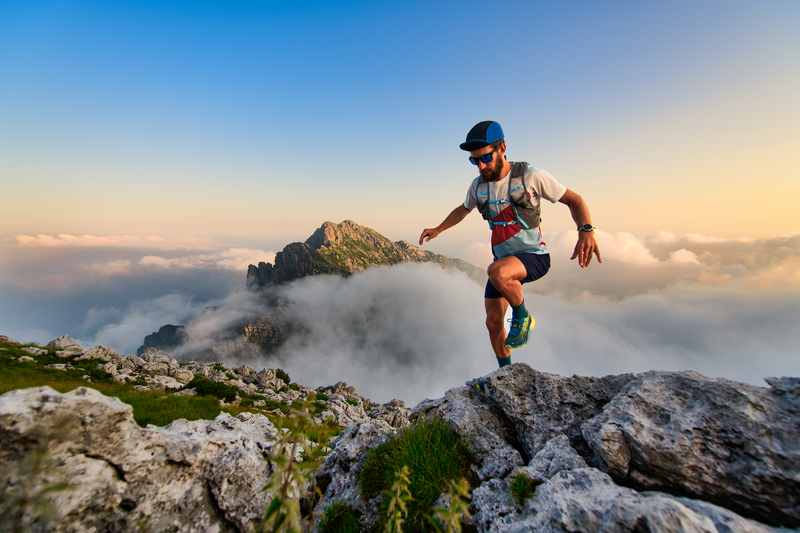
95% of researchers rate our articles as excellent or good
Learn more about the work of our research integrity team to safeguard the quality of each article we publish.
Find out more
ORIGINAL RESEARCH article
Front. For. Glob. Change , 15 April 2021
Sec. Forest Hydrology
Volume 4 - 2021 | https://doi.org/10.3389/ffgc.2021.654375
This article is part of the Research Topic Bark-Water Interactions View all 13 articles
Stemflow can be an important pathway for the drainage of precipitation and related solutes through tree canopies to forest soils. As stemflow must drain along bark surfaces, the effects of bark structure on stemflow chemical composition is merited. This study examines the relationship between stemflow chemistry and bark surface structure for six species of varying bark morphology (four deciduous broadleaf trees and two evergreen coniferous trees) at a montane and an urban site in Japan. Stemflow from smooth-barked species contained greater concentrations of solutes that appear to be rinsed from the stem surface (i.e., sea salt aerosols); while, rougher-barked tree species contained greater or less concentrations of solutes that appear to be leached (e.g., Ca2+) or taken-up (e.g., inorganic N) by the bark, respectively. Site-specific atmospheric environments also influenced thee bark-stemflow chemistry relationships—where the greater elemental deposition in the urban plot generally resulted in greater stemflow chemistry than observed in the lower-deposition montane plot. Our results therefore suggest that the dynamics of dry deposition wash-off by stemflow, and the exchange of dissolved solutes between stemflow and the bark surface, are influenced by the surface structure of the bark and the site’s atmospheric environment. Therefore, the interactions between bark surface structure and its surrounding atmospheric environment are important factors in the stemflow-related elemental cycling between the tree and precipitation.
As rainfall drains through tree canopies, it becomes an important nutrient input to the forest floor. This is because a portion of the rainwater contacts the leaves, branches, and epiphytes of the tree canopy, becoming throughfall (droplets that pass through, and drip from the canopy), and stemflow (a portion that travels over the bark surface to the base of the tree stem). Throughfall and stemflow become chemically altered as solutes are leached from the tree canopy surfaces, dry-deposited components are washed away by the draining rainwaters, and these surfaces (and/or their resident flora and fauna) uptake or transform solutes within the rainwater (Ponette-González et al., 2020). In forests, compared to other vegetated landscapes, throughfall accounts for the majority of the rainfall (Lin et al., 2020), while stemflow from trees rarely exceeds 2% of total rainfall (Van Stan and Gordon, 2018). Stemflow’s small proportion of the canopy rainwater balance, however, does not prevent it from contributing significantly to the net rainfall nutrient flux (Levia and Germer, 2015). Because stemflow can collect water from a large area of the tree canopy and spatially concentrate this water to a small area at the base of the tree, 10–2–101 m2 tree–1 (Van Stan and Allen, 2020), it can be a concentrated nutrient flux worthy of investigation in ecosystem ecology and hydrology disciplines (Levia and Germer, 2015; Van Stan and Gordon, 2018). In fact, several studies in various forest systems have found stemflow to be able to contribute 10–51% of various dissolved inorganic N fluxes per hectare, despite being <10% of gross rainfall (Liu et al., 2003; Pilegaard et al., 2003; Oziegbe et al., 2011; Germer et al., 2012). Thus, it is necessary to incorporate stemflow in investigations of forest water and nutrient cycling processes.
Stemflow can be highly enriched in a variety of solutes, including inorganic cations (e.g., K+, Na+, Ca2+, Mg2+, NH4+), anions (e.g., Cl–, SO2–, NO3–) (Parker, 1983), and dissolved organic C (DOC: Stubbins et al., 2020). The chemical composition of stemflow can differ substantially among tree species and across forest settings (e.g., Limpert and Siegert, 2019; Liu et al., 2019; Su et al., 2019). Variability in stemflow chemistry across forest settings may be related to differences in surrounding landscape properties (Rodrigo et al., 2003), and storm synoptic patterns (Siegert et al., 2017). Stemflow chemical variability across species at the same site, may be due to interspecific differences in canopy structures that alter the canopies’ ability to capture and accumulate aerosols or change the solute leaching and uptake properties of canopy surfaces (Levia et al., 2011). As stemflow drains primarily down bark-covered canopy elements (like branches and stems), some past research examining interspecific differences in stemflow chemistry has focused on differences in bark external structure (Levia and Herwitz, 2005; McGee et al., 2019).
