- 1Applied Coastal Research Laboratory, Georgia Southern University, Savannah, GA, United States
- 2Department of Geology and Geography, Georgia Southern University, Savannah, GA, United States
- 3Department of Earth and Environmental Sciences, University of Minnesota Duluth, Duluth, MN, United States
- 4Department of Forest Utilization Engineering and Forest Technology, University of Agriculture in Krakow, Kraków, Poland
To date, the perspective of forest ecohydrologists has heavily focused on leaf-water interactions – leaving the ecohydrological roles of bark under-studied, oversimplified, or omitted from the forest water cycle. Of course, the lack of study, oversimplification, or omission of processes is not inherently problematic to advancing ecohydrological theory or operational practice. Thus, this perspective outlines the relevance of bark-water interactions to advancing ecohydrological theory and practice: (i) across scales (by briefly examining the geography of bark); (ii) across ecosystem compartments (i.e., living and dead bark on canopies, stems, and in litter layers); and, thereby, (iii) across all major hydrologic states and fluxes in forests (providing estimates and contexts where available in the scant literature). The relevance of bark-water interactions to biogeochemical aspects of forest ecosystems is also highlighted, like canopy-soil nutrient exchanges and soil properties. We conclude that a broad ecohydrological perspective of bark-water interactions is currently merited.
Introduction
Woody plants are some of Earth’s tallest, largest (in terms of mass), and longest-lived organisms. Materials derived from woody plants literally provided the structural support for human development worldwide (Fernow, 1913), continue to do so (Westoby, 1989; Wiersum, 1995), and are critical variables in plans to combat and cope with climate change (Pinkard et al., 2015). Woody plants owe their ecological achievements and societal importance, in part, to an anatomical interface between the external world and their internal stem tissues, called “bark.” Despite generally being <15% of total stem volume (c.f., Rosell et al., 2017), bark protects plants from disturbances like fire (Pausas, 2015) and insects (Ferrenberg and Mitton, 2014), can contribute significantly to stem mechanics (Rosell and Olson, 2014), and plays key roles in stem damage recovery (Romero et al., 2009).
Besides these plant physiological roles, bark surfaces engage in profound passive interactions with other biotic and abiotic variables. The porous outer bark layer can host a diverse microbial community (Magyar, 2008; Lambais et al., 2014) as well as an abundant epiphyte assemblage (Van Stan and Pypker, 2015) which is capable of hosting its own extensive microbial community (Anderson, 2014). Bark surface structure also impacts invertebrate communities, for example: affecting resource discovery time for arboreal ants (Yanoviak et al., 2017) and acting as an environmental filter for total invertebrate communities on dead trees (Zuo et al., 2016). These passive biotic roles of bark, to some extent, hinge on bark’s hydrologic functions. Indeed, the water dynamics that support and control life on bark surfaces are a product of that surface’s hygroscopic interactions with water vapor (Kapur and Narayanamurti, 1934; Ilek et al., 2017, 2021), absorption and chemical exchange with liquid precipitation (Voigt and Zwolinski, 1964; Levia and Herwitz, 2005), evaporation response to micrometeorological conditions (Van Stan et al., 2017a), and its melt-related (e.g., albedo) and adhesive properties with regard to ice precipitation (Levia and Underwood, 2004; Roesch and Roeckner, 2006). Of these processes, particularly little research has focused on the ability of bark to absorb and retain water vapor (hygroscopic) despite its strong interconnection to the other bark biogeochemical functions.
Generally, the forest ecohydrological research community has focused on leaf-water interactions to date (e.g., Novick et al., 2019; Holder, 2020). As a result, hydrologic processes operating in and on bark are currently underrepresented (both in magnitude and in parametrization) by land surface models. This may be due to the lack of experimental work on the subject to date, since little-to-no data exist regarding many apparent bark surface and internal properties hypothesized to influence its water storage states, as well as its drainage and evaporative fluxes. The breadth of unobserved properties and unexamined processes within bark-water interactions yield a diversity of research opportunities that may improve land surface and climate models and their applications. To initiate a broader discussion of these opportunities, here we describe a perspective of bark-water interactions that illustrates the broad geographic (spatiotemporal) extent of bark and discuss its connections to ecohydrological processes across forest ecosystem states and fluxes.
A Brief Look at the Geography of Bark
Bark is both spatially expansive and temporally persistent and, therefore, its interactions with the hydrologic cycle may be as well. Regarding the spatial extent, if we estimate bark’s global surface area from the same type of land surface model input data used to estimate global leaf surface area (i.e., Vorholt, 2012), then the bark surface is nearly as large as the Asian continent, ∼41 million km2 (Van Stan et al., 2020). The surface area of this “bark continent” is likely an underestimate as it is based on stem area index (SAI) of standing plants (Figures 1a,b), which does not include the added surface area due to bark surface structural complexity or due to bark on fallen woody debris – see Fang et al. (2019), and references therein, regarding SAI estimation methods. These different bark compartments (standing live, standing dead, and fallen debris) would likely have different effects on ecosystem water fluxes. Across these compartments, the additional surface area due to bark microrelief will hypothetically vary across species. For example, an embryonic step toward estimating the additional surface area due to bark microrelief on live stems is achievable using LaserBark scans (a high-resolution stem lidar system: Van Stan et al., 2010; see Supplementary Section 1). This analysis suggests that there is negligible additional bark surface area in the case of smooth-barked trees, like Fagus sylvatica; however, moderately rough bark (e.g., Pinus contorta) and deeply furrowed bark (e.g., Quercus robur) can add ∼10% and ∼16% to bark surface area, respectively, when compared with a fitted circular circumference (Supplementary Figure 1). Although only supported by a relatively small set of high-resolution lidar scans, woody surface area approximations that do not account for bark microrelief may meaningfully underestimate the surface area of this continent of bark.
