- 1Animal Ecology, J.F. Blumenbach Institute of Zoology and Anthropology, University of Göttingen, Göttingen, Germany
- 2Department of Taxonomy and Ecology, Branch of the National Institute of Research and Development for Biological Sciences (NIRDBS) Bucharest, Institute of Biological Research, Cluj-Napoca, Romania
- 3Centre of Biodiversity and Sustainable Land Use, University of Göttingen, Göttingen, Germany
Forest soil food webs have been assumed to be fueled substantially by root-derived resources. However, until today the flux of root-derived resources into soil animals has been investigated virtually exclusively using isotope labeling experiments, whereas studies on the consequences of disrupting the flux of root-derived resources into the soil animal food web are scarce. We here investigated the importance of root-derived resources for a wide range of soil animals by interrupting the resource flux into the soil of different forest types in Central Europe using a trenching experiment. We recorded the abundance of soil animal taxa varying in body size (micro-, meso-, and macrofauna) 1 and 3 years after root trenching, and quantified changes in biomass, species composition, and trophic shift using stable isotopes and NLFA analysis. Among the microfauna groups studied (trophic groups of Nematoda) only the abundance of plant feeding nematodes showed a trend in being decreased by -58% due to root trenching. Major soil mesofauna groups, including Collembola and Oribatida, suffered to a similar extent from root trenching with their abundance and biomass being reduced by about 30–40%. The soil macrofauna groups studied (Diplopoda, Isopoda, Chilopoda, Araneae, Coleoptera) generally were only little affected by root trenching suggesting that they rely less on root-derived resources than micro- and in particular mesofauna. Notably, the community structure of micro-, meso-, and macrofauna was not affected by root trenching. Further, we observed trophic shifts only in 2 out of 10 investigated species with the shifts generally being only minor. The results indicate that soil animal communities are markedly resilient to deprivation of root-derived resources suggesting that links to root-derived resources are non-specific. However, this resilience appears to vary with body size, with mesofauna including both decomposers as well as predators being more sensitive to the deprivation of root-derived resources than microfauna (except for root feeders) and macrofauna. Overall, this suggests that body size constrains the channeling of energy through soil food webs, with root-derived resources in temperate forests being channeled predominantly via soil taxa of intermediate size, i.e., mesofauna.
Introduction
Interactions between the above- and the belowground components of terrestrial ecosystems are receiving increased attention as they strongly affect carbon (C) cycling (Bardgett and Wardle, 2010). Soil animal communities are linked to the aboveground system via two trophic pathways, i.e., via leaf litter input and root-derived resources (Wardle, 2002; Pollierer et al., 2007). Most litter C is bound in structural compounds and locked up in polymers rich in aliphatic and aromatic compounds (Tan, 2014) not readily available for soil animals without previous digestion by microbial enzymes. In addition, nitrogen (N) concentrations in litter are low (typically only 1–2%) compared to those in animal tissue (ca. 10%) (White, 1993; Vitousek et al., 2002). Nevertheless, leaf litter has been assumed to function as main food resource of soil animals as up to 90% of net primary plant production is entering the soil as detritus (Cebrian, 1999), providing ample resources for the decomposer system. However, recent studies indicate that root-derived resources may surpass those derived from leaf litter in fueling soil animal food webs (Ruf et al., 2006; Pollierer et al., 2007; Eissfeller et al., 2013; Gilbert et al., 2014). Roots release substances actively or passively into the soil (Curl and Truelove, 1986; Jones et al., 2009), which serve as an easily digestible C resource for microorganisms and are preferred compared to recalcitrant leaf litter (Farrar et al., 2003; van Hees et al., 2005). These rhizodeposits consist of sugars, organic acids, amino acids, phenols, and other secondary metabolites, as well as polysaccharides and proteins (Bais et al., 2006). Spatial and temporal patterns of the release of rhizodeposits lead to “hot spots” and “hot moments” in the rhizosphere, triggering the activity of soil microbial-driven processes (Beare et al., 1995; Kuzyakov and Blagodatskaya, 2015). The rhizosphere, thereby, is preferentially colonized by soil organisms such as bacteria, mycorrhizal and saprotrophic fungi and protists, which acquire resources from root deposits. Depending on plant species and season, 10–60% of the photosynthetically fixed C is allocated to roots (Kuzyakov and Domanski, 2000; Litton et al., 2007), and part of this C enters the soil food web via root-feeding species or animals feeding on mycorrhizal fungi and microorganisms that incorporated root-derived C (Ruf et al., 2006; Bonkowski et al., 2009; Högberg et al., 2010).
In forest soils a large fraction of the microbial biomass comprises ectomycorrhizal (ECM) fungi (Högberg and Högberg, 2002; Wallander et al., 2004; Ekblad et al., 2013). They contribute to element cycling, provide N to plants and channel large amounts of plant-derived C into the soil (Treseder et al., 2006; Clemmensen et al., 2013; Ekblad et al., 2013). The production rate of the extra-matrical mycelia of ECM fungi in the upper 10 cm of forest soil has been estimated to be about 160 kg dry matter ha–1 y–1 (Ekblad et al., 2013) and due to large biomass of ECM fungi it has been suggested as an important food resource for soil invertebrates (Fitter and Garbaye, 1994; Pollierer et al., 2012). However, recent evidence indicates that consumption of ECM fungi by soil invertebrates is limited (Potapov and Tiunov, 2016; Bluhm et al., 2019b).
The flux of root-derived resources through soil food webs and their assimilation by soil animals has been demonstrated by variations in the natural abundance of stable isotopes (Albers et al., 2006; Scheunemann et al., 2015) and isotope labeling experiments (Pollierer et al., 2007, 2009; Eissfeller et al., 2013; Scheunemann et al., 2016; Zieger et al., 2017a) conducted in grasslands (Ostle et al., 2007; Lemanski and Scheu, 2014), arable fields (Albers et al., 2006; Scheunemann et al., 2016), and forests (Pollierer et al., 2007; Zieger et al., 2017b). In long-term (Pollierer et al., 2007, 2009; Eissfeller et al., 2013) and short-term isotope labeling experiments (Scheunemann et al., 2016; Zieger et al., 2017a) it has been shown that root-derived resources are incorporated into the whole soil animal community and channeled across trophic levels. The addition of easily accessible resources, imitating root exudates, has been shown to increase the abundance of some species of soil invertebrates including Lumbricidae, Diplopoda, and Isopoda (Scheu and Schaefer, 1998; Tiunov and Scheu, 2004). However, the effect of deprivation of root-derived resources has only been investigated in Nematoda (Parmelee et al., 1993), as well as in Oribatida and Collembola by girdling of trees (Remén et al., 2008; Malmström and Persson, 2011) and trenching of roots (Siira-Pietikäinen et al., 2001, 2003).
Shortage of resources likely is among the main factors driving the abundance and biomass of soil animals (Scheu and Schaefer, 1998). Resource deprivation, however, also is likely to induce dietary shifts counteracting changes in abundance and biomass. Such dietary shifts may be traced by analyzing variations in stable isotope ratios as well as lipid patterns (Traugott et al., 2013). Variations in stable isotope ratios allow ascribing species to trophic levels as 15N concentrations increase with trophic level due to fractionation processes (Post, 2002; Potapov et al., 2019b). Further, variations in 13C concentrations in soil animal consumers allow to trace changes in the use of C resources (Albers et al., 2006; Pollierer et al., 2009; Potapov et al., 2019b). Neutral lipid fatty acid patterns in consumers allow to trace the incorporation of basal resources, such as bacteria, fungi and plants, into consumers as lipids in the diet are incorporated into consumers without major change (“dietary routing”; Pollierer et al., 2010; Ruess and Chamberlain, 2010).
In the present study, we investigated the effect of deprivation of resource input via tree roots on the abundance, biomass and community structure of micro-, meso-, and macrofauna in forests differing in management intensity, tree species composition and soil type in two regions of Germany using root trenching. To investigate potential shifts on basal resource use, we additionally analyzed stable isotope and neutral lipid fatty acids of abundant soil animal species.
We hypothesized that (1) the importance of root-derived resources varies with soil animal body size, with more pronounced effects on the abundance, biomass and community structure of fungal feeding micro- and mesofauna (relying heavily on root-derived resources) than macrofauna (relying more heavily on litter resources), (2) deprivation of fresh root-derived resources detrimentally affects abundance and biomass of soil animal communities with the detrimental effects increasing over time, (3) the response of microbivore soil micro- and mesofauna mirrors that of microbial biomass (and therefore varies more between regions than forest types; Bluhm et al., 2019a), and (4) soil invertebrates switch diet in response to shortage of root-derived resources, which is reflected in changes in 13C and 15N stable isotope values and neutral lipid fatty acid patterns.
Materials and Methods
Study Sites and Experimental Set-Up
The experiment was established in two regions of Germany at sites of the “Biodiversity Exploratories,” a large-scale and long-term biodiversity project (Fischer et al., 2010)1. Schorfheide-Chorin (hereafter Schorfheide) is located in the lowlands of Northeastern Germany (3–140 m a.s.l.) and characterized by postglacial geomorphological structures, and the Hainich-Dün (hereafter Hainich), located in the uplands of Central Germany (285–550 m a.s.l.). Mean annual temperatures at Schorfheide and Hainich are 8.0–8.5 and 6.5–8.0°C, and mean annual precipitation 500–600 and 500–800 mm, respectively. The forests stock on glacial till in the Schorfheide with the main soil type being Cambisol and in the Hainich on Triassic limestone with the main soil type being Luvisols with soil pH averaging 3.00 ± 0.19 and 4.59 ± 0.67, respectively. For more details on the study sites see Fischer et al. (2010) and Klarner et al. (2014). In each of the two regions four different forest types were selected: (1) managed coniferous forests with Norway spruce (Picea abies) in the Hainich (“spruce”) and Scots pine (Pinus sylvestris) in the Schorfheide (“pine”), (2) 30 years old managed beech (Fagus sylvatica) forests (“young beech”), (3) 70 years old managed beech forests (“old beech”), and (4) unmanaged natural beech forests left out of management for at least 60 years with some trees being 120–150 years old (“unmanaged beech”). Beech forests were dominated by F. sylvatica, interspersed with ash (Fraxinus excelsior), and sycamore (Acer pseudoplatanus). Each forest type was replicated four times per region, resulting in 32 plots. The minimum distance between plots was 500 m.