The structure of the bark surface can affect stemflow chemistry by altering the amount and type of dry deposited materials capture on bark (Xu et al., 2019), the corticolous epiphytic vegetation and microbes (Magyar, 2008; McDonald et al., 2017), the bark surface area for solute exchange (Whittaker and Woodwell, 1967), and the water storage and residence time in the canopy (Levia and Herwitz, 2005). Few studies explicitly examine the interaction between bark surface structure and stemflow chemistry. Levia and Herwitz (2005) conducted a comparative study of the chemical composition and water retention of stemflow in three broad-leaved trees with different bark morphologies (Betula lenta L., Carya glabra Mill., and Quercus rubra L.) with a purpose similar to that of the present study, and reported that capacity of bark water storage and stemflow chemistry were different among bark surface morphology. Limpert and Siegert (2019) examined stemflow for a wider suite of solutes, including macronutrient ions and dissolved organic matter, and reported that thicker-barked (fire-tolerant) oaks produced more enriched stemflow than thinner-bark species, theoretically due to greater residence time. Su et al. (2019) reported the flux of inorganic ions in forests by stemflow analysis for deciduous trees with deeply furrowed bark and evergreen trees with smoother bark, finding that the stemflow from the deciduous trees was more chemically enriched due to greater surface area, contact time, and more dead materials in the rougher bark.
These studies show that the dynamics of bark surface-mediated solute exchange with stemflow can play a part in the nutrient cycle of forests. Thus, this study aims to build on these previous findings by conducting comparative evaluations of the impact of tree bark surface structure on stemflow chemistry (pH, dissolved organic carbon (DOC), dissolved inorganic carbon (DIC), Cl–, NO3–, SO42–, Na+, NH4+, Mg2+, Ca2+, and K+) across multiple tree species situated within study sites that represent pristine (the Minami Alps mountains) and suburban (Tokyo) sites in Japan. Seven tree species representing a range of bark surface structure, from smooth-to-rough, were selected for the study (Figure 1). We expect to reject the null hypothesis: stemflow chemistry will not differ among species of differing bark surface structure. Results are expected to show that, for stemflow from smoother-to-rougher bark: (i) solutes predominantly originating from wash-off (like Na+, Cl–, and DOC) and leaching (like Mg2+, Ca2+, and K+) will become more concentrated; but (ii) solutes susceptible to being taken up or transformed by the bark surface (like NO3– and NH4+) will become less concentrated. The relationships between stemflow solute concentrations and bark surface structure are also expected to (iii) differ between sites due to the influence of human activities and differences in rainfall itself.
Figure 1. (A) The study sites. The map scale is 1:5,164,000. The bark morphology of target species. (B) Clethra barbinervis, (C) Padus grayana, (D) Magnolia obovata, (F) Abies firma, (G) Castanea crenata, and (H) Tsuga diversifolia. The bark morphology of Tsuga sieboldii is the same as T. diversifolia.
The study sites included a montane, temperate forest in Tashiro of Shizuoka City, central Japan (the Shizuoka site, Figure 1); and, a suburban, temperate forest at the Tsukuba Experimental Forest, Mountain Research Center, University of Tsukuba, Japan (the Tsukuba site, Figure 1). For the Shizuoka site (35.307672 N, 138.199925 E), the elevation is 966 m a.s.l., the annual rainfall is 3110.1 mm, and the annual mean temperature is 11.4°C; the Tsukuba site (36.115326 N, 140.101504 E) has an elevation of 25 m a.s.l. and an annual rainfall of 1282.9 mm and an annual average temperature of 13.8°C (1981–2010 statistical period per AMeDAS: Japan Meteorological Agency, 2019). Canopy height of the study site at the Shizuoka site was approx. 20 m (Seino and Endoh, 2019), and at the Tsukuba site was almost the same as at the Shizuoka site (Seino, personal observations). The Shizuoka site is approx. 48 km far from the nearest coast, and the Tsukuba site is approx. 45 km away.
The tree species selected for this study included deciduous broadleaved and evergreen conifer species (Figure 1). The selected deciduous broadleaved trees were found at both the montane and the suburban forest sites, and included Clethra barbinervis Siebold. & Zucc. (Japanese Clethra), Padus grayana Maxim. (Japanese bird cherry), Magnolia obovata Thunb. (Japanese whitebark magnolia), and Castanea crenata Siebold. & Zucc. (Japanese chestnut). The selected evergreen conifers included Abies firma Siebold. & Zucc. (momi fir) at both sites, Tsuga sieboldii Carr. (Japanese hemlock) only at Shizuoka site, and Tsuga diversifolia (Maxim.) Mast. (kometsuga) only at Tsukuba site. The bark types were categorized and photos showing the bark surface structure are provided in Figure 1. Both of the Tsuga species have a coarse, thick, exfoliating bark with fissures, where T. diversifolia has a vertically peeling bark structure while T. sieboldii has a square-like cracked and flaky bark structure. The bark of A. firma is scaley and has resinous blisters, which is smoother than the Tsuga species but rougher and thicker than many of the broadleaved species. Clethra barbinervis also has exfoliating bark, but it is the thinnest bark of all the study species—although the exfoliating bark flakes add a “mottling” to the surface. The bark of P. grayana is also quite smooth and thin; however, it is laterally marked by shallow lenticels. Magnolia obovata presents a stippled, gray bark that is moderately rough and thick, yet C. crenata has the thickest bark of all the study species and is deeply furrowed.