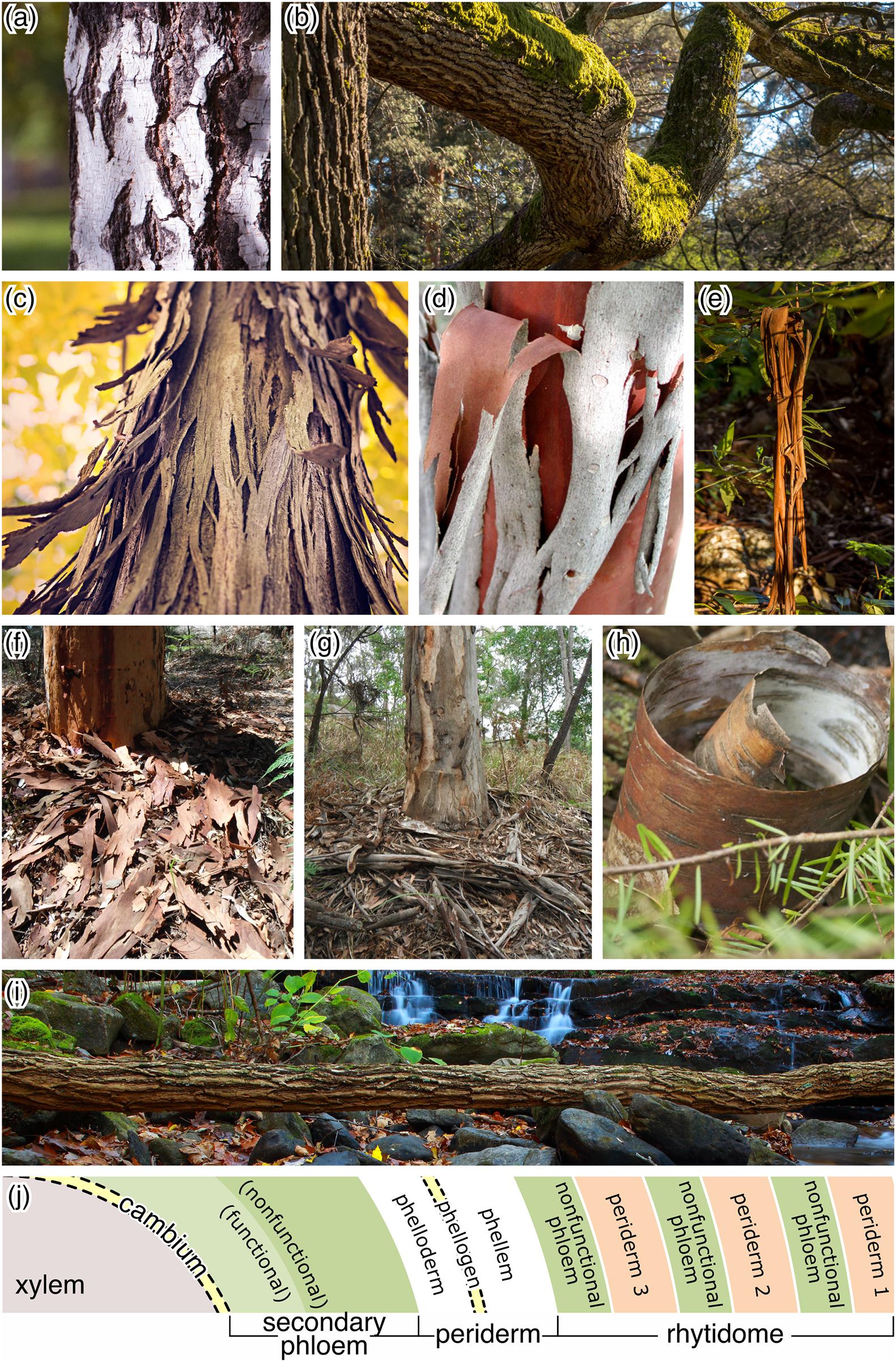
Figure 1. Bark is present throughout a forest – beyond tree (a) stems and (b) branches. Bark shedding can (c,d) temporarily increase on-stem bark surface area, (e) release peelings that become trapped in understory canopies, and (f–h) cover the forest floor. Bark must also be broken down on (i) fallen dead trees. (j) Anatomy of the inner and outer bark. All photographs from open source databases (e.g., Pixabay, Wikimedia Commons) unless credited otherwise. Credits: (a) Tatyana Fyodorova; (b) Thomas B. Didgeman; (c) Ryan McGuire; (d) Niel Sperry; (e) Sandid (Pixabay); (f,g) permission from Saskia Grootemaat; (h) Justin Leonard; (i) Troy Lilly.
This estimate of global bark surface area appears small compared to the ∼1 billion km2 estimate of global leaf surface area (Vorholt, 2012); however, unlike many leaf surfaces, the bark surface is present across all seasons. Plant phenology can include bark shedding events (Borger, 1973); however, these events differ meaningfully from leaf shedding events. Most notably, bark shedding temporarily increases surface area for external bark-water interactions (due to the flaking; Figures 1c,d) and it results in bark becoming present not just in the tree canopy (i.e., new bark), but in the understory (i.e., trapped sheddings; Figure 1e) and litter layer (i.e., bark litter; Figures 1f,g). Based on the few measurements to date, bark litter decomposes much slower than leaf litter (Grootemaat et al., 2017) – with decomposition half-lives ranging from 4.9 to 9.4 years in the litter layer (Johnson et al., 2014). Thus, a brief look at the geography of bark reveals that it can be present in wooded watersheds all year round, on live and dead plants, from the canopy top to the litter below.
The Anatomy of Bark-Water Interactions
“Bark” is anatomically defined as all stem tissues outside of the vascular cambium (Figure 1f). More specifically, bark consists of the functional and non-functional phloem (produced by the vascular cambium), and either a single periderm (for some species) or alternating layers of old periderm and phloem [for species that have successive periderms, called the rhytidome (Evert, 2006; Figure 1f)]. The periderm is made up of three types of tissues – the phellogen, or cork cambium, is a radial meristem that produces phelloderm to the inside, and phellem, or cork, to the outside. These bark anatomical layers are often grouped into “inner” and “outer” bark functional layers; however, the distinction between these functional layers can change across studies. A useful distinction for ecologists and hydrologists is clearly one which separates the metabolically active and inactive (i.e., living vs. dead) tissues into the inner bark and outer bark, respectively. Using this distinction, the collection of tissues between the most recent layers of phloem and the most recently produced phelloderm may be classified as the living “inner bark,” while the phellem (in species with one periderm) or the rhytidome (in species with multiple periderms) is the “outer bark” (Kramer and Kozlowski, 1960). Although the ontogeny of bark is poorly understood (Rosell and Olson, 2007, 2014), in general, the living part of a plant’s periderm, its cellular structure, and its relationship with environmental and growth factors interact to control the outer characteristics of a tree’s bark and can result in a wide array of bark structural properties (see examples in Figure 1). For smooth-barked trees such as Fagus grandifolia or Populus tremuloides, a single periderm may persist without rupturing for long periods of time or the entirety of the plant’s life. For rough-barked trees, species with a single periderm can rupture as the plant grows, or the first (of successive) periderm can rupture as the plant grows and significant tangential tensile stress is placed on the bark. These ruptured, successive periderms may exfoliate over time for some species; for others, they may persist and develop rough and thick bark. The physical structure of the inner and outer bark layers contain biochemical components (e.g., resin and suberin) that can interact to alter these interfaces’ interactions with internal and external waters. Since bark is present in and below the forest canopy, it theoretically could interact with the forest water and energy budget at several points (Figure 2). Additionally, because bark can exist in litter, on coarse debris, and in the canopy, it has multiple opportunities to interact (both internally and externally) with different hydrological fluxes.