Establishment and Maintenance of Root-Trenching Plots
In each of the 32 plots, one “trenched” and one “control” subplot 1–2 m apart from each other of 120 × 120 cm was established between September and October 2011. In the trenched subplots trenches were cut into soil to a depth of 40–50 cm using a chainsaw, thereby cutting all tree roots. To prevent re-colonization of the plots by tree roots, polyethylene barriers (120 × 60 × 0.5 cm) were inserted into the trenches on each of the four sides of the plot. In addition, aluminium linings were inserted at the edges to close the gap between adjacent barriers. Polyethylene barriers extended ca. 10 cm above the ground to restrict animal migrations between the trenched plots and the surrounding. To control for potential side effects of aboveground parts of the barriers, control plots, which were established in close vicinity of the treatment plots, were also equipped with respective barriers aboveground.
At regular intervals during the growth period above-ground parts of herbaceous plants and grasses in trenched and control plots were removed to minimize input of root-derived resources from understory plants. Soil moisture was measured gravimetrically from soil cores and water was added to control plots equalizing the amount of water in the upper 10 cm of the soil in control and trenched plots, for details see Bluhm et al. (2019a).
Sampling
Soil samples for meso- and macrofauna were taken from each control and trenched plot after one (October 2012) and three (October 2014) years. Soil mesofauna was extracted from soil cores of a diameter of 5 cm (one core per subplot). For soil macrofauna soil cores of a diameter of 20 cm were taken (one core per subplot). The litter layer and upper 5 cm of the soil from each soil core were extracted separately by a heat gradient for macrofauna (Kempson et al., 1963) and mesofauna (Macfadyen, 1961). Extracted animals were stored in 70% ethanol, sorted and identified to order level. Soil mesofauna samples included springtails (Collembola), mites (Oribatida, Mesostigmata), proturans (Protura), diplurans (Diplura), and symphylans (Symphyla). Diplura and Symphyla were taken from the 20 cm soil cores for macrofauna as their abundance in the 5 cm cores was too low for statistical analysis. The macrofauna included millipedes (Diplopoda), woodlice (Isopoda), spiders (Araneae), centipedes (Chilopoda), and beetles (Coleoptera; adults and larvae).
Soil samples for nematode extraction (one core per subplot) were taken after 3 years of the experiment (October 2014) using a steel corer (2.5 cm diameter, 10 cm deep). Nematodes were extracted by modified Baermann method, see Ruess (1995), and transferred into 3%-formaldehyde solution. Nematodes in vials were concentrated by removing part of the formaldehyde solution. At least 100 well-preserved specimens (if available in the sample) were randomly selected and identified to genus (adults and most of the juveniles) or family level (juveniles) at 400 × magnification using a Leica DMI 4000B light microscope. Nematode taxa were classified according to Bongers (1994) and then ascribed to trophic groups (Yeates et al., 1993; Bongers and Bongers, 1998; Okada et al., 2005), ordered according to the colonization–persistence gradient (c–p values) (Bongers, 1990; Bongers and Bongers, 1998) and assigned to trophic groups (Bongers and Bongers, 1998; Ferris et al., 2001).
In addition to the soil core sampling, in October 2015 Lumbricidae were collected from an area of 0.25 m2 from each subplot by using mustard solution (Eisenhauer et al., 2008). The solution consisted of 100 g mustard powder (Semen Sinapis plv.; CAELO, Cesar & Loretz GmBH, 40721 Hilden, Germany) dissolved in 10 l water. Before applying the solution to the plots, the litter layer was removed and checked for earthworm specimens. Five liters of the solution were spilled evenly on the area, which was repeated after 15 min. Emerging Lumbricidae were hand-collected and placed into ethanol containing 5% formalin for preservation. For the analysis of trophic changes of soil animals, additional soil and litter samples were taken in autumn 2015 and the animals were extracted by heat (Kempson et al., 1963). To obtain enough animal tissue for NLFA analysis, we sampled litter and soil from a large area of each of the plots at the end of the experiment and extracted the animals by heat (see above). These samples were stored in glycerin at −10°C for later NLFA analysis (Zieger and Scheu, 2018).
For the sampling in 2014 abundant taxa were identified to species level and species richness and biomass was calculated. Collembola and Oribatida were identified to species level using Weigmann (2006) and Hopkin (2007), respectively. Collembola were ascribed to ecological groups using Gisin (1943), Isopoda were determined using Schaefer et al. (2018), Diplopoda using Blower (1985) and Chilopoda using Latzel (1880), Koren (1992), and Barber (2008). Lumbricidae species were identified to species level using Sims and Gerard (1985).
Biomass Calculation
The biomass of Oribatida (dry weight) was calculated using regression model 4 from Caruso and Migliorini (2009) based on species-specific mean lengths and widths as given in Weigmann (2006). For Collembola, biomass (fresh weight) was calculated using the mean length of species in millimeter as given in Hopkin (2007) and the formula log(Mfw) = 1.339 + 1.992 log(length), with Mfw being the fresh weight in microgram (Mercer et al., 2001). Fresh weight was converted to dry weight by using the formula log (Mdw) = −0.5499 + 0.9402 log(Mfw) as given in Mercer et al. (2001). For Myriapoda and Isopoda the length and maximum width of all individuals were measured. For the calculation of the biomass of Geophilomorpha and Scolopendromorpha the formula given in Klarner et al. (2017) was used. For Polydesmida, Isopoda, Lithobiomorpha model 1 given in Sohlström et al. (2018) was used. For other Diplopoda the formula given in Sprengel (1986) was used. Lumbricidae were weighed using a scale (Sartorius Secura 125-1S, Göttingen, Germany). To convert fresh weight to dry weight of Polydesmida, Isopoda, Trichoniscus and Lithobiomorpha the following factors were used: 0.42, 0.34, 0.42, and 0.29, respectively (S. Bluhm, unpubl. data).
Stable Isotope Analysis
To investigate diet shifts we selected abundant macrofauna species (one Isopoda species and four Lumbricidae taxa). For Isopoda Trichoniscus pusillus from the Hainich was selected including specimens from each of the two beech forest types (beech old and beech natural); the beech young and spruce forest could not be included as T. pusillus was rare in these forest types. For Lumbricidae Aporrectodea sp. and Octolasion lacteum in the Hainich, and Dendrobaena octaedra and Lumbricus rubellus in the Schorfheide were used including specimens from each of the four forest types; only these species were present in sufficient numbers to allow stable isotope analysis. For the analysis appropriate amounts of animal tissue (600–1,000 μg dry weight) were transferred into tin capsules using a Sartorius Cubis scale. For Isopoda whole animals were analyzed; for Lumbricidae the first sixteen segments of each worm were detached, freeze-dried and weighed into tin capsules.
Samples of T. pusillus were analyzed using a system consisting of an elemental analyzer (Euro EA 3000, EuroVector S.p.A, Milano, Italy) and a mass spectrometer (Delta V Plus, Thermo Electron, Bremen, Germany) coupled by a Conflo IV interface (Thermo Electron Corporation, Bremen, Germany). Lumbricidae were measured by a coupled system consisting of an elemental analyzer (NA1110, CE-Instruments, Rodano, Milano, Italy) and a mass spectrometer (Delta Plus, Thermo Electron, Bremen, Germany) coupled by a Conflo III interface (Thermo Electron Corporation, Bremen, Germany). The computer controlled systems allow on-line measurement of stable isotopes (13C, 15N) (Reineking et al., 1993; Werner et al., 1999; Langel and Dyckmans, 2014). Their abundance was expressed using the δ notation with δ13C and δ15N (‰) = (Rsample - Rstandard)/Rstandard × 1,000. Rsample and Rstandard represent the 13C/12C and 15N/14N ratios of samples and standard, respectively. For 13C Vienna PD belemnite (V-PDB) served as the primary standard. For 15N air served as the primary standard and acetanilide (C8H9NO; Merck, Darmstadt, Germany) for internal calibration.
Neutral Lipid Fatty Acid Analysis
For the NFLA analysis we used species of different trophic levels (decomposers and predators) and body size (meso- and macrofauna) from samples after 4 years of root trenching (2015). Only abundant species were included as ca. 600 μg dry weight was needed for NLFA analysis. The species/taxa included one mesofauna decomposer (Collembola: Onychiuridae), one macrofauna decomposer (T. pusillus), one mesofauna predator (Uropodina: Uropoda cassidea), and three macrofauna predators (Chilopoda: Strigamia acuminate and Schendyla nemorensis, and Pseudoscorpionida: Neobisium carcinoides). Animals were crushed and fatty acids were extracted by shaking in 5 ml single phase extraction solvent (chloroform - methanol - 0.05 M phosphate buffer, 1:2:0.8, pH 7.4) overnight, for details see Haubert et al. (2004). Neutral lipid fatty acids were dried in a rotation vacuum concentrator, saponified, methylated, and washed following the procedures given for the Sherlock Microbial Identification System (MIDI Inc., Newark, NJ, United States; see Ruess et al., 2002). Then, the lipid fraction was transferred into test tubes and stored at −20°C until analysis via gas chromatography. The gas chromatograph (CLARUS 500, Perkin Elmer, Waltham, United States) was equipped with a flame ionization detector and an Elite-5 capillary column (30 m × 0.25 mm i.d., 0.25 μm film thickness; Perkin Elmer, Waltham, United States). Fatty acid methyl esters (FAMEs) were identified by comparing retention times of samples with standard mixtures composed of 37 different FAMEs ranging from C11 to C24 and bacterial FAMEs, for details see Ferlian and Scheu (2013). NLFAs are given in percentages of total fatty acids extracted from the respective sample. The following FAs served as biomarkers for bacteria: i15:0, a15:0, i16:0, i17:0, cy17:0 and cy19:0, and 16:1ω7 and 18:1ω7 (Ruess and Chamberlain, 2010). The 18:1ω9-to-18:2ω6,9 FA ratio was used as indicator for the relative contribution of plants as compared to fungi to the diet of the animals (Ruess et al., 2007).
Statistical Analyses
Statistical analyses were performed using R v 4.0.2 (R Core Team, 2020). To inspect for the effect of trenching (control, trenching), forest type (beech old, beech young and beech natural, conifer), region (Schorfheide, Hainich) and sampling date (2012, 2014) on the abundance of the investigated order-level animal taxa, linear mixed effects models (LME) in the “nlme” package (Pinheiro et al., 2013) were conducted with the random term sampling date nested in plot identity (Plot ID/Sampling date) to account for control and trenched subplots being nested in Sampling subplots and Plot ID. To inspect for the effect of trenching, forest type and region on biomass and abundance of the investigated taxa and abundant species after 3 years of trenching (2014 data only) linear mixed effects models (LME) in the “nlme” package were conducted with the random term plot identity (Plot ID) to account for control and trenched subplots being nested in Plot ID. If necessary data were log(x + 1) transformed to improve homogeneity of variances. The effect of trenching, forest type and region on the community composition of each Collembola, Oribatida, macrofauna decomposers, and macrofauna predators from the 2014 sampling were analyzed using pManova (permutation multivariate analysis of variance) of species scores from non-metric multidimensional scaling (NMDS). NMDS was carried out using the metaMDS function in the “vegan” package (Oksanen et al., 2018). pManova was performed with Euclidean distance matrices and type II sum of square tests using the adonis. II function in the “RVAideMemoire” package (Hervé, 2020) with restricted permutation within Plot ID using strata with 9,999 permutations. To correct for multiple testing of the abundance, biomass species richness, and functional groups of Nematoda we used the Benjamini-Hochberg (BH) correction (Benjamini and Hochberg, 1995) with a false discovery rate of 5% for each of the four response variables. Corrected p-values were calculated using method “BH” in the “p.adjust” function in R.
