- 1Centre d’étude de la forêt, Université du Québec à Montréal, Québec, QC, Canada
- 2Département Science et Technologie, Téluq, Université du Québec, Québec, QC, Canada
Conifers and deciduous trees greatly differ in regard to their phylogenetics and physiology as well as their influence on soil microclimate and chemical properties. Soil respiration (Rs) in forests can therefore differ depending on tree species composition, and assessments of the variation in Rs in various forest types will lead to a more thorough understanding of the carbon cycle and more robust long-term simulations of soil carbon. We measured Rs in 2019 and 2020 in stands of various species composition in a sugar maple forest near the northern range limit of temperate deciduous forests in Quebec, Canada. Seasonal variations in soil temperature had the largest influence on Rs, but conditions created by the stands also exerted a significant effect. Relative to the typical sugar maple-yellow birch forest (hardwoods), Rs in stands with >20% of basal area from balsam fir (mixedwoods) was increased by 21%. Whilst, when American beech contributed >20% of litterfall mass (hardwood-beech stands), Rs was decreased by 11 and 36% relative to hardwoods and mixedwoods, respectively. As a whole, Rs was significantly higher in mixedwoods than in other forest types, and Rs was significantly higher in hardwoods than in hardwood-beech stands. Sugar maple and American beech at the study site are near their northern range limit, whereas balsam fir is near its southern limit. Rs in mixedwoods was therefore higher than in hardwoods and hardwood-beech stands due to high root activity in the presence of fir, despite colder and drier soils. We estimated that root respiration in mixedwoods was more than threefold that in hardwoods and hardwood-beech stands. The lower Rs in hardwood-beech stands compared to hardwoods points to the lower soil temperature as well as the poor quality of beech litter (low decomposability) as indicated by a generally lower heterotrophic respiration. Other than soil temperature, regression models identified mixedwoods, soil water potential and Mg2+ activity in the soil solution as important predictor variables of Rs with about 90% of its variation explained. Our study shows the benefits of combining forest-specific properties to climatic data for more robust predictions of Rs.
Introduction
Soil respiration (Rs) is the second largest CO2 flux in forests after plant respiration and in turn, it has a high potential to modify atmospheric CO2 levels (Schlesinger and Andrews, 2000; Raich et al., 2002). It is constituted of heterotrophic (Rh) and autotrophic (Ra) respiration. The Rh component comes from the respiration of the soil faunal and microbial communities that physically breakdown and biochemically decompose dead organic matter, whereas the Ra component comes from root and rhizosphere respiration. Soil temperature is the main abiotic control of Rs in forests (Davidson and Janssens, 2006; Subke and Bahn, 2010). The temperature sensitivity of Rs is often expressed as Q10 − it is the proportional change in respiration with a 10°C increase in soil temperature (Curiel Yuste et al., 2004). Soil moisture also interacts with soil temperature to influence Rs in forests (Cisneros-Dozal et al., 2006; Moyano et al., 2012). Soil temperature and moisture may vary seasonally and across years, thereby modifying the temperature sensitivity of Rs in forest ecosystems (Davidson et al., 1998). Other factors also influence the seasonal patterns of Rs in forests such as variation in root activity during the growing season and fresh litter additions in the fall (Boone et al., 1998; Lavigne et al., 2004; Prévost-Bourré et al., 2010).
Changes in forest structure and species composition that lead to a modification in soil conditions are thus expected to influence Rs. Soil respiration tends to increase with stand age due to root growth and respiration (Subke et al., 2006), but this trend can be absent in forests where crown closure reduces soil temperature (e.g., Gough et al., 2005). Forest species composition may also influence Rs by affecting: (1) soil chemical properties (e.g., pH, N, and P availability), (2) root biomass, respiration and phenology, (3) litter inputs, quality and decomposability, and (4) microbial communities and activity (Raich and Tufekcioglu, 2000). Side-by-side comparisons of Rs between deciduous and coniferous stands generally suggest higher fluxes under deciduous trees (Tewary et al., 1982; Weber, 1985, 1990; Raich and Tufekcioglu, 2000; Curiel Yuste et al., 2004; Fahey et al., 2005), but lower Rs under deciduous (Lee et al., 2010) or no difference in Rs between forest types (Reiners, 1968; Raich and Potter, 1995; Davidson et al., 1998; Borken et al., 2002; Fernández-Alonso et al., 2018) have also been reported.
The generally lower quality of conifer litter and Rh relative to deciduous tree species was a common explanation for the lower Rs measured in coniferous stands, whereas differences in phenology and physiology between tree species explained no change or higher Rs in coniferous stands.
Worldwide, temperate deciduous forests were estimated to hold 7–8% of global terrestrial C stocks (Pregitzer and Euskirchen, 2004). Cool temperate deciduous forests in eastern Canada offer high C sequestration potential because they are among the most productive ecosystems in the country due to their relatively long growing season and sufficient precipitation falling during the growing season (Kurz and Apps, 1999; Stinson et al., 2011). Also, there is little fire disturbance or forest harvesting (mostly uneven age management) that could release large amounts of C back to the atmosphere (Saucier et al., 2009a). Fahey et al. (2005) provided Rs estimates for various deciduous and coniferous stands in the cool temperate Hubbard Brook Experimental Forest, New Hampshire. However, there is no study that investigated the variations in Rs near the northern limit of temperate deciduous forests in northeastern North America. These forests are associated with common tree species changes due to the presence of deciduous species to the south and coniferous (boreal) species to the north. Furthermore, northeastern North American deciduous forests are characterized by replacement patterns between sugar maple (Acer saccharum) and American beech (Fagus grandifolia). This replacement pattern is also observed at their northern limit (Collin et al., 2017). Light, water and nutrient availability as well as reproduction rates govern the replacement patterns between the two species. These factors can be modified due to natural disturbances such as ice storms, disease and herbivory (Arii and Lechowicz, 2002; Bohn and Nyland, 2003; Nyland et al., 2006), forest management practices (Nolet et al., 2008; Bannon et al., 2015) and atmospheric acid deposition (Bailey et al., 2004; Long et al., 2009). Water limitations for tree growth are suspected to increase in frequency and intensity under climate change in eastern North America (Gustafson and Sturtevant, 2013), whereas sugar maple is likely more sensitive than American beech to water stress (Nolet and Kneeshaw, 2018). Overall, the recent increase in the dominance of beech in the understory of maple stands suggests that beech stands will occupy forest land in the future (Hane, 2003; Gravel et al., 2010). Similar to conifer litter, the high recalcitrance of American beech litter (Melillo et al., 1982; Côté and Fyles, 1994) has the potential of reducing Rs by slowing down litter turnover and thus decreasing Rh (Bowden et al., 1993; Cisneros-Dozal et al., 2007).
Climate change is causing physiological constraints that have the potential of impacting the survival, growth and distribution of plant species globally (Rosenzweig et al., 2008; Chen et al., 2011), including northeastern North American tree species (Beckage et al., 2008; Charney et al., 2016; Sittaro et al., 2017; Iverson et al., 2019; Boivert-Marsh and de Blois, 2021). In addition to understanding how tree growth and thus forest C uptake will be affected by climate change, analyses of how forest species compositions affect Rs are needed for more robust predictions of temporal and spatial changes in ecosystem C pools and atmospheric CO2 (Raich and Tufekcioglu, 2000; Buchholz et al., 2014). As sugar maple, American beech and conifers differ in regard to their physiology, biogeochemistry (e.g., litter quality/decomposition) and phylogenetics (angiosperm vs. gymnosperm), Rs is expected to vary depending on the proportion of these tree species within a forest. The objective of this study was to assess Rs across a range of plots that captured variations in the abundance of conifers and American beech within a forest dominated by sugar maple near the northern range limit of temperate deciduous forests in eastern North America. Mostly due to their lower litter qualities compared to maple litter, it was hypothesized that an increase in the abundance of conifers and beech would decrease Rs in this maple forest.
Materials and Methods
Study Site
The study was conducted at the Station de Biologie des Laurentides (SBL) of Université de Montréal in St. Hippolyte, Quebec (Figure 1). The SBL is found within the northern limit of the maple-yellow birch (Betula alleghaniensis) bioclimatic domain of the lower Laurentians. The maple-yellow birch domain is the northernmost deciduous forest domain in Quebec (Saucier et al., 2009b). A mosaic of tree species is found at the site. It is composed mostly of sugar maple, red maple (Acer rubrum), American beech, yellow birch (Betula alleghaniensis), white birch (Betula payrifera), largetooth aspen (Populus grandidentata), eastern white cedar (Thuja occidentalis), white pine (Pinus strobus), and red spruce (Picea rubens) (Savage, 2001). Because SBL is only 35 km south of La Macaza, i.e., the doorway to temperate mixedwoods at that specific longitude in Quebec (Figure 1), stands dominated with tree species that are typically boreal [e.g., balsam fir (Abies balsamea) and white spruce (Picea glauca)] are not uncommon at the site. Also, stands exhibiting sugar maple regeneration failures and expansion of beech are frequent at SBL (Collin et al., 2017). The mean annual temperature at SBL simulated with the BioSIM model (Régnière and Bolstad, 1994) between 2003 and 2013 was 4.9°C, mean degree-days were 2845, mean days without frost were 153 and mean precipitation was 1,270 mm, with about 30% falling as snow. Soils were developed from glacial till made mostly of anorthosite and felsic rocks of the Precambrian Shield (Bélanger et al., 2012). They are classified as Orthic Humo-Ferric and Ferro-Humic Podzols with a sandy loam texture and the forest floor is characterized by a moder humus form (Soil Classification Working Group, 1998).