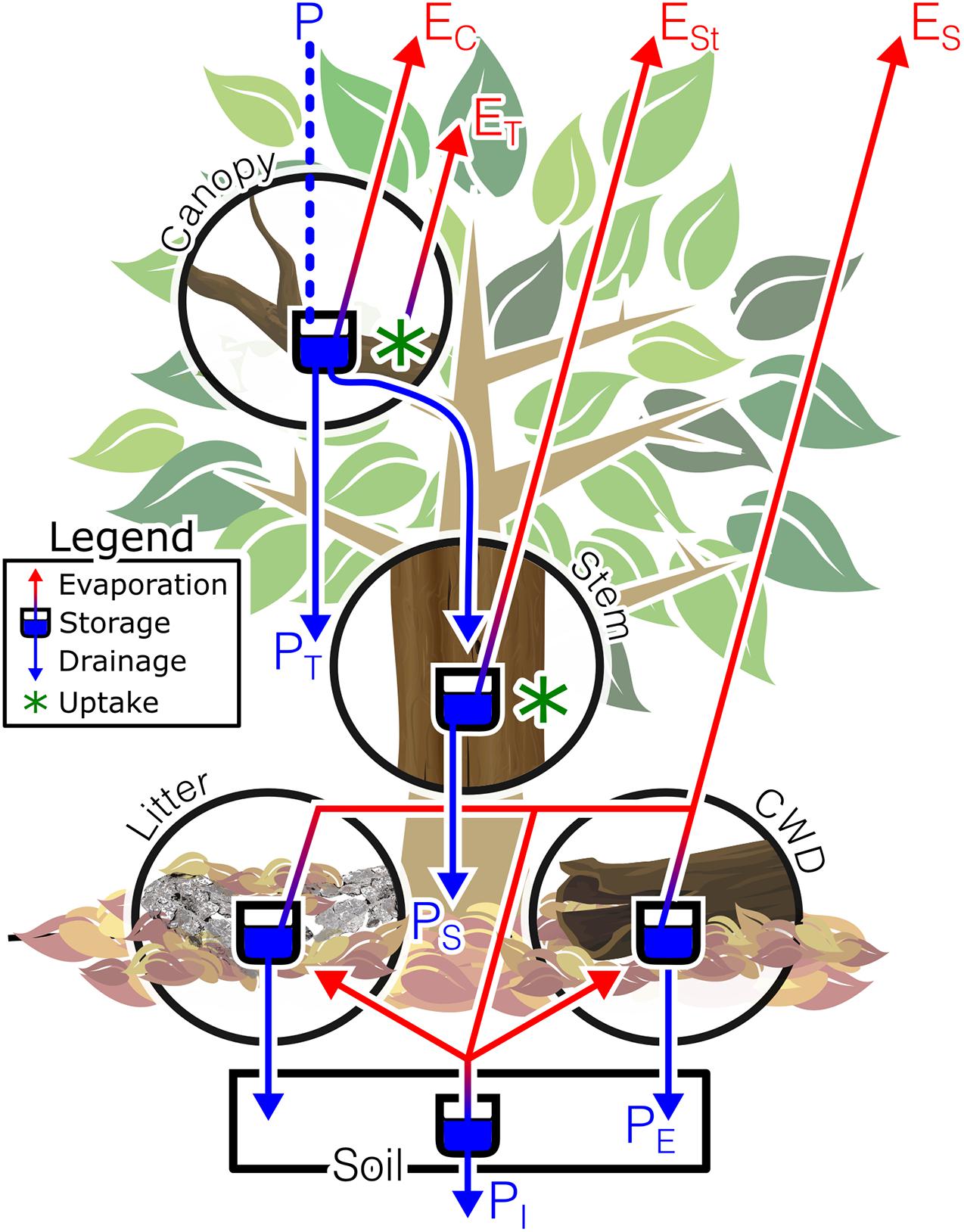
Figure 2. The forest water cycle and opportunities for bark-water interactions. Incoming precipitation (P) can be stored and evaporated at various points, from the bark on the canopy (EC), stem (ESt) and in the litter (including coarse woody debris, CWD), and soil surface (ES). In the canopy and on the stem, bark-water interactions can result in uptake and a portion of this uptaken water may be transpired (ET). Precipitation will also interact with canopy bark as it drains as throughfall (PT), stemflow (PS); litter bark and CWD before becoming effective precipitation (PE) and infiltration (PI).
Of course, each bark functional layer can interact with the water cycle. Starting from the outside-in, the outer bark passively intercepts water during storms. The interception of rainfall by the outer bark can be significant, accounting for 50–80% of the forest canopy’s total water storage capacity (depending on storm conditions) at an Australian tropical rainforest, even for smooth-barked trees (Herwitz, 1985). The evaporation of intercepted precipitation from the outer bark is rarely quantified, and generally has been assumed to be trivial: <2% of total canopy interception-related evaporation (Linhoss and Siegert, 2016). However, in an urban tree row, evaporation from the outer bark represented a non-trivial portion of canopy evaporation: up to 40% (Van Stan et al., 2017a). For solid precipitation, outer bark microrelief has long been known to enable the adhesion of large ice deposits to branches, stems, and exposed tree roots (Klamerus-Iwan et al., 2020a) – even creating “champignons de neige” (snow mushrooms) on the bark of stumps (see photographs 2–6 in Salamin, 1959). Interestingly, Salamin (1959) discusses the effect of outer bark roughness on the mode of snow interception, saying that “on the trunks of trees with smooth bark, it is deposited in a narrow band [whereas] on trunks with very rough bark, it is stored in a heap with great adhesive power1.” Intercepted snow may remain on the bark until air temperatures warm (inducing melt) or a mechanical force (like wind) redistributes it; however, the outer bark’s albedo can play a role in initiating snowmelt in the canopy even under freezing conditions (Levia and Underwood, 2004). These same bark structures interact with draining precipitation waters, affecting the timing and spatial distribution of throughfall and stemflow fluxes (Pypker et al., 2011).