To analyze if root trenching affected the trophic niche of soil animal species, the proportion of fungal marker, plant marker and sum of bacterial marker fatty acids in Onychiuridae, T. pusillus, U. cassidea, S. acuminata and N. carcinoides as well as 13C and 15N signatures of Lumbricidae and T. pusillus were analyzed using linear mixed effects models (LME) in the “nlme” package with the random term plot identity (Plot ID).
To analyze if root trenching and forest type affected the NLFA composition of each Onychiuridae, T. pusillus, U. cassidea, S. acuminata, and N. carcinoides of the 2015 sampling DFA after NMDS was carried out in Statistica version 12 (Dell Inc., Tulsa, OK, United States). Data provided in the text represent means and standard deviations.
Results
Abundance and Biomass
Macrofauna. Root trenching did not affect the sum of soil macrofauna abundance (Supplementary Table S1). Only the abundance of Chilopoda and Coleoptera larvae was significantly lower in trenched plots, each by −23% (Figure 1C).
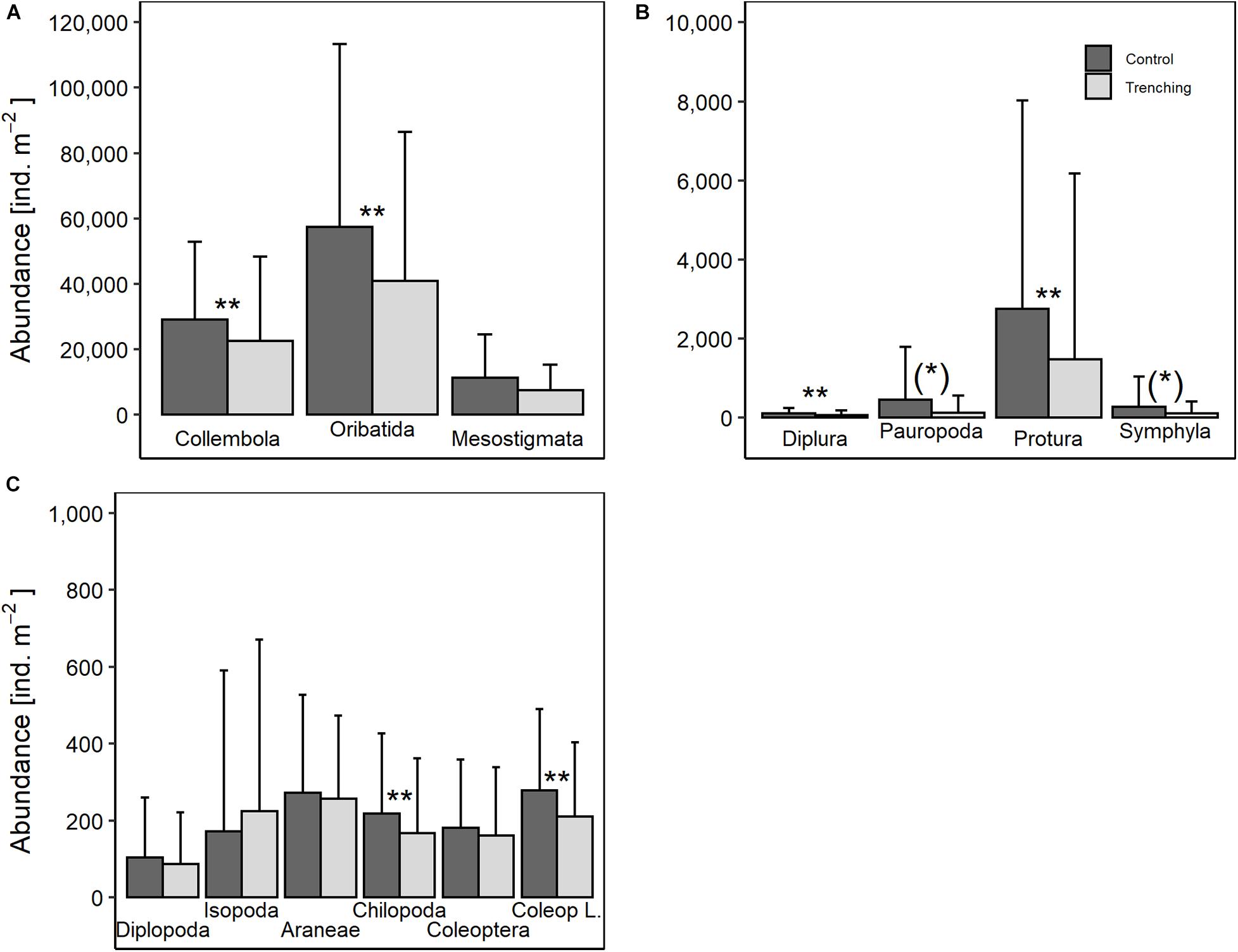
Figure 1. Effect of root trenching on the abundance of mesofauna (A,B) and macrofauna taxa (C); note different scale of y-axis in (A,B); means and standard deviation pooled for forest type, region and sampling date. (*)p < 0.1; *p < 0.05; **p < 0.01; for statistical analyses see Supplementary Table S1.
After 3 years, the biomass of the three macrofauna taxa (Isopoda, Chilopoda, Diplopoda) and that of Lumbricidae was not significantly affected by root trenching (Figure 2 and Supplementary Table S2). However, the abundance of the decomposer species Allajulus nitidus (Diplopoda) [F(1,25) = 5.67, p = 0.025] and the predator species Monotarsobius crassipes (Chilopoda) [F(1,25) = 4.68, p = 0.040] was significantly lower in trenched plots by more than −50% (8.7 ± 4.8 and 7.7 ± 3.6 ind. m–2, respectively) compared to the control (18.6 ± 6.5 and 17.7 ± 6.2 ind. m–2, respectively). By contrast, the abundance of the decomposer species T. pusillus (Isopoda) was higher in trenched plots by +30% (259 ± 98.5 ind. m–2) compared to the control (196 ± 93.4 ind. m–2), but this was only marginally significant [F(1,29) = 3.36, p = 0.0643].
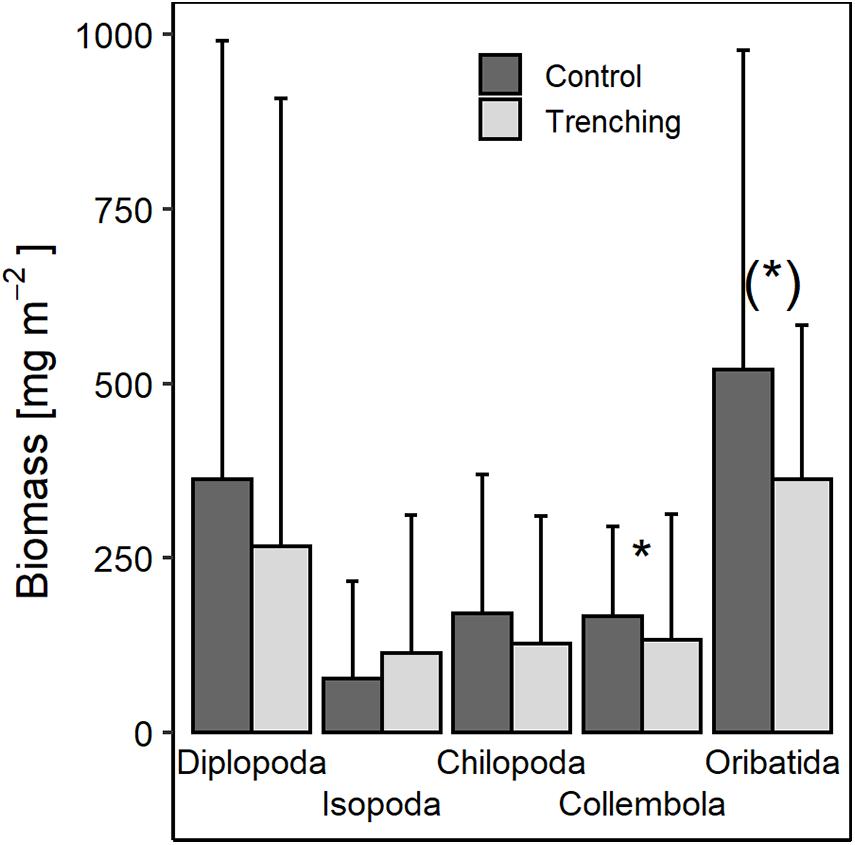
Figure 2. Effect of root trenching on the biomass of soil macrofauna (Diplopoda, Isopoda, and Chilopoda) and mesofauna (Collembola and Oribatida) 3 years after root trenching; means and standard deviation pooled for forest type and region. (*)p < 0.1; *p < 0.05; for statistical analyses see Supplementary Table S2.
Mesofauna. Irrespective of sampling date, total mesofauna abundance was significantly lower in trenched plots by −31%, with the abundance of Oribatida and Collembola being lowered to a similar extent (−29 and −22%, respectively) (Figure 1A and Supplementary Table S1). The abundance of Diplura was lower in trenched plots (−40%), but also their overall density was much lower than that of Oribatida and Collembola (Figure 1B). The abundance of Protura also was lower in trenched plots (Figure 1B). For Pauropoda the effect of root trenching depended on region, in the Hainich the abundance was lower in trenched plots by −85% while in the Schorfheide the abundance was similar in trenched and control plots, but this was only marginally significant after BH correction (Supplementary Table S1). Generally, the effect of root trenching did not vary significantly with forest type.
In addition to the significantly lower abundance of Collembola in trenched plots (−34%; Figure 1A and Supplementary Table S1) after 3 years Collembola biomass also was lower in trenched compared to control plots by −20% (Figure 2 and Supplementary Table S2). The lower biomass of Collembola in trenched plots was mainly due to hemiedaphic Collembola with their biomass in trenched plots (70.8 ± 66.1 mg m–2) being lower by −42% compared to control plots [40.7 ± 61.1 mg m–2; F(1,27) = 11.95, p = 0.002]. Similarly, the lower abundance of Oribatida in trenched plots (−36%; Figure 1A and Supplementary Table S1) was associated by −30% lower biomass of Oribatida in trenched compared to control plots, but this was only marginally significant (Figure 2).