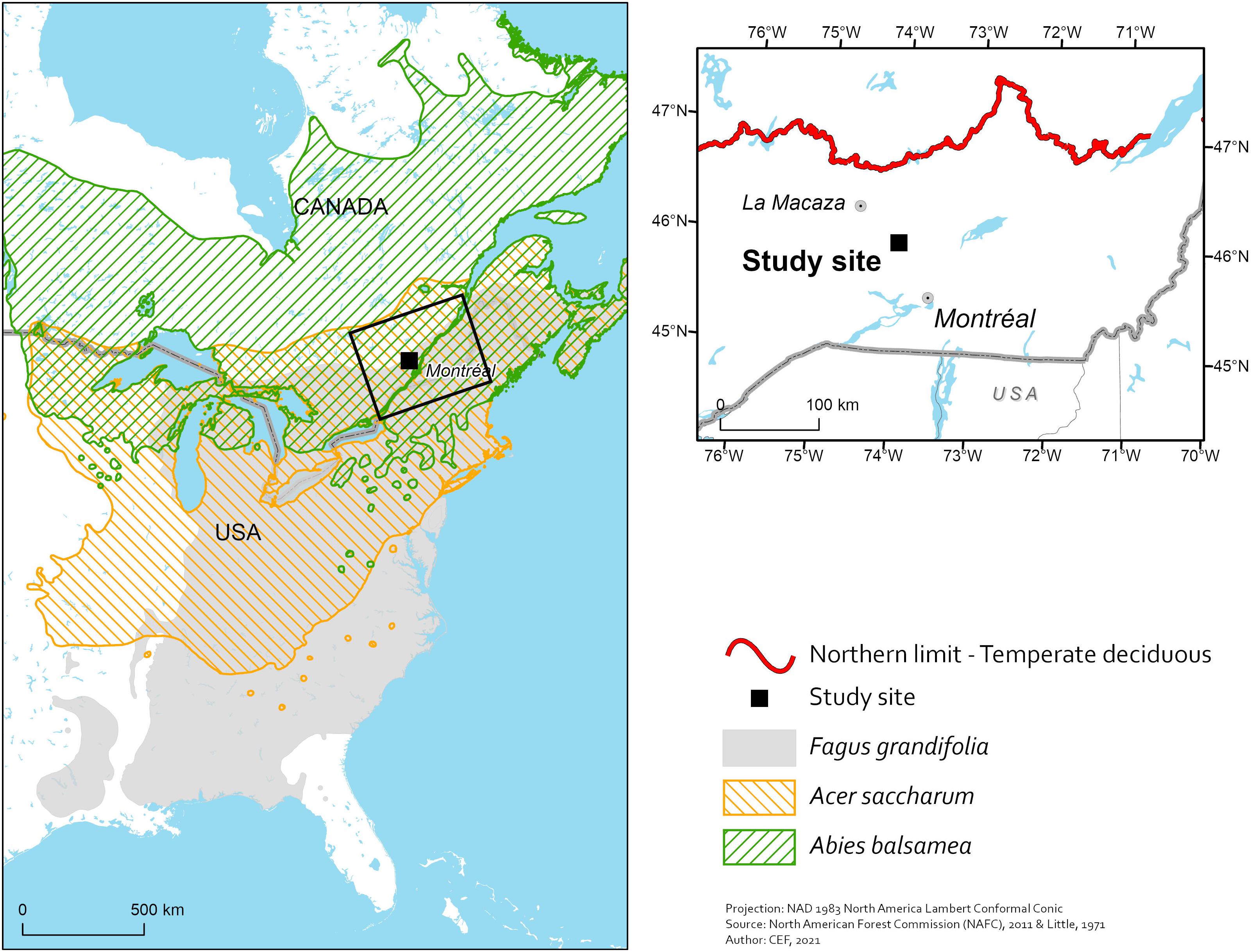
Figure 1. Location of Station de biologie des Laurentides (Study site) in southern Quebec, Canada, along with the distributions of sugar maple (Acer saccharum), American beech (Fagus grandifolia) and balsam fir (Abies balsamea) (left-hand map) as well as the northern limit of temperate deciduous forests (right-hand map).
Experimental Design and Stand/Plot Characterization
In 2018, eight stands with four 3 × 3 m plots within each stand (total of 32 plots) were selected in three zones distributed within a 18 ha area. Stands were selected to capture as much variation as possible in regard to tree species composition. Stands were a minimum of 0.5 ha. The four plots within each stand were delineated in the center of the stand (within a maximum of 15 m between the most distant plots) under a very similar tree species composition. A series of ecological variables were collected at the stand level between 2017 and 2020, allowing for a detailed classification of tree species composition. These included (1) basal area by species based on trees with a stem diameter at breast height above 9 cm (Table 1) and (2) litterfall mass by species (Table 2). At the center of each stand, litterfall was collected in a plastic bin (0.9 cm × 0.7 cm × 0.5 cm) that was perforated at the bottom and filled with silica sand (depth of about 5 cm) to drain the bin and thus prevent the trapped litter to immerse in water between samplings. Litter was collected each fall and dried in an oven at 65°C for 48 h before being weighted by species. Leaf area index was also measured in the center of each plot on a sunny morning of mid-August 2020 when the canopy was fully developed using a CI-110 Plant Canopy Imager (CID Bio-Science, Camas, WA).
Stands were classified as mixedwoods when basal area of balsam fir contributed to more than 20% of total basal area (i.e., stands 1, 4, and 7, Table 1). However, basal area by species may not be fully representative of deciduous litterfall type in the plots. For example, the high American beech sapling density in some stands was not captured in the basal area measurements, despite that beech litterfall is high (i.e., stands 3 and 8, Table 2). Because Rh is a significant component of Rs in temperate deciduous forests [25–35% of Rs according to Bowden et al. (1993) and Cisneros-Dozal et al. (2007)], we used litterfall mass by species to separate between hardwoods and hardwood-beech stands. In this respect, hardwood-beech stands were identified as having at least 20% of their total litterfall mass from beech (stands 3, 5, and 8), whereas hardwoods had less than 20% (stands 2 and 6, Table 2). In the case of stand 2, there was no presence of beech.
Soil temperature and water availability at a 10 cm depth were measured every 15 min in each plot from two temperature sensors (Spectrum Technologies, United States) and two water potential sensors (Irrometer 200SS-5, Watermark, United States), all connected to the same WatchDog 1650 Micro Station data logger (Spectrum Technologies). Plant Root Simulator (PRS) probes (Western Ag Innovations, Canada) were used to assess ionic activity (i.e., NO3–, NH4+, H2PO4–, Ca2+, Mg2+, K+, Al3+, Fe3+, and Mn2+) in the soil. Four pairs of cation and anion probes were carefully inserted vertically into the forest floor at random locations within each plot. They were installed in early June of 2019 and 2020 and collected 6 weeks later. The PRS probes represent a dynamic measurement of ions flowing through the soil over time compared to conventional soil extraction methods that provide a measurement of soil nutrient availability at a particular point in time. The probes are now frequently used in forest ecology research (Hangs et al., 2004; Bilodeau-Gauthier et al., 2013). Once extracted from the soil, the probes were cleaned with deionized H2O and stored in the fridge in zipseal bags until analysis. Probes were eluted for 1 h with 0.5 M HCl. NH4-N and NO3-N were determined colorimetrically by continuous flow analysis (Autoanalyser III, Bran and Luebbe, United States), whereas other ions were determined by inductively coupled plasma atomic emission spectroscopy (Optima 3000-DV, PerkinElmer, United States).
Gas Sampling, Laboratory Analysis, and Flux Calculations
Four circular bases were installed in each plot in July 2018. The bases were set out in a square shape pattern with a distance of 1.5 m between them. They were fabricated from high-density PVC 15 cm inside diameter pipes (i.d., surface area of 177 cm2) with smooth 6 mm thick side walls. The bases were cut to a length of 12 cm and were inserted at a soil depth of 6 cm. The flux chambers were fabricated from the female connection end of the pipes (i.d. of 15.5 cm, surface area of 189 cm2) with a double seal locked-in gasket, thus securing a tight seal with the base. The chamber was closed with a 4 mm thick Teflon sheet and the exterior was wrapped with reflective insolation to avoid overheating. The combination of the base and the length of the chamber results in a headspace volume of 4.56 L. The risk of pressurization of the flux chamber was eliminated by (1) venting with a Tygon tube (4 mm i.d.) that passed through the top of the chamber and (2) avoiding to sample under windy conditions. Gas sampling was done from an injection site (Bung Interlink IV, Baxter, United States). Debris were removed only when they obstructed the placement of the chamber on the base.
In 2019, gas sampling started in June and finished just before soil freezing in November 2019. It was done in all the plots within each stand and at five different dates (n = 160). Despite the large number of plots (4 plots per stand, 8 stands, and 32 plots in total), sampling was done within a day (∼6 h). In 2020, we opted for a higher sampling frequency but did not sample all stands or all four plots in each stand each time. We sampled two plots in all stands on June 9, June 22, July 9, July 22, August 26, and September 23, all plots in stands 1, 2, 3, and 4 (i.e., block 1) on June 15, June 30, July 21, and August 11, and one plot per stand on October 22. A rotation of plots was done for a better assessment of spatial variability within each stand. In total, 160 gas samples were collected at 11 different dates in 2020, but 4 samples were discarded due to sampling errors (n = 156).
Gas sampling was done as soon as the flux chamber was deposited on the base (t0). To do so, 5 ml of gas sample was withdrawn from the chamber using a 50 ml polypropylene syringe equipped with a 25-gauge 5/8-inch needle. The gas samples collected from each chamber (4×) were injected in the same pre-evacuated (ca. 0.005 atm.) 12 ml Exertainer® vial (LabCo, United Kingdom). The same procedure was repeated at 4 (t4), 8 (t8), 16 (t16), and 24 (t24) min. The gas samples were stored under a positive pressure of approximately 1.7-atm, i.e., respectively 20 ml of headspace gas into a 12 ml vial, to minimize any gaseous exchange with atmospheric air. The sampling scheme (i.e., pooling gas samples) followed the recommendation of Arias-Navarro et al. (2013) as a means to better capture spatial variability of soil gas efflux within the plots.
To provide an estimate of the contribution of the autotrophic (Ra) and heterotrophic (Rh) components to Rs, we used a root exclusion approach similar to Kelting et al. (1998). In mid-May 2020, we created four 1 × 1 m root-free plots by severing all the roots to a depth of 40 cm. This depth was considered to capture most of the roots because Lajeunesse (1990) measured that 70% of root mass at SBL is found within the first 25 cm of soil, i.e., the foret floor and upper podzolic B horizon. We had one plot in stands 1, 2, 3, and 4, being, respectively, mixedwood, hardwood, hardwood-beech and mixedwood. All four plots were delineated in proximity of the other sampling plots. There was barely any vegetation in those plots and any new growth was removed by clipping. We used the same sampling procedure as described above. No barrier was used to further limit root development because CO2 efflux measurements were planned to be performed in 2020 only. Sampling of these plots started on June 15, exactly 1 month after trenching, and continued on June 30, July 21, August 11. This sampling was done at the same time as the sampling scheme described above. For each forest type and sampling date, root respiration (Rroot) was calculated as the difference between Rs (i.e., mean of the standard 3 × 3 m plots) and soil respiration in the root-free plots (1 × 1 m plot), whereas Rh was assumed as the net flux from the trenched plots. The percent contributions of Rroot and Rh to Rs were also calculated. Soils can become wetter in the trenched plots due to the absence of water uptake by roots and this can affect soil respiration patterns when they exhibit extremes in moisture levels (Hanson et al., 2000). In addition, soil respiration from the trenched plots could include an added flux of CO2 because of a possible increase in the decomposition of dead roots [rhizosphere (or rhizomicrobial) respiration], especially a few months after trenching (thus the use of Rroot and not Ra). This means that Rroot could be an underestimation of root respiration, whereas Rh could be overestimated. Bowden et al. (1993) provided a strong argument that the contribution of decomposition of dead roots in trenched plots is small, at least in the short term. However, because we do not have estimates of root decomposition in the trenched plots, we need to interpret the data with care. The estimates were therefore used as a means to exhibit the main contrasts in Rroot and Rh patterns among the three forest types studied.
