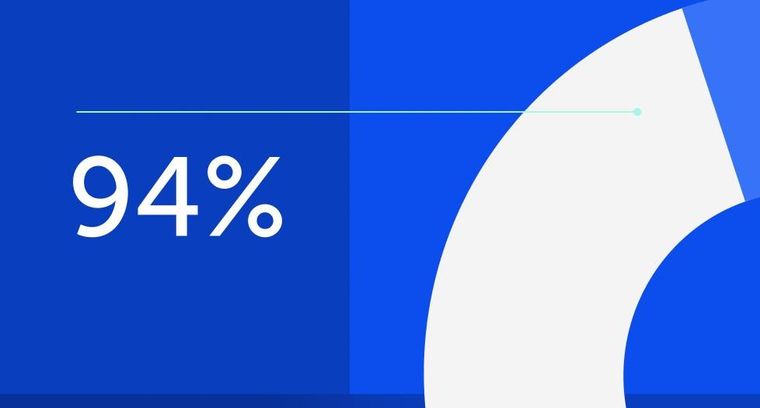
94% of researchers rate our articles as excellent or good
Learn more about the work of our research integrity team to safeguard the quality of each article we publish.
Find out more
ORIGINAL RESEARCH article
Front. For. Glob. Change, 13 May 2021
Sec. Tropical Forests
Volume 4 - 2021 | https://doi.org/10.3389/ffgc.2021.620450
This article is part of the Research TopicIntact ForestsView all 18 articles
Pre-settlement New England was heavily forested, with trees exceeding 2 m in diameter. The forests have regrown since farm abandonment, representing what is arguably the most successful regional reforestation on record and identified recently in the “Global Safety Net.” Temperate “old-growth” forest and remnant stands demonstrate that native tree species can live several hundred years and continue to add to forest biomass and structural and ecological complexity. Forests globally are an essential natural climate solution that accumulate carbon and reduce annual increases in atmospheric CO2 by approximately 30%. Some studies emphasize young, fast-growing trees and forests while others highlight carbon storage and accumulation in old trees and intact forests. We addressed this directly within New England with long-term, accurate field measurements and volume modeling of individual trees and two stands of eastern white pines (Pinaceae: Pinus strobus) and compared our results to models developed by the U.S. Forest Service. Within this sample and species, our major findings complement and clarify previous findings and are threefold: (1) beyond 80 years, an intact eastern white pine forest can accumulate carbon above-ground in living trees at a high rate and double the carbon stored in this compartment in subsequent years; (2) large trees dominate above-ground carbon and can continue to accumulate carbon; (3) productive stands can continue to accumulate high amounts of carbon in live trees for well over 150 years. Because the next decades are critical in addressing the climate emergency, and most New England forests are less than 100 years old, a major implication of this work is that maintaining and accumulating carbon in some existing forests—proforestation—is a powerful regional climate solution. Furthermore, older and old-growth trees and forests are rare, complex, highly dynamic and biodiverse: dedication of some forests to proforestation will produce large carbon-dense trees and also protect ecosystem integrity, special habitats, and native biodiversity long-term. In sum, strategic policies to grow and protect suitable existing forests in New England will optimize a proven, low cost, natural climate solution that also protects and restores biodiversity across the landscape.
A global priority for the climate has long been reducing ongoing emissions of heat-trapping greenhouse gases (GHGs) produced by burning carbon-based fuels. While this is essential, it is not sufficient for halting the rise in global temperatures. It is necessary to also simultaneously increase carbon dioxide (CO2) removal (CDR) and keep carbon stored within natural systems. Clearing and harvesting forests, draining and developing wetlands, and degrading soils account for one-third of all the CO2 added to the atmosphere by humans since the beginning of the industrial revolution (Simmons and Matthews, 2016). Together, these ongoing actions continue to add approximately 1.6 PgC/year (1 Pg equals 1 Gt or 1015 grams or 1 billion metric tons; Friedlingstein et al., 2020). Burning wood for heat and electricity adds additional CO2, and current forest management practices limit the potential of this natural solution to accumulate carbon above and below ground and keep it out of the atmosphere (Sterman et al., 2018).
Two recent Intergovernmental Panel on Climate Change (IPCC) reports identify the urgent and unprecedented imperative to simultaneously and rapidly reduce Carbon Dioxide Emissions and achieve additional Carbon Dioxide Removal (CDR) from the atmosphere (Intergovernmental Panel on Climate Change, 2018, 2019). These reports identify forests as playing a major role in accumulating carbon out of the atmosphere. However, for CDR the focus is primarily on afforestation (planting new forests) and reforestation (regrowing forests) and ignores the more rapid climate mitigation and adaptation benefits of additional growth by existing forests, termed “proforestation” (Moomaw et al., 2019).
Even achieving the goal of “zero net carbon” will only “probably” limit global average temperatures to 1.5∘C (Intergovernmental Panel on Climate Change, 2018) above the pre-industrial global temperature and a significant increase above the current level (∼1.2∘C). This additional temperature increase will result in greater disruption to the climate system and will accelerate ecological decline. To avoid ever more serious consequences of a changed climate, the goal must be to become net carbon negative as soon as possible. Growing suitable existing forests is an effective and low cost means for reducing the atmospheric stock of carbon as others have noted (Fargione et al., 2018; Hudiburg et al., 2019; Moomaw et al., 2019; Mildrexler et al., 2020) and will be demonstrated by the findings reported in this paper. Natural regeneration of forests has recently been found to accumulate more carbon in the first 30 years than managed reforestation (Cook-Patton et al., 2020).
A second and perhaps even more urgent priority is the strong protection of intact biodiverse natural systems (Watson et al., 2018), as verified in the Global Assessment Report on Biodiversity and Ecosystem Services (Intergovernmental Science-Policy on Biodiversity and Ecosystem Services, 2019) and the recent “Global Deal for Nature”(Dinerstein et al., 2019). A global review with a dual focus on carbon and biodiversity identified regions that are part of a “Global Safety Net” (Dinerstein et al., 2020), and the safety net must be now be translated to local levels. This joint climate/biodiversity priority was also highlighted in the peer-reviewed declaration of a Climate Emergency signed by over 13,000 scientists in late 2019 and which highlighted proforestation as a global climate solution (Ripple et al., 2020).
