi: 10.1016/j.foreco.2013.02.015
Heckman, K., Welty-Bernard, A., Rasmussen, C., and Schwartz, E. (2009). Geologic controls of soil carbon cycling and microbial dynamics in temperate conifer forests. Chem. Geol. 267, 12–23. doi: 10.1016/j.chemgeo.2009.01.004
Heděnec, P., Nilsson, L. O., Zheng, H., Gundersen, P., Schmidt, I. K., Rousk, J., et al. (2020). Mycorrhizal association of common European tree species shapes biomass and metabolic activity of bacterial and fungal communities in soil. Soil Biol. Biochem. 149:107933. doi: 10.1016/j.soilbio.2020.107933
Heitkötter, J., Heinze, S., and Marschner, B. (2017). Relevance of substrate quality and nutrients for microbial C-turnover in top- and subsoil of a Dystric Cambisol. Geoderma 302, 89–99. doi: 10.1016/j.geoderma.2017.04.029
Hobbie, S. E., Ogdahl, M., Chorover, J., Chadwick, O. A., Oleksyn, J., Zytkowiak, R., et al. (2007). Tree species effects on soil organic matter dynamics: the role of soil cation composition. Ecosystems 10, 999–1018. doi: 10.1007/s10021-007-9073-4
IGBP-DIS (1998). SoilData(V.0) A Program for Creating Global Soil-Property Databases, IGBP Global Soils Data Task, France. Paris: IGBP-DIS.
Jackson, R. B., Lajtha, K., Crow, S. E., Hugelius, G., Kramer, M. G., and Piñeiro, G. (2017). The ecology of soil carbon: pools, vulnerabilities, and biotic and abiotic controls. Annu. Rev. Ecol. Evol. Syst. 48, 419–445. doi: 10.1146/annurev-ecolsys-112414-054234
Jandl, R., Lindner, M., Vesterdal, L., Bauwens, B., Baritz, R., Hagedorn, F., et al. (2007). How strongly can forest management influence soil carbon sequestration? Geoderma 137, 253–268. doi: 10.1016/J.GEODERMA.2006.09.003
Joly, F.-X., Milcu, A., Scherer-Lorenzen, M., Jean, L.-K., Bussotti, F., Dawud, S. M., et al. (2017). Tree species diversity affects decomposition through modified micro-environmental conditions across European forests. New Phytol. 214, 1281–1293. doi: 10.1111/nph.14452
Kögel-Knabner, I., and Amelung, W. (2021). Soil organic matter in major pedogenic soil groups. Geoderma 384:114785. doi: 10.1016/j.geoderma.2020.114785
Kooijman, A. M., and Martinez-Hernandez, G. B. (2009). Effects of litter quality and parent material on organic matter characteristics and N-dynamics in Luxembourg beech and hornbeam forests. For. Ecol. Manag. 257, 1732–1739. doi: 10.1016/j.foreco.2009.01.030
Korboulewsky, N., Perez, G., and Chauvat, M. (2016). How tree diversity affects soil fauna diversity: a review. Soil Biol. Biochem. 94, 94–106. doi: 10.1016/j.soilbio.2015.11.024
Laganière, J., Angers, D. A., Paré, D., Bergeron, Y., and Chen, H. Y. H. (2011). Black spruce soils accumulate more uncomplexed organic matter than aspen soils. Soil Sci. Soc. Am. J. 75, 1125–1132. doi: 10.2136/sssaj2010.0275
Lehmann, J., Hansel, C. M., Kaiser, C., Kleber, M., Maher, K., Manzoni, S., et al. (2020). Persistence of soil organic carbon caused by functional complexity. Nat. Geosci. 13, 529–534. doi: 10.1038/s41561-020-0612-3
Liang, C., Schimel, J. P., and Jastrow, J. D. (2017). The importance of anabolism in microbial control over soil carbon storage. Nat. Microbiol. 2:17105. doi: 10.1038/nmicrobiol.2017.105
Lin, G., McCormack, M. L., Ma, C., and Guo, D. (2017). Similar below-ground carbon cycling dynamics but contrasting modes of nitrogen cycling between arbuscular mycorrhizal and ectomycorrhizal forests. New Phytol. 213, 1440–1451. doi: 10.1111/nph.14206
Mareschal, L., Bonnaud, P., Turpault, M. P., and Ranger, J. (2010). Impact of common European tree species on the chemical and physicochemical properties of fine earth: an unusual pattern. Eur. J. Soil Sci. 61, 14–23. doi: 10.1111/j.1365-2389.2009.01206.x
Mayer, M., Prescott, C. E., Abaker, W. E. A., Augusto, L., Cécillon, L., Ferreira, G. W. D., et al. (2020). Influence of forest management activities on soil organic carbon stocks: a knowledge synthesis. For. Ecol. Manag. 466:118127. doi: 10.1016/j.foreco.2020.118127
Messier, C. C., Puettmann, K. J., and Coates, K. D. (2013). Managing Forests as Complex Adaptive Systems: Building Resilience to the Challenge of Global Change. Milton Park: Routledge.