Carbon dioxide and CH4 analysis of gas samples was carried out within 48 h of completion of each sampling campaign. Cavity-ring down (CRD) spectroscopy (G2201-i Isotopic Analyzer, Picarro, United States) and ultra-zero air as the carrier gas were used for analysis. Before analysis, water vapor from gas samples was selectively removed using a monotube NafionTM gas dryer (MD-050-12-2, PermaPure, United States).
Raw CO2 fluxes were estimated by fitting t0, t4 (exp. 2 only), t8, t16, and t24 data to a linear model using the HMR package in R (Pedersen et al., 2010). We used a linear model for all flux samples for two main reasons: (1) it was the best fit for over 95% of samples, and (2) it provides more consistent flux results for short enclosure times and in cases of a concave flux curve, i.e., the most common non-linear pattern for the remaining 5% of samples (Kutzbach et al., 2007; Görres et al., 2014; Kandel et al., 2016). Selecting a single linear model thus avoided calculating differences in CO2 fluxes due to differences in models and not CO2 fluxes per se. Air temperature and pressure were used to adjust raw gas fluxes (Rochette and Bertrand, 2007). The well drained soils resulted in small CH4 sinks and therefore, CH4 fluxes were not investigated any further.
Data Analysis
To confirm the influence of soil temperature on Rs, we first fitted single exponential models of Rs with soil temperature using the equation Rs = a × e(b × soiltemp), where a and b are parameters to be estimated, e is the base of the natural logarithm (2.71828) and soil temp is measured soil temperature in °C. Models were fitted for each forest type and for all forest types combined (general model) for both years combined. We calculated R2 from the linear regression between measured vs. predicted Rs. The Q10 value for each model was obtained with the equation Q10 = e(b × 10). The residuals of the general model were then modeled using a forward stepwise regression with the following independent variables: soil water potential, total basal area, LAI, and NO3–, NH4+, H2PO4–, Ca2+, Mg2+, K+, Al3+, Fe3+, and Mn2+ activity in the soil solution as measured by PRS probes. Forest types were also tested after they were converted into dummy variables, i.e., mixedwoods (0) vs. other forest types (1), hardwood-beech stands (0) vs. other forest types (1), and hardwoods (0) vs. other forest types (1). Only the first three selected variables were kept in the model. This procedure may leave out some variables that are not necessarily unimportant. However, our purpose was to find a small set of logical predictor variables that did an adequate job of prediction while being relevant to biological theory (Sokal and Rohlf, 2012). All predictor variables individually improved the fit between the observed and predicted values at P < 0.01. The marginal sum of squares (type III) was also used to measure the predictive information contained in the predictor variables individually while considering the other predictor variables in the model. The variance inflation factor (VIF) was used to detect any co-linearity between the independent variables in the model. No VIF exceeded a value of 2.8. Transformation of the raw data was not necessary to assure equal variance and normality in the distribution of the residuals. Predicted Rs was plotted against measured Rs and the R2 of the linear regression was calculated as an indication of the capacity of this two stage modeling approach to explain the variation in Rs.
To assess the effect of forest types on Rs, we used two linear mixed effect models with forest type as the fixed factor in both models. In the first model, sampling date was used as the random factor, whereas soil temperature was used as the random factor in the second model. We proceeded this way to consider that soil temperature differences may be produced by the forest types and their canopies and, in turn, this could lead to differences in Rs. A square root transformation was used in both models to normalize the residuals, whereas predicted values were computed for subsequent display. To assess the effects of forest types on soil temperature and water potential, we first computed daily averages from May 15 to October 15 in 2019, and from May 15 to August 31 in 2020, i.e., the data that we could secure while avoiding logistical problems associated with snow cover in both years. Technical problems with dataloggers reduced the continuity of the data in 2020 and thus, we did not use time-series data for September and October for further analysis. With these data, we used linear mixed effect models with forest type as the fixed factor and sampling date as the random factor. A logarithmic transformation was used to warrant normality of the residuals. Finally, one-way ANOVA was used to detect differences in Rroot and Rh between forest types as well as their respective contributions (in %) to Rs. Block identity was not tested in any of the linear mixed effect models and ANOVAs because it was not possible to obtain all forest types within each zone and thus, zones should not be considered as a blocking structure per se. Significant differences between forest types detected with these tests were further depicted with post hoc multiple comparisons using the Tukey’s honest significant difference (HSD) test. Linear mixed models and ANOVAs were, respectively, conducted with the R statistical software (version 4.0.4) and the “Analysis” module in SigmaPlot 12.0.
Results
Soil respiration rates varied from as low as 21 mg CO2 m–2 h–1 in hardwood-beech stands on November 8, 2019, to as high as 726 mg CO2 m–2 h–1 in mixedwoods in July 9, 2020 (Figure 2). The large variation in Rs was associated to seasonality, increasing from June to July–August and decreasing again to the lowest values just before snow in late October of 2020 or early November of 2019 (Supplementary Figure 1). The seasonal effect was more apparent in 2020 because of a higher sampling frequency. Relatively strong exponential relationships were found between soil temperature and Rs for each forest type and all forest types combined (general model) (Figure 2). Model parameter estimates, Q10 and R2 are shown in Table 3. The modeled curve for mixedwoods was consistently above all other models. Conversely, the modeled curve for hardwood-beech stands was below all other models, although the regression lines between hardwoods and hardwood-beech stands crossed each other at a soil temperature of 15.5°C. Soil temperature alone explained 59% of the variance in Rs rates (see general models in Table 3). The predictive ability was higher for hardwood-beech stands and mixedwoods (64–65%) and lower for hardwoods (55%). Computed Q10 values were 2.26 for hardwoods, 2.44 for mixedwoods, 2.58 for all forest types, and 2.97 for hardwood-beech stands (Table 3).
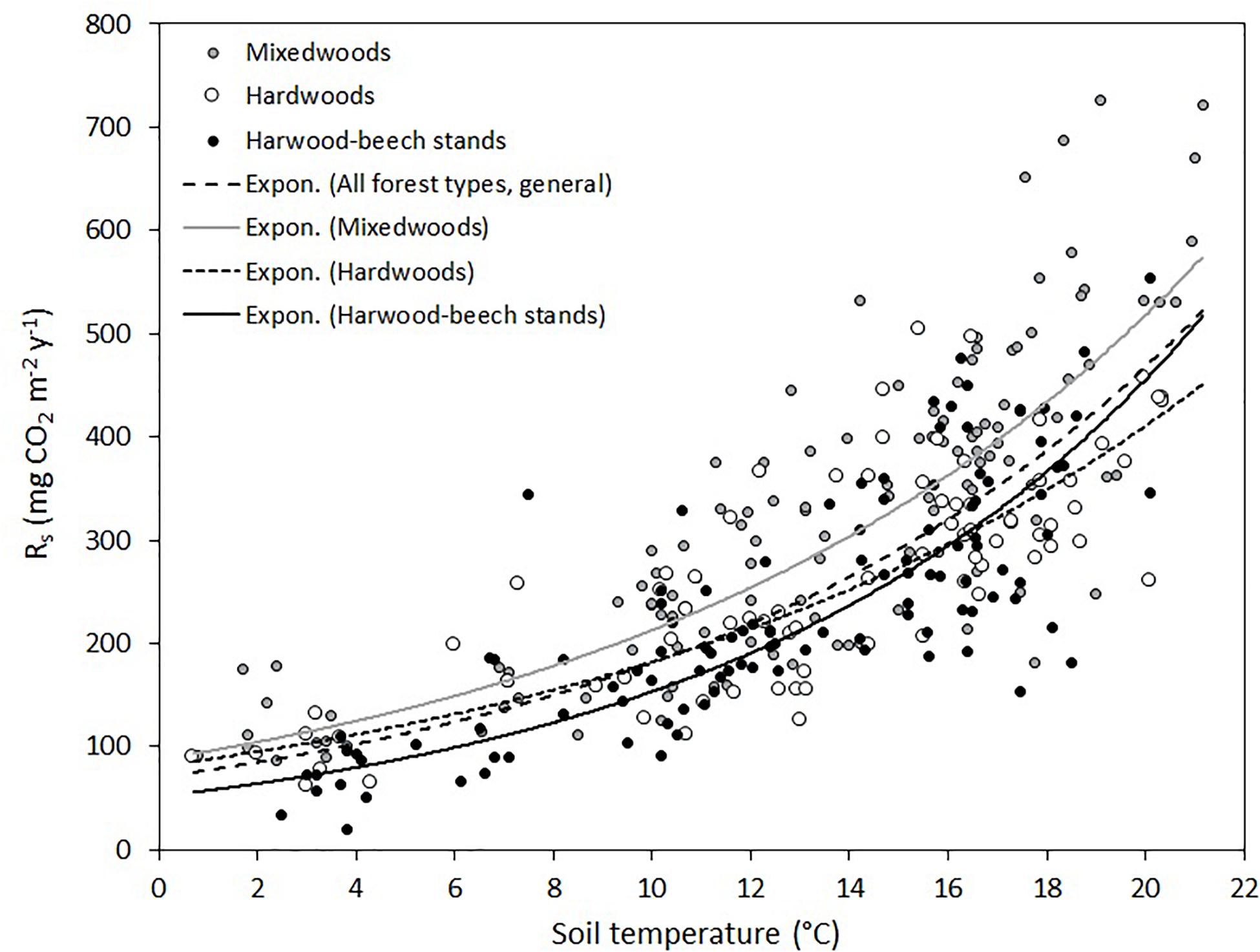
Figure 2. Exponential relationships between soil temperature and soil respiration rates (Rs) for each forest type individually and for all forest types combined (general model). Parameter estimates and Q10 of equation Rs = a × e(b × soil temperature) are presented in Table 3.