Between storms, the outer bark passively exchanges water vapor with the atmosphere (i.e., hygroscopically). The hygroscopic response of the outer bark can be observable daily, and has fascinated scientists since its first reported observation by Gregor Kraus in 1877 (Haasis, 1934). This hygroscopic response can vary widely across tree species (0.5–1.5 mm per 1 cm of thickness), depending on the outer bark’s bulk density and porosity (Ilek et al., 2017, 2021). How physicochemical properties affect hygroscopicity of the outer bark remains little researched, especially under natural conditions; however, Ilek et al. (2017) found that 10–30% of the outer bark’s water storage capacity could be filled hygroscopically for several species representing a range of common European coniferous and deciduous trees in a temperate continental climate – but, this contribution exceeded 60%, at times, for a humid forest site (Ilek et al., 2021). Hypothetically, the hygroscopic (partial) filling of outer bark pore space may explain some of the variability in canopy water storage capacity between storms. If the outer bark is wet enough, some tree species have been observed to uptake rhytidome-stored water internally (e.g., Katz et al., 1989; Mayr et al., 2014; Earles et al., 2016). While bark is not typically considered in estimates of plant water-use or water redistribution, it has been shown that bark can both take up and lose water across a variety of species (Wittmann and Pfanz, 2008; Earles et al., 2016; Wolfe, 2020). The physical path that intercepted (or hygroscopic) rhytidome water follows into the inner bark may be through non-suberized areas of the phellem cell walls, which can be hydrophilic (Earles et al., 2016). From there, a strong osmotic gradient may draw this intercepted water into the xylem (Zwieniecki and Holbrook, 2009). Water can also move from bark rays into the xylem – a route previously called an “undervalued route of water transport” (e.g., van Bel, 1990; Pfautsch et al., 2015).
As a result of these processes, the inner bark also hosts a dynamic reservoir of water storage (Srivastava, 1964). A study of 90 species across a range of woody ecosystems found that inner bark water storage can account for a non-trivial portion, 17–76%, of the total stem water storage (Rosell et al., 2014). For coastal redwood trees (Sequoia sempervirens), the saturated bark is thought to increase xylem hydraulic conductivity through refilling of tracheid cells (Earles et al., 2016). Similar movement of water between the bark and inner xylem cells has been observed for white spruce (Picea glauca) as well (Katz et al., 1989). While the percent contribution of bark water to total tree transpiration may be relatively small compared to the supply from soils during stress-free periods, this contribution may become significant during periods of drought. For woody species in dryland or seasonally dry ecosystems, bark water storage could represent a crucial buffer against daily-to-seasonal scale changes in water availability (Scholz et al., 2007; Rosell and Olson, 2014), where phloem rays could transport water from the inner bark to the xylem (Pfautsch et al., 2015). Bark water vapor conductance in eight tropical tree species was correlated with higher degrees of stem water deficit and mortality in both natural and greenhouse conditions (Wolfe, 2020). Bark-water interactions (i.e., bark hydraulics) such as these may be scaled via parametrization into tree-scale water flow-storage models (e.g., Steppe and Lemeur, 2007; Mencuccini et al., 2013; Chan et al., 2016) – a future effort along this vein could provide a pathway for first-order estimates of impacts on water states and fluxes at the regional and watershed scales. Altogether, such findings indicate that there is hydrologic connectivity between bark and xylem, the area of stems that carry out water transportation, and that more research is needed to quantify bark-water-uptake contributions to overall plant water use.
After the Bark Departs: Off-The-Tree Opportunities to Influence Forest Hydrology
The bark can depart from the canopy and enter the litter layer during seasonal shedding events or episodic disturbances, like branch breakage or tree throw. In forests where seasonal bark shedding occurs (Figure 1), it can compose ∼20–50% of the litter layer (McColl, 1966; Woods and Raison, 1983; Lamb, 1985; Van Stan et al., 2017b). Scant data exist to estimate the contribution of bark sheddings to litter water storage and evaporation. The thin bark sheddings of Pinus elliottii, composing 18% of the litter layer, could account for ∼10–30% of the litter water storage capacity, reducing throughfall reaching soils (Van Stan et al., 2017b). Recent work finds that the litter layer’s water storage dynamics can also be considerably influenced from below, by intercepting soil vapor flux during wet-to-dry transitions (Zhao et al., 2021). Hypothetically, the unique structure of bark sheddings (i.e., hydrophobic strips that cover wide areas relative to a leaf; Figures 1f,g) could aid the litter layer to intercept soil vapor fluxes. Of course, there are much larger bark-covered impediments to inputs from above (throughfall) and below (soil vapor) in forest litter: coarse woody debris (CWD). The specific influence of bark on the water balance of CWD has not, to the authors’ knowledge, been assessed; however, recent work on deadwood found that the least decomposed wood samples (those with “fragmented bark”) had the lowest initial water absorbability, highest water repellency and, as a result, the lowest storage capacity compared to samples without bark (Błońska et al., 2018; Klamerus-Iwan et al., 2020b). This is not to say that the localized water storage capacity of CWD is negligible. For example, logs with in-tact bark from four common tree species in the H.J. Andrews Experimental Forest (OR, United States), were observed to store and evaporate up to 60% of throughfall (Sexton and Harmon, 2009). Sexton and Harmon (2009) noted that the bark surface could repel 3–29% of rainfall as runoff from the logs. Clearly there are many open, fundamental questions related to bark’s hydrological interactions after its departure from the canopy. Perhaps most fundamentally, how do bark hydrological traits significantly differ when on a living tree vs. coarse woody debris vs. shed bark flakes? And, if bark hydrological traits do differ between these states, is it relevant to hydrological processes?