There is scientific consensus that we can substantially close the gap between CO2 emissions and removals by maximizing a range of nature-based solutions (Griscom et al., 2017; Fargione et al., 2018). Regarding biodiversity, the beneficial role of protected areas in supporting species abundance and diversity was confirmed in a global meta-analysis (Coetzee et al., 2014), and the benefit of protecting intact ecosystems was quantified by comparing the probability of extinction in the six major global regions. On average, “wilderness” reduces the rate of species’ extinction by half due to higher rates of species loss in unprotected areas (Di Marco et al., 2019); the quantified benefit of wilderness in preventing extinction is even higher in regions, including the Eastern United States. Biodiverse intact forests can simultaneously provide long-term protection to natural processes and biodiversity, reduce extinction, and provide pathways for migration while accumulating atmospheric carbon moderating local and global temperature increases (Friedlingstein et al., 2020). Taken together, it is practical and possible to act immediately to protect ecosystems and prevent extinction while we maintain increased CDR rates and store and accumulate additional carbon in forests and forest soils.
Forest conservation studies tend to focus on high-biodiversity tropical forests (Mitchard, 2018), yet temperate forests are also biodiverse (Hilmers et al., 2018), benefit human health and well-being in highly populated areas (Karjalainen et al., 2010), and provide many essential ecosystem services (United States Forest Service, 2021). They also have a large additional potential for CDR that has been underestimated by 32% (Cook-Patton et al., 2020). New England Acadian Forests are the only region in the lower 48 United States identified as part of the “Global Safety Net” as a Tier 1 climate stabilization area (Dinerstein et al., 2020). Current forest CDR in the United States reduces annual net nation-wide greenhouse gas emissions by 11.6% (United States Environmental Protection, and Agency, 2018), with the potential for much more (Keeton et al., 2011; Moomaw et al., 2019). Houghton and Nassikas (2018) estimate the current gross carbon sink in forests recovering from harvests and in abandoned agriculture to be −4.4 PgC/year (negative means removal) globally, consistent with the IPCC 1.5°C report that identified forests as key to increasing accumulation rates. This potential carbon sink from recovering forests is nearly as large as the gap between anthropogenic emissions and removal rates, 5.1 PgC/year (Friedlingstein et al., 2020).
In the context of resource production and forest management, some forest carbon is stored in lasting wood products, and responsible forestry can provide a reliable wood supply from a semi-natural forest. However, multiple analyses have found that more carbon associated with timber harvests is lost to the atmosphere than is stored in the harvested wood products (Nunery and Keeton, 2010; Harris et al., 2016). For example, just 19% of the original carbon stock in Oregon forests in 1900 is in long lived wood products; approximately 16% is in landfills, and the remaining 65% is in the atmosphere as carbon dioxide (Hudiburg et al., 2019). Updated models indicate that the product substitution benefits of wood products are overestimated between 2 and 100-fold (Harmon, 2019) and any near-term carbon benefit relies on product subsitution (Hudiburg et al., 2019; Leturcq, 2020). Biogenic emissions from harvesting in the United States are estimated to be 640 MtC/year or 85% of total forestry emissions, exceeding the commercial and residential building sectors, and fossil fuel emissions from harvesting add an additional 17% CO2 to the atmosphere above biogenic emissions (Harris et al., 2016).
Strategic planning for responsible resource production can both mitigate these emissions and ensure a protected network of intact natural areas. For example, the US Climate Alliance underestimates the importance of “net carbon accumulation” in forests (United States Climate Alliance, 2021). Forests do accumulate net carbon now, but carbon above and below ground is far below historic levels and far below its potential (Law et al., 2018; Hudiburg et al., 2019). A critical and explicit goal is to increase and optimize carbon accumulation by utilizing some forests for responsible resource production as needed and protecting other forests for climate protection, long-term full biodiversity, science, and human health and well-being.
At a global level, if deforestation were halted, and existing secondary forests allowed to continue growing, a network of these intact forests would protect the highest number of species from extinction (Di Marco et al., 2019; World Wildlife Federation, 2020) and it is estimated that they could accumulate ∼120 PgC in the 84 years between 2016 and 2100 (Houghton and Nassikas, 2018). This is equivalent to about 12 years of current global fossil fuel carbon emissions. These global numbers are conservative as outlined in recent analyses (Cook-Patton et al., 2020) and they do not factor in the enhanced regional CDR potential and high cumulative carbon that can be achieved with proforestation in such carbon-dense temperate forests of the Pacific Northwest (Law et al., 2018) and New England (Nunery and Keeton, 2010; Keeton et al., 2011; Moomaw et al., 2019; Dinerstein et al., 2020).
Because these global and regional projections can be difficult to translate locally, particularly over time, we focused on a detailed analysis of individual trees and stands in New England. Historically, between 80 and 90% of the New England landscape was heavily forested, and early chroniclers describe pre-settlement forests with many large, mature trees reaching 1–1.5 m in diameter (Whitney, 1996). Fast-growing riparian species like sycamores and cottonwoods could reach or exceed 2 m. Today, New England trees of this size are mostly found as isolated individuals in open areas, parks, and old estates. Old-growth forests (primary forests) and remnants are currently less than 0.2% of northern New England’s landscape, and less than 0.03% in Southern New England. Ongoing attempts to document their value and identify their locations is underway (Davis, 1996; Kershner and Leverett, 2004; Ruddat, 2020). Secondary forests in New England consist mostly of smaller, relatively young trees (on average less than 100 years old). The U.S. Forest Service estimates that fewer than 7% of the nation’s forests exceed 100 years in age.
Our goal in this study was to measure carbon directly in individual trees and in an “average” vs. an older stand of eastern white pine (Pinaceae: Pinus strobus) in New England. Most forest carbon studies focus on large geographical areas, and utilize “net” carbon data gathered from LIDAR (Light Detection And Ranging) and satellite technology, as well as statistical modeling based on the US Forest Service methods. Upon examining these options we note that carbon estimates from different tools and models can lead to disparate results at the level of individual trees—and these errors can be extrapolated to stands (Leverett et al., 2020). Therefore, we capitalized on the extensive tree-measuring protocols and experience of the Native Tree Society (NTS) to conduct highly accurate direct field measurements and measure volume precisely in younger vs. older trees growing in stands (Native Tree Society, 2021). We used direct measurements to evaluate volume-biomass models from multiple sources and developed a hybrid—termed FIA-COLE—to capitalize on the strengths of each model. We calculated the live above-ground carbon (in metric tons) in individual eastern white pines and individuals of other species in pine stands using conservative assumptions and direct measurements in pines up to 190 years old.
This paper centers primarily on (1) individual eastern white pines (Pinaceae: Pinus strobus), (2) a representative older pine stand in Western Massachusetts, named the Trees of Peace (TOP: located in Mohawk Trail State Forest, Charlemont, MA), and (3) a nearby younger pine stand (∼230 m center to center from the TOP). Both stands regenerated naturally from pasture and they share abiotic conditions such as a similar elevation, soil type (Hinkley loamy), temperature and precipitation. The younger stand is slightly downslope, and neither shows evidence of major recent disturbance. In 1989 the TOP lost 6 trees in a storm. Currently the TOP has 76 pines covering 0.6–0.7 ha.