In addition to abundance and biomass, in Oribatida also species richness was lower in trenched (13.4 ± 4.6 species per soil core) compared to control plots (15.6 ± 4.5 species per soil core; Supplementary Table S2), but this was only marginally significant after BH correction. By contrast, although the abundance of Collembola and Oribatida was reduced in trenched plots to a similar extent, in Collembola species richness did not change significantly with root trenching.
Microfauna. After 3 years, the abundance of plant feeding nematodes in trenched plots (44,029 ± 21,519 ind. m–2) was lower by −58% than in control plots (103,769 ± 34,519 ind. m–2; Supplementary Table S2), but this was only marginally significant after BH correction. Nematodes feeding on fungi generally did not significantly respond to root trenching.
Community Structure
Macrofauna community composition did not change significantly with root trenching [pManova; F(1,59) = 0.99, p = 0.12; Figure 3A], and this was true for both macrofauna decomposers as well as macrofauna predators. Similarly, neither the community structure of Oribatida [pManova; F(1,63) = 0.50, p = 0.49; Figure 3B] nor that of Collembola [pManova; F(1,63) = 1.17, p = 0.25; Figure 3C] nor that of Nematoda [pManova; F(1,31) = 0.36, p = 0.91; Figure 3D] was significantly affected by root trenching.
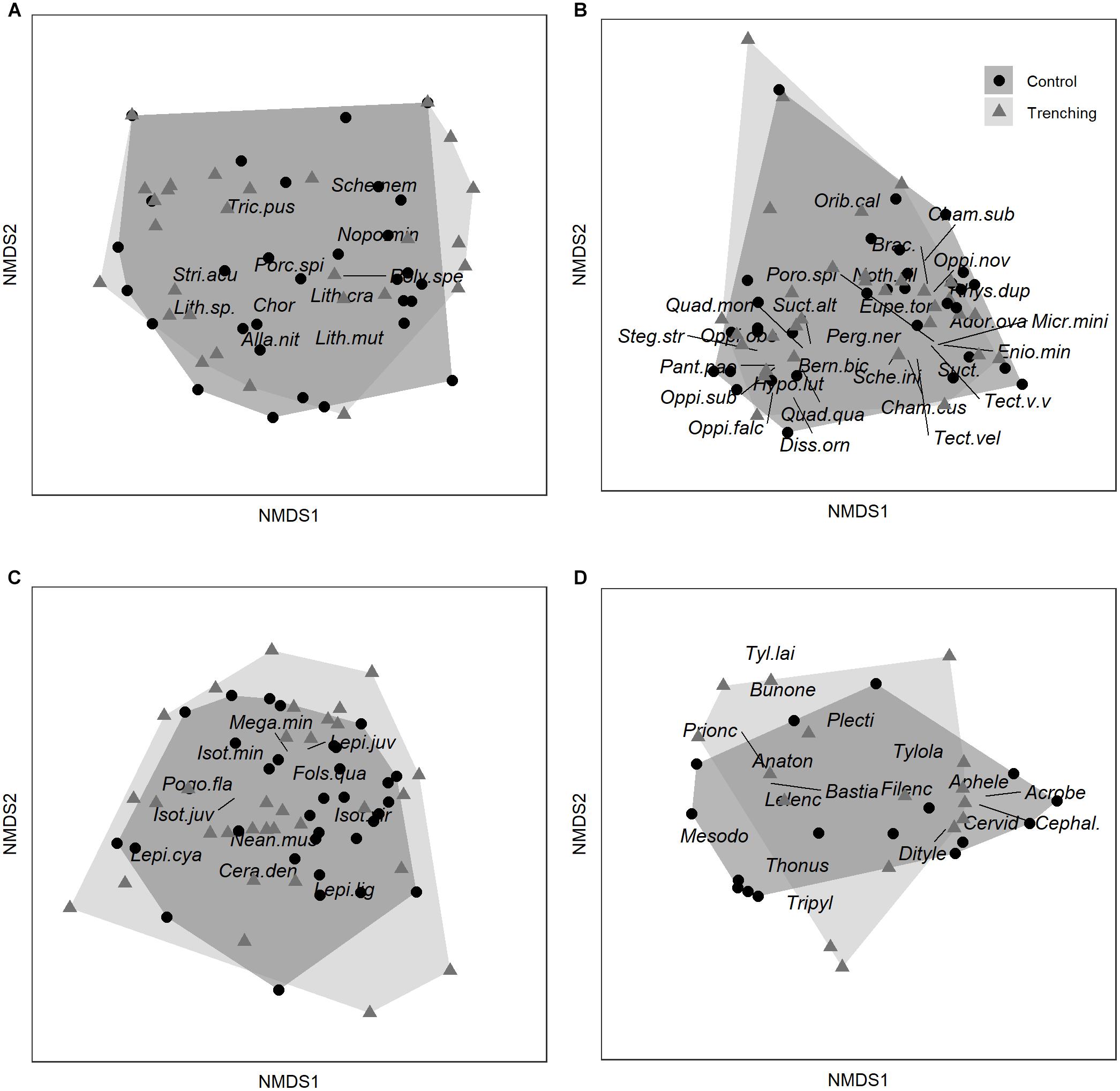
Figure 3. NMDS (non-metric multidimensional scaling) of macrofauna (Diplopoda, Isopoda, Chilopoda) (A), Oribatida (B), Collembola (C), and Nematoda communities (D) in control (Control) and root trenched plots (Trenching), only species significantly correlating with one of the first two NMDS axes shown. (A): Macrofauna (Alla.nit, Allajulus nitidus; Chor, Chordeumatidae; Lith.sp., Lithobius sp.; Lith.cra, Lithobius crassipes; Lith.mut, Lithobius mutabilis; Nopo.min, Nopoiulus minutus; Poly.spe, Polydesmus sp.; Porc.spi, Porcellio spinicornis; Sche.nem, Schendyla nemorensis; Stri.acu, Strigamia acuminata; Tric.pus, Trichoniscus pusillus); (B): Oribatida (Ador.ova, Adoristes ovatus; Bern.bic, Berniniella bicarinata; Brac., Brachychthoniidae; Cham.cus, Chamobates cuspidatus; Cham.sub, Chamobates subglobulus; Diss.orn, Dissorhina ornata; Enio.min, Eniochthonius minutissimus; Eupe.tor, Eupelops torulosus; Hypo.lut, Hypochthonius luteus; Micr.mini, Microtritia minima; Noth.sil, Nothrus silvestris; Oppi.falc, Oppiella falcata; Oppi.nov, Oppiella nova; Oppi.obs, Oppiella obsoleta; Oppi.sub, Oppiella subpectinata; Orib.cal, Oribatella calcarata; Pant.pao, Pantelozetes paolii; Perg.ner, Pergalumna nervosa; Poro.spi, Porobelba spinosa; Quad.mon, Quadroppia monstruosa; Quad.qua, Quadroppia quadricarinata; Rhys.dup, Rhysotritia duplicata; Sche.ini, Scheloribates initialis; Steg.str, Steganacarus striculus; Suct.alt, Suctobelba altvateri; Suct., Suctobelbella spp.; Tect.vel, Tectocepheus velatus sp.; Tect.v.v, Tectocepheus velatus velatus); (C): Collembola (Cera.den, Ceratophysella denticulata; Fols.qua, Folsomia quadroculata; Isot.vir, Isotoma viridis; Isot.juv, Isotomidae juvenil; Isot.min, Isotomiella minor; Lepi.cya, Lepidocyrtus cyaneus; Lepi.juv, Lepidocyrtus juvenil; Lepi.lig, Lepidocyrtus lignorum; Mega.min, Megalothorax minimus; Nean.mus, Neanura muscorum; Pogo.fla, Pogonognathellus flavescens); (D): Nematoda (Acrobe, Acrobeloides; Anaton, Anatonchus; Aphele, Aphelenchoides; Bastia, Bastiania; Bunone, Bunonema; Cephal., Cephalobidae; Cervid, Cervidellus; Dityle, Ditylenchus; Filenc, Filenchus; Lelenc, Lelenchus; Mesodo, Mesodorylaimus; Plecti, Plectidae; Prionc, Prionchulus; Thonus, Thonus; Tripyl, Tripyla; Tyl.lai, Tylencholaimus; Tylola, Tylolaimophorus).
Trophic Shifts
The Δ13C signature of Lumbricidae was not significantly affected by root trenching (Figure 4A). By contrast, the Δ15N signature of two endogeic Lumbricidae taxa (O. lacteum and Aporrectodea sp.) were higher in trenched plots [F(1,4) = 4.63, p = 0.098 and F(1,4) = 4.63, p = 0.098, respectively; Figure 4B]. Further, Δ13C values of T. pusillus in natural beech forests were higher in trenched compared to control plots, while the Δ13C values in old beech forests were lower in trenched compared to control plots [significant Trenching × Forest type interaction; F(1,6) = 10.3, p = 0.018; Figure 4C]. By contrast, Δ15N values of T. pusillus were not significantly affected by root trenching (Figure 4D).