Bark-Water Interactions and Biogeochemistry
The bark-water interactions discussed above have biogeochemical impacts, both external and internal to the plant. Externally, bark can exchange both inorganic and organic solutes with the comparatively dilute precipitation waters as they drain to the surface as throughfall and, even more so, as stemflow. Solute uptake, leaching, wash-off and transformation during bark-water interactions have all been reported (Katz et al., 1989; Tobón et al., 2004; Gaige et al., 2007; Hofhansl et al., 2012). Leaching of ionic solutes, especially K+, Mg2+, and Ca2+, from bark to draining precipitation waters has been reported in past work (Levia and Herwitz, 2005; Hofhansl et al., 2012). Bark uptake of NH4+ and NO3– from net precipitation fluxes has also been reported (Parker, 1983; Crockford et al., 1996; André et al., 2008). Biochemical transformations have also been reported for water-bark interactions, for example, the optical characteristics of dissolved organic matter in stemflow appears to change with increasing bark residence time (Van Stan et al., 2017c). Bark surfaces can also be excellent traps for coarse particles (Xu et al., 2019), including fungal spores (Magyar, 2008), nutrient-rich pollen (Groenman-van Waateringe, 1998), and pollutants (Catinon et al., 2009) – all of which can be scoured and transported to the surface by branchflow and stemflow (Ponette-González et al., 2020). For vegetation residing on bark – corticolous lichen and bryophytes, for example – studies have found strong relationships with bark water storage and the nutrient content of bark leachates (i.e., stemflow) (Farmer et al., 1991; McGee et al., 2019). Internally, bark water uptake and carbohydrate storage may interact to enable embolism repair (Nardini et al., 2011; Pfautsch et al., 2015; Rosell, 2019). Indeed, recent research finds that (non-structural) carbohydrate storage in the inner bark (for 45 woody, tropical species) can be substantial, accounting for 17–36% of total storage (Rosell et al., 2020). For deadwood, recent work has found that the dissolved organic matter leached into soils during storms can alter soil properties, increasing the retention of soil water beneath (Piaszczyk et al., 2020). For bark alone, its decomposition effects on soil properties has also received renewed attention. At the Shale Hills Critical Zone Observatory (PA, United States), for example, bark decomposition strongly influenced the composition of nearby soil microbial communities and this influence varied by bark type (Malik et al., 2020). Thus, bark-water interactions are connected to biogeochemical processes, from solute and particulate cycling and transport to soil physicochemistry and microbial properties.
Conclusion
Given the expansive and temporally persistent geography of bark, as well as the diversity of its anatomical structures and water-related functions, we conclude that a broad ecohydrological perspective of bark-water interactions is currently merited. Observations available to date, especially recent observations, suggest that bark-water interactions play relevant roles in most major water (mass and energy) states and fluxes in a forest ecosystem. Moreover, bark – whether alive or dead – appears to couple water to other biogeochemical aspects of forest ecosystems, from canopy-to-soil nutrient exchanges to soil physicochemistry and microbial community structure. This perspective therefore urges ecohydrology research to more comprehensively consider the roles of bark across ecosystem compartments (as dead wood, sheddings, and on standing trees), as well as its structure and properties, to test the conditions under which bark-water interactions may be relevant (or may be ignored) in ecological and hydrological processes. Recent insights regarding bark’s possible hydrological importance to distressed plants (e.g., as a water source during drought or as an agent of embolism repair) are especially of interest. Thus, future work on the ecohydrological roles of bark (whether thick or thin) may provide key insights to forest ecohydrological functions (through thick and thin).
Data Availability Statement
The original contributions presented in the study are included in the article/Supplementary Material, further inquiries can be directed to the corresponding author.
Author Contributions
JV conceived the study, then structured the manuscript in collaboration with SD and AK-I. Figure 1 was initially designed by JV, Figure 2 was initially designed by SD, then both figures were finalized after comment by JV, SD, and AK-I. Writing tasks were split among the authors, so that all contributed draft text and reviewed each other’s draft text. All authors contributed to the article and approved the submitted version.
Funding
JV acknowledges the support from the United States-NSF (EAR-1954907).
Conflict of Interest
The authors declare that the research was conducted in the absence of any commercial or financial relationships that could be construed as a potential conflict of interest.
Acknowledgments
We would like to thank the peer reviewers, and Travis Swanson for his discussion and programming assistance with the bark surface area analyses.
Supplementary Material
The Supplementary Material for this article can be found online at: https://www.frontiersin.org/articles/10.3389/ffgc.2021.660662/full#supplementary-material
Footnotes
- ^ Translated from French on page 53 of Salamin (1959): “sur le tronc des arbres à écorce lisse, elle se dépose en bande étroite. sur les troncs dont l’écorce est fortement rugueuse, elle se conserve en tas ayant un grand pouvoir adhésif…”
References
Anderson, O. R. (2014). Microbial communities associated with tree bark foliose lichens: a perspective on their microecology. J. Eukaryot. Microbiol. 61, 364–370. doi: 10.1111/jeu.12116
André, F., Jonard, M., and Ponette, Q. (2008). Effects of biological and meteorological factors on stemflow chemistry within a temperate mixed oak–beech stand. Sci. Total Environ. 393, 72–83. doi: 10.1016/j.scitotenv.2007.12.002
Błońska, E., Klamerus-Iwan, A., Łagan, S., and Lasota, J. (2018). Changes to the water repellency and storage of different species of deadwood based on decomposition rate in a temperate climate. Ecohydrology 11:e2023. doi: 10.1002/eco.2023
Borger, G. A. (1973). Development and Shedding of Bark. In Shedding of plant parts. New York: Academic Press, 205–236.