While not discussed in detail herein, we have also collected and analyzed data from NTS measurements in 38 other sites with white pines in the Eastern United States. Since 1990, NTS has taken thousands of on-site direct measurements of individual trees in dozens of stands of eastern white pines (see examples Supplement 1). Measurements are published on the society’s website (Native Tree Society, 2021) and comprehensive measurement protocols were adopted from those developed by NTS (Leverett et al., 2020) and incorporated into the American Forests Tree Measuring Guidelines Handbook (Leverett and Bertolette, 2014). A brief description of the measurement methods and models is provided in “Height and Diameter Direct Measurement Methodology,” Supplement 2 and in Leverett et al. (2020). Here, in all cases, the best mathematical processes were applied, e.g., the sine instead of the tangent height method and the best statistical models.
In the pine stands, a point-centered plot was established with a radius of 35.89 m, covering 0.403 hectares (subsequently referred to as 0.4 ha), with the goal of evaluating a standard acre (radius: 117.75 ft), and thus relevant to forestry conventions in the U.S. Within the TOP, 44 mature white pine stems were tallied along with 20 hardwoods and eastern hemlocks greater than 10 cm in diameter at breast height (DBH, 4′ 5″ or 1.37 m from the ground). The measured acre had 50 pines in July 1989 when six trees were lost in a wind event. The pines are ∼160 years old; the hardwoods and hemlocks are estimated to be between 80 and 100 years old.
We quantified the volume of the trunk and limbs of each tree from heights and diameters measured with laser-based hypsometers, monoculars with range-finding reticles, traditional diameter tapes, and calipers (described in detail in Leverett et al., 2020). Each instrument was calibrated and independently tested for accuracy over a wide range of distances and conditions (see Supplement 2 for an example). Absolute accuracies of the two main infrared lasers were verified as ±2.5 cm for distance, surpassing the manufacturer’s stated accuracy of ±4.0 cm. The tilt sensors were accurate to ±0.1°, meeting the manufacturer’s stated accuracy. The combination of these distance and angle error ranges, along with the most accurate trigonometric methods noted above (sine vs. tangent method), gave us height accuracies to within 10–15 cm on the most distant targets being measured and approximately half that on the closest targets. We distinguished the rated and/or tested accuracy of a particular sensor of an instrument (such as an infrared laser or tilt sensor) from the results of a measurement that utilized multiple sensors.
Tree heights were measured directly for each pine with a visible top, using the sine method (Supplement 2) whenever possible rather than the traditional tangent method. Our preference for the sine method is supported by NTS, the US Forest Service (Bragg et al., 2011) and American Forests (Leverett and Bertolette, 2014). The more traditional tangent method often over/under-estimates heights by treating the sprig being measured (interpreted as the top), as if it were located vertically over the end of the baseline. The heights of 38 white pines in the TOP with visible tops were measured directly using the sine method.
To compute trunk volume directly from the base to the absolute top of a tree, diameters at base and breast height were measured with conventional calibrated tapes according to the procedures established and published by NTS. Diameters aloft were measured with the combination of laser range-finders and high performance monoculars with range-finding reticles. A miniature surveying device, the LTI Trupoint 300, was also used. Its Class II, phase-based laser is rated at an accuracy of ±1.0 mm to clear targets and its tilt sensor is accurate to ±0.1 degrees. In the TOP, we computed the volume of each pine’s trunk and limbs using diameter at breast height, full tree height, trunk form, and limb factors. (See Supplement 3 for a discussion on the development of the form factor and its importance in measuring volume, with comparisons to other methods of measurement).
Detailed measurements of 39 sample trees established an average form factor (see NTS measurements in Supplement 3, Table S3.2). The volume of each sample tree was determined by dividing the trunk into adjacent sections, with the length of each section guided by observed changes in trunk taper and/or visibility. Each section was modeled as the frustum of a regular geometric solid (neiloid, cone, paraboloid; see Supplement 3 and Leverett et al., 2020, for formulas). The form factor for each pine was computed by adding its section volumes to obtain total trunk volume and then dividing the result by the product of the pine’s height and breast-high cross-sectional area. This produced an average factor that would fit the pines growing in a stand. We applied the average form factor to all pines included in the TOP as one determination of trunk volume.
For comparison to our direct volume measurements, we applied a hybrid volume-biomass model to compute trunk volumes for pines in the TOP. This hybrid allowed us to make use of the extensive analysis of the US Forest Service Forest Inventory and Analysis (FIA) program and database (which determines volume and biomass through the use of allometric equations; United States Forest Service, 2020) as well as the Carbon On-Line Estimator (COLE; National Council for Air Stream Improvement, 2020). This hybrid was termed FIA-COLE. See Supplement 4 for a full explanation of the variables and equations for defining trunk volume. We finalized volumes for the pines in the TOP by averaging our direct measurements with those of FIA-COLE.
For the total volume of the above-ground portion of a pine, we derived a factor for limbs, branches, and twigs as a proportion of the trunk volume using the FIA-COLE model (Supplement 5). That model includes all the branching in what is defined as the “top” in a biomass calculation and the limb factor for large trees is typically an additional 15–16%. We ran the model for each of the individuals in the TOP and calculated the volume. This was converted to biomass (density) and then to carbon mass using a conservative carbon mass fractional factor of 48%.
In addition to the TOP, and older exemplary pines, we quantified above-ground carbon in younger trees and a representative stand. To determine an “average” pine at 50 years we defined two populations: (1) trees at 50 years that are still alive today, and (2) trees that were alive at 50 years but are missing today. This allowed us to compute an average trunk size for the missing trees and the associated carbon. We also measured white pines from young to older ages to estimate growth rates and volumes. The number of pines alive at 50 years but not alive today was determined from stand density data coming from both field counts and FIA (United States Forest Service, 2020).
We extensively studied an ∼80-year-old stand of pines adjacent to the TOP (Supplement 6) growing on a terrace located just downslope from the TOP in an area fairly well protected from wind and with similar abiotic conditions and adequate soil depth. This age is more representative of the average stand of eastern white pine in New England (60–80 years; United States Forest Service, 2019). We also considered the range of pines of known ages from stands within the vicinity and elsewhere. Where we could, we examined ring growth and height patterns for individual pines during their early years on a variety of sites in different geographical locations. In some cases, we examined stumps and measured the average ring width. In other cases, we measured trees and counted limb whorls to get age estimates.