The study period ran from May to December 2018 with tree selection and equipment installation from May to August 2018. Sound individual trees with low inclination of the stem and little influence by other canopy cover or vine plants were selected as target trees. Rain and stemflow water samples were collected and chemically analyzed for four storms. Sampling dates for the Shizuoka site were September 11, September 26, October 17, and November 29 2018; and, for the Tsukuba site were September 12, September 23, October 10, and December 9 2018 (Figure 2). We recognize that sampling only four storms limits the power of our analyses due to under-replication. These storms represent a range of their rainfall amount and duration.
Figure 2. Rainfall and sampling date from 1 September to 31 December 2018 at the Shizuoka and Tsukuba sites. Rainfall data at the Shizuoka site is from the Water Information System of the Ministry of Land, Infrastructure, Transport and Tourism (http://www1.river.go.jp/cgi-bin/SrchRainData.exe?ID=105031285523010&KIND=1&PAGE=0, last viewed 3 December 2019), and at Tsukuba site is measured by the meteorological station of the Tsukuba Experimental Forest, Mountain Science Center, University of Tsukuba.
Rainfall was collected by drilling a hole in the lid of a 5 L polyethylene tank and attaching a 9 cm diameter funnel. To prevent litter and insects from entering the funnel, a ping-pong ball was placed in the funnel, and the entire funnel was covered with a net (Figure 3). One gauge each was installed at the Shizuoka and Tsukuba site. Stemflow was collected by installing collars to capture waters draining down the outside of the stem which drained these waters to polyethylene collection bins using the following methods. First, to prevent rainwater from seeping out of the collar, the lichen on the stems where the collar was to be installed was removed, and paraffin wax was applied. Second, a 5 cm wide, 5 mm thick rubber roll was placed beneath and fixed to the tree stem to divert the water draining down the bark surface into a 25 cm wide, 3 mm thick rubber sheet with a water intake valve at the bottom (which was wrapped around the stem). Instant adhesive and silicone for caulking were used to bond the rubber to the tree stem. One end of a vinyl hose, with water pipe sealing tape wrapped around both ends, was inserted into the outer rubber collar where the water outlet was located, and the other end was connected to a 5 L polyethylene tank with a hole drilled in the lid. Finally, rubber bands were used to secure the poly tank to the stem (Figure 3). In total, 148 water samples were obtained during the study period for the six target species with three replicate trees per species (Shizuoka site: rainfall (n = 4), stemflow (n = 72); Tsukuba site: rainfall (n = 4), stemflow (n = 68)). Between storms, rainfall and stemflow samplers were rinsed by DW after every sampling. Gauges were sampled within 24 h of the end of a storm event.
Figure 3. Photograph and diagrams of the water sampling system. (a) Photograph of the implementation on Abies firma at the Shizuoka site, (b) diagram of water collector for direct rainfall, (c) water collection system for stemflow.
Each water sample was filtered through a 0.45 μm filter (GLCTD-MCE2545, Shimadzu GLC, Tokyo), then the following chemical parameters were measured: pH, dissolved organic carbon (DOC), inorganic carbon (DIC), and inorganic ion concentrations (Cl–, NO3–, SO42–, Na+, NH4+, Mg2+, Ca2+, and K+). A total organic carbon analyzer (TOC-V CSH, Shimadzu, Kyoto) was used to measure DOC and DIC. Inorganic ion concentrations were measured using ion chromatography (Prominence series, Shimadzu, Kyoto). Calibration curves were prepared using five mixed standard solution for the peak area of each ion, and quantification was performed after confirming their correlation coefficients.
Statistical analyses were performed using R version 3.4.2 (R Core Team, 2017) with a multiple-way analysis of variance (ANOVA) for comparing effects between sites and tree species, and differences among species were compared by one-way ANOVA and Tukey’s multiple comparisons for chemistries of rainfall and stemflow. Heteroscedasticity of each values was checked by Bartlett’s test before ANOVA. To evaluate patterns within the several stemflow chemical parameters, altogether, we applied a Principal Components Analysis on a matrix of log-transformed solute concentration data using the online ClustVis application1 by Metsalu and Vilo (2015). ClustVis was chosen, not only due to its simplicity, but due to its ease of reproducibility.
Rainwater solute concentrations were all lower for the montane site (Shizuoka) compared to the urban site (Tsukuba) (Figure 4). Mean (±SD) of rainwater concentrations in DOC, Cl–, SO42–, Na+, Mg2+, Ca2+, and K+ for Shizuoka were 1.4 ± 0.6 mg L–1, 6.3 ± 4.1 μmol L–1, 2.2 ± 1.6 μmol L–1, 7.7 ± 2.9 μmol L–1, 1.3 ± 0.9 μmol L–1, 0.1 ± 0.2 μmol L–1, and 1.3 ± 2.2 μmol L–1, respectively. For the Tsukuba site, rainwater concentrations of the same solutes were larger and more variable, being 4.4 ± 3.8 mg L–1, 46.7 ± 31.9 μmol L–1, 9.0 ± 3.4 μmol L–1, 40.5 ± 27.6 μmol L–1, 6.7 ± 3.0 μmol L–1, 9.2 ± 4.0 μmol L–1, and 7.4 ± 4.9 μmol L–1, respectively. For the N solutes, all observations were below observation limits in rain samples from the montane Shizuoka site; while mean (±SD) NO3– and NH4+ concentrations for the urban site were 13.4 ± 9.1 μmol L–1 and 16.8 ± 15.3 μmol L–1, respectively. Thus, all chemical parameters measured in rainfall, barring pH, were statistically significant between sites (unpaired t-test, p > 0.05) for pH, p < 0.05 for excepted pH).