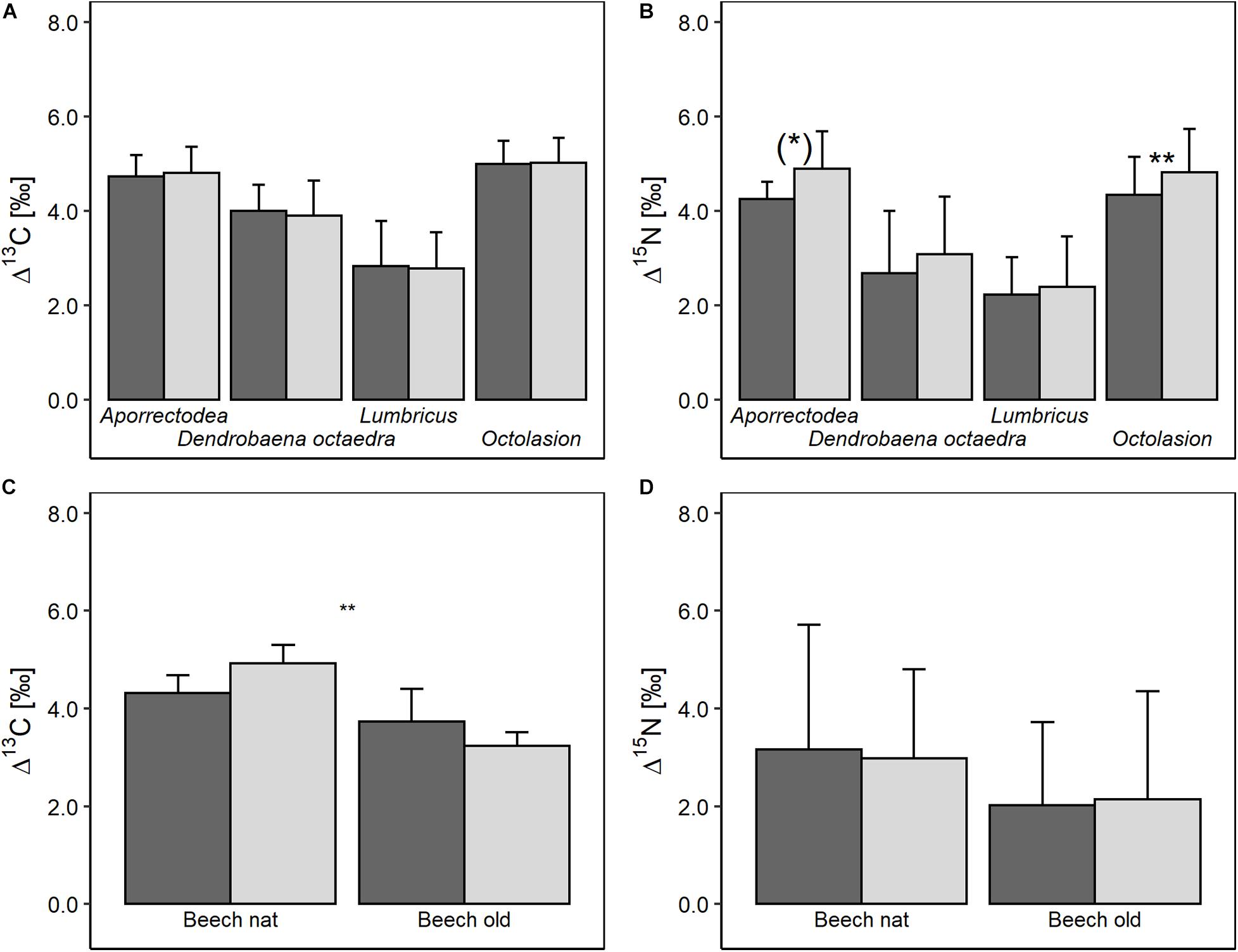
Figure 4. Effect of root trenching on stable isotope values of 13C (A) and 15N (B) of Lumbricidae taxa, and on stable isotope values of 13C (C) and 15N (D) of the isopod Trichoniscus pusillus in natural (Beech nat) and 70 years old beech stands (Beech old). Stable isotope values are calibated against stable isotope values of litter in the respective plot; means and standard deviation. (*)p < 0.1; **p < 0.01.
In addition to stable isotopes, root trenching also affected the fatty acid patterns of consumers. In T. pusillus the proportion of the fungal biomarker fatty acid 18:2ω6,9 was significantly lower in trenched (18.59 ± 4.71%) compared to control plots (23.87 ± 7.25%) [F(1,8) = 7.25, p = 0.027]. Fatty acid patterns in the other analyzed macro- and mesofauna taxa were not significantly affected by root trenching.
Discussion
Body Size-Compartments
Macrofauna. Supporting our hypothesis (1), root trenching detrimentally affected abundance and biomass of soil invertebrates with the effects varying with body size. The response of macrofauna groups varied, but with an average reduction in abundance of −4% in trenched plots the response was less pronounced than in mesofauna. This indicates that macrofauna more intensively integrates different energy channels than mesofauna (Wolkovich, 2016), and supports the suggestion that soil food webs are size-compartmentalized with the use of basal resources, such as bacteria, fungi and plant litter, varying with body size (Potapov et al., 2019a). Notably, the abundance and biomass of macrofauna decomposer groups (Diplopoda, Isopoda) were not significantly reduced by root trenching, indicating that they predominantly feed on litter resources as proposed earlier (Scheu and Falca, 2000; Semenyuk and Tiunov, 2011). Presumably, this is due to the ability of large detritivores to chew up leaf litter material and to use structural organic matter compounds.
Interestingly, in Isopoda the abundance of T. pusillus increased by 30% by root trenching, suggesting that they benefited from the deprivation of root-derived resources. This either reflects that they benefited from decomposing roots cut by trenching the plots or from the decrease in the abundance of Collembola and Oribatida, implying that certain macrofauna detritivore species are competing for food resources with mesofauna detritivores. However, as the biomass of T. pusillus was not affected by root trenching, the overall resources exploited presumably changed little, but trenched plots contained more small T. pusillus (mean dry weight of 0.26 mg in trenched plots compared to 0.28 mg in the control), suggesting that recruitment of juveniles was increased. In Diplopoda, only the density of A. nitidus was reduced after 3 years of root trenching. A. nitidus has been classified as secondary decomposer (Scheu and Falca, 2000) feeding on microorganisms and their remains after death, being an exception compared to other Diplopoda species, which have a narrow trophic niche and predominantly feed on litter and function as primary decomposers (Semenyuk and Tiunov, 2011).
Root trenching also negatively affected the abundance of two macrofauna predators, Chilopoda and Coleoptera larvae (comprising mainly of Staphylinidae), indicating that shortage of belowground resources propagates to higher trophic levels (Ruf et al., 2006; Pollierer et al., 2007). Overall, this argues for the predominance of bottom-up forces and suggests that macrofauna predators rely on mesofauna prey suffering most from deprivation of root-derived resources (see below). Although Chilopoda suffered little from root trenching, the abundance of M. crassipes strongly declined in root trenched plots after 3 years. Again, this supports size-compartmentalization of soil food webs. M. crassipes is a small Chilopoda species exploiting prey from deeper litter layers than other Chilopoda species (Günther et al., 2014; Potapov et al., 2019a), and these prey species are likely to heavily rely on root-derived resources channeled into the soil food web via bacteria as proposed earlier (Ferlian and Scheu, 2013). The abundance and biomass of Lumbricidae was also not significantly affected by root trenching even though soil microbial biomass in trenched plots was reduced by 25% (Bluhm et al., 2019a). This supports earlier findings suggesting that soil microbial biomass is a poor predictor for resource availability for (predominantly endogeic) Lumbricidae (Tiunov and Scheu, 2004), and that endogeic Lumbricidae feed little on root-derived resources (Albers et al., 2006).
Mesofauna. The abundance of soil mesofauna strongly and uniformly declined by root trenching (on average by −31%). This is in line with results of the study of Siira-Pietikäinen et al. (2001) and underlines the importance of root-derived resources for this size-compartment of the soil animal food web. All mesofauna detritivore taxa except Thysanoptera declined due to root trenching. Two pathways might have contributed to this reduction: reduced feeding on living roots and root hairs in particular by Diplura and some Collembola species (Gunn and Cherrett, 1993; Endlweber et al., 2009), or reduced feeding on fungi and bacteria associated with living roots in particular by Collembola, Oribatida and Protura (Fujii et al., 2016; Bluhm et al., 2019b). Indeed, root trenching in our study reduced vital fine roots by 50%, and also bacterial and fungal PLFA markers declined due to root trenching (Bluhm et al., 2019a). For Collembola, Oribatida and Protura it has been shown before that they rely on root-derived resources (Siira-Pietikäinen et al., 2001; Remén et al., 2008; Bluhm et al., 2019b). The fact that the abundance of both Oribatida and Collembola were reduced to a similar level indicates that both taxa are using root-derived resources to a similar extent. Notably, only hemiedaphic Collembola species were significantly affected by root trenching indicating that the importance of root-derived resources varies among ecological groups of Collembola. Supporting this conclusion, recent labeling studies also found ecological groups of Collembola to vary in their use of root-derived resources (Li et al., 2020).
Similar to mesofauna detritivores also mesofauna predators declined due to root trenching. The abundance of Diplura shown to live as predators by stable isotope studies (Scheu and Falca, 2000; Albers et al., 2006) declined by −40% and the density of Mesostigmata showed a decreasing trend (−33%; Figure 1A) indicating that they suffered from the decline in mesofauna prey.
Microfauna. In contrast to our hypothesis (1), root trenching did not significantly reduce the abundance of fungal feeding Nematoda. This suggests that either fungal feeding Nematoda do not feed on ECM fungi or they were able to compensate for the reduction in ECM fungi by switching to feeding on saprotrophic fungi. The findings are in contrast to results of the study of Li et al. (2009), who found the abundance of fungivorous Nematoda to be reduced after tree girdling. However, plant feeding Nematoda showed a trend of being reduced by root trenching, on average by −58%, presumably due to reduced vital root biomass and the inability of root feeding nematodes to switch to other food resources.
Community Structure
In contrast to our hypothesis (1), the community composition (only analyzed after 3 years of root trenching, see “Materials and Methods”) was not affected in macrofauna (Isopoda, Diplopoda, Chilopoda), mesofauna (Oribatida and Collembola), and microfauna (Nematoda). Similarly, Siira-Pietikäinen et al. (2001) documented that, despite the abundance of Oribatida decreased, the community structure remained unaffected due to exclusion of roots in a coniferous forest in Finland. The lack of changes in community structure due to root trenching indicates that virtually all analyzed species suffered to a similar extent from the deprivation of root-derived resources or were independent of it.
Temporal Changes
Contrasting our hypothesis (2), detrimental effects did not increase with time after root trenching. This is consistent with the response of microorganisms to root trenching where the effect even decreased with time suggesting that they compensated for the deprivation of root-derived resources (Bluhm et al., 2019a). Presumably, decomposing roots (and associated mycorrhizal fungi) cut by trenching increased the availability of resources to microorganisms and this may also have alleviated the detrimental effect of root trenching for soil animals later in the experiment (Hagerman et al., 1999).
Variations With Forest Type and Region
Supporting our hypothesis (3), the effect of root trenching generally did not vary with forest type and only little with region. The stronger reduction in microbial biomass due to root trenching in the Hainich compared to the Schorfheide (Bluhm et al., 2019a) was only paralleled in one of the studied taxa of soil animals, i.e., Pauropda. Soils in the Hainich are rich in nutrients and characterized by shallow organic layers, while those in the Schorfheide are poor in nutrients and characterized by thick organic layers. Unfortunately, however, information on food resources of Pauropoda is lacking almost entirely (Starling, 1944; Voigtländer et al., 2016) and the parallel response to microorganisms may not necessarily have been due to trophic interactions.