We measured the tallest pine in the TOP over a long time-span (referred to as Pine #58, its research tag number). Pine #58 has been measured carefully and regularly over a period of 28 years. In 1992 the tree was 47.24 m tall and 2.93 m in circumference. Since then, it has been climbed 4 times, tape-drop-measured, and volume-determined. Pine #58 continues to grow and has enabled us to quantify the changes in carbon accumulation in a dominant tree over decades. See Supplement 7 for a detailed measurement history of Pine #58.
Live tree above-ground volumes were converted to mass using standard wood density tables (United States Department of Agriculture, 2009). The air-dried density for white pine is 385.3 kg/m3 (0.3853 metric tons/m3). As noted above, we calculated the amount of carbon in each pine conservatively as 48% of total air-dried weight, whereby a cubic meter of white pine trunk or limbs holds 0.18494 metric tons of carbon (at least 50% is used more commonly; the percentage of carbon content in different species ranges from ∼47% to 52+% and there is evidence that pine is at the upper range (Nicodemus and Williams, 2004). Note that the carbon in a cubic meter of wood varies depending on the species and is usually greater in hardwoods (United States Department of Agriculture, 2009).
Our measurements indicate that individual eastern white pines can accumulate significant above-ground volume/carbon up to at least 190 years, that this volume/carbon accumulation in an individual tree can accelerate beyond 100 years, and that a stand of pines can double its above-ground live carbon between ∼80 and 160 years.
As Pine #58 is the tallest and the largest tree (volume) in the Trees of Peace (TOP), its performance over time was analyzed in great detail. It started growing as part of a more tightly packed stand, but presently has ample space. Its circumference at breast height is 3.30 m, its height is 53.71 m, and its crown spread is approximately 15.5 m. Over a period of 26 years, beginning in 1992, Pine #58 has grown in circumference at an average rate of 1.39 cm per year and grown in height 23.71 cm per year. For a chronosequence, we assumed that Pine #58 grew a lot when it was young—an average of up to 61 cm per year in its first 50 years. Its trunk and limb volume was 23.33 m3 at the end of the 2018 growing season (Supplement 7).
Figure 1 shows the increase in height, circumference and volume of Pine #58 within each 50-year interval up to 150 years and includes a photo of the tree. Its estimated age is ∼160 years, and we used a chronosequence to determine previous epochs. For dominant pines in stands on good sites, ring widths for the first 50 years average ∼0.6 cm and thus a 1.88 m circumference at 50 years. (Note that we measured one exceptional pine at 2.13 m in circumference.) Heights of stands at age 50 depend largely on site characteristics and expressed as site index (the average height of a stand at 50 years). The average index for white pine in Massachusetts is approximately 20 m (William Van Doren, Massachusetts Department of Conservation and Recreation, pers. comm.). For Pine #58 we calculated a much higher index to assume rapid early growth in the first 50 years. Based on these principles, the change in circumference and growth in height were greatest in the first 50 years, and decreased in the next two 50-year periods, confirming young pines “grow more rapidly” in terms of annual height and radial increases. However, volume growth, and thus carbon accumulation, continued to increase in the epochs studied here. This is primarily because volume increases linearly with height but increases as the square of the diameter (see Figure 1 and Supplement 8).
Figure 1. Changes in circumference, height and volume of a stand-grown individual eastern white pine (Pine #58) in three 50-y intervals. Upper panels (A) Change in circumference during 0–50, 50–100, and 100–150 years. (B) Change in height between 0–50, 50–100, and 100–150 years. (C) Change in above-ground tree volume (trunk plus limbs) between 0–50, 50–100, and 100–150 years. Lower panels (D) Cumulative circumference at 50, 100, and 150 years compared to cumulative above-ground volume. (E) Cumulative height at 50, 100, and 150 years compared to cumulative above-ground volume. On each lower panel initial slopes were matched to reflect the rapid change in circumference and height during the first 50-years interval. Note that volume is a proxy for above-ground carbon. Values for circumference, height and volume of Pine #58 were determined by a combination of direct measurement and chronosequence and described in the text and in Supplement. (F) Pine #58 (center) being readied for climbing and measuring.
As noted, we assumed Pine #58 had optimal rapid growth in the first 50 years. Even so, our analysis supports the conclusion that the pine accumulated the majority of its current carbon after age 50 and at an increased rate during subsequent epochs. Pine #58 now stores 4.33 tC above ground and continues to grow. For comparison, the carbon stored in the trunk of the highest volume 50-year-old pine that we encountered (2.13 m circumference, 34.75 m height, and 0.4346 form factor) is 1.16 tC. Therefore, even in the best-case scenario Pine #58 would have acquired only a quarter of its current carbon by age 50. Note that the same crown area occupied by multiple younger trees cannot achieve the carbon in this larger tree (Leverett, unpublished observations).
Up to a point, the carbon advantage gained by the older trees accelerates with their increasing age and size, a finding that has been affirmed globally (Stephenson et al., 2014). Figure 2 documents the average volume in individual pines in the stands at ∼80 and 160 years as well as several additional large pines. MSF Pine #1, the largest pine in Monroe State Forest, western Massachusetts, has a trunk volume of 35.9 m3 at approximately 190 years (6.62 tC; Figure 2). Assuming its early years accumulated 1.16 tC at 50 years, which is the fastest growing 50-year old pine we measured in all sampled locations, the large pine added 5.46 tC between 50 and 190 years, or 1.95 tC per 50-year cycle after year 50. This is at least 1.68 times the rate of growth for the first 50 years. This compares to a 1.6 ratio for Pine #58. In both cases ∼75% of the carbon they accumulated occurred after their first 50 years even when assuming the most optimal growth observed during the first 50 years.
Figure 2. Metric tons of above-ground carbon (tC) in an “average” eastern white pine in a measured research acre (green locants) and in five individual trees measured directly on site at three separate locations in Massachusetts. Average tC and standard deviation is based on pines in a stand at ∼80 years (0.66 ± 0.38 tC) and ∼160 years (1.93 ± 0.73 tC) as described in the text. Direct measurement of tC is shown for individual trees in western Massachusetts at these ages and locations (the centers of the X symbols indicate the data points): ∼190 years: MSF #1 and #2, Monroe State Forest; ∼160 years: Pine #58, Mohawk Trail State Forest; more details of Pine #58 shown in Figure 1; ∼150 years: Totem, Northampton, MA; ∼120 years: BB #2, Broad Brook, Florence, MA.
Detailed measurements were taken in comparable pine stands at ∼80 and 160 years (TOP). As noted, the average tree in each stand is shown in Figure 2, and the distribution of tree sizes in the TOP is shown in Figure 3A. The largest pine in the TOP holds 4.33 tC and the smallest holds 0.53, an eightfold difference. A comparison of the stand density and above ground carbon at ∼80 vs. ∼160 yr are shown in Figure 3B.