Figure 4. Box-plot of TOC, pH, Cl–, NO3–, SO42–, Na+, NH4+, Mg2+, Ca2+, and K+concentrations of stemflow by species at foliated and defoliated periods. Species abbreviations are Clethra barbinervis (CB), Padus grayana (PG), Magnolia obovate (MO), Abies firma (AF), Castanea crenata (CC), Tsuga diversifolia (TD), and Tsuga sieboldii (TS). Different letters should indicate significant differences between factor levels. Bars in figure are SD. The letters in the figure mean that there is no difference according to Tukey’s comparison.
Across the study species, there was a general trend of decreasing stemflow pH with increasing bark roughness, from 6.0 ± 0.4 for smooth-barked (broadleaved) species like C. barbinervis and P. grayana to 5.5 ± 0.4 for rough-barked (evergreen) species like T. sieboldii and T. diversifolia (Figure 4). Na+, SO42–, Cl–, and Ca2+ also showed the same trend as pH, and species and site effects were detected for Na+ and Ca2+ [Two-way ANOVA, F(5, 132) = 3.07, p < 0.001 for Na+; F(0.129) = 2.81, p < 0.001 for Ca2+], while only site effects were detected for SO42– [Two-way ANOVA, F(1, 132) = 3.07, p < 0.001]. Deep teared bark type as C. crenata especially high in Ca2+. Patterns of NH4+ were low in C. barbinervis and coniferous species. In broad-leaved trees, the concentration in C. crenata stemflow tended to be higher at both the Shizuoka and Tsukuba sites, while for conifers, the stemflow of T. sieboldii was higher than that of A. firma at the Shizuoka site, whereas that of T. diversifolia was lower than that of A. firma at the Tsukuba site (Figure 4).
Further exploration of the several stemflow chemical parameters across species and sites was done by applying a principal components analysis (Figure 5). Two principal components were selected that represented 76% of the variability in the stemflow chemistry dataset [64% in component 1 (PC1) and 12% in component 2 (PC2)]. Individual stemflow observations per species at each site (faded-color symbols) were widely distributed across the principal components space (Figure 5). The mean principal component scores for each species’ stemflow chemistry at each site (represented by solid-colored symbols) show more distinctly how these observations amalgamated into two broad clusters, based primarily on site-specific differences (rather than species- or bark-related differences). The loadings plot (lower right of Figure 5) indicates that primarily “washed off” solutes (i.e., Na+, Cl–, SO42–) may explain the site-related clustering. This major site-related clustering based on solutes that were likely washed-off agrees with the rainfall data showing that the urban (Tsukuba) site had significantly higher rainwater solute concentrations compared to the montane (Shizuoka) site (Figure 4).
Figure 5. Principal component analysis with TOC, pH, Cl–, NO3–, SO42–, Na+, NH4+, Mg2+, Ca2+, and K+ concentrations measured in the Shizuoka site (solid line) and the Tsukuba site (dotted line). The arrows represent variable eigenvectors in the space plotted by the first two PCA axes. The projection of the lines along each axis indicates their relative importance.
Secondarily, the loadings plot indicates that interspecies variability within these site-specific PCA clusters spread out in relation to their primarily “exchanged” solute concentrations (i.e., DOC, Ca2+, K+, and Mg2+). The ordering of species with differing bark structure along the PCA plot’s representation of solute “exchange” differs between sites. For the montane site, where solute deposition contributions were lower, the roughest-barked tree species, both broadleaved and needeleaved (C. crenata, T. sieboldii) plotted high on PC2 (Figure 5). A cluster of smoother-barked tree species at Shizuoka, also both broadleaved and needleleaved (C. barbinervis, A. firma, M. obovata) is observed in the negative domain of PC2. The smooth-barked tree with deep lenticels, P. grayana, however, plotted intermediately between these other Shizuoka clusters on PC2. Interestingly, at the high-deposition urban site, Tsukuba, the interspecies variability within the PCA cluster is more evenly spaced along a continuum of smooth-to-rough bark (Figure 5). Although T. diversifolia is a rougher bark, it’s peeling bark structure is qualitatively smoother than T. sieboldii (see Figure 1), which may explain its location within the PCA space, nearby to the other more moderately rough barked species (A. firma, M. obovata). Thus, this PCA plot suggests that deposition chemistry is the principal driver of stemflow chemistry in this study; moreover, the site with higher deposition chemistry (Tsukuba) also produced a more evenly spaced solute “exchange” continuum along bark roughness (Figure 5).
Stemflow solute concentration and composition varied significantly across the bark roughness continuum represented by our seven study tree species, including both broadleaved and evergreen species (Figure 1). Thus, these results reject the null hypothesis that stemflow chemistry will not differ among species of differing bark surface structure. Relationships along the smoother-to-rougher bark continuum for stemflow chemistry were consistent across sites, where solutes predominantly originating from atmospheric deposition and wash-off (like Na+, Cl–, and DOC) and leaching (like Mg2+, Ca2+, and K+) generally increased with increasing bark roughness (Figure 4). Indeed, the PCA plot for stemflow data from the site with higher deposition, the urban Tsukuba site, showed this continuum relatively clearly (Figure 5). Reported solute concentrations fall within the range of those reported in previous stemflow literature (Parker, 1983; Levia and Germer, 2015; Van Stan and Stubbins, 2018). The higher stemflow solute concentrations from rough-barked trees, like C. crenata and T. sieboldii, also agree with the highly enriched stemflow solutions observed from similarly rough barked tree species, like Quercus species (Levia and Herwitz, 2005; Van Stan et al., 2017), Cyclobalanopsis species (Su et al., 2019), Fraxinus pennsylvanica (Schooling et al., 2017), Liriodendron tulipifera (Levia et al., 2011). Therefore, in combination with previous results, our study supports the conceptual model that bark structure may decrease bulk stemflow solute concentration by influencing both (a) the amount of materials captured by bark and (b) the amount of stemflow available to dilute those washed off (and exchanged) materials (Van Stan and Gordon, 2018).