Catinon, M., Ayrault, S., Clocchiatti, R., Boudouma, O., Asta, J., Tissut, M., et al. (2009). The anthropogenic atmospheric elements fraction: a new interpretation of elemental deposits on tree barks. Atmospheric Environ. 43, 1124–1130. doi: 10.1016/j.atmosenv.2008.11.004
Chan, T., Hölttä, T., Berninger, F., Mäkinen, H., Nöjd, P., Mencuccini, M., et al. (2016). Separating water-potential induced swelling and shrinking from measured radial stem variations reveals a cambial growth and osmotic concentration signal. Plant Cell Environ. 39, 233–244. doi: 10.1111/pce.12541
Crockford, R. H., Richardson, D. P., and Sageman, R. (1996). Chemistry of rainfall, throughfall and stemflow in a eucalypt forest and a pine plantation in south-eastern australia: 3. Stemflow and total inputs. Hydrol. Proc. 10, 25–42. doi: 10.1002/(sici)1099-1085(199601)10:1<25::aid-hyp297>3.0.co;2-w
Earles, J. M., Sperling, O., Silva, L. C., McElrone, A. J., Brodersen, C. R., North, M. P., et al. (2016). Bark water uptake promotes localized hydraulic recovery in coastal redwood crown. Plant Cell. Environ. 39, 320–328. doi: 10.1111/pce.12612
Evert, R. F. (2006). Esau’s Plant Anatomy: Meristems, Cells, and Tissues of the Plant Body: Their Structure, Function, and Development. New Jersey: John Wiley & Sons, Inc.
Fang, H., Baret, F., Plummer, S., and Schaepman-Strub, G. (2019). An overview of global leaf area index (LAI): methods, products, validation, and applications. Rev. Geophys. 57, 739–799. doi: 10.1029/2018rg000608
Farmer, A. M., Bates, J. W., and Bell, J. N. B. (1991). Seasonal variations in acidic pollutant inputs and their effects on the chemistry of stemflow, bark and epiphyte tissues in three oak∗ woodlands in NW Britain. New Phytol. 118, 441–451. doi: 10.1111/j.1469-8137.1991.tb00026.x
Fernow, B. E. (1913). A brief history of forestry in Europe, the United States and other countries, 3rd Edn. Canada: University Press Toronto.
Ferrenberg, S., and Mitton, J. B. (2014). Smooth bark surfaces can defend trees against insect attack: resurrecting a ‘slippery’ hypothesis. Funct. Ecol. 28, 837–845. doi: 10.1111/1365-2435.12228
Gaige, E., Dail, D. B., Hollinger, D. Y., Davidson, E. A., Fernandez, I. J., Sievering, H., et al. (2007). Changes in canopy processes following whole-forest canopy nitrogen fertilization of a mature spruce-hemlock forest. Ecosystems 10, 1133–1147. doi: 10.1007/s10021-007-9081-4
Groenman-van Waateringe, W. (1998). Bark as a natural pollen trap. Rev. Palaeobot. Palynol. 103, 289–294. doi: 10.1016/s0034-6667(98)00040-2
Grootemaat, S., Wright, I. J., Van Bodegom, P. M., Cornelissen, J. H., and Shaw, V. (2017). Bark traits, decomposition and flammability of australian forest trees. Aus. J. Bot. 65, 327–338. doi: 10.1071/bt16258
Haasis, F. W. (1934). Diametral changes in tree trunks (No. 581.134 H112). Washington, DC (EUA): Carnegie Institution of Washington.
Herwitz, S. R. (1985). Interception storage capacities of tropical rainforest canopy trees. J. Hydrol. 77, 237–252. doi: 10.1016/0022-1694(85)90209-4
Hofhansl, F., Wanek, W., Drage, S., Huber, W., Weissenhofer, A., and Richter, A. (2012). Controls of hydrochemical fluxes via stemflow in tropical lowland rainforests: effects of meteorology and vegetation characteristics. J. Hydrol. 452, 247–258. doi: 10.1016/j.jhydrol.2012.05.057
Holder, C. D. (2020). Advances and Future Research Directions in the Study of Leaf Water Repellency. In Forest-Water Interactions. Cham: Springer, 261–278.
Ilek, A., Kucza, J., and Morkisz, K. (2017). Hygroscopicity of the bark of selected forest tree species. iForest Biogeosci. For. 10, 220–226. doi: 10.3832/ifor1979-009
Ilek, A., Siegert, C. M., and Wade, A. (2021). Hygroscopic contributions to bark water storage and controls exerted by internal bark structure over water vapor absorption. Trees 24, 1–13. doi: 10.1007/s00468-021-02084-0
Johnson, C. E., Siccama, T. G., Denny, E. G., Koppers, M. M., and Vogt, D. J. (2014). In situ decomposition of northern hardwood tree boles: decay rates and nutrient dynamics in wood and bark. Can. J. Forest Res. 44, 1515–1524. doi: 10.1139/cjfr-2014-0221
Katz, C., Oren, R., Schulze, E. D., and Milburn, J. A. (1989). Uptake of water and solutes through twigs of Picea abies (L.) Karst. Trees 3, 33–37.