Figure 3. Carbon distribution, stand density and cumulative carbon in predominantly eastern white pine stands at ∼80 and 160 years. These two stands were regrown from land previously used as pasture (i.e., not recovering from a harvest at time zero). (A) Distribution of above-ground carbon (tC) among 76 eastern white pines of different sizes in the full TOP stand at ∼160 years old. The majority contained 1–3 tC. (B) Stand density and above-ground carbon measured directly on site in a research acre of eastern white pine at ∼80 and 160 years. Stand density (# of stems) declined while above-ground carbon increased. The older stand includes some non-pine species that added to the number of stems and total carbon (open locants).
Complete data for 76 individual pines in the TOP (the 0.4 ha primary plot plus additional trees in the stand) is provided in Supplement 9. Similar data were collected from 0.4 ha of the ∼80-year old stand (Supplement 6). This age is more representative of the average stand of eastern white pine in New England. Average values for both stands are summarized in Table 1. As shown in Figure 2, we found an average of 0.66 tC per tree compared to 1.93 tC per tree in the TOP, a near tripling of carbon in the average individual pine in the older stand. We found a robust size distribution among the pines in the older stand (Figure 3A), as well as a lower stand density (fewer stems), and a higher level of carbon in the TOP (Figure 3B). Pines predominated both plots, and non-pine species added ∼10% to the total above ground carbon in the TOP (Figure 3B).
Table 1. Summary of key measurements within a 160-year pine stand (TOP) and a comparable ∼80 year old stand (2018–2019 values).
We emphasize that all of our calculations are based on a conservative value for the carbon mass fractional factor in the pines (48%) and only include above-ground live tree-based carbon—they do not include more labile sources of additional carbon in needles, leaves and understory plants, or the accumulation of carbon in downed woody debris in older stands. Our measurements also do not include the large store of underground carbon (the root system is typically estimated as an additional 15–20% of the above-ground tree volume, and total soil organic carbon can be an additional 50% or more (Birdsey and Heath, 1995). Therefore, the total carbon is considerably higher. Nevertheless, the live trees in the older stand hold twice the carbon of the younger stand: the above-ground tree-based carbon measured directly in the primary acre in the 80 year old stand is 46.9 tC and the 160-year-old stand is 94.4 tC, translating to 117.2 and 236.0 tC per hectare, respectively. Approximately 10% of the tree-based carbon in the older stand is non-pine whereas non-pine live tree carbon in the younger stand is negligible (Table 1).
We found that above-ground carbon stored in individual eastern white pines (Pinaceae: Pinus strobus) and stands can continue to increase well beyond 150 years. A chronosequence coupled with decades of direct measurements of a dominant stand-grown individual pine in Massachusetts demonstrate that height and circumference increase rapidly during the first 50-year epoch with smaller increases in 50-year epochs thereafter. In contrast, volume (and therefore carbon) shows the smallest increment in the first 50 years and the biggest in the 50-yr epoch between 100 and 150 years. This superior carbon sequestration in older trees is consistent with recent reports of recent rapid sequestration of older oak trees in Massachusetts (Finzi et al., 2020) and the outsized forest accumulation in large trees (Stephenson et al., 2014; Mildrexler et al., 2020). Here, the largest pine measured in Massachusetts (by volume) achieved 6.62 tC at 190 years old, and we found very large pines at ages ranging up to 350 years at dozens of sites in the Eastern United States.
Using direct measurement of above-ground carbon in different-aged pine stands, we found that live tree carbon can continue to increase in a pine stand up to at least 160 years. We found twice as much above-ground live tree carbon in a measured research acre within the older vs. the younger stand. The live pines in the older stand also exhibited marked size diversity and the stand had a higher tree species diversity.
The representative stands in this analysis approximate the average pine forest age in New England (∼80 years old) and a comparable stand approximately twice that age. To determine the biomass and above ground carbon in living trees as a function of tree size and age, we have used a combination of direct measurements and a hybrid FIA-COLE (Forest Inventory and Analysis—Carbon On-Line Estimator) volume and biomass model to quantify individual trees and stands of eastern white pine. We found that individual trees continue accumulating carbon well past 150 years, and ∼75% of the carbon in pines up to 190 years is gained after the first 50 years. Despite a lower stand density (fewer stems), total above-ground carbon is greatest in older stands and continues to increase past 150 years. The carbon per hectare quantified in these stands aligns with previous averages for the region and previous regional estimates that New England forests can accumulate between 2.3 and 4.2 times as much carbon as they now contain on productive sites (Keeton et al., 2011). The total carbon stored is much greater when below-ground carbon in roots, coarse woody debris, standing dead trees, smaller plants and soils are included (Birdsey and Heath, 1995; Nunery and Keeton, 2010; Tomasso and Leighton, 2014).
Forest managers stress the high accumulation rates of younger forests as important in absorbing atmospheric CO2. This is an important consideration for production forests as well as to help optimize between growing a wood resource and accumulating carbon. Younger individual trees do not accumulate absolute amounts of carbon more rapidly than larger more mature trees, and we did not find evidence for a significant benefit for a young stand compared to an older stand. We note this is a limited sample, and we did not estimate rates of accumulation below 80 years (Table 1).
Multi-use forests provide a source of wood products and can support recreation but active management practices limit forest carbon accumulation long-term. At a range of scales, chronic intervention eliminates the ability for that forest to host the full biodiversity of some of our rarest species of plants, animals, insects, fungi, lichens, reptiles and amphibians found in older and continuously forested areas (McMullin and Wiersma, 2019; Moose et al., 2019) as well as climate-sensitive birds that may benefit from mature or old-growth forests (Betts et al., 2017). These older unmanaged forests also have fewer invasive species (Riitters et al., 2018).
The pine stands studied here grew from abandoned sheep pasture, and therefore were unlikely to have been severely disturbed prior to natural regeneration. Site history influences growth and net carbon accumulation, especially in the early years, since disturbed soil can continue to lose carbon for more than a decade (Birdsey and Heath, 1995; Hamburg et al., 2019). We recognize that at some point the above-ground carbon in living trees will no longer increase as the live trees in the stand eventually will reach a steady state of death and renewal. Pines easily reach 200 years and some live 400 years; today the TOP is less than halfway to that age and the younger stand is only ∼25% of that lifespan. Previous work shows that pine stands continue to add above ground carbon beyond 200 years (Seymour, 2011, 2016), and even when above-ground live carbon reaches asymptote, total forest carbon continues to increase, even in some primary (“old-growth”) forests (Mackey et al., 2015): after tree death or forest disturbance there is a new growth as well as transfer of live carbon to dead wood and woody debris, the litter layer, and into the soil. For example, 70 years after an old-growth (virgin) eastern hemlock (Tsuga canadensis) and eastern white pine stand blew down (the 1938 Hurricane in New England) that forest stored as much carbon as forests that were 250 years old (D’Amato et al., 2017).