On the other hand, stemflow solutes susceptible to being taken up or transformed by the bark surface (like NO3– and NH4+) displayed more complex responses across the species with changing bark roughness. In some cases, concentrations increased for smoother-barked trees, like mean NH4+ was higher for P. grayana than (the very rough-barked) C. crenata at the Tsukuba site (Figure 4). We note that concentrations of NO3– and NH4+ were low or almost undetectable for stemflow from many species and many storms (Figure 4). And that the loadings plot in the PCA shows a very small effect of N ion concentrations on overall patterns of stemflow chemistry (Figure 5). The generally low concentrations of these solutes may be due to strong uptake, as several studies have reported net uptake of both NH4+ and NO3– across forest sites, for throughfall as well as stemflow (Parker, 1983; Kloeti et al., 1989; Crockford et al., 1996; Cavalier et al., 1997; André et al., 2008). The instances of high inorganic N concentrations (higher than rainfall N concentrations) for some species at the urban Tsukuba site—especially those with moderate-to-rough bark—may reflect the amount of N build-up within these barks due to the urban deposition of particulates (i.e., Xu et al., 2019) rather than increased leaching. Although, we acknowledge the possibility of canopy N losses due to urban impacts on its nutrient intrasystem cycling (i.e., Decina et al., 2018). There was no difference in pH between the bark types. In the case of broad-leaved species, the pH of the stemflow of C. crenata was low, while that of B. grossa, which has the same coarse longitudinally split bark, was higher in Takenaka et al. (1998) (C. crenata, 5.61; Quercus crispula Blume, 5.79 at Aichi, central Japan) and Tsukahara (1994) (C. crenata, 5.6; Q. crispula, 6.2; at Yamagata, northern Japan), suggesting species specificity due to another factors (regional differences and so on) than the bark characters.
For stemflow, the Shizuoka (montane) and Tsukuba (urban) sites showed very different trends in solute concentrations and solute composition. NO3– concentrations at the Shizuoka site were not sufficiently high to be considered, while at the Tsukuba site, concentrations were high in most samples (Figure 4). This difference may be due to the urban atmospheric deposition at Tsukuba compared to Shizuoka (Lovett et al., 2000). The stemflow of C. crenata, A. firma, and T. sieboldii tended to be relatively high, and the stemflow of A. firma in particular increased rapidly. The concentration of Na+ was 10–20 times higher at the Tsukuba site than at the Shizuoka site. In contrast to the Shizuoka site, there was no difference between species at the Tsukuba site. NH4+ was hardly detected in samples at the Shizuoka site but was detected in many samples at the Tsukuba site. Although the concentrations of Mg2+ and Ca2+ were remarkably higher at the Tsukuba site than at the Shizuoka site, the trends at each site were similar (Figure 4). There was a common tendency for lower concentrations of direct rainfall and higher concentrations of C. crenata in stemflow relative to that of C. barbinervis, P. grayana, and M. obovata. In the stemflow of conifers at the Shizuoka site, A. firma was higher, while A. firma was higher at the Tsukuba site. For K+, the results were similar to those of Mg2+ and Ca2+, while bark type had no effect, except for stemflow in M. obovata, and stemflow in C. barbinervis. Katagiri (1977) reported high Mg2+ and Ca2+ content in the trunk of C. crenata and high K+ content in the leaves of C. barbinervis, suggesting that the nutrient content in each layer of the tree is involved in the dynamics of leaching substances.
Overall, the major differences between the Shizuoka and Tsukuba sites are thought to be differences in component concentrations due to differences in rainfall (Figure 4), and the presence or absence of nitrogen components due to differences in human activities such as traffic volume. The concentrations of Mg2+, Ca2+, and K+ for TOC and inorganic ions in Oka et al. (2019) suggested that organic acids are leaching along with these base cations, while the current results suggest that the counterparts to organic acids are mainly Mg2+ and Ca2+, which are influenced by bark. Besides, the concentrations of nitrogen and K+ obtained from the Tsukuba site suggest that NH4+ and K+ moving in conjunction. One reason for this is that the Tsukuba site is close to a farm, so components from the application of fertilizer were dispersed and detected in the stem stream. Besides, a comparison of the leaf-dressing and leaf-declining seasons showed a tendency for higher concentrations in the leaf-declining season, which may be due to the greater precipitation in summer than in winter (Eaton et al., 1973; Su et al., 2019).
An interesting cross-site finding, from the PCA plot, was that the solutes generally considered as exchangeable (DOC, K+, etc.), showed little trend across the species of varying bark roughness at the montane site. However, there was a clear “lining up” of the species’ stemflow chemistry (with regard to highly exchangeable solutes) along a general bark roughness continuum for the urban site, where deposition was much higher (Figure 5). We believe that this finding is interesting, because one would expect that higher deposition of soluble elements to these tree canopies would “mask” any potential trends in bark solute exchange—yet, the alignment along these loadings for PC2 is apparent (Figure 5). Is this an indication that high-deposition environments impact, not only the capture and washoff of solutes, but the exchange of related solutes? This is a reasonable question as urban atmospheric deposition is known to impact canopy physiology (Calfapietra et al., 2015). Alternatively, the trend observed in the PCA related to bark roughness and exchangeable solutes may have little-to-do with increased bark-water-solute exchange, and more-to-do with the increased capture of soluble aerosols by the more effective (rougher) “bark traps” for particulates (Magyar, 2008; Xu et al., 2019).