Trophic Shifts
In contrast to our hypothesis (4), root trenching little affected the composition of food resources of soil animals as indicated by stable isotope and NLFA analysis. The decomposer species T. pusillus (Isopoda) was among the most responsive macrofauna species to root trenching, with its abundance being increased by trenching, but this was only marginally significant after BH correction (see above). Stable isotope analysis showed that the increase in abundance was associated by a shift in the carbon resources used, but the changes varied with forest types and were restricted to the Hainich. Also, in Lumbricidae root trenching resulted in changes in stable isotope values. However, in contrast to T. pusillus, the changes were due to changes in δ15N values of the two endogeic Lumbricidae species analyzed (Hainich only). Presumably, root trenching resulted in a dietary shift towards older, more decomposed carbon resources more enriched in 15N (Kramer et al., 2003; Potapov et al., 2019b).
Unexpectedly, root trenching did not affect the NLFA composition of any of the analyzed detritivore or predator species except in trend for T. pusillus. This likely reflects that, although microbial biomass was reduced strongly by root trenching, it did not alter microbial community composition as measured by PLFA analysis (Bluhm et al., 2019a). In T. pusillus the fungal marker fatty acid showed a trend of being reduced by root trenching indicating that this species switched its diet towards bacteria based diets. This shift paralleled the decreased fungal and increased bacterial PLFAs in trenched plots in the Hainich after 3 years of root trenching (Bluhm et al., 2019a). As the trophic shift in T. pusillus was associated by an increase in abundance but not biomass in trenched plots, the shift only beneficially affected recruitment of juveniles of this species (see above).
Experimental Limitations
Investigating the role of root-derived resources for the belowground system by using root trenching also has limitations. Due to cutting of roots soil moisture might be increased during the growing period due to the lack of water uptake by plants. To control for the reduced water uptake by trees in trenched plots we irrigated the control plots, but this may not have fully equalized soil moisture conditions in control and trenched plots. Further, dead roots in the trenched plots may serve as additional resource for decomposers. However, the general reduction in soil microorganisms as well as soil arthropod taxa in trenched plots argues that additional dead roots were of minor importance as resource for the decomposer food web, at least in the studied upper litter and soil horizons.
Conclusion
Root trenching affected the abundance and biomass of soil animal taxa but the effect depended on the size of the taxa. Macrofauna and microfauna were only slightly decreased due to trenching of roots, while mesofauna declined much stronger. The consistent detrimental effect across mesofauna taxa suggests that energy in soil food webs is channeled via size-based pathways with mesofauna detritivores as well as mesofauna predators relying heavily on root-derived resources. The effect of root trenching generally did not vary with forest type and only little with region. Only one taxon responded parallel to that of microorganisms, suggesting that microbial biomass inadequately reflects basal resources of the soil animal food web. Notably, despite the in part marked reduction in abundance and biomass of soil animal taxa by root trenching, community composition of each micro-, meso-, and macrofauna remained unaffected. This suggests that the species of the detrimentally affected taxa suffered in a similar way from deprivation of root-derived resources, pointing to the dominance of generalist/omnivore species in soil food webs. The lack of or only slight change in the diet of soil animals to root trenching either points to the inability of soil animals to switch diet or reflects that root-derived resources only little affect the composition of microbial communities. Overall, the findings indicate that root-derived resources are predominantly incorporated into soil animal food webs via mesofauna taxa affecting both their abundance and biomass, whereas community composition and trophic niches of soil animals generally are not or only little affected by root-derived resources. The fact that mesofauna detritivores and predators suffered to a similar extent from root trenching points to the dominance of bottom-up forces in regulating this size-compartment of soil animal food webs.
Data Availability Statement
The datasets generated for this study can be found in the online repositories. The names of the repository/repositories and accession number(s) can be found below: the datasets will be public available after acceptance of the manuscript for publication: the datasets IDs 27526, 27487, 27486, 27468, 27467, 27308, 27307, and 27306 for this study can be found in the BExIS (Biodiversity Exploratories Information System), https://www.bexis.uni-jena.de/PublicData/PublicData.aspx.
Author Contributions
BE, OF, CB, MM, and SS: conceptualization. SB: formal analysis. MM and SS: funding acquisition. SB, BE, CB, OF, KH, MC, MM, and SS: investigation and writing—review and editing: SB and SS: visualization and writing—original draft. All authors contributed to the article and approved the submitted version.
Funding
The work has been funded by the German Research Foundation (DFG Priority Program 1374 “Infrastructure-Biodiversity-Exploratories,” DFG- SCHE 376/22-3).
Conflict of Interest
The authors declare that the research was conducted in the absence of any commercial or financial relationships that could be construed as a potential conflict of interest.
Acknowledgments
We are indebted to Andreas Küttner, Bernhard Klarner, Christoph Digel, Dana Augustin, Emily Solly, David Ott, Christel Fischer, Guido Humpert, and Dieter Nünchert for help during field and laboratory work. We thank Anton Potapov for valuable comments on the manuscript. We thank the managers of the two Exploratories Hainich-Dün and Schorfheide-Chorin, Kerstin Wiesner, Katrin Lorenzen, Martin Gorke, and all former managers for their work in maintaining the plot and project infrastructure; Simone Pfeiffer, Christiane Fischer, and Victoria Grießmeier for giving support through the central office, Britta König-Ries, Michael Owonibi, and Andreas Ostrowski for managing the central data base, and Markus Fischer, Eduard Linsenmair, Dominik Hessenmöller, Daniel Prati, Ingo Schöning, François Buscot, Ernst-Detlef Schulze, Wolfgang W. Weisser, and the late Elisabeth Kalko for their role in setting up the Biodiversity Exploratories project. Field work permits were issued by the responsible state environmental offices of Thüringen and Brandenburg.
Supplementary Material
The Supplementary Material for this article can be found online at: https://www.frontiersin.org/articles/10.3389/ffgc.2021.622370/full#supplementary-material
Footnotes
References
Albers, D., Schaefer, M., and Scheu, S. (2006). Incorporation of plant carbon into the soil animal food web of an arable system. Ecology 87, 235–245. doi: 10.1890/04-1728
Bais, H. P., Weir, T. L., Perry, L. G., Gilroy, S., and Vivanco, J. M. (2006). The role of root exudates in rhizosphere interactions with plants and other organisms. Annu. Rev. Plant Biol. 57, 233–266. doi: 10.1146/annurev.arplant.57.032905.105159
Barber, A. D. (2008). Key to the Identification of British Centipedes. Shrewsbury: Field Studies Council.
Bardgett, R. D., and Wardle, D. A. (2010). Aboveground- Belowground Linkages. Biotic Interactions, Ecosystem Processes, and Global Change. Oxford: Oxford University Press.
Beare, M. H., Coleman, D. C., Crossley, D. A., Hendrix, P. F., and Odum, E. P. (1995). A hierarchical approach to evaluating the significance of soil biodiversity to biogeochemical cycling. Plant Soil 170, 5–22. doi: 10.1007/BF02183051
Benjamini, Y., and Hochberg, Y. (1995). Controlling the false discovery rate: a practical and powerful approach to multiple testing. J. R. Stat. Soc. Ser. B 57, 289–300. doi: 10.1111/j.2517-6161.1995.tb02031.x
Blower, J. G. (1985). Millipedes: Keys and Notes for the Identification of the Species. Leiden: Backhuys Publishers.
Bluhm, S. L., Eitzinger, B., Ferlian, O., Bluhm, C., Schröter, K., Pena, R., et al. (2019a). Deprivation of root-derived resources affects microbial biomass but not community structure in litter and soil. PLoS One 14:e0214233. doi: 10.1371/journal.pone.0214233
Bluhm, S. L., Potapov, A. M., Shrubovych, J., Ammerschubert, S., Polle, A., and Scheu, S. (2019b). Protura are unique: first evidence of specialized feeding on ectomycorrhizal fungi in soil invertebrates. BMC Ecol. 19:10. doi: 10.1186/s12898-019-0227-y
Bongers, T. (1990). The maturity index: an ecological measure of environmental disturbance based on nematode species composition. Oecologia 83, 14–19. doi: 10.1007/BF00324627
Bongers, T., and Bongers, M. (1998). Functional diversity of nematodes. Appl. Soil Ecol. 10, 239–251. doi: 10.1016/S0929-1393(98)00123-1
Bonkowski, M., Villenave, C., and Griffiths, B. S. (2009). Rhizosphere fauna: the functional and structural diversity of intimate interactions of soil fauna with plant roots. Plant Soil 321, 213–233. doi: 10.1007/s11104-009-0013-2
Caruso, T., and Migliorini, M. (2009). Euclidean geometry explains why lengths allow precise body mass estimates in terrestrial invertebrates: the case of oribatid mites. J. Theor. Biol. 256, 436–440. doi: 10.1016/j.jtbi.2008.09.033
Cebrian, J. (1999). Patterns in the fate of production in plant communities. Am. Nat. 154, 449–468. doi: 10.1086/303244
Clemmensen, K. E., Bahr, A., Ovaskainen, O., Dahlberg, A., Ekblad, A., Wallander, H., et al. (2013). Roots and associated fungi drive long-term carbon sequestration in boreal forest. Science 339, 1615–1618. doi: 10.1126/science.1231923
Eisenhauer, N., Straube, D., and Scheu, S. (2008). Efficiency of two widespread non-destructive extraction methods under dry soil conditions for different ecological earthworm groups. Eur. J. Soil Biol. 44, 141–145. doi: 10.1016/j.ejsobi.2007.10.002
Eissfeller, V., Beyer, F., Valtanen, K., Hertel, D., Maraun, M., Polle, A., et al. (2013). Incorporation of plant carbon and microbial nitrogen into the rhizosphere food web of beech and ash. Soil Biol. Biochem. 62, 76–81. doi: 10.1016/j.soilbio.2013.03.002
Ekblad, A., Wallander, H., Godbold, D. L., Cruz, C., Johnson, D., Baldrian, P., et al. (2013). The production and turnover of extramatrical mycelium of ectomycorrhizal fungi in forest soils: role in carbon cycling. Plant Soil 366, 1–27. doi: 10.1007/s11104-013-1630-3
Endlweber, K., Ruess, L. R., and Scheu, S. (2009). Collembola switch diet in presence of plant roots thereby functioning as herbivores. Soil Biol. Biochem. 41, 1151–1154. doi: 10.1016/j.soilbio.2009.02.022
Farrar, J., Hawes, M., Jones, D., and Lindow, S. (2003). How roots control the flux of carbon to the rhizosphere. Ecology 84, 827–837.