There is no evidence of recent disturbance in either research plot herein. A major storm in 1989 blew over six large pines in the older research acre, reducing stand density by >10% and thereby reducing above-ground live-tree carbon. Downed wood due to death and disturbance contributes to total forest carbon and biodiversity. The older pine stand shows an increased prevalence and growth of trees of other species (including more carbon-dense hardwoods), and for multiple reasons it is unlikely it has reached maximal above-ground live carbon or total carbon. Rather, this forest appears to be transitioning into a phase where the structural diversity, species diversity and total carbon load will continue to rise. A goal for future research is a better understanding of tree and stand-level carbon accumulation and dynamics as well as many other ecological features in different forest types and in stands well beyond 150 years—a time when old-growth characteristics are starting to redevelop in eastern forests.
Public forests in New England are typically older than private forests (but still predominantly less than 100 years old), and provide the greatest possibility for future carbon-dense and biodiverse intact forests across the landscape. Native tree species can live for several hundred years (and in the case of eastern hemlock (Tsuga canadensis) and black gum (Nyssa sylvatica), up to and exceeding 500 years) (Whitney, 1996; Sperduto et al., 2000). Despite the shortage of old and old-growth forests (and their proven resilience to disturbance (D’Amato et al., 2017), and the increased prevalence of natural disturbances (e.g., insect outbreaks, windstorms) creating forest diversity and forest openings (Oswalt et al., 2019), a major focus across public land has been to make forests younger. These programs assert that these habitats prevent a suite of species from declining, that they accumulate carbon more rapidly, and that they are more resistant to disturbance than their older counterparts (Anwar, 2001). This approach downplays the rate of the natural development of niches for multiple species (Zlonis and Niemi, 2014) and the accumulation of biodiversity in temperate forests during natural forest succession (Hilmers et al., 2018). It also overlooks cumulative forest carbon (Moomaw et al., 2019) as well as the superior resilience of older forests to the stresses of climate change (Thom et al., 2019). Comparing details of age and location (tropical, temperate, boreal, etc.) are important, as is evaluating the term “young”—in some cases it is considered up to 140 years (Pugh et al., 2019).
Our findings are consistent with Stephenson et al. (2014) who found that absolute growth increases with tree size for most of 403 tropical and temperate tree species, and a study of 48 forest plots found that in older forests, regardless of geographical location, half of all above-ground biomass (and hence carbon), is stored in the largest 1% of trees as measured by diameter at breast height (Lutz et al., 2018). An increase in carbon density per hectare was found as the age of the stand increased in the Northeast U.S. (Keeton et al., 2011), and a recent study in China found that forests with older trees and greater species richness had twice the levels of carbon storage than did less diverse forests with younger trees (Liu et al., 2018). Earlier work demonstrated that intact old growth forests in the Pacific Northwest contained more than twice the amount of carbon as did those that were harvested on a fixed rotation basis (Harmon et al., 1990).
Globally, forests are capable of accumulating twice as much atmospheric carbon, and the current deficit is due to a combination of conversion and management (Erb et al., 2018). Continuing current management in the Northeast will result in a large difference between the potential for land-based carbon and the current trajectory (Duveneck and Thompson, 2019). Meanwhile, natural regeneration and reforestation is a superior climate solution compared to managed reforestation and tree planting (Cook-Patton et al., 2020). Proforestation—growing existing natural forests—complements and extends natural regeneration as an ongoing climate solution by leveraging the accumulation potential in forests that are already established (Moomaw et al., 2019). These strategies are outlined in the recent report on “ten golden rules” for restoring forests wherein the first rule is protect existing forest (Di Sacco et al., 2021). Proforestation recognizes implicitly that older forests and large trees are critical to a global strategy for carbon accumulation and biodiversity protection (Lindenmayer and Laurance, 2016). Rapidly moving large stocks of atmospheric carbon as CO2 into forests and reducing emissions are both essential to limiting the increase in global temperatures, and protecting intact and connected habitat is essential in preventing extinction. These time-sensitive dual goals and the importance of traditional indigenous land use are explicitly recognized internationally in the Global Deal for Nature, the Global Safety Net, and the recent “Campaign for Nature” or “30 × 30”—i.e., protecting 30% of the planet’s land and water by 2030 (Campaign for Nature, 2021), and in the ambitious coalition goal of “Nature Needs Half” (Nature Needs Half, 2021).
An important additional implication of our study is that the estimated potential additional carbon dioxide (CO2) removal (CDR) achieved by future growth of secondary forests as reported by Houghton and Nassikas (2018) is likely an underestimate because it does not account for high ongoing accumulation rates as trees age in regions with relatively young (compared to tree lifespan) forests like those of the Northeast United States. The global study of natural forest carbon accumulation by Cook-Patton et al. (2020) and the synthesis of quantified carbon and biodiversity by Moomaw et al. (2019) provide evidence for the power of natural forest processes throughout their growth and development. These reports and the current site-specific findings support the high regional contribution of carbon accumulation in the coming decades by Northeastern temperate forests and their designation as a Tier 1 climate stabilization region (Dinerstein et al., 2020).
Whereas the IPCC clearly identified forests as essential for accumulating additional carbon for climate stability, it focused on production forests that are currently recovering from being harvested or on unforested areas where forests could be planted (afforestation). Bastin et al. (2019) proposes an afforestation project on 0.9 billion ha but acknowledges the relatively long time before large amounts of carbon would be stored. Global tree planting efforts are under way, but are presented too simplistically (Holl and Brancalion, 2020); for example, there is little data on how to plant an ecosystem, and tree planting efforts can suffer from numerous challenges, including high mortality (Cao et al., 2011). In contrast, growing existing forests is an established near-term strategy (Moomaw et al., 2019). Overall, afforestation and reforestation are valuable, but neither can keep as much carbon out of the atmosphere as proforestation in the next 50 years—the timeline when it is needed most to avoid irreversible consequences of a changed climate.