Many studies have found a tendency of low pH in coniferous stemflows (Sassa et al., 1990; Levia and Germer, 2015). The results also showed a tendency to lower stemflow, especially in two Tsuga species. The pH of C. crenata and A. firma also tends to decrease in response to stemflow. It has been reported that stemflow in broad-leaved trees inhibits the acidification of soil during the process of rainfall reaching the soil (Sassa and Hasegawa, 1992; Neary and Gizyn, 1994). This may explain why this effect is lower in C. crenata and conifer leaves, which are less able to repel rainfall and stay in the tree canopy due to their hard and well-developed cuticle, and why it tends to be different in coarse-barked Q. crispula and J. mandshurica, as mentioned in the comparison with previous studies (Shibata and Sakuma, 1996; Levia and Herwitz, 2005; Oka et al., 2019). In terms of TOC, SO42–, Mg2+, and Ca2+, the relationship between M. obovata and C. crenata at the Shizuoka site corresponded to the relationship between A. firma and two Tsuga species, suggesting that there is also a bark influence between conifers and broad-leaved trees. On the other hand, in stemflow at the Shizuoka site, Cl– was higher in M. obovata than in C. crenata, and that in A. firma was lower than in T. sieboldii; however, at the Tsukuba site, Cl– was lower in M. obovata than in C. crenata, and that in A. firma was higher than that in T. diversifolia, which was the complete opposite of the trend for conifers and broad-leaved trees. This is because the amount of stock of dry deposition on trees differs due to the difference in rainfall between sites, and the amount of stock is low in M. obovata and easy to retain in C. crenata. Rainfall capture by canopy leaves were different broad-leaved trees and confers, and this was affected to stemflow chemistry (Shibata and Sakuma, 1996; Abbasian et al., 2015; Su et al., 2019). Besides, the leaf area of Magnolia and Castanea is relatively large, even as compared to other broad-leaved trees (White, 1983), and conifers have small needle leaves.
The results of this study agree with research at other sites that bark surface structure is related to stemflow chemistry. Additionally, this relationship between bark surface structure and stemflow chemistry at our sites depended on the tree canopies external environment (specifically, whether the atmospheric deposition environment was montane or urban). Site-specific differences appear to be driven by increased wet deposition (more concentrated rainfall chemistry) at the urban site compared to the montane site. The retention capacity of (assumed) dry deposited elements was different between study trees with smooth and rough barks—and their mobilization by stemflow depended on the rainfall amount at each site. At the Shizuoka site, where there is more (chemically dilute) rainfall, the smooth bark type, with its thin bark structure without grooves, appears to immediately rinse the bark of soluble minerals. On the other hand, the coarse bark type, with multi-layered and wavy surface structures, permitted the bark to store substantial amounts of soluble materials that became available during the stemflow process in the Shizuoka site. Our results support a growing body of literature that suggest bark surface structure can affect the dynamics of elemental cycling in the forest ecosystem by influencing stemflow chemistry.
The original contributions presented in the study are included in the article/supplementary material, further inquiries can be directed to the corresponding author/s.
AO, YE, and TS collected samples. AO and JT carried out chemical analyses. All authors contributed to the writing of the manuscript, conceived and designed the research plan.
This study was partly supported by a Grant-in-Aid from the Mountain Research Center, University of Tsukuba.
The authors declare that the research was conducted in the absence of any commercial or financial relationships that could be construed as a potential conflict of interest.
We are grateful to Yoshihiko Tsumura, Takashi Kamijo, and laboratory members of Ikurinken for their helpful advice; JV and the two reviewers for manuscript improvements; Shozo Kato of Kato Limited Partnership Company and staff members of the Ikawa Forest Station, Mountain Research Center, University of Tsukuba, for their kind support at the Shizuoka site; and Jun-ya Tamaoki and Miho Sato of the Tsukuba Experimental Forest, Mountain Research Center, University of Tsukuba, for their kind support at the Tsukuba site. This research is part of a Master thesis of the University of Tsukuba by AO.
Abbasian, P., Attarod, P., Sadeghi, S. M. M., Van Stan, J. T., and Hojjati, S. M. (2015). Throughfall nutrients in a degraded indigenous forest and a plantation in the of North of Iran. Forest Systems 24:e035. doi: 10.5424/fs/2015243-06764
André, F., Jonard, M., and Ponette, Q. (2008). Effects of biological and meteorological factors on stemflow chemistry within a temperate mixed oak–beech stand. Sci. Total Environ. 393, 72–83.
Calfapietra, C., Peñuelas, J., and Niinemets, Ü (2015). Urban plant physiology: adaptation-mitigation strategies under permanent stress. Trends Plant Sci. 20, 72–75. doi: 10.1016/j.tplants.2014.11.001
Cavalier, J., Jaramillo, M., Solis, D., and de León, D. (1997). Water balance and nutrient inputs in bulk precipitation in tropical montane cloud forest in Panama. J. Hydrol. 193, 83–96. doi: 10.1016/S0022-1694(96)03151-4
Crockford, R. H., Richardson, D. P., and Sageman, R. (1996). Chemistry of rainfall, throughfall and stemflow in a Eucalypt forest and a Pine plantation in south-eastern Australia: 3. Stemflow Total Inputs 10, 25–42.
Decina, S. M., Templer, P. H., and Hutyra, L. R. (2018). Atmospheric inputs of nitrogen, carbon, and phosphorus across an urban area: unaccounted fluxes and canopy influences. Earth’s Future 6, 134–148. doi: 10.1002/2017ef000653
Eaton, J. S., Likens, G. E., and Bormann, H. (1973). Throughfall and stemflow chemistry in a northern hardwood forest. J. Ecol. 61, 495–508. doi: 10.2307/2259041
Germer, S., Zimmermann, A., Neill, C., Krusche, A. V., and Elsenbeer, H. (2012). Disproportionate single-species contribution to canopy-soil nutrient flux in an Amazonian rainforest, For. Ecol. Manage 267, 40–49. doi: 10.1016/j.foreco.2011.11.041
Japan Meteorological Agency (2019). AMeDAS: 1981–2010, Ministry of Land, Infrastructure, Transport, and Tourism Meteorological Agency HP. Tokyo: Japan Meteorological Agency.