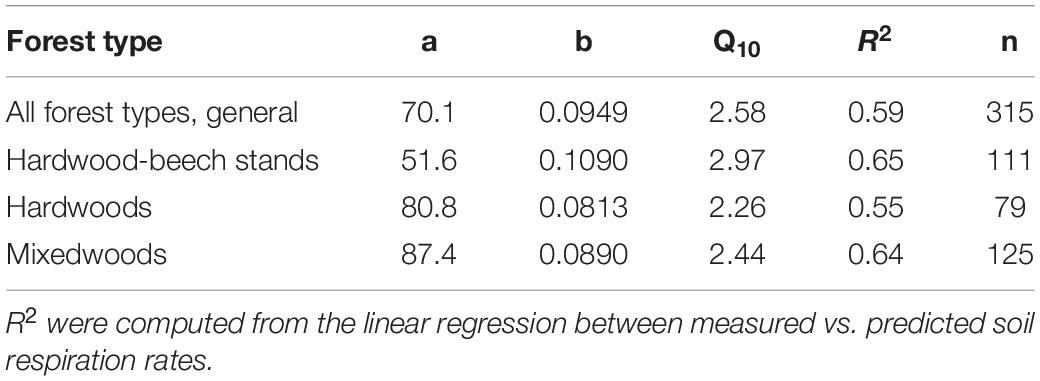
Table 3. Parameter estimates and Q10 values of exponential models (Figure 3) between soil temperature respiration rates for each forest type individually and for all forest types combined (general model).
The forward stepwise regression model of the residuals of the general exponential model (i.e., soil temperature vs. Rs) explained an additional 19% of the variation in Rs (Table 4). The dummy variable representing mixedwoods (0) vs. other forest types (1) was selected as the first predictor variable in all models and explained between 11% of the variation in Rs. Soil water potential was selected as the second predictor variable (5.3%), whereas Mg2+ activity in the soil solution was selected as the last predictor variable (3.0%). In the end, the linear relationship between measured and predicted Rs over the two study years suggest that the combination of both models (i.e., exponential model followed by multiple linear model) captured 89% of the total variation in Rs. A graphical illustration between measured and predicted values shows that this approach underestimated lower Rs values and overestimated higher Rs values (Figure 3).

Table 4. Multiple linear regression of the residuals of the general exponential relationship between soil respiration and soil temperature.
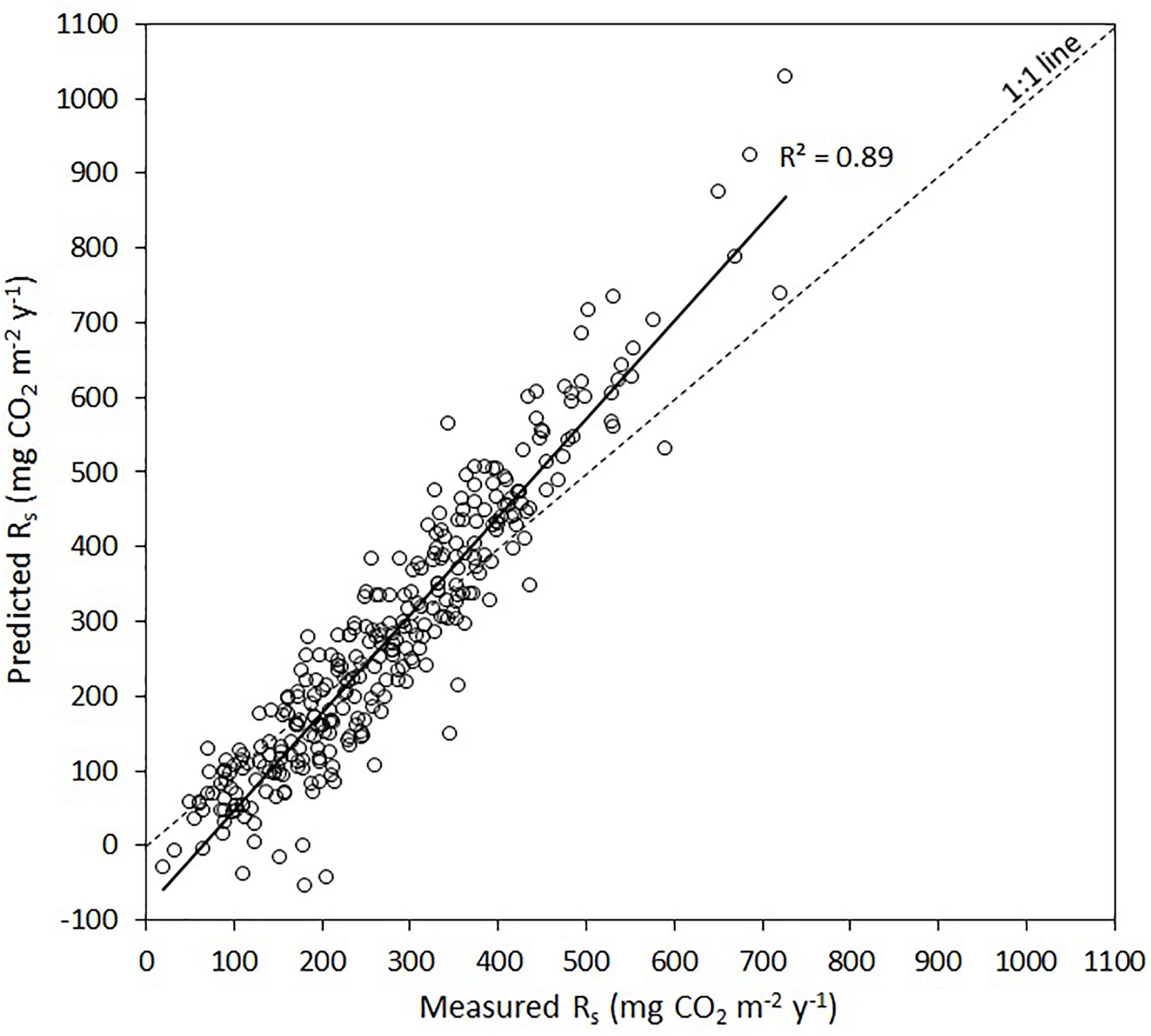
Figure 3. Measured soil respiration rates (Rs) vs. predicted Rs after the two stage modeling approach, i.e., exponential of the raw Rs data and soil temperature (Figure 2 and Table 3) followed by a multiple linear regression of the residuals (Table 4). The solid line is the linear regression between measured vs. predicted Rs, whereas the dashed line is the 1:1 line.
Over the two study years, Rs in mixedwoods was 21 and 36% higher than hardwoods and hardwood-beech stands, respectively (Figure 4). The difference in Rs between hardwood and hardwood-beech stands was 11%. Mixed model analysis with sampling date as a random variable suggest a significant difference in Rs between all forest types, whereas mixed model analysis using soil temperature as a random variable suggest a significantly higher Rs in mixedwoods than in hardwoods and hardwood-beech stands, but not between hardwoods and hardwood-beech stands (Table 3).
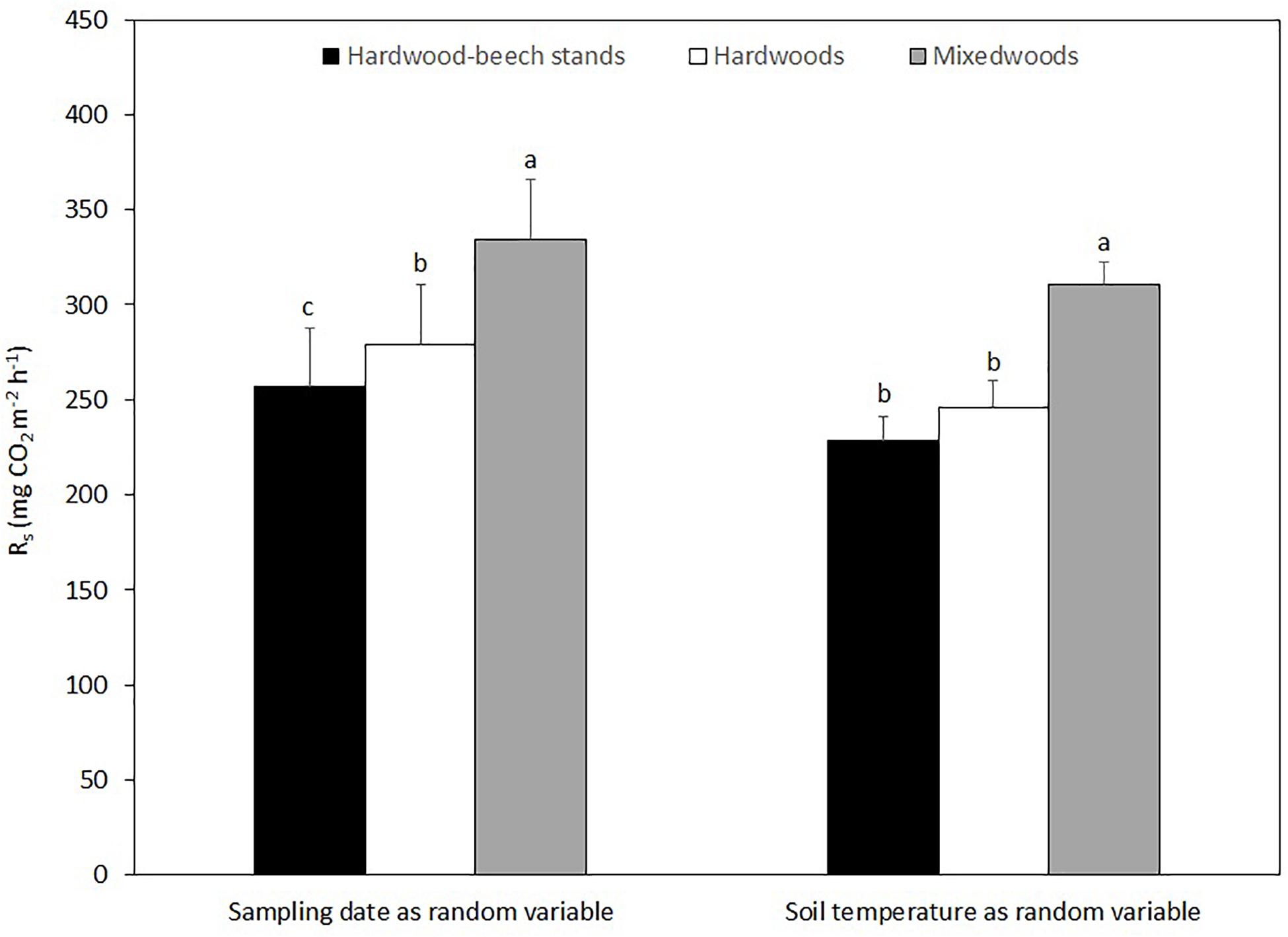
Figure 4. Mean soil respiration rates (Rs) predicted from the linear mixed effect models for each forest type using sampling date and soil temperature as the random variables. Predicted values were back-transformed as a square root transformation was used in both models to assure normality of the residuals. Standard errors are shown. Different letters indicate a significant difference at P < 0.01 between forest types using the Tukey’s honest significant difference test.