Although this study focused exclusively on above-ground live tree carbon accumulation, we emphasize that additional carbon exists and accumulates above and below ground. Other ecosystem services of proforestation also accrue, and the essential goal of protecting a “Global Safety Net” of nature extends explicitly beyond greenhouse gas emissions and mitigating the climate crisis (Dinerstein et al., 2020). Nevertheless, an accurate carbon-centric model of “business as usual” vs. proforestation must include comprehensive real-world carbon fluxes. Removing carbon from the forest releases carbon into the atmosphere, and in some cases a portion of the carbon is stored in wood and/or wood is substituting for other materials. Recent work shows that near-term carbon benefits associated with wood products and substitution have been overestimated based on outdated assumptions or neglecting or underestimating future accumulation (Harmon, 2019; Leturcq, 2020). Efforts should be made on consumption and conservation to ensure we protect primary forests and additional secondary forests where possible: carbon storage in forests is low-risk, high-capacity and practical—therefore preferable to experimental bioenergy with carbon capture and storage (BECCS) suggested by the IPCC report (Anderson and Peters, 2016; Intergovernmental Panel on Climate Change, 2018). Finally, letting existing secondary forests grow creates a network of nature that can provide equity, access to natural heritage, scientific discovery, and cumulative health benefits for people. Protecting and growing a network of suitable existing forests as a carbon sink in New England is cost-effective (Tomasso and Leighton, 2014) and does not compete directly with agriculture and other demands for land use.
The direct measurements at the tree and stand level in this paper are consistent with parameterized and other studies at larger scale in verifying that larger trees (Stephenson et al., 2014; Lutz et al., 2018) and stands of larger trees accumulate the most carbon over time compared to smaller trees (Mildrexler et al., 2020). They support the proforestation strategy of growing existing forests to achieve their natural capacity to accumulate carbon and achieve their ecological potential (Moomaw et al., 2019) to redress the balance of carbon lost to the atmosphere from global forests due to human activity (Hudiburg et al., 2019). The important implication of these findings is that the trees and the forests that we need most for carbon storage and CDR to help limit near-term climate change are the ones that are already established.
Currently, plantations and forests managed for forest products account for 71% of all forest area globally (Intergovernmental Panel on Climate Change, 2019), more than sufficient for resource production. Strategic decisions can enable some of these forests to be dedicated to climate protection and research, and the remaining 29% should be protected wherever possible. This would be a major step toward the goal of 30 × 30—with additional climate stabilization areas needed beyond that. Together 30 × 30 plus climate stabilization will move us toward long-term protection of “half-earth” (Wilson, 2016). High levels of carbon accumulation and biodiversity protection are integral to resiliency in a changing climate—including the resiliency achieved by protecting species networks and interactions, genetic diversity and the potential for specific adaptive epigenetic changes (Hanlon et al., 2019). These complexities are poorly understood—science and technology is evolving, and new techniques can discover new species (Schulz et al., 2018)—and any areas, even on public land, lack a detailed ecological inventory due to resource constraints or a focus on other priorities. Meanwhile, intensive biodiversity inventories have yielded many hundreds of new species—often small species such microbes, lichen, fungi, algae and insects; i.e., Smokies Species Tally (Discover Life in America, 2021). Much more research is needed, and essential ecological processes develop and diversify at timescales far beyond a human lifetime.
In sum, the current findings ground-truth the capacity for a representative New England eastern white pine stand to at least double its above-ground live tree carbon in the coming decades, confirming previous chronosequencing of pine stands in the region (Seymour, 2011). We did not attempt to quantify or estimate the flux in other carbon compartments above or below ground. With a small fraction of New England (∼3% overall, ∼1% in Southern New England) prioritized for proforestation and natural processes, protection of a suitable network of land from unneeded intervention is urgent, and public land is the most logical place to start: funding to ensure evidence-based intervention and additional data collection will generate policies that protect the long-term public trust. At the same time, systems to support local wood use and reuse are equally needed to ensure the highest and best use of this resource, protect local expertise and jobs, and reduce emissions associated with the forest industry; in some states it is the largest source of emissions (Law et al., 2018). Comprehensive education, information and compensation programs should be established to provide private landowners a range of options based on numerous ecosystem services, including maximal carbon and biodiversity accumulation, with the goal of optimizing natural solutions that address the Climate Emergency immediately (Ripple et al., 2020). Failing to protect natural systems erodes the wealth and well-being that is essential to meet this unprecedented challenge and avoid “a ghastly future” (Bradshaw et al., 2021).
The original contributions presented in the study are included in the article/Supplementary Material, further inquiries can be directed to the corresponding author/s.
RL chose site locations and individual trees, established measurement methods and protocols, did the on-site tree measuring, and performed the subsequent analysis. SM analyzed and organized the content and supplements, and participated in drafting and finalizing the text. WM framed the analysis in the context of other studies and the larger context of climate change, assisted with data analysis and presentation, and drafting and editing the text. All authors contributed to the article and approved the submitted version.
This work was supported by Trinity College (SM), the Charles Bullard Fellowship in Forest Research (SM), the Faculty Research Grant from the NASA Connecticut Space Grant Consortium (RL, SM) and the Rockefeller Brothers Fund (WM).
The authors declare that the research was conducted in the absence of any commercial or financial relationships that could be construed as a potential conflict of interest.
We acknowledge the critical technical assistance of Jared D. Lockwood in measuring pines in the TOP, the younger pine stand, and elsewhere as needed. We thank Ray Asselin for photographing pines for further analysis, and for ring and whorl counts to establish ages. We thank Monica Jakuc Leverett for invaluable assistance in editing and revising the original text thank, Dr. David Ruskin for tireless efforts throughout the process, and thank Dr. Edward Faison for constructive suggestions. We also thank the reviewers for constructive comments.
The Supplementary Material for this article can be found online at: https://www.frontiersin.org/articles/10.3389/ffgc.2021.620450/full#supplementary-material
Anderson, K., and Peters, G. (2016). The trouble with negative emissions. Science 354, 182–183. doi: 10.1126/science.aah4567
Anwar, A. (2001). Does the Age of a Tree Affect Carbon Storage? [Online]. GISS Institute on Climate and Planets, National Aeronautics and Space Administration. Available online at: https://icp.giss.nasa.gov/research/ppa/2001/anwar/ (accessed 2021).
Bastin, J. F., Finegold, Y., Garcia, C., Mollicone, D., Rezende, M., Routh, D., et al. (2019). The global tree restoration potential. Science 365, 76–79. doi: 10.1126/science.aax0848
Betts, M. G., Phalan, B., Frey, S. J. K., Rousseau, J. S., and Yang, Z. (2017). Old-growth forests buffer climate-sensitive bird populations from warming. Divers. Distrib. 24, 439–447. doi: 10.1111/ddi.12688
Birdsey, R. A., and Heath, L. S. (1995). “Carbon changes in U.S. forests,” in Productivity of America’s Forests and Climate Change. General Technical Report RM-GTR-271, ed. L. A. Joyce (Fort Collins, CO: U.S.D.A. Forest Service, Rocky Mountain Forest and Experiment Station), 56–70.