Katagiri, S. (1977). Studies on Mineral Cycling in a Deciduous Broad-leaved Forest at Sanbe Forest of Shimane University (IV): Concentration of Nutrient Elements of Trees. Rome: FAO.
Kloeti, P., Keller, H. M., and Guecheva, M. (1989). Effects of Forest Canopy on Throughfall Precipitation Chemistry. Baltimore: Baltimore Symposium.
Levia, D. F., and Germer, S. (2015). A review of stemflow generation dynamics and stemflow-environment interactions in forests and shrublands. Rev. Geophys. 53, 673–714. doi: 10.1002/2015RG000479
Levia, D. F., and Herwitz, S. R. (2005). Interspecific variation of bark water storage capacity of three deciduous tree species in relation to stemflow yield and solute flux to forest soils. Catena 64, 117–137. doi: 10.1016/j.catena.2005.08.001
Levia, D. F., Van Stan, J. T., Siegert, C. M., Inamdar, S. P., Mitchell, M. J., Mage, S. M., et al. (2011). Atmospheric deposition and corresponding variability of stemflow chemistry across temporal scales in a mid-Atlantic broadleaved deciduous forest. Atmospheric Environ. 45, 3046–3054.
Limpert, K., and Siegert, C. (2019). Interspecific differences in canopy-derived water, carbon, and nitrogen in upland oak-hickory forest. Forests 10:1121.
Lin, M., Sadeghi, S. M. M., and Van Stan, J. T. (2020). Partitioning of rainfall and sprinkler-irrigation by crop canopies: a global review and evaluation of available research. Hydrology 7:76. doi: 10.3390/hydrology7040076
Liu, W., Fox, J. E. D., and Xu, Z. (2003). Nutrient budget of a montane evergreen broad-leaved forest at ailao mountain national nature reserve, yunnan, southwest China. Hydrol. Processes 17, 1119–1134.
Liu, Y., Jiang, L., You, C., Li, H., Tan, S., Tan, B., et al. (2019). Base cation fluxes from the stemflow in three mixed plantations in the rainy zone of western China. Forests 10:1101. doi: 10.3390/f10121101
Lovett, G. M., Traynor, M. M., Pouyat, R. V., Carreiro, M. M., Zhu, W. X., and Baxter, J. W. (2000). Atmospheric deposition to oak forests along an urban–rural gradient. Environ. Sci. Technol. 34, 4294–4300. doi: 10.1021/es001077q
McDonald, L., Van Woudenberg, M., Dorin, B., Adcock, A. M., McMullin, R. T., and Cottenie, K. (2017). The effects of bark quality on corticolous lichen community composition in urban parks of southern Ontario. Botany 95, 1141–1149. doi: 10.1139/cjb-2017-0113
McGee, G. G., Cardon, M. E., and Kiernan, D. H. (2019). Variation in Acer saccharum Marshall (sugar maple) bark and stemflow characteristics: implications for epiphytic bryophyte communities. Northeastern Nat. 26, 214–235. doi: 10.1656/045.026.0118
Metsalu, T., and Vilo, J. (2015). ClustVis: a web tool for visualizing clustering of multivariate data using principal component analysis and heatmap. Nucleic Acids Res. 43, W566–W570.
Neary, A. J., and Gizyn, W. I. (1994). Throughfall and stemflow chemistry under deciduous and coniferous forest canopies in south-central Ontario. Canadian J. Forest Res. 24, 1089–1100. doi: 10.1139/x94-145
Oka, A., Takahashi, J., Endoh, Y., and Seino, T. (2019). Effect of bark characteristics on stemflow and its chemical components. Nagoya Repositary. 67, 29–34.
Oziegbe, M. B., Muoghalu, J. I., and Oke, S. O. (2011). Litterfall, precipitation and nutrient fluxes in a secondary lowland rain forest in Ile-Ife, Nigeria. Acta Bot. Brasil. 25, 664–671. doi: 10.1590/S0102-33062011000300020
Parker, G. G. (1983). Throughfall and stemflow in the forest nutrient cycle. Adv. Ecol. Res. 13, 57–133. doi: 10.1016/s0065-2504(08)60108-7
Pilegaard, K., Mikkelsen, T. N., Beier, C., Jensen, N. O., Ambus, P., and Ro-Poulsen, H. (2003). Field measurements of atmosphere-biosphere interactions in a Danish beech forest. Boreal Environ. Res. 8, 315–333.