Minasny, B., Malone, B. P., McBratney, A. B., Angers, D. A., Arrouays, D., Chambers, A., et al. (2017). Soil carbon 4 per mille. Geoderma 292, 59–86. doi: 10.1016/J.GEODERMA.2017.01.002
Mueller, K. E., Hobbie, S. E., Chorover, J., Reich, P. B., Eisenhauer, N., Castellano, M. J., et al. (2015). Effects of litter traits, soil biota, and soil chemistry on soil carbon stocks at a common garden with 14 tree species. Biogeochemistry 123, 313–327. doi: 10.1007/s10533-015-0083-6
Muys, B., Lust, N., and Granval, P. (1992). Effects of grassland afforestation with different tree species on earthworm communities, litter decomposition and nutrient status. Soil Biol. Biochem. 24, 1459–1466. doi: 10.1016/0038-0717(92)90133-I
Oostra, S., Majdi, H., and Olsson, M. (2006). Impact of tree species on soil carbon stocks and soil acidity in southern Sweden. Scand. J. For. Res. 21, 364–371. doi: 10.1080/02827580600950172
Palozzi, J. E., and Lindo, Z. (2018). Are leaf litter and microbes team players? Interpreting home-field advantage decomposition dynamics. Soil Biol. Biochem. 124, 189–198. doi: 10.1016/j.soilbio.2018.06.018
Peng, Y., Schmidt, I. K., Zheng, H., Heděnec, P., Bachega, L. R., Yue, K., et al. (2020). Tree species effects on topsoil carbon stock and concentration are mediated by tree species type, mycorrhizal association, and N-fixing ability at the global scale. For. Ecol. Manag. 478:118510. doi: 10.1016/j.foreco.2020.118510
Phillips, R. P., Brzostek, E., and Midgley, M. G. (2013). The mycorrhizal-associated nutrient economy: a new framework for predicting carbon-nutrient couplings in temperate forests. New Phytol. 199, 41–51. doi: 10.1111/nph.12221
Ponge, J., Zanella, A., Sartori, G., Jabiol, B., Ponge, J., Zanella, A., et al. (2010). Terrestrial Humus Forms: Ecological Relevance and Classification. Available online at: https://hal.archives-ouvertes.fr/hal-00521337. (accessed December 18, 2020).
Ponge, J.-F. (2003). Humus forms in terrestrial ecosystems: a framework to biodiversity. Soil Biol. Biochem. 35, 935–945.
Ponge, J.-F. (2013). Plant-soil feedbacks mediated by humus forms: a review. Soil Biol. Biochem. 57, 1048–1060. doi: 10.1016/j.soilbio.2012.07.019
Prescott, C. E., and Vesterdal, L. (2013). Tree species effects on soils in temperate and boreal forests: emerging themes and research needs. For. Ecol. Manag. 309, 1–3. doi: 10.1016/j.foreco.2013.06.042
Rasmussen, C., Heckman, K., Wieder, W. R., Keiluweit, M., Lawrence, C. R., Berhe, A. A., et al. (2018). Beyond clay: towards an improved set of variables for predicting soil organic matter content. Biogeochemistry 137, 297–306. doi: 10.1007/s10533-018-0424-3
Raulund-Rasmussen, K., and Vejre, H. (1995). Effect of tree species and soil properties on nutrient immobilization in the forest floor. Plant Soil 16, 345–352. doi: 10.1007/bf00029347
Reich, P. B., Oleksyn, J., Modrzynski, J., Mrozinski, P., Hobbie, S. E., Eissenstat, D. M., et al. (2005). Linking litter calcium, earthworms and soil properties: a common garden test with 14 tree species. Ecol. Lett. 8, 811–818.