Ferlian, O., and Scheu, S. (2013). Shifts in trophic interactions with forest type in soil generalist predators as indicated by complementary analyses of fatty acids and stable isotopes. Oikos 123, 1182–1191. doi: 10.1111/j.1600-0706.2013.00848.x
Ferris, H., Bongers, T., and de Goede, R. G. M. (2001). A framework for soil food web diagnostics: extension of the nematode faunal analysis concept. Appl. Soil Ecol. 18, 13–29. doi: 10.1016/S0929-1393(01)00152-4
Fischer, M., Bossdorf, O., Gockel, S., Hänsel, F., Hemp, A., Hessenmöller, D., et al. (2010). Implementing large-scale and long-term functional biodiversity research: the biodiversity Exploratories. Basic Appl. Ecol. 11, 473–485. doi: 10.1016/j.baae.2010.07.009
Fitter, A. H., and Garbaye, J. (1994). Interactions between mycorrhizal fungi and other soil organisms. Plant Soil 159, 123–132. doi: 10.1007/BF00000101
Fujii, S., Mori, A. S., Kominami, Y., Tawa, Y., Inagaki, Y., Takanashi, S., et al. (2016). Differential utilization of root-derived carbon among collembolan species. Pedobiologia 59, 225–227. doi: 10.1016/j.pedobi.2016.05.001
Gilbert, K. J., Fahey, T. J., Maerz, J. C., Sherman, R. E., Bohlen, P., Dombroskie, J. J., et al. (2014). Exploring carbon flow through the root channel in a temperate forest soil food web. Soil Biol. Biochem. 76, 45–52. doi: 10.1016/j.soilbio.2014.05.005
Gisin, H. (1943). ökologie und Lebensgemeinschaften der Collembolen im schweizerischen Exkursionsgebiet Basels. Rev. Suisse Zool. 50, 131–224.
Gunn, A., and Cherrett, J. M. (1993). The exploitation of food resources by soil meso-invertebrates and macro-invertebrates. Pedobiologia 37, 303–320.
Günther, B., Rall, B. C., Ferlian, O., Scheu, S., and Eitzinger, B. (2014). Variations in prey consumption of centipede predators in forest soils as indicated by molecular gut content analysis. Oikos 123, 1192–1198. doi: 10.1111/j.1600-0706.2013.00868.x
Hagerman, S. M., Jones, M. D., Bradfield, G. E., Gillespie, M., and Durall, D. M. (1999). Effects of clear-cut logging on the diversity and persistence of ectomycorrhizae at a subalpine forest. Can. J. For. Res. 29, 124–134. doi: 10.1139/x98-186
Haubert, D., Häggblom, M. M., Scheu, S., and Ruess, L. R. (2004). Effects of fungal food quality and starvation on the fatty acid composition of Protaphorura fimata (Collembola). Comp. Biochem. Physiol. Biochem. Mol. Biol. 138, 41–52. doi: 10.1016/j.cbpc.2004.02.009
Hervé, M. (2020). RVAideMemoire: Testing and Plotting Procedures for Biostatistics. R package version 0.9-77. Available online at: https://CRAN.R-project.org/package=RVAideMemoire
Högberg, M. N., Briones, M. J. I., Keel, S. G., Metcalfe, D. B., Campbell, C., Midwood, A. J., et al. (2010). Quantification of effects of season and nitrogen supply on tree below-ground carbon transfer to ectomycorrhizal fungi and other soil organisms in a boreal pine forest. New Phytol. 187, 485–493. doi: 10.1111/j.1469-8137.2010.03274.x
Högberg, M. N., and Högberg, P. (2002). Extramatrical ectomycorrhizal mycelium contributes one-third of microbial biomass and produces, together with associated roots, half the dissolved organic carbon in a forest soil. New Phytol. 154, 791–795. doi: 10.1046/j.1469-8137.2002.00417.x
Hopkin, S. P. (2007). A Key to the Collembola (Springtails) of Britain and Ireland. Shrewsbury: Field Studies Council.
Jones, D. L., Nguyen, C., and Finlay, R. D. (2009). Carbon flow in the rhizosphere: carbon trading at the soil-root interface. Plant Soil 321, 5–33. doi: 10.1007/s11104-009-9925-0
Kempson, D., Lloyd, M., and Ghelardi, R. (1963). A new extractor for woodland litter. Pedobiologia 3, 1–21.
Klarner, B., Ehnes, R. B., Erdmann, G., Eitzinger, B., Pollierer, M. M., Maraun, M., et al. (2014). Trophic shift of soil animal species with forest type as indicated by stable isotope analysis. Oikos 123, 1173–1181. doi: 10.1111/j.1600-0706.2013.00939.x
Klarner, B., Winkelmann, H., Krashevska, V., Maraun, M., Widyastuti, R., and Scheu, S. (2017). Trophic niches, diversity and community composition of invertebrate top predators (Chilopoda) as affected by conversion of tropical lowland rainforest in Sumatra (Indonesia). PLoS One 12:e0180915. doi: 10.1371/journal.pone.0180915
Koren, A. (1992). Die Chilopodenfauna von Kärnten und Osttirol, Teil 2: Lithobiomorpha. Carinthia II 52, 1–138.
Kramer, M. G., Sollins, P., Sletten, R. S., and Swart, P. K. (2003). N isotope fractionation and measures of organic matter alteration during decomposition. Ecology 84, 2021–2025. doi: 10.1890/02-3097
Kuzyakov, Y., and Blagodatskaya, E. (2015). Microbial hotspots and hot moments in soil: concept & review. Soil Biol. Biochem. 83, 184–199. doi: 10.1016/j.soilbio.2015.01.025
Kuzyakov, Y., and Domanski, G. (2000). Carbon input by plants into the soil. Review. J. Plant Nutr. Soil Sci. 163, 421–431.
Langel, R., and Dyckmans, J. (2014). Combined (13) C and (15) N isotope analysis on small samples using a near-conventional elemental analyzer/isotope ratio mass spectrometer setup. Rapid Commun. Mass Spectrom. 28, 1019–1022. doi: 10.1002/rcm.6878
Latzel, R. (1880). Die Myriopoden der Österreichisch-ungarischen Monarchie. Erste Hälfte: Die Chilopoda. Wien: Hölder.
Lemanski, K., and Scheu, S. (2014). Fertilizer addition lessens the flux of microbial carbon to higher trophic levels in soil food webs of grassland. Oecologia 176, 487–496. doi: 10.1007/s00442-014-3037-0
Li, Y., Yang, X., and Wu, J. (2009). Response of soil nematode communities to tree girdling in a subtropical evergreen broad-leaved forest of southwest China. Soil Biol. Biochem. 41, 877–882. doi: 10.1016/J.SOILBIO.2008.07.031
Li, Z., Scheunemann, N., Potapov, A. M., Shi, L., Pausch, J., Scheu, S., et al. (2020). Incorporation of root-derived carbon into soil microarthropods varies between cropping systems. Biol. Fertil. Soils 56, 839–851. doi: 10.1007/s00374-020-01467-8
Litton, C. M., Raich, J. W., and Ryan, M. G. (2007). Carbon allocation in forest ecosystems. Glob. Chang. Biol. 13, 2089–2109. doi: 10.1111/j.1365-2486.2007.01420.x
Macfadyen, A. (1961). Improved funnel-type extractors for soil arthropods. J. Anim. Ecol. 30, 171–184. doi: 10.2307/2120
Malmström, A., and Persson, T. (2011). Responses of Collembola and Protura to tree girdling – some support for ectomycorrhizal feeding. Soil Org. 83, 279–285.
Mercer, R. D., Gabriel, A. G. A., Barendse, J., Marshall, D. J., and Chown, S. L. (2001). Invertebrate body sizes from Marion Island. Antarct. Sci. 13, 135–143. doi: 10.1017/S0954102001000219
Okada, H., Harada, H., and Kadota, I. (2005). Fungal-feeding habits of six nematode isolates in the genus Filenchus. Soil Biol. Biochem. 37, 1113–1120. doi: 10.1016/J.SOILBIO.2004.11.010
Oksanen, J., Blanchet, F. G., Friendly, M., Kindt, R., Legendre, P., McGlinn, D., et al. (2018). vegan: Community Ecology Package. R Packag. version 2.4–6. Available online at: https//CRAN.R-project.org/package=vegan
Ostle, N. J., Briones, M. J. I., Ineson, P., Cole, L., Staddon, P., and Sleep, D. (2007). Isotopic detection of recent photosynthate carbon flow into grassland rhizosphere fauna. Soil Biol. Biochem. 39, 768–777. doi: 10.1016/j.soilbio.2006.09.025
Parmelee, R. W., Ehrenfeld, J. G., and Tate, R. L. (1993). Effects of pine roots on microorganisms, fauna, and nitrogen availability in two soil horizons of a coniferous forest spodosol. Biol. Fertil. Soils 15, 113–119. doi: 10.1007/BF00336428
Pinheiro, J., Bates, D., DebRoy, S., and Sarkar, D. (2013). nlme: Linear and Nonlinear Mixed Effects Models. R Package Version 3.1-109. Vienna: R Foundation for Statistical Computing.
Pollierer, M. M., Dyckmans, J., Scheu, S., and Haubert, D. (2012). Carbon flux through fungi and bacteria into the forest soil animal food web as indicated by compound-specific 13C fatty acid analysis. Funct. Ecol. 26, 978–990. doi: 10.1111/j.1365-2435.2012.02005.x
Pollierer, M. M., Langel, R., Körner, C., Maraun, M., and Scheu, S. (2007). The underestimated importance of belowground carbon input for forest soil animal food webs. Ecol. Lett. 10, 729–736. doi: 10.1111/j.1461-0248.2007.01064.x
Pollierer, M. M., Langel, R., Scheu, S., and Maraun, M. (2009). Compartmentalization of the soil animal food web as indicated by dual analysis of stable isotope ratios (15N/14N and 13C/12C). Soil Biol. Biochem. 41, 1221–1226. doi: 10.1016/j.soilbio.2009.03.002
Pollierer, M. M., Scheu, S., and Haubert, D. (2010). Taking it to the next level: trophic transfer of marker fatty acids from basal resource to predators. Soil Biol. Biochem. 42, 919–925. doi: 10.1016/j.soilbio.2010.02.008
Post, D. M. (2002). Using stable isotopes to estimate trophic position: models, methods, and assumptions. Ecology 83, 703–718. doi: 10.2307/3071875
Potapov, A. M., Brose, U., Scheu, S., and Tiunov, A. V. (2019a). Trophic position of consumers and size structure of food webs across aquatic and terrestrial ecosystems. Am. Nat. 194, 823–839. doi: 10.1086/705811
Potapov, A. M., Tiunov, A. V., and Scheu, S. (2019b). Uncovering trophic positions and food resources of soil animals using bulk natural stable isotope composition. Biol. Rev. 94, 37–59. doi: 10.1111/brv.12434
Potapov, A. M., and Tiunov, A. V. (2016). Stable isotope composition of mycophagous collembolans versus mycotrophic plants: Do soil invertebrates feed on mycorrhizal fungi? Soil Biol. Biochem. 93, 115–118. doi: 10.1016/j.soilbio.2015.11.001
R Core Team (2020). “R: A language and environment for statistical computing”, in R Foundation for Statistical Computing, Vienna. Available online at: https://www.R-project.org/
Reineking, A., Langel, R., and Schikowski, J. (1993). C-On-line Measurements with an Elemental Analyser (Carlo Erba, Isotopenpraxis. Isot. Environ. Health Sci. 29, 169–174. doi: 10.1080/10256019308046151
Remén, C., Persson, T., Finlay, R. D., and Ahlström, K. (2008). Responses of oribatid mites to tree girdling and nutrient addition in boreal coniferous forests. Soil Biol. Biochem. 40, 2881–2890. doi: 10.1016/j.soilbio.2008.08.006
Ruess, L. (1995). Studies on the nematode fauna of an acid forest soil: spatial distribution and extraction. Nematologica 41, 229–239. doi: 10.1163/003925995X00198
Ruess, L. R., and Chamberlain, P. M. (2010). The fat that matters: soil food web analysis using fatty acids and their carbon stable isotope signature. Soil Biol. Biochem. 42, 1898–1910. doi: 10.1016/j.soilbio.2010.07.020
Ruess, L. R., Häggblom, M. M., Zapata, E. J. G., and Dighton, J. (2002). Fatty acids of fungi and nematodes—possible biomarkers in the soil food chain? Soil Biol. Biochem. 34, 745–756.