Soil temperature exhibited a seasonal variation similar to Rs, increasing from June to July–August and decreasing again to < 10°C in October and < 4°C in November (Supplementary Figure 2). Soils reached higher temperatures in 2020 than in 2019. In 2019, soil water potential reached a maximum in early August (Supplementary Figure 2). In 2020, a series of early heatwaves and drought events explain the unusually high soil temperature and very high water potential recorded in June. Overall, soil water potential in 2020 reached higher values than in 2019. Linear mixed effect models detected significantly lower soil temperature in mixedwoods, followed by hardwood-beech stands, and then hardwoods, whereas soil water potential was significantly lower in hardwood-beech stands, followed by hardwoods and then mixedwoods (Supplementary Figure 2).
Root-free plots were used to estimate Rroot and Rh in the three forest types and their contributions (%) to Rs. Root respiration in mixedwoods was approximately threefold higher than in hardwoods and hardwood-beech stands, whereas Rroot in hardwood-beech stands was 22% higher than in hardwoods (Table 5). The Rh component in mixedwoods and hardwood-beech stands generated a smaller CO2 flux than hardwoods by about 26 and 11%, respectively. Due to the low degrees of freedom of the ANOVAs, however, the only significant difference was found between Rroot of mixedwoods and Rroot of hardwood and hardwood-beech stands. Root respiration contributed about 20% of Rs in hardwoods, 25% in hardwood-beech stands, and 50% in mixedwoods (Table 5). The remaining CO2 flux thus originated from the heterotrophic component. The contributions of Rroot and Rh to Rs were, respectively, significantly lower and higher in hardwoods and hardwood-beech stands than in mixedwoods.
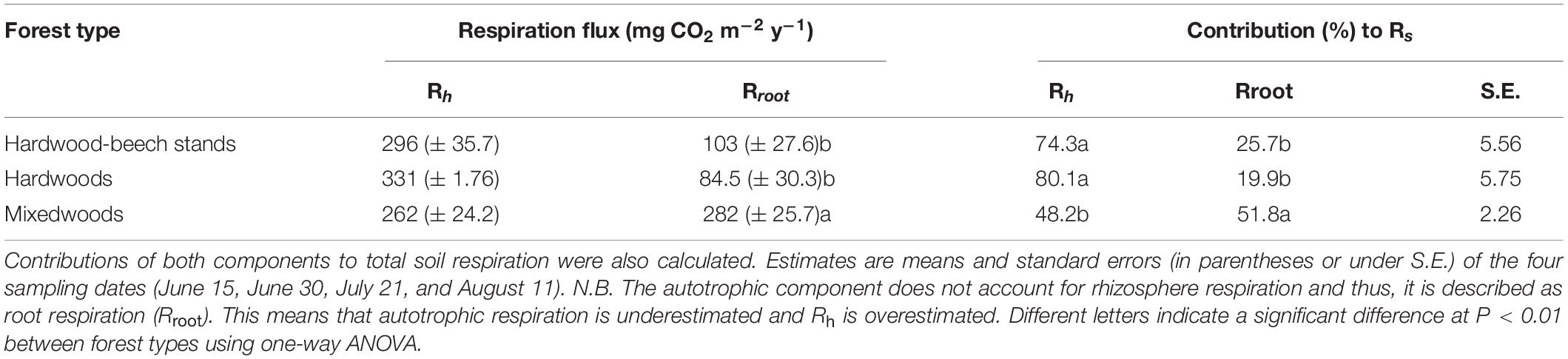
Table 5. Root (Rroot) and heterotrophic (Rh) respiration fluxes from the root exclusion plots in summer 2020.
Discussion
The seasonal variation in soil temperature in this sugar maple forest near the northern distribution limit of temperature deciduous forests was conducive to relatively strong exponential relationships between soil temperature and Rs for all forest types. This result was expected because, at all spatial scales, soil temperature is the main abiotic control of Rs and its associated processes (Kutsch et al., 2009) and the key variable for predicting Rs in the context of climate change (Subke and Bahn, 2010). However, further statistical analyses of our data indicated that, over the 2 years of measurements at SBL, tree species composition has had a substantial influence on Rs as well. First, forest stands where balsam fir contributed at least 20% of basal area (i.e., mixedwoods) significantly increased Rs, whereas forests where American beech contributed at least 20% of litterfall (i.e., hardwood-beech stands) significantly decreased Rs. These results contradict our initial hypothesis that conifers would lower Rs in this typically maple dominated forest, but they validate our hypothesis that beech would lower Rs.
Influence of Mixedwoods on Rs
Most studies that investigated the influence of forest type on Rs were conducted at spatially broad experimental scales and thus emphasized the direct effect of temperature and forest productivity across landscapes instead of forest type per se. Because Rs is largely dependent on the translocation of photosynthates produced aboveground to the roots (Ra component, Högberg et al., 2001), the expected trend is for Rs to increase from colder to warmer forests. As such, meta-analyses show an increase in Rs from boreal to temperate to tropical forests (Raich and Schlesinger, 1992; Subke et al., 2006). However, within a constrained geographical location, an increasing abundance in conifers can also modify the soil system to conditions (e.g., lower temperatures, pH and nutrient availability) that are less suitable for microbial degradation of soil organic matter and leaf litter (Binkley and Giardina, 1998; Binkley and Fisher, 2012; Prescott and Grayston, 2013; Joly et al., 2017). Such conditions would be expected to lower Rh, i.e., a significant component of Rs (25–35%) in temperate deciduous forests (Bowden et al., 1993; Cisneros-Dozal et al., 2007). Therefore, in a side-by-side study of deciduous and coniferous stands with similar photosynthetic rates, it would seem reasonable to find higher Rs under deciduous stands because of the greater organic matter turnover (Raich and Tufekcioglu, 2000; Curiel Yuste et al., 2004; Fahey et al., 2005). At SBL, Collin et al. (2017, 2018) observed more acidic and nutrient-poor soils under mixedwoods and conifer dominated forests than hardwoods and hardwood-beech stands. Furthermore, in a study of four sites in the sugar maple-basswood (Tilia Americana) and sugar maple-yellow birch domains of Quebec, including SBL, Bélanger et al. (2019) found that decomposition rates of sugar maple leaf litter were slower under pure coniferous stands than under hardwood and hardwood-beech stands, and that decomposition rates under mixedwoods were intermediate. We thus hypothesized that the slower leaf litter decomposition in mixedwoods at SBL would lead to lower Rs than hardwoods, but consistently higher Rs under mixedwoods compared to the other forest types contradicts this hypothesis.
In a similar cool temperate climate in Korea, Lee et al. (2010) measured lower Rs under deciduous forests than coniferous forests. They explained that there were greater photosynthetic constraints on leaves of deciduous trees. In turn, this led to limited photosynthate transport and lower root activity and Ra. We do not have reliable dendrochronological data to infer differences in productivity among tree species and forest types at SBL. However, biomass data provided by Maliondo et al. (1990) suggest that balsam fir and white spruce stands produce equivalent or more biomass annually than sugar and red maple stands in northern temperate deciduous forests in eastern Canada. In this respect, mixedwoods at SBL, with balsam fir as the main coniferous species, are expected to perform very well. Conversely, growing conditions are sub-optimal for maple spp. and beech at SBL as they develop near their northern range limit and are supported by acidic and N-poor soils (Collin et al., 2017, 2018). We thus argue that Rs in mixedwood plots were balanced by a greater contribution of Ra relative to hardwoods and hardwood-beech stands. This is well supported by our partitioning analysis of Rroot and Rh. Despite colder and drier soils under mixedwoods, Rroot was substantially and significantly higher (about threefold) in mixedwoods than in the other forest types studied. We also estimated that Rroot in mixedwoods was about 50% of Rs compared to only 20 and 25% in hardwoods and hardwood-beech stands, respectively. Raich and Schlesinger (1992) suggested that correlation between ecosystem productivity and Rs was largely due to a regional/continental effect of climate, whereas Valentini et al. (2000) argued that ecosystem productivity can overshadow the influence of climatic variables on Rs at large spatial scales. Similarly, Reichstein et al. (2003) were able to partition the contributions of climate and vegetation types on Rs, but this was over an array of sites in Europe and America that encompassed a large gradient in soil microclimate and vegetation productivity. In our study, a dummy variable that characterized the mixedwood plots (0) (vs. hardwood-beech and hardwood plots 1) was the first predictor variable in a multiple linear regression of the residuals produced from the exponential model between Rs and soil temperature. We were thus able to separate the large temperature effect on Rs from the vegetation effect reflecting differences in productivity between mixedwoods and deciduous forests at a very small spatial scale.
Raich and Potter (1995) and Fernández-Alonso et al. (2018) reported no difference in Rs between deciduous and coniferous forests. In a Mediterranean ecotone forest of central Spain, Fernández-Alonso et al. (2018) partitioned the contribution of Ra and Rh to Rs and found that deciduous stands had a lower Rh and a greater Ra than coniferous forests, but Rs rates were similar between the two forests. This is an opposite scenario to the one at SBL where fir, not maple spp. or beech, produced higher Rs due to higher Ra. As discussed above, this finding is reasonable given that growing conditions at SBL are more suitable for fir than sugar maple and beech. It thus appear that patterns in Rs between deciduous and coniferous forests under similar growth conditions cannot be easily generalized and that further side-by-side studies are needed to fully elucidate the factors, notably soils and climate, that govern differences in Rs between trees with diverging phylogenetics and physiology.
Influence of Hardwood-Beech Stands on Rs
Based on data extracted from Gosz et al. (1973); Melillo et al. (1982), Côté and Fyles (1994), and Moore et al. (1999), rates of American beech leaf litter decomposition are estimated to fall toward the lower end of the species present at the study sites: birch spp. ≥ red maple, aspen spp. ≥ sugar maple, spruce spp. > white pine > balsam fir, American beech > eastern white cedar. Our results are consistent with this sequence because the Rh component is generally lower when leaf litter at SBL is enriched with beech and fir. However, due to the small sample and variability in the data, differences were not significant. In this respect, the poor quality and low decomposability of beech litter can be associated only in part to the significantly lower Rs under hardwood-beech stands compared to hardwoods where there is no significant addition of beech litter.