Klamerus-Iwan, A., Link, T. E., Keim, R. F., and Van Stan, J. T. (2020a). “Storage and routing of precipitation through canopies,” in Precipitation Partitioning by Vegetation: A Global Synthesis, eds J. T. Van Stan, E. D. Gutmann, and J. Friesen (Cham, Switzerland: Springer Nature), 17–34. doi: 10.1007/978-3-030-29702-2_2
Klamerus-Iwan, A., Lasota, J., and Błońska, E. (2020b). Interspecific variability of water storage capacity and absorbability of deadwood. Forests 11:575. doi: 10.3390/f11050575
Lamb, R. (1985). Litter fall and nutrient turnover in two eucalypt woodlands. Aus. J. Bot. 33, 1–14. doi: 10.1071/bt9850001
Lambais, M. R., Lucheta, A. R., and Crowley, D. E. (2014). Bacterial community assemblages associated with the phyllosphere, dermosphere, and rhizosphere of tree species of the atlantic forest are host taxon dependent. Microb. Ecol. 68, 567–574. doi: 10.1007/s00248-014-0433-2
Levia, D. F., and Herwitz, S. R. (2005). Interspecific variation of bark water storage capacity of three deciduous tree species in relation to stemflow yield and solute flux to forest soils. Catena 64, 117–137. doi: 10.1016/j.catena.2005.08.001
Levia, D. F., and Underwood, S. J. (2004). Snowmelt induced stemflow in northern hardwood forests: a theoretical explanation on the causation of a neglected hydrological process. Adv. Water Res. 27, 121–128. doi: 10.1016/j.advwatres.2003.12.001
Linhoss, A. C., and Siegert, C. M. (2016). A comparison of five forest interception models using global sensitivity and uncertainty analysis. J. Hydrol. 538, 109–116. doi: 10.1016/j.jhydrol.2016.04.011
Malik, R. J., Trexler, R. V., Eissenstat, D. M., and Bell, T. H. (2020). Bark decomposition in white oak soil outperforms eastern hemlock soil, while bark type leads to consistent changes in soil microbial composition. Biogeochemistry 150, 329–343. doi: 10.1007/s10533-020-00701-7
Mayr, S., Schmid, P., Laur, J., Rosner, S., Charra-Vaskou, K., Dämon, B., et al. (2014). Uptake of water via branches helps timberline conifers refill embolized xylem in late winter. Plant Physiol. 164, 1731–1740. doi: 10.1104/pp.114.236646
McColl, J. G. (1966). Accession and decomposition of litter in spotted gum forests. Aus. Forestry 30, 191–198. doi: 10.1080/00049158.1966.10675413
McGee, G. G., Cardon, M. E., and Kiernan, D. H. (2019). Variation in acer saccharum marshall (sugar maple) bark and stemflow characteristics: implications for epiphytic bryophyte communities. Northeastern Nat. 26, 214–235. doi: 10.1656/045.026.0118
Mencuccini, M., Hölttä, T., Sevanto, S., and Nikinmaa, E. (2013). Concurrent measurements of change in the bark and xylem diameters of trees reveal a phloem-generated turgor signal. New Phytol. 198, 1143–1154. doi: 10.1111/nph.12224
Nardini, A., Gullo, M. A. L., and Salleo, S. (2011). Refilling embolized xylem conduits: is it a matter of phloem unloading? Plant Sci. 180, 604–611. doi: 10.1016/j.plantsci.2010.12.011
Novick, K. A., Konings, A. G., and Gentine, P. (2019). Beyond soil water potential: an expanded view on isohydricity including land–atmosphere interactions and phenology. Plant Cell. Environ. 42, 1802–1815. doi: 10.1111/pce.13517
Parker, G. G. (1983). Throughfall and stemflow in the forest nutrient cycle. Adv. Ecol. Res. 13, 57–133. doi: 10.1016/s0065-2504(08)60108-7
Pausas, J. G. (2015). Bark thickness and fire regime. Funct. Ecol. 29, 315–327. doi: 10.1111/1365-2435.12372
Pfautsch, S., Renard, J., Tjoelker, M. G., and Salih, A. (2015). Phloem as capacitor: radial transfer of water into xylem of tree stems occurs via symplastic transport in ray parenchyma. Plant Physiol. 167, 963–971. doi: 10.1104/pp.114.254581
Piaszczyk, W., Lasota, J., and Błońska, E. (2020). Effect of organic matter released from deadwood at different decomposition stages on physical properties of forest soil. Forests 11:24. doi: 10.3390/f11010024
Pinkard, E., Battaglia, M., Bruce, J., Matthews, S., Callister, A. N., Hetherington, S., et al. (2015). A history of forestry management responses to climatic variability and their current relevance for developing climate change adaptation strategies. For. An. Int. J. For. Res. 88, 155–171. doi: 10.1093/forestry/cpu040
Ponette-González, A. G., Van Stan, J. T., and Magyar, D. (2020). Things Seen and Unseen in Throughfall and Stemflow. In Precipitation Partitioning by Vegetation. Cham: Springer, 71–88.
Pypker, T. G., Levia, D. F., Staelens, J., and Van Stan, J. T. (2011). “Canopy structure in relation to hydrological and biogeochemical fluxes,” in Forest hydrology and biogeochemistry. Cham: Springer, 371–388.
Roesch, A., and Roeckner, E. (2006). Assessment of snow cover and surface albedo in the ECHAM5 general circulation model. J. Clim. 19, 3828–3843. doi: 10.1175/jcli3825.1
Romero, C., Bolker, B., and Edwards, C. E. (2009). Stem responses to damage: the evolutionary ecology of Quercus species in contrasting fire regimes. New Phytol. 182, 261–271. doi: 10.1111/j.1469-8137.2008.02733.x
Rosell, J. A. (2019). Bark in woody plants: understanding the diversity of a multifunctional structure. Integr. Compar. Biol. 59, 535–547. doi: 10.1093/icb/icz057
Rosell, J. A., Gleason, S., Méndez-Alonzo, R., Chang, Y., and Westoby, M. (2014). Bark functional ecology: evidence for tradeoffs, functional coordination, and environment producing bark diversity. New Phytol. 201, 486–497. doi: 10.1111/nph.12541
Rosell, J. A., and Olson, M. E. (2007). Testing implicit assumptions regarding the age vs. size dependence of stem biomechanics using pittocaulon (senecio) praecox (asteraceae). Am. J. Bot. 94, 161–172. doi: 10.3732/ajb.94.2.161
Rosell, J. A., and Olson, M. E. (2014). The evolution of bark mechanics and storage across habitats in a clade of tropical trees. Am. J. Bot. 101, 764–777. doi: 10.3732/ajb.1400109
Rosell, J. A., Piper, F. I, Jiménez-Vera, C., Vergílio, P. C., Marcati, C. R., Castorena, M., et al. (2020). Inner bark as a crucial tissue for non-structural carbohydrate storage across three tropical woody plant communities. Plant Cell. Environ. 44, 156–170. doi: 10.1111/pce.13903
Rosell, J. A., Wehenkel, C., Pérez-Martínez, A., Arreola Palacios, J. A., García-Jácome, S. P., and Olguín, M. (2017). Updating bark proportions for the estimation of tropical timber volumes by indigenous community-based forest enterprises in quintana roo, mexico. Forests 8:338. doi: 10.3390/f8090338
Salamin, P. (1959). Le manteau de neige dans les forêts de Hongrie. Hydrol. Sci. J. 4, 47–79. doi: 10.1080/02626665909493143
Scholz, F. G., Bucci, S. J., Goldstein, G., Meinzer, F. C., Franco, A. C., and & Miralles-Wilhelm, F. (2007). Biophysical properties and functional significance of stem water storage tissues in neotropical savanna trees. Plant Cell. Environ. 30, 236–248. doi: 10.1111/j.1365-3040.2006.01623.x
Sexton, J. M., and Harmon, M. E. (2009). Water dynamics in conifer logs in early stages of decay in the pacific northwest, USA. Northwest Sci. 83, 131–139. doi: 10.3955/046.083.0204
Srivastava, L. M. (1964). Anatomy, chemistry, and physiology of bark. In International review of forestry research, Vol. 1. Amsterdam: Elsevier, 203–277.