Ruess, L. R., Schütz, K., Migge-Kleian, S., Häggblom, M. M., Kandeler, E., and Scheu, S. (2007). Lipid composition of Collembola and their food resources in deciduous forest stands—Implications for feeding strategies. Soil Biol. Biochem. 39, 1990–2000. doi: 10.1016/j.soilbio.2007.03.002
Ruf, A., Kuzyakov, Y., and Lopatovskaya, O. (2006). Carbon fluxes in soil food webs of increasing complexity revealed by 14C labelling and 13C natural abundance. Soil Biol. Biochem. 38, 2390–2400. doi: 10.1016/j.soilbio.2006.03.008
Schaefer, M., Ansorge, H., Brehm, G., Fiedler, K., Sattler, K., Scheu, S., et al. (2018). Brohmer - Fauna von Deutschland, 25th Edn, ed. M. Schaefer (Wiebelsheim: Quelle & Meyer).
Scheu, S., and Falca, M. (2000). The soil food web of two beech forests (Fagus sylvatica) of contrasting humus type: stable isotope analysis of a macro- and a mesofauna-dominated community. Oecologia 123, 285–296. doi: 10.1007/s004420051015
Scheu, S., and Schaefer, M. (1998). Bottom-up control of the soil macrofauna community in a beechwood on limestone: manipulation of food resources. Ecology 79, 1573–1585. doi: 10.2307/176777
Scheunemann, N., Maraun, M., Scheu, S., and Butenschoen, O. (2015). The role of shoot residues vs. crop species for soil arthropod diversity and abundance of arable systems. Soil Biol. Biochem. 81, 81–88. doi: 10.1016/j.soilbio.2014.11.006
Scheunemann, N., Pausch, J., Digel, C., Kramer, S., Scharroba, A., Kuzyakov, Y., et al. (2016). Incorporation of root C and fertilizer N into the food web of an arable field: variations with functional group and energy channel. Food Webs 9, 39–45. doi: 10.1016/j.fooweb.2016.02.006
Semenyuk, I. I., and Tiunov, A. V. (2011). Isotopic signature (15N/14N and 13C/12C) confirms similarity of trophic niches of millipedes (Myriapoda, Diplopoda) in a temperate deciduous forest. Biol. Bull. 38, 283–291. doi: 10.1134/S1062359011030137
Siira-Pietikäinen, A., Haimi, J., and Fritze, H. (2003). Organisms, decomposition and growth of pine seedlings in boreal forest soil affected by sod cutting and trenching. Biol. Fertil. Soils 37, 163–174. doi: 10.1007/s00374-002-0571-4
Siira-Pietikäinen, A., Haimi, J., Kanninen, A., Pietikäinen, J., and Fritze, H. (2001). Responses of decomposer community to root-isolation and addition of slash. Soil Biol. Biochem. 33, 1993–2004. doi: 10.1016/S0038-0717(01)00135-3
Sims, R. W., and Gerard, B. M. (1985). Earthworms: Keys and Notes for the Identification and Study of the Species. Leiden: Brackish-Water Sciences.
Sohlström, E. H., Marian, L., Barnes, A. D., Haneda, N. F., Scheu, S., Rall, B. C., et al. (2018). Applying generalized allometric regressions to predict live body mass of tropical and temperate arthropods. Ecol. Evol. 8, 12737–12749. doi: 10.1002/ece3.4702
Sprengel, T. (1986). Die Doppelfüßer (Diplopoda) eines Kalkbuchenwaldes und ihre Funktion beim Abbau der Laubstreu. Ph.D. dissertation, Universität Göttingen, Göttingen.
Starling, J. H. (1944). Ecological studies of the Pauropoda of the Duke Forest. Ecol. Monogr. 14, 291–310. doi: 10.2307/1948445
Tan, K. H. (2014). Humic Matter in Soil and the Environment: Principles and Controversies, 2nd Edn. Oakville: CRC Press.
Tiunov, A. V., and Scheu, S. (2004). Carbon availability controls the growth of detritivores (Lumbricidae) and their effect on nitrogen mineralization. Oecologia 138, 83–90. doi: 10.1007/s00442-003-1391-4
Traugott, M., Kamenova, S., Ruess, L., Seeber, J., and Plantegenest, M. (2013). Empirically Characterising Trophic Networks: What Emerging DNA- Based Methods, Stable Isotope and Fatty Acid Analyses Can Offer. Adv. Ecol. Res. 49, 177–224. doi: 10.1016/B978-0-12-420002-9.00003-2
Treseder, K. K., Torn, M. S., and Masiello, C. A. (2006). An ecosystem-scale radiocarbon tracer to test use of litter carbon by ectomycorrhizal fungi. Soil Biol. Biochem. 38, 1077–1082. doi: 10.1016/j.soilbio.2005.09.006
van Hees, P. A. W., Jones, D. L., Finlay, R., Godbold, D. L., and Lundström, U. S. (2005). The carbon we do not see—the impact of low molecular weight compounds on carbon dynamics and respiration in forest soils: a review. Soil Biol. Biochem. 37, 1–13. doi: 10.1016/j.soilbio.2004.06.010
Vitousek, P. M., Hättenschwiler, S., Olander, L., and Allison, S. (2002). Nitrogen and nature. Ambio 31, 97–101. doi: 10.1579/0044-7447-31.2.97
Voigtländer, K., Decker, P., Burkhardt, U., and Spelda, J. (2016). The present knowledge of the Symphyla and Pauropoda (Myriapoda) in Germany – An annotated checklist. Acta Soc. Zool. Bohem. 80, 51–85.
Wallander, H., Göransson, H., and Rosengren, U. (2004). Production, standing biomass and natural abundance of 15N and 13C in ectomycorrhizal mycelia collected at different soil depths in two forest types. Oecologia 139, 89–97. doi: 10.1007/s00442-003-1477-z
Wardle, D. A. (2002). Communities and Ecosystems: Linking the Aboveground and Belowground Components. Princeton, NJ: Princeton University Press.
Werner, R. A., Bruch, B. A., and Brand, W. A. (1999). ConFlo III - an interface for high precision δ13C and δ15N analysis with an extended dynamic range. Rapid Commun. Mass Spectrom. 13, 1237–1241.
White, T. C. R. (1993). The Inadequate Environment: Nitrogen and the Abundance of Animals. Berlin: Springer. doi: 10.1007/978-3-642-78299-2
Wolkovich, E. M. M. (2016). Reticulated channels in soil food webs. Soil Biol. Biochem. 102, 18–21. doi: 10.1016/j.soilbio.2016.06.021
Yeates, G. W., Bongers, T., De Goede, R. G., Freckman, D. W., and Georgieva, S. S. (1993). Feeding habits in soil nematode families and genera-an outline for soil ecologists. J. Nematol. 25, 315–331.
Zieger, S. L., Ammerschubert, S., Polle, A., and Scheu, S. (2017a). Root-derived carbon and nitrogen from beech and ash trees differentially fuel soil animal food webs of deciduous forests. PLoS One 12:e0189502. doi: 10.1371/journal.pone.0189502
Zieger, S. L., Holczinger, A., Sommer, J., Rath, M., Kuzyakov, Y., Polle, A., et al. (2017b). Beech trees fuel soil animal food webs via root-derived nitrogen. Basic Appl. Ecol. 22, 28–35. doi: 10.1016/j.baae.2017.06.006
Keywords: root-derived resources, mesofauna, macrofauna, trenching, forest, soil food web, stable isotopes, NLFA
Citation: Bluhm SL, Eitzinger B, Bluhm C, Ferlian O, Heidemann K, Ciobanu M, Maraun M and Scheu S (2021) The Impact of Root-Derived Resources on Forest Soil Invertebrates Depends on Body Size and Trophic Position. Front. For. Glob. Change 4:622370. doi: 10.3389/ffgc.2021.622370
Received: 28 October 2020; Accepted: 03 February 2021;
Published: 04 March 2021.
Edited by:
Alberto Canarini, Kyoto University, JapanReviewed by:
Andrey S. Zaitsev, University of Giessen, GermanyMaria Minor, Massey University, New Zealand
Copyright © 2021 Bluhm, Eitzinger, Bluhm, Ferlian, Heidemann, Ciobanu, Maraun and Scheu. This is an open-access article distributed under the terms of the Creative Commons Attribution License (CC BY). The use, distribution or reproduction in other forums is permitted, provided the original author(s) and the copyright owner(s) are credited and that the original publication in this journal is cited, in accordance with accepted academic practice. No use, distribution or reproduction is permitted which does not comply with these terms.
*Correspondence: Sarah L. Bluhm, c3ppZWdlckBnd2RnLmRl
†Present address: Bernhard Eitzinger, Chair of Nature Conservation & Landscape Ecology, University of Freiburg, Freiburg, Germany; Christian Bluhm, Forstliche Versuchs- und Forschungsanstalt Baden-Württemberg, Freiburg, Germany; Olga Ferlian, Institute of Biology, Leipzig University, Leipzig, Germany; German Centre for Integrative Biodiversity Research (iDiv) Halle-Jena-Leipzig, Leipzig, Germany