Collin et al. (2017, 2018) measured low canopy openness and light transmission under hardwood-beech stands compared to hardwoods and mixedwoods at SBL. For example, canopy openness in July was as low as 10.7% in hardwood-beech stands compared to 23.8 and 30.6% in hardwoods and mixedwoods, respectively. This resulted in light transmission of < 5 mol m–2 d–1 at the soil surface under hardwood-beech stands and > 10 mol m–2 d–1 under the other forest types. Castin-Buchet and Andre (1998) and Kunhamu et al. (2009) suggested that low light transmission can supress microfaunal and microbial activity in the leaf litter by negatively affecting immediate air temperature and relative humidity. At SBL, this is corroborated by significantly lower soil temperature and higher soil water potential in hardwood-beech stands than in other forest types. Differences in soil water potential also appeared to increase during drier periods. In this respect, the lower light transmission in these stands during the full leaf period likely limited overheating of the understory vegetation and soils and in turn, reduced water losses from evapotranspiration. In addition, two results at SBL suggest that differences in Rs were due to the lower soil temperature and/or a different response to changes in soil temperature (i.e., greater sensitivity) under hardwood-beech stands compared to other forest types: (1) mixed model analysis with soil temperature as a random variable detected no difference in Rs between hardwoods and hardwood-beech stands, and (2) the Q10 value produced with data from hardwood-beech stands (2.97) was quite lower than Q10 values produced for hardwoods (2.26) and mixedwoods (2.44). We conclude that low light transmission at the soil surface and low surface soil temperature during the full leaf period were acting in combination with the recalcitrant beech leaf litter in hardwood-beech stands to suppress Rh and Rs relative to hardwoods without beech or with only small proportions of the species in the canopy.
Annual soil respiration rates under four stands dominated by American beech at Hubbard Brook, New Hampshire, were estimated at 629 g C m–2 y–1 compared to 728 g C m–2 y–1 for three stands dominated by either sugar maple or white birch (Fahey et al., 2005). Although there are some differences in the approach to classify the stands between studies, forests at SBL and Hubbard Brook are quite similar in composition and grow on acidic soils. The main difference is that beech is at approximately 250 km south of its distribution at Hubbard Brook, whereas it is very near its northern limit at SBL. Thus, there are likely similar mechanisms associated with the presence of beech that are decreasing Rs rates relative to the other forest types at these sites.
Influence of Soil Water and Magnesium on Rs
Soil water potential was also an important effect on Rs at SBL as it was the second predictor variable in the multiple regression model. The inclusion of soil water potential in the model is consistent with previous findings. Soil temperature, relative to soil moisture, generally exerts a more consistent control on Rs during the growing season in temperate and boreal forests (Maier and Kress, 2000; Gough et al., 2005; Kumpu et al., 2018). However, soil moisture can become an important control of Rs during dry periods, namely by affecting the decomposition of leaf litter and thus Rh (Epron et al., 1999; Fang and Moncrieff, 2001; Cisneros-Dozal et al., 2007; Vogel et al., 2013; Santonja et al., 2015). In 2020, there were two heatwaves recorded before June 21 and the second one affected southern Quebec and SBL for more than 7 days. This is the first time that two heatwaves were recorded to hit Quebec before the summer solstice. An exceptional drought accompanied these heatwaves, with 70% less rain than normal in June. A shorter heatwave hit Quebec in mid-July and then conditions cooled off and precipitation was normal thereafter. This series of events led to unusually high soil temperature and water potential in June, only about 1 month after complete snow melt at the site, and likely explain the inclusion of an indicator of soil water availability in the multiple regression model.
Soil nutrient availability effects on Rs also appeared important at SBL as Mg2+ activity in the soil solution was selected as the third predictor variable in the multiple regression model. More specifically, Mg2+ activity in the soil solution may reflect the benefits of an improved soil acid-base status on decomposer biota and activity (Blagodatskaya and Anderson, 1998; Bååth and Anderson, 2003; Han et al., 2008) under hardwoods. Indeed, 4 years of PRS data (2017–2020) in the plots at SBL suggest significantly higher Ca2+ and Mg2+ activities under hardwoods than under hardwood-beech stands and mixedwoods (Bélanger, unpublished data). Interestingly, soil solution Ca2+ activity, another indicator of alkalinity, was selected as the fourth predictor variable in the model (results not shown). The higher activity of these base cations in the soil solution in hardwoods are likely associated with increased biocycling, especially by the abundant Betula spp. in these plots (Table 1, Bélanger et al., 2004).
Conclusion
We assessed Rs within a sugar maple forest near the northern limit of deciduous temperate forests and where the abundance of conifers and American beech varied. Seasonal variations in soil temperature exerted the largest influence on Rs in these stands, but the results also illustrate how admixtures of balsam fir and beech are affecting Rs in opposite directions. The admixture of fir consistently yielded the highest Rs, whereas the admixture of beech yielded the lowest Rs. The larger Rs in the presence of fir is associated to increased photosynthate transport and root activity, whereas the lower Rs in the presence of beech is due to the creation of a soil microclimate favored by the dense canopy as well as the poor litter quality (low decomposability). Other factors explaining the variation in Rs include low soil moisture during subsequent heatwaves and an improved soil acid-base status due to biocycling by birch trees. Sugar maple was used in this study as the “control” species, but understanding the effects of a change in tree species composition on Rs is relevant to all biogeographic contexts. Such studies will help to better predict future temporal and spatial changes in the biogeochemistry of forest ecosystems, including C pools and atmospheric CO2 concentrations, and their changing trajectories in terms of species composition under climate change (Lafleur et al., 2010; Ettinger and HilleRisLambers, 2013).
Data Availability Statement
The raw data supporting the conclusions of this article will be made available by the authors, without undue reservation.
Author Contributions
NB conceived and designed the experiment and drafted the manuscript as the lead author. AC, RK, and SL-D acquired the data and revised and edited the manuscript. NB and AC analyzed the data. All authors contributed to the interpretation of the findings.
Funding
Financial support was provided through Natural Sciences and Engineering Research Council of Canada Discovery grants (RGPIN 2015-03699 and 2020-04931) and Canada Foundation for Innovation John R. Evans Leaders Fund (35370) and Innovation Fund (36014) grants to NB.
Conflict of Interest
The authors declare that the research was conducted in the absence of any commercial or financial relationships that could be construed as a potential conflict of interest.
Acknowledgments
We wish to acknowledge Charlène Mélançon, Theo Stathopoulos, Anne-Marie Riopel, Dominique Caron, Laurence Grimond, and Justin Bélanger for their help in the field and laboratory. We are also grateful to Mélanie Desrochers for preparing Figure 1. Finally, we thank the staff at SBL for providing access to the site and research facilities.
Supplementary Material
The Supplementary Material for this article can be found online at: https://www.frontiersin.org/articles/10.3389/ffgc.2021.664584/full#supplementary-material
References
Arias-Navarro, C., Diaz-Pines, E., Kiese, R., Rosenstock, T. S., Rufino, M. C., Stern, D., et al. (2013). Gas pooling: a sampling technique to overcome spatial heterogeneity of soil carbon dioxide and nitrous oxide fluxes. Soil Biol. Biochem. 67, 20–23. doi: 10.1016/j.soilbio.2013.08.011
Arii, K., and Lechowicz, M. J. (2002). The influence of overstory trees and abiotic factors on the sapling community in an old-growth Fagus–Acer forest. Ecoscience 9, 386–396. doi: 10.1080/11956860.2002.11682726
Bååth, E., and Anderson, T.-H. (2003). Comparison of soil fungal/bacterial ratios in a pH gradient using physiological and PLFA-based techniques. Soil Biol. Biochem. 35, 955–963. doi: 10.1016/s0038-0717(03)00154-8
Bailey, S. W., Horsley, S. B., Long, R. P., and Hallett, R. A. (2004). Influence of edaphic factors on sugar maple nutrition and health on the Allegheny Plateau. Soil Sci. Soc. Am. J. 68, 243–252. doi: 10.2136/sssaj2004.0243
Bannon, K., Delagrange, S., Bélanger, N., and Messier, C. (2015). American beech and sugar maple sapling relative abundance and growth are not modified by light availability following partial and total canopy disturbances. Can. J. For. Res. 45, 632–638. doi: 10.1139/cjfr-2014-0240
Beckage, B., Osbrone, B., Gavin, D. G., Pucko, C., Siccama, T., and Perkins, T. (2008). A rapid upward shift of a forest ecotone during 40 years of warming in the Green Mountains of Vermont. Proc. Natl. Acad. Sci. U.S.A. 105, 4197–4202. doi: 10.1073/pnas.0708921105
Bélanger, N., Collin, A., Ricard-Piché, J., Kembel, S. W., and Rivest, D. (2019). Microsite conditions govern leaf litter decomposition in the sugar maple bioclimatic domain of Quebec. Biogeochemistry 145, 107–126. doi: 10.1007/s10533-019-00594-1
Bélanger, N., Côté, B., Fyles, J. W., Courchesne, F., and Hendershot, W. H. (2004). Forest regrowth as the controlling factor of soil nutrient availability 75 years after fire in a deciduous forest of southern Quebec. Plant Soil 262, 363–372. doi: 10.1023/b:plso.0000037054.21561.85
Bélanger, N., Holmden, C., Courchesne, F., Côté, J., and Hendershot, W. H. (2012). Constraining soil mineral weathering 87Sr/86Sr for calcium apportionment studies of a deciduous forest growing on soils developed from granitoid igneous rocks. Geoderma 185, 84–96. doi: 10.1016/j.geoderma.2012.03.024
Bilodeau-Gauthier, S., Paré, D., Messier, C., and Bélanger, N. (2013). Root production of hybrid poplars and nitrogen mineralization improve following mounding of boreal Podzols. Can. J. For. Res. 43, 1092–1103. doi: 10.1139/cjfr-2013-0338
Binkley, D., and Giardina, C. (1998). Why do tree species affect soils? The warp and woof of tree-soil interactions. Biogeochemistry 42, 89–106. doi: 10.1007/978-94-017-2691-7_5
Blagodatskaya, E. V., and Anderson, T.-H. (1998). Interactive effects of pH and substrate quality on the fungal-to-bacterial ratio and qCO2 of microbial communities in forest soils. Soil Biol. Biochem. 30, 1269–1274. doi: 10.1016/s0038-0717(98)00050-9
Bohn, K. K., and Nyland, R. D. (2003). Forecasting development of understory American beech after partial cutting in uneven-aged northern hardwood stands. For. Ecol. Manage. 180, 453–461. doi: 10.1016/s0378-1127(02)00614-x
Boivert-Marsh, L., and de Blois, S. (2021). Unravelling potential northward migration pathways for tree species under climate change. J. Biogeogr. [Epub ahead of print]. doi: 10.1111/jbi.14060
Boone, R. D., Nadelhoffer, K. J., Canary, J. D., and Kaye, J. P. (1998). Roots exert a strong influence on the temperature sensitivity of soil respiration. Nature 396, 570–572. doi: 10.1038/25119
Borken, W., Xu, Y.-J., Davidson, E. A., and Beese, F. (2002). Stand and temporal variation of soil respiration in European beech, Norway spruce, and Scots pine forests. Glob. Change Biol. 8, 1205–1216. doi: 10.1046/j.1365-2486.2002.00547.x
Bowden, R. D., Nadelhoffer, K. J., Boone, R. D., Melillo, J. M., and Garrison, J. B. (1993). Contributions of aboveground litter, and root respiration to total soil respiration in a temperate mixed hardwood forest. Can. J. For. Res. 23, 1402–1407. doi: 10.1139/x93-177
Buchholz, T., Friedland, A. J., Hornig, C. E., Keeton, W. S., Zanchi, G., and Nunery, J. (2014). Mineral soil carbon fluxes in forests and implications for carbon balance assessments. Glob. Change Biol. Bioenergy 6, 305–311. doi: 10.1111/gcbb.12044
Castin-Buchet, V., and Andre, P. (1998). The influence of intensive thinning on earthworm populations in the litters of Norway spruce and Douglas fir. Pedobiologia 42, 63–70.