Ribbons, R. R., Kepfer-Rojas, S., Kosawang, C., Hansen, O. K., Ambus, P., McDonald, M., et al. (2018). Context-dependent tree species effects on soil nitrogen transformations and related microbial functional genes. Biogeochemistry 140, 145–160. doi: 10.1007/s10533-018-0480-8
Rumpel, C., and Kögel-Knabner, I. (2011). Deep soil organic matter—a key but poorly understood component of terrestrial C cycle. Plant Soil 338, 143–158. doi: 10.1007/s11104-010-0391-5
Scheffer, M., Carpenter, S., Foley, J. A., Folke, C., and Walker, B. (2001). Catastrophic shifts in ecosystems. Nature 413, 591–596. doi: 10.1038/35098000
Schelfhout, S., Mertens, J., Verheyen, K., Vesterdal, L., Baeten, L., Muys, B., et al. (2017). Tree species identity shapes earthworm communities. Forests 8:85. doi: 10.3390/f8030085
Schmidt, M. W. I., Torn, M. S., Abiven, S., Dittmar, T., Guggenberger, G., Janssens, I. A., et al. (2011). Persistence of soil organic matter as an ecosystem property. Nature 478, 49–56. doi: 10.1038/nature10386
Schwertmann, U., Süsser, P., and Nätscher, L. (1987). Protonenpuffersubstanzen in Böden. J. Plant Nutr. Soil Sci. 150, 174–178.
Slessarev, E. W., Lin, Y., Bingham, N. L., Johnson, J. E., Dai, Y., Schimel, J. P., et al. (2016). Water balance creates a threshold in soil pH at the global scale. Nature 540, 567–569. doi: 10.1038/nature20139
Solly, E. F., Weber, V., Zimmermann, S., Walthert, L., Hagedorn, F., and Schmidt, M. W. I. (2020). A critical evaluation of the relationship between the effective cation exchange capacity and soil organic carbon content in swiss forest soils. Front. For. Glob. Change 3:98. doi: 10.3389/ffgc.2020.00098
Spielvogel, S., Prietzel, J., Leide, J., Riedel, M., Zemke, J., and Kögel-knabner, I. (2014). Distribution of cutin and suberin biomarkers under forest trees with different root systems. Plant Soil 381, 95–110. doi: 10.1007/s11104-014-2103-z
Taylor, A. R., Lenoir, L., Vegerfors, B., and Persson, T. (2019). Ant and earthworm bioturbation in cold-temperate ecosystems. Ecosystems 22, 981–994. doi: 10.1007/s10021-018-0317-2
Thomas, C. L., Jansen, B., Loon, E. E., and Van Wiesenberg, G. L. B. (2021). Transformation of n-alkanes from plant to soil: a review. Soil Discuss. doi: 10.5194/soil-2020-107, in review, 2021
Tiessen, H., Cuevas, E., and Chacon, P. (1994). The role of soil organic matter in sustaining soil fertility. Nature 371, 783–785. doi: 10.1038/371783a0
Toutain, F. (1981). Les humus forestiers: biodynamique et modes de fonctionnement. Rev. For. Française 33, 449–477.
Ulrich, B. (1987). Stability, elasticity, and resilience of terrestrial ecosystems with respect to matter balance. Ecol. Stud. 61, 11–49.