Ponette-González, A. G., Van Stan, J. T., and Magyar, D. (2020). “Things seen and unseen in throughfall and stemflow,” in Precipitation Partitioning by Vegetation, eds J. T. Van Stan, E. Gutmann, and J. Friesen (Cham: Springer), 71–88. doi: 10.1007/978-3-030-29702-2_5
Rodrigo, A., Avila, A., and Roda, F. (2003). The chemistry of precipitation, throughfall and stemflow in two holm oak (Quercus ilex L.) forests under a contrasted pollution environment in NE Spain. Sci. Total Environ. 305, 195–205. doi: 10.1016/s0048-9697(02)00470-9
Sassa, T., Goto, K., Hasegawa, K., and Ikeda, S. (1990). Acidity and Nutrient Elements of the Rainfal1, Throughfall and Stemflow in the Typical Forests Around Morioka City, Iwate Pref., Japan. Characteristic pH Value of the Stemflow in Several Tree Species. Rome: FAO, doi: 10.18922/jjfe.32.2_43
Sassa, T., and Hasegawa, K. (1992). Suppression of Soil Acidification by the Stemflow of Some Tree Species. The Case of Yellow-poplar (tulip tree). Rome: FAO, doi: 10.11519/jjfs1953.74.5_437
Schooling, J. T., Levia, D. F., Carlyle-Moses, D. E., Dowtin, A. L., Brewer, S. E., Donkor, K. K., et al. (2017). Stemflow chemistry in relation to tree size: A preliminary investigation of eleven urban park trees in British Columbia, Canada. Urban Forestry Urban Green. 21, 129–133. doi: 10.1016/j.ufug.2016.11.013
Seino, T., and Endoh, Y. (2019). Ecological positions of conifers on the stand structure of the temperate forest in central Japan. Chubu Forestry Res. 67, 25–28. doi: 10.18999/chufr.67.25
Shibata, H., and Sakuma, T. (1996). Canopy modification of precipitation chemistry in deciduous and coniferous forests affected by acidic deposition. Soil Sci. Plant Nutrit. 42, 1–10. doi: 10.1080/00380768.1996.10414683
Siegert, C. M., Levia, D. F., Leathers, D. J., Van Stan, J. T., and Mitchell, M. J. (2017). Do storm synoptic patterns affect biogeochemical fluxes from temperate deciduous forest canopies? Biogeochemistry 132, 273–292.
Stubbins, A., Guillemette, F., and Van Stan, J. T. II (2020). “Throughfall and stemflow: the crowning headwaters of the aquatic carbon cycle,” in In Precipitation Partitioning by Vegetation, eds J. T. Van Stan, E. Gutmann, and J. Friesen (Cham: Springer), 121–132. doi: 10.1007/978-3-030-29702-2_8
Su, L., Zhao, C., Xu, W., and Xie, Z. (2019). Hydrochemical fluxes in bulk precipitation, throughfall, and stemflow in a mixed evergreen and deciduous broadleaved forest. Forests 10:507. doi: 10.3390/f10060507
Takenaka, C., Suzuki, M., Yamaguchi, N., and Shibata, E. (1998). Chemical characteristics of stem flow collected from deciduous trees. Nagoya University Forest Sci. 17, 11–17.
Tsukahara, H. (1994). Studies on the chemical properties of stemflow (I) differences in EC, pH, and major ion concentrations among tree species. Trans. Japanese For. Society 105, 407–410. (in Japanese)
Van Stan, J. T., and Allen, S. T. (2020). What we know about stemflow’s infiltration area. Front. For. Glob. Chang. 3, 1–7. doi: 10.3389/ffgc.2020.00061
Van Stan, J. T., and Gordon, D. A. (2018). Mini-Review: stemflow as a resource limitation to near-stem soils. Front. Plant Sci. 9:248. doi: 10.3389/fpls.2018.00248
Van Stan, J. T., and Stubbins, A. (2018). Tree-DOM: dissolved organic matter in throughfall and stemflow. Limnol. Oceanography Lett. 3, 199–214. doi: 10.1002/lol2.10059
Van Stan, J. T., Wagner, S., Guillemette, F., Whitetree, A., Lewis, J., Silva, L., et al. (2017). Temporal dynamics in the concentration, flux, and optical properties of tree-derived dissolved organic matter in an epiphyte-laden oak-cedar forest. J. Geophys. Res.: Biogeosci. 122, 2982–2997. doi: 10.1002/2017jg004111
White, P. S. (1983). Corner’s rules in eastern deciduous trees: allometry and its implications for the adaptive architecture of trees. Bull. Torrey Botan. Club. 110, 203–212. doi: 10.2307/2996342
Whittaker, R. H., and Woodwell, G. M. (1967). Surface area relations of woody plants and forest communities. Am. J. Botany 54, 931–939. doi: 10.1002/j.1537-2197.1967.tb10717.x
Keywords: bark morphology, direct rainfall, inorganic carbon, mineral cycling, stemflow, throughfall
Citation: Oka A, Takahashi J, Endoh Y and Seino T (2021) Bark Effects on Stemflow Chemistry in a Japanese Temperate Forest I. The Role of Bark Surface Morphology. Front. For. Glob. Change 4:654375. doi: 10.3389/ffgc.2021.654375
Received: 16 January 2021; Accepted: 22 March 2021;
Published: 15 April 2021.
Edited by:
John T. Van Stan, Georgia Southern University, United StatesReviewed by:
Juan Whitworth-Hulse, CONICET Institute of Applied Mathematics San Luis (IMASL), ArgentinaCopyright © 2021 Oka, Takahashi, Endoh and Seino. This is an open-access article distributed under the terms of the Creative Commons Attribution License (CC BY). The use, distribution or reproduction in other forums is permitted, provided the original author(s) and the copyright owner(s) are credited and that the original publication in this journal is cited, in accordance with accepted academic practice. No use, distribution or reproduction is permitted which does not comply with these terms.
*Correspondence: Ayano Oka, c2J0bi5ub3RjaGlAZ21haWwuY29t
†Present address: Tatsuyuki Seino, Yatsugatake Forest Station, Mountain Science Center, University of Tsukuba, Minamimaki, Japan
Disclaimer: All claims expressed in this article are solely those of the authors and do not necessarily represent those of their affiliated organizations, or those of the publisher, the editors and the reviewers. Any product that may be evaluated in this article or claim that may be made by its manufacturer is not guaranteed or endorsed by the publisher.
Research integrity at Frontiers
Learn more about the work of our research integrity team to safeguard the quality of each article we publish.