Charney, N. D., Babst, F., Poulter, B., Record, S., Trouet, V. M., Frank, D., et al. (2016). Observed forest sensitivity to climate implies large changes in 21st century North American forest growth. Ecol. Lett. 19, 1119–1128. doi: 10.1111/ele.12650
Chen, I.-C., Hill, J. K., Ohlemüller, R., Roy, D. B., and Thomas, C. D. (2011). Rapid range shifts of species associated with high levels of climate warming. Science 333, 1024–1026. doi: 10.1126/science.1206432
Cisneros-Dozal, L. M., Trumbore, S., and Hanson, P. J. (2006). Partitioning sources of soil-respired CO2 and their seasonal variation using a unique radiocarbon tracer. Glob. Change Biol. 12, 194–204. doi: 10.1111/j.1365-2486.2005.001061.x
Cisneros-Dozal, L. M., Trumbore, S. E., and Hanson, P. J. (2007). Effect of moisture on leaf litter decomposition and its contribution to soil respiration in a temperate forest. J. Geophys. Res. Biogeosci. 12:G01013.
Collin, A., Messier, C., and Bélanger, N. (2017). Conifer presence may negatively affect sugar maple’s ability to migrate into the boreal forest through reduced foliar nutritional status. Ecosystems 20, 701–716. doi: 10.1007/s10021-016-0045-4
Collin, A., Messier, C., Kembel, S. W., and Bélanger, N. (2018). Can sugar maple establish into the boreal forest? Insights from seedlings under various canopies in southern Quebec. Ecosphere 9:e02022.
Côté, B., and Fyles, J. W. (1994). Nutrient concentration and acid-base status of leaf litter of tree species characteristic of the hardwood forest of southern Quebec. Can. J. For. Res. 24, 192–196. doi: 10.1139/x94-027
Curiel Yuste, J., Janssens, I. A., Carrara, A., and Ceulemans, R. (2004). Annual Q10 of soil respiration reflects plant phenological patterns as well as temperature sensitivity. Glob. Change Biol. 10, 161–169. doi: 10.1111/j.1529-8817.2003.00727.x
Davidson, E. A., Belk, E., and Boone, R. D. (1998). Soil water content and temperature as independent or confounded factors controlling soil respiration in a temperate mixed hardwood forest. Glob. Change Biol. 4, 217–227. doi: 10.1046/j.1365-2486.1998.00128.x
Davidson, E. A., and Janssens, Y. A. (2006). Temperature sensitivity of soil carbon decomposition and feedbacks to climate change. Nature 440, 165–173. doi: 10.1038/nature04514
Epron, D., Farque, L., Lucot, E., and Badot, P. M. (1999). Soil CO2 efflux in a beech forest: dependence on soil temperature and soil water content. Ann. For. Sci. 56, 221–226. doi: 10.1051/forest:19990304
Ettinger, A. K., and HilleRisLambers, J. (2013). Climate isn’t everything: competitive interactions and variation by life stage will also affect range shifts in a warming world. Am. J. Bot. 100, 1344–1355. doi: 10.3732/ajb.1200489
Fahey, T. J., Tierney, G. L., Fitzhugh, R. D., Wilson, G. F., and Siccama, T. G. (2005). Soil respiration and soil carbon balance in a northern hardwood forest ecosystem. Can. J. For. Res. 35, 244–253. doi: 10.1139/x04-182
Fang, C., and Moncrieff, J. B. (2001). The dependence of soil CO2 efflux on temperature. Soil Biol. Biochem. 33, 155–165. doi: 10.1016/s0038-0717(00)00125-5
Fernández-Alonso, M. J., Díaz-Pinés, E., Ortiz, C., and Rubio, A. (2018). Disentangling the effects of tree species and microclimate on heterotrophic and autotrophic soil respiration in a Mediterranean ecotone forest. For. Ecol. Manage. 430, 533–544. doi: 10.1016/j.foreco.2018.08.046
Görres, C. M., Kutzbach, L., and Elsgaard, L. (2014). Comparative modeling of annual CO2 flux of temperate peat soils under permanent grassland management. Agric. Ecosyst. Environ. 186, 64–76. doi: 10.1016/j.agee.2014.01.014
Gosz, J. R., Likens, G. E., and Bormann, F. (1973). Nutrient release from decomposing leaf and branch litter in the Hubbard Brook Forest, New Hampshire. Ecol. Monogr. 43, 173–191. doi: 10.2307/1942193
Gough, C. M., Seiler, J. R., Wiseman, P. E., and Maier, C. A. (2005). Soil CO2 efflux in loblolly pine (Pinus taeda L.) plantations on the Virginia Piedmont and South Carolina Plain over a rotation-length chronosequence. Biogeochemistry 73, 127–147. doi: 10.1007/s10533-004-0566-3
Gravel, D., Beaudet, M., and Messier, C. (2010). Large-scale synchrony of gap dynamics and the distribution of understory tree species in maple–beech forests. Oecologia 162, 153–161. doi: 10.1007/s00442-009-1426-6
Gustafson, E. J., and Sturtevant, B. R. (2013). Modeling forest mortality caused by drought stress: implications for climate change. Ecosystems 16, 60–74. doi: 10.1007/s10021-012-9596-1
Han, W., Kemmitt, S. J., and Brookes, P. C. (2008). Soil microbial biomass and activity in Chinese tea gardens of varying stand age and productivity. Soil Biol. Biochem. 39, 1468–1478. doi: 10.1016/j.soilbio.2006.12.029
Hane, E. N. (2003). Indirect effects of beech bark disease on sugar maple seedling survival. Can. J. For. Res. 33, 807–813. doi: 10.1139/x03-008
Hangs, R. D., Greer, K. J., and Sulewski, C. A. (2004). The effect of interspecific competition on conifer seedling growth and nitrogen availability measured using ion-exchange membranes. Can. J. For. Res. 34, 754–761. doi: 10.1139/x03-229
Hanson, P. J., Edwards, N. T., Garten, C. T., and Andrews, J. A. (2000). Separating root and soil microbial contributions to soil respiration: a review of methods and observations. Biogeochemistry 48, 115–146.
Högberg, P., Nordgren, A., Buchmann, N., Taylor, A. F. S., Ekblad, A., Högberg, M. N., et al. (2001). Large-scale forest girdling shows that current photosynthesis drives soil respiration. Nature 411, 789–792. doi: 10.1038/35081058
Iverson, L. I., Peters, M. P., Prasad, A. M., and Matthews, S. N. (2019). Analysis of climate change impacts on tree species of the eastern US: results of DISTRIB-II modeling. Forests 10:302. doi: 10.3390/f10040302
Joly, F.-X., Milcu, A., Scherer-Lorenzen, M., Jean, L.-K., Bussotti, F., Dawud, S. M., et al. (2017). Tree species diversity affects decomposition through modified micro-environmental conditions across European forests. New Phytol. 214, 1281–1293. doi: 10.1111/nph.14452
Kandel, T. P., Lærke, P. E., and Elsgaard, L. (2016). Effect of chamber enclosure time on soil respiration flux: a comparison of linear and non-linear flux calculation methods. Atmos. Environ. 141, 245–254. doi: 10.1016/j.atmosenv.2016.06.062
Kelting, D. L., Burger, J. A., and Edwards, G. S. (1998). Estimating root respiration, microbial respiration in the rhizosphere, and root-free soil respiration in forest soils. Soil Biol. Biochem. 30, 961–968. doi: 10.1016/s0038-0717(97)00186-7
Kumpu, A., Mäkelä, A., Pumpanen, J., Saarinen, J., and Berninger, F. (2018). Soil CO2 efflux in uneven-aged and even-aged Norway spruce stands in southern Finland. iForests 11, 705–712. doi: 10.3832/ifor2658-011
Kunhamu, T. K., Kumar, B. M., and Viswanath, S. (2009). Does thinning affect litterfall, litter decomposition, and associated nutrient release in Acacia mangium stands of Kerala in peninsular India? Can. J. For. Res. 39, 792–801. doi: 10.1139/x09-008
Kurz, W. A., and Apps, M. J. (1999). A 70-year retrospective analysis of carbon fluxes in the Canadaian forests sector. Ecol. Appl. 9, 526–547. doi: 10.1890/1051-0761(1999)009[0526:ayraoc]2.0.co;2
Kutsch, W. L., Bahn, M., and Heinemeyer, A. (2009). Soil Carbon Dynamics. Cambridge: Cambridge University Press.
Kutzbach, L., Schneider, J., Sachs, T., Giebels, M., Nykänen, H., Shurpali, N. J., et al. (2007). CO2 flux determination by closed-chamber methods can be seriously biased by innapropriate application of linear regression. Biogeosciences 4, 1005–1025. doi: 10.5194/bg-4-1005-2007
Lafleur, B., Paré, D., Munson, A. D., and Bergeron, Y. (2010). Response of northeastern North American forests to climate change: will soil conditions constrain tree species migration? Environ. Rev. 18, 279–289. doi: 10.1139/a10-013
Lajeunesse, D. (1990). Le système racinaire de quelques érablières du Québec. [master’s thesis]. Montréal, QC: McGill University.