Steppe, K., and Lemeur, R. (2007). Effects of ring-porous and diffuse-porous stem wood anatomy on the hydraulic parameters used in a water flow and storage model. Tree Physiol. 27, 43–52. doi: 10.1093/treephys/27.1.43
Tobón, C., Sevink, J., and Verstraten, J. M. (2004). Solute fluxes in throughfall and stemflow in four forest ecosystems in northwest Amazonia. Biogeochemistry 70, 1–25. doi: 10.1023/b:biog.0000049334.10381.f8
van Bel, A. J. E. (1990). Xylem-phloem exchange via the rays: the undervalued route of transport. J. Exp. Bot. 41, 631–644. doi: 10.1093/jxb/41.6.631
Van Stan, J. T., Jarvis, M. T., and Levia, D. F. (2010). An automated instrument for the measurement of bark microrelief. IEEE Trans. Instrumen. Measur. 59, 491–493. doi: 10.1109/tim.2009.2031338
Van Stan, J. T., Morris, C. E., Aung, K., Kuzyakov, Y., Magyar, D., Rebollar, E. A., et al. (2020). Precipitation Partitioning—Hydrologic Highways Between Microbial Communities of the Plant Microbiome? In Precipitation Partitioning by Vegetation. Cham: Springer, 229–252.
Van Stan, J. T., Norman, Z., Meghoo, A., Friesen, J., Hildebrandt, A., Côté, J.-F., et al. (2017a). Edge-to-stem variability in wet-canopy evaporation from an urban tree row. Boundary-Layer Meteorol. 165, 295–310. doi: 10.1007/s10546-017-0277-7
Van Stan, J. T., Coenders-Gerrits, M., Dibble, M., Bogeholz, P., and Norman, Z. (2017b). Effects of phenology and meteorological disturbance on litter rainfall interception for a Pinus elliottii stand in the southeastern united states. Hydrol. Proc. 31, 3719–3728. doi: 10.1002/hyp.11292
Van Stan, J. T., Wagner, S., Guillemette, F., Whitetree, A., Lewis, J., Silva, L., et al. (2017c). Temporal dynamics in the concentration, flux, and optical properties of tree-derived dissolved organic matter in an epiphyte-laden oak-cedar forest. J. Geophys. Res. Biogeosci. 122, 2982–2997. doi: 10.1002/2017jg004111
Van Stan, J. T., and Pypker, T. G. (2015). A review and evaluation of forest canopy epiphyte roles in the partitioning and chemical alteration of precipitation. Sci. Total Env. 536, 813–824. doi: 10.1016/j.scitotenv.2015.07.134
Voigt, G. K., and Zwolinski, M. J. (1964). Absorption of stemflow by bark of young red and white pines. For. Sci. 10, 277–282.
Vorholt, J. A. (2012). Microbial life in the phyllosphere. Nat. Rev. Microbiol. 10, 828–840. doi: 10.1038/nrmicro2910
Westoby, J. (1989). Introduction to world forestry: people and their trees. Oxford: Basil Blackwell.
Wiersum, K. F. (1995). 200 years of sustainability in forestry: lessons from history. Environ. Manage. 19, 321–329. doi: 10.1007/bf02471975
Wittmann, C., and Pfanz, H. (2008). Antitranspirant functions of stem periderms and their influence on corticular photosynthesis under drought stress. Trees 22, 187–196. doi: 10.1007/s00468-007-0194-3
Wolfe, B. T. (2020). Bark water vapour conductance is associated with drought performance in tropical trees. Biol. Lett. 16:20200263. doi: 10.1098/rsbl.2020.0263
Woods, P. V., and Raison, R. J. (1983). Decomposition of litter in sub-alpine forests of Eucalyptus delegatensis, E. pauciflora and E. dives. Aus. J. Ecol. 8, 287–299. doi: 10.1111/j.1442-9993.1983.tb01326.x
Xu, X., Yu, X., Mo, L., Xu, Y., Bao, L., and Lun, X. (2019). Atmospheric particulate matter accumulation on trees: a comparison of boles, branches and leaves. J. Cleaner Produc. 226, 349–356. doi: 10.1016/j.jclepro.2019.04.072
Yanoviak, S. P., Silveri, C., Stark, A. Y., Van Stan, J. T., and Levia, D. F. (2017). Surface roughness affects the running speed of tropical canopy ants. Biotropica 49, 92–100. doi: 10.1111/btp.12349
Zhao, L., Yebra, M., van Dijk, A. I., Cary, G. J., Matthews, S., and Sheridan, G. (2021). The influence of soil moisture on surface and sub-surface litter fuel moisture simulation at five australian sites. Agric. Forest Meteorol. 298:108282. doi: 10.1016/j.agrformet.2020.108282
Zuo, J., Berg, M. P., Klein, R., Nusselder, J., Neurink, G., Decker, O., et al. (2016). Faunal community consequence of interspecific bark trait dissimilarity in early-stage decomposing logs. Funct. Ecol. 30, 1957–1966. doi: 10.1111/1365-2435.12676
Keywords: hydrology, precipitation, transpiration, evaporation, water uptake, biogeochemistry, bark, forest
Citation: Van Stan JT, Dymond SF and Klamerus-Iwan A (2021) Bark-Water Interactions Across Ecosystem States and Fluxes. Front. For. Glob. Change 4:660662. doi: 10.3389/ffgc.2021.660662
Received: 29 January 2021; Accepted: 18 March 2021;
Published: 09 April 2021.
Edited by:
Tom Grant Pypker, Thompson Rivers University, CanadaReviewed by:
Julieta A. Rosell, National Autonomous University of Mexico, MexicoTaehee Hwang, Indiana University Bloomington, United States
Copyright © 2021 Van Stan, Dymond and Klamerus-Iwan. This is an open-access article distributed under the terms of the Creative Commons Attribution License (CC BY). The use, distribution or reproduction in other forums is permitted, provided the original author(s) and the copyright owner(s) are credited and that the original publication in this journal is cited, in accordance with accepted academic practice. No use, distribution or reproduction is permitted which does not comply with these terms.
*Correspondence: John T. Van Stan, cHJvZmVzc29yLnZhbnN0YW5AZ21haWwuY29t