Ulrich, B. (1991). “An ecosystem approach to soil acidification,” in Soil Acidity, eds B. Ulrich and M. E. Sumner (Berlin: Springer), 28–79.
van Breemen, N., Finzi, A. C., and Canham, C. D. (1997). Canopy tree-soil interactions within temperate forests: effects of soil elemental composition and texture on species distributions. Can. J. For. Res. 27, 1110–1116. doi: 10.1139/x97-061
van Oijen, O., Markus, F., Hommel, P., den Ouden, J., and de Waal, R. (2005). Effects of tree species composition on within-forest distribution of understorey species. Appl. Veg. Sci. 8, 155–166. doi: 10.1111/j.1654-109X.2005.tb00641.x
Vancampenhout, K., Wouters, K., De Vos, B., Buurman, P., Swennen, R., and Deckers, J. (2009). Differences in chemical composition of soil organic matter in natural ecosystems from different climatic regions – a pyrolysis–GC/MS study. Soil Biol. Biochem. 41, 568–579. doi: 10.1016/j.soilbio.2008.12.023
Vedy, J. E. (1973). Relations Entre le Cycle Biogéochimique Des Cations et L’humification en Milieu Acide. Thèsis Doctoral. Nancy: University of Nancy, 206.
Verstraeten, G., Vancampenhout, K., Desie, E., De Schrijver, A., Hlava, J., Schelfhout, S., et al. (2018). Tree species effects are amplified by clay content in acidic soils. Soil Biol. Biochem. 121, 43–49. doi: 10.1016/j.soilbio.2018.02.021
Vesterdal, L., and Raulund-Rasmussen, K. (1998). Forest floor chemistry under seven tree species along a soil fertility gradient. Can. J. For. Res. 28, 1636–1647. doi: 10.1139/x98-140
Vesterdal, L., Clarke, N., Sigurdsson, B. D., and Gundersen, P. (2013). Do tree species influence soil carbon stocks in temperate and boreal forests? For. Ecol. Manag. 309, 4–18. doi: 10.1016/j.foreco.2013.01.017
Vesterdal, L., Schmidt, I. K., Callesen, I., Nilsson, L. O., and Gundersen, P. (2008). Carbon and nitrogen in forest floor and mineral soil under six common European tree species. For. Ecol. Manag. 255, 35–48. doi: 10.1016/J.FORECO.2007.08.015
von Lützow, M., Kogel-Knabner, I., Ekschmitt, K., Matzner, E., Guggenberger, G., Marschner, B., et al. (2006). Stabilization of organic matter in temperate soils: mechanisms and their relevance under different soil conditions— a review. Eur. J. Soil Sci. 57, 426–445.
Wardle, D. A., Bardgett, R. D., Klironomos, J. N., Setälä, H., van der Putten, W. H., and Wall, D. H. (2004). Ecological linkages between aboveground and belowground biota. Science 304, 1629–1633. doi: 10.1126/science.1094875
Wiesmeier, M., Urbanski, L., Hobley, E., Lang, B., Von Lützow, M., Marin-Spiotta, E., et al. (2019). Soil organic carbon storage as a key function of soils - a review of drivers and indicators at various scales. Geoderma 333, 149–162. doi: 10.1016/j.geoderma.2018.07.026
Keywords: SOC, tree species effect, litter quality, soil acidity, soil process domain, acid buffering, CEC, context-dependency
Citation: Desie E, Muys B, Jansen B, Vesterdal L and Vancampenhout K (2021) Pedogenic Threshold in Acidity Explains Context-Dependent Tree Species Effects on Soil Carbon. Front. For. Glob. Change 4:679813. doi: 10.3389/ffgc.2021.679813
Received: 12 March 2021; Accepted: 03 May 2021;
Published: 25 May 2021.
Edited by:
Sandra Spielvogel, University of Kiel, GermanyReviewed by:
Jörg Prietzel, Technical University of Munich, GermanyStephan Zimmermann, Swiss Federal Institute for Forest, Snow and Landscape Research (WSL), Switzerland
Copyright © 2021 Desie, Muys, Jansen, Vesterdal and Vancampenhout. This is an open-access article distributed under the terms of the Creative Commons Attribution License (CC BY). The use, distribution or reproduction in other forums is permitted, provided the original author(s) and the copyright owner(s) are credited and that the original publication in this journal is cited, in accordance with accepted academic practice. No use, distribution or reproduction is permitted which does not comply with these terms.
*Correspondence: Ellen Desie, ZWxsZW4uZGVzaWVAa3VsZXV2ZW4uYmU=