Lavigne, M. B., Foster, R. L., and Goodine, G. (2004). Seasonal and annual changes in soil respiration in relation to soil temperature, water potential and trenching. Tree Physiol. 24, 415–424. doi: 10.1093/treephys/24.4.415
Lee, N.-Y., Koo, J.-W., No, N. J., Kim, J., and Son, Y. (2010). Autotrophic and heterotrophic respiration in needle fir and Quercus-dominated stands in a cool-temperate forest, central Korea. J. Plant Res. 123, 485–495. doi: 10.1007/s10265-010-0316-7
Long, R. P., Horsley, S. B., Hallett, R. A., and Bailey, S. W. (2009). Sugar maple growth in relation to nutrition and stress in the northeastern United States. Ecol. Appl. 19, 1454–1466. doi: 10.1890/08-1535.1
Maier, C. A., and Kress, L. W. (2000). Soil CO2 evolution and root respiration in 11 year-old loblolly pine (Pinus taeda) plantations as affected by moisture and nutrient availability. Can. J. For. 30, 347–359. doi: 10.1139/x99-218
Maliondo, S. M., Mahendrappa, M. K., and van Raalte, G. D. (1990). Distribution of Biomass and Nutrients in Some New Brunswick Forest Stands: Possible Implications of Whole-Tree Harvesting. Ottawa: Forestry Canada.
Melillo, J. M., Aber, J. D., and Muratore, J. F. (1982). Nitrogen and lignin control of hardwood leaf litter decomposition dynamics. Ecology 63, 621–626. doi: 10.2307/1936780
Moore, T. R., Trofymow, J. A., Taylor, B., Prescott, C., Camiré, C., Duschene, L., et al. (1999). Rates of litter decomposition in Canadian forests. Glob. Change Biol. 5, 75–82.
Moyano, F. E., Vasilyeva, N., Bouckaert, L., Cook, F., Craine, J., Curiel Yuste, J., et al. (2012). The moisture response of soil heterotrophic respiration: interaction with soil properties. Biogeosciences 9, 1173–1182. doi: 10.5194/bg-9-1173-2012
Nolet, P., Bouffard, D., Doyon, F., and Delagrange, S. (2008). Relationship between canopy disturbance history and current sapling density of Fagus grandifolia and Acer saccharum in a northern hardwood landscape. Can. J. For. Res. 38, 216–225. doi: 10.1139/x07-160
Nolet, P., and Kneeshaw, D. (2018). Extreme events and subtle ecological effects: lessons from a long-term sugar maple-American beech comparison. Ecosphere 9:e02336. doi: 10.1002/ecs2.2336
Nyland, D. R., Bashant, A. L., Bohn, K. K., and Verostek, J. M. (2006). Interference to hardwood regeneration in northeastern North America: controlling effects of American beech, striped maple, and hobblebush. N. J. Appl. For. 23, 122–132. doi: 10.1093/njaf/23.2.122
Pedersen, A. R., Petersen, S. O., and Schelde, K. (2010). A comprehensive approach to soil-atmosphere trace-gas flux estimation with static chambers. Eur. J. Soil Sci. 61, 888–902. doi: 10.1111/j.1365-2389.2010.01291.x
Pregitzer, S., and Euskirchen, E. S. (2004). Carbon cycling and storage in world forests: biome patterns related to forest age. Glob. Change Biol. 10, 2052–2077. doi: 10.1111/j.1365-2486.2004.00866.x
Prescott, C. E., and Grayston, S. J. (2013). Tree species influence on microbial communities in litter and soil: current knowledge and research needs. For. Ecol. Manage. 309, 19–27. doi: 10.1016/j.foreco.2013.02.034
Prévost-Bourré, N. C., Soudani, K., Damesin, C., Berveiller, D., Lata, J.-C., and Dufrêne, E. (2010). Increase in aboveground fresh litter quantity over-stimulates soil respiration in a temperate deciduous forest. Appl. Soil Ecol. 46, 26–34. doi: 10.1016/j.apsoil.2010.06.004
Raich, J. W., and Potter, C. S. (1995). Global patterns of carbon dioxide emissions from soils. Glob. Biogeochem. Cycles 9, 23–36. doi: 10.1029/94gb02723
Raich, J. W., Potter, C. S., and Bhagawati, D. (2002). Interannual variability in global soil respiration: 1980-1994. Glob. Change Biol. 8, 800–812. doi: 10.1046/j.1365-2486.2002.00511.x
Raich, J. W., and Schlesinger, W. H. (1992). The global carbon dioxide flux in soil respiration and its relationship to vegetation and climate. Tellus 44B, 81–99. doi: 10.1034/j.1600-0889.1992.t01-1-00001.x
Raich, J. W., and Tufekcioglu, A. (2000). Vegetation and soil respiration: correlations and controls. Biogeochemistry 48, 71–90.
Régnière, J., and Bolstad, P. (1994). Statistical simulation of daily air temperature patterns eastern North America to forecast seasonal events in insect pest management. Environ. Entomol. 23, 1368–1380. doi: 10.1093/ee/23.6.1368
Reichstein, M., Rey, A., Freibauer, A., Tenhunen, J., Valentini, R., Banza, J., et al. (2003). Modeling temporal and large-scale spatial variability of soil respiration from soil water availability, temperature and vegetation productivity indices. Glob. Biogeochem. Cycles 4:1104.
Reiners, W. A. (1968). Carbon dioxide evolution from the floor of three Minnesota forests. Ecology 49, 471–483. doi: 10.2307/1934114
Rochette, P., and Bertrand, N. (2007). “Soil-surface gas emissions,” in Soil Sampling and Methods of Analysis, eds M. R. Carter and E. G. Gregorich (Boca Raton, FL: CRC Press), 851–861.
Rosenzweig, C., Karoly, D., Vicarelli, M., Neofotis, P., Wu, Q., Casassa, G., et al. (2008). Attributing physical and biological impacts to anthropogenic climate change. Nature 453, 353–357. doi: 10.1038/nature06937
Santonja, M., Fernandez, C., Gauquelin, T., and Baldy, V. (2015). Climate change effects on litter decomposition: intensive drought leads to a strong decrease of litter mixture interactions. Plant Soil 393, 69–82. doi: 10.1007/s11104-015-2471-z
Saucier, J.-P., Grondin, P., Robitaille, A., Gosselin, J., Morneau, C., Richard, P. J. H., et al. (2009a). “Écologie forestière,” in Manuel de Foresterie, 2ième édition, ed. Ordre des ingénieurs forestiers du Québec (Québec, QC: MultiMondes), 165–315.
Saucier, J.-P., Robitaille, A., and Grondin, P. (2009b). “Cadre bioclimatique du Québec,” in Manuel de Foresterie, 2ième édition, ed. Ordre des ingénieurs forestiers du Québec (Québec, QC: MultiMondes), 186–205.
Savage, C. (2001). Recolonisation Forestière Dans les Basses Laurentides au Sud du Domaine Climacique de L’érablière à Bouleau Jaune. [master’s thesis]. Montréal, QC: Université de Montréal.
Schlesinger, W. H., and Andrews, J. A. (2000). Soil respiration and the global carbon cycle. Biogeochemistry 48, 7–20.
Sittaro, F., Paquette, A., Messier, C., and Nock, C. A. (2017). Tree range expansion in eastern North America fails to keep pace with climate warming at northern range limits. Glob. Change Biol. 23, 3292–3301. doi: 10.1111/gcb.13622
Soil Classification Working Group (1998). The Canadian System of Soil Classification, Third edition. Ottawa: Agriculture and Agri-Food Canada Publication and NRC Research Press.
Stinson, G., Kurz, W. A., Smyth, C. E., Neilson, E. T., Dymond, C. C., Metsaranta, J. M., et al. (2011). An inventory-based analysis of Canada’s managed forest carbon dynamics, 1990-2008. Glob. Change Biol. 17, 2227–2244. doi: 10.1111/j.1365-2486.2010.02369.x
Subke, J.-A., and Bahn, M. (2010). On the ‘temperature sensitivity’ of soil respiration: can we use the immeasurable to predict the unknown? Soil Biol. Biochem. 42, 1653–1656. doi: 10.1016/j.soilbio.2010.05.026
Subke, J.-A., Inglima, I., and Cotrufo, M. F. (2006). Trends and methodological impacts in soil CO2 efflux partitioning: a metaanalytical review. Glob. Change Biol. 12, 921–943. doi: 10.1111/j.1365-2486.2006.01117.x
Tewary, C. K., Pandey, U., and Singh, J. S. (1982). Soil and litter respiration rates in different microhabitats of a mixed oak-conifer forest and their control by edaphic conditions and substrate quality. Plant Soil 65, 233–238. doi: 10.1007/bf02374653
Valentini, R., Matteucci, G., Dolman, A. J., Schulze, E.-D., Rebmann, C., Moors, E. J., et al. (2000). Respiration as the main determinant of carbon balance in European forests. Nature 404, 861–865. doi: 10.1038/35009084
Vogel, A., Eisenhauer, N., Weigelt, A., and Scherer-Lorenzen, M. (2013). Plant diversity does not buffer drought effects on early-stage litter mass loss rates and microbial properties. Glob. Change Biol. 19, 2795–2803. doi: 10.1111/gcb.12225
Weber, M. G. (1985). Forest soil respiration in eastern Ontario jack pine ecosystems. Can. J. For. Res. 15, 1069–1073. doi: 10.1139/x85-174
Keywords: cool temperate forests, tree species composition, CO2 efflux, soil respiration, root respiration, litter quality, soil water
Citation: Bélanger N, Collin A, Khlifa R and Lebel-Desrosiers S (2021) Balsam Fir and American Beech Influence Soil Respiration Rates in Opposite Directions in a Sugar Maple Forest Near Its Northern Range Limit. Front. For. Glob. Change 4:664584. doi: 10.3389/ffgc.2021.664584
Received: 05 February 2021; Accepted: 21 April 2021;
Published: 14 May 2021.
Edited by:
Jérôme Laganière, Canadian Forest Service, CanadaReviewed by:
Antra Boca, Latvia University of Agriculture, LatviaRichard Drew Bowden, Allegheny College, United States
Copyright © 2021 Bélanger, Collin, Khlifa and Lebel-Desrosiers. This is an open-access article distributed under the terms of the Creative Commons Attribution License (CC BY). The use, distribution or reproduction in other forums is permitted, provided the original author(s) and the copyright owner(s) are credited and that the original publication in this journal is cited, in accordance with accepted academic practice. No use, distribution or reproduction is permitted which does not comply with these terms.
*Correspondence: Nicolas Bélanger, bmljb2xhcy5iZWxhbmdlckB0ZWx1cS5jYQ==