Bradshaw, C. J. A., Ehrlich, P. R., Beattie, A., Ceballos, G., Crist, E., Diamond, J., et al. (2021). Underestimating the challenges of avoiding a ghastly future. Front. Conserv. Sci. 1:615419. doi: 10.3389/fcosc.2020.615419
Bragg, D. C., Frelich, L. E., Leverett, R. T., Blozan, W., and Luthringer, D. J. (2011). The sine method: An alternative height measurement technique, Research Note SRS-22. Asheville, NC: F.S. U.S. Department of Agriculture, Southern Research Station.
Campaign for Nature (2021). Availableonline at: https://www.campaignfornature.org/ (accessed 2021).
Cao, S., Chen, L., Shankman, D., Wang, C., Wang, X., and Zhang, H. (2011). Excessive reliance on afforestation in China’s arid and semi-arid regions: lessons in ecological restoration. Earth Sci. Rev. 104, 240–245. doi: 10.1016/j.earscirev.2010.11.002
Coetzee, B. W., Gaston, K. J., and Chown, S. L. (2014). Local scale comparisons of biodiversity as a test for global protected area ecological performance: a meta-analysis. PLoS One 9:e105824. doi: 10.1371/journal.pone.0105824
Cook-Patton, S. C., Leavitt, S. M., Gibbs, D., Harris, N. L., Lister, K., Anderson-Teixeira, K. J., et al. (2020). Mapping carbon accumulation potential from global natural forest regrowth. Nature 585, 545–550. doi: 10.1038/s41586-020-2686-x
D’Amato, A. W., Orwig, D. A., Foster, D. R., Barker Plotkin, A., Schoonmaker, P. K., and Wagner, M. R. (2017). Long-term structural and biomass dynamics of virgin Tsuga canadensis-Pinus strobus forests after hurricane disturbance. Ecology 98, 721–733. doi: 10.1002/ecy.1684
Davis, M. B. (1996). Eastern Old-Growth Forests: Prospects For Rediscovery And Recovery. Washington DC: Island Press.
Di Marco, M., Ferrier, S., Harwood, T. D., Hoskins, A. J., and Watson, J. E. M. (2019). Wilderness areas halve the extinction risk of terrestrial biodiversity. Nature 573, 582–585. doi: 10.1038/s41586-019-1567-7
Dinerstein, E., Joshi, A. R., Vynne, C., Lee, A. T. L., Pharand-Deschenes, F., Franca, M., et al. (2020). A “Global Safety Net” to reverse biodiversity loss and stabilize Earth’s climate. Sci. Adv. 6:eabb2824. doi: 10.1126/sciadv.abb2824
Dinerstein, E., Vynne, C., Sala, E., Joshi, A. R., Fernando, S., Lovejoy, T. E., et al. (2019). A global deal for nature: guiding principles, milestones, and targets. Sci. Adv. 5:eaaw2869. doi: 10.1126/sciadv.aaw2869
Di Sacco, A., Hardwick, K. A., Blakesley, D., Brancalion, P. H. S., Breman, E., Cecilio Rebola, L., et al. (2021). Ten golden rules for reforestation to optimize carbon sequestration, biodiversity recovery and livelihood benefits. Glob. Chang. Biol. 27, 1328–1348. doi: 10.1111/gcb.15498
Discover Life in America (2021). Available online at: https://dlia.org/smokies-species-tally (accessed 2021).
Duveneck, M. J., and Thompson, J. R. (2019). Social and biophysical determinants of future forest conditions in New England: effects of a modern land-use regime. Glob. Environ. Change 55, 115–129. doi: 10.1016/j.gloenvcha.2019.01.009
Erb, K.-H., Kastner, T., Plutzar, C., Bais, A. L. S., Carvalhais, N., Fetzel, T., et al. (2018). Unexpectedly large impact of forest management and grazing on global vegetation biomass. Nature 553, 73–76. doi: 10.1038/nature25138
Fargione, J. E., Bassett, S., Boucher, T., Bridgham, S. D., Conant, R. T., Cook-Patton, S. C., et al. (2018). Natural climate solutions for the United States. Sci. Adv. 4:eaat1869.
Finzi, A. C., Giasson, M.-A., Barker Plotkin, A. A., Aber, J. D., Boose, E. R., Davidson, E. A., et al. (2020). Carbon budget of the harvard forest long-term ecological research site: pattern, process, and response to global change. Ecol. Monogr. 90:e01423.
Friedlingstein, P., O’Sullivan, M., Jones, M. W., Andrew, R. M., Hauck, J., Olsen, A., et al. (2020). Global carbon budget 2020. Earth Syst. Sci. Data 12, 3269–3340.
Griscom, B. W., Adams, J., Ellis, P. W., Houghton, R. A., Lomax, G., Miteva, D. A., et al. (2017). Natural climate solutions. Proc. Natl. Acad. Sci. U.S.A. 114, 11645–11650.
Hamburg, S. P., Vadeboncoeur, M. A., Johnson, C. E., and Sanderman, J. (2019). Losses of mineral soil carbon largely offset biomass accumulation 15 years after whole-tree harvest in a northern hardwood forest. Biogeochemistry 144, 1–14. doi: 10.1007/s10533-019-00568-3
Hanlon, V. C. T., Otto, S. P., and Aitken, S. N. (2019). Somatic mutations substantially increase the per-generation mutation rate in the conifer Picea sitchensis. Evol. Lett. 3, 348–358. doi: 10.1002/evl3.121
Harmon, M. E. (2019). Have product substitution carbon benefits been overestimated? A sensitivity analysis of key assumptions. Environ. Res. Lett. 14:065008. doi: 10.1088/1748-9326/ab1e95
Harmon, M. E., Ferrell, W. K., and Franklin, J. F. (1990). Effects on carbon storage of conversion of old-growth forests to young forests. Science 247, 699–702. doi: 10.1126/science.247.4943.699
Harris, N. L., Hagen, S. C., Saatchi, S. S., Pearson, T. R. H., Woodall, C. W., Domke, G. M., et al. (2016). Attribution of net carbon change by disturbance type across forest lands of the conterminous United States. Carbon Balance Manag. 11:24.
Hilmers, T., Friess, N., Bassler, C., Heurich, M., Brandi, R., Pretzsch, H., et al. (2018). Biodiversity along temperate forest succession. J. Appl. Ecol. 55, 2756–2766. doi: 10.1111/1365-2664.13238
Holl, K. D., and Brancalion, P. H. S. (2020). Tree planting is not a simple solution. Science 368, 580–581. doi: 10.1126/science.aba8232
Houghton, R. A., and Nassikas, A. A. (2018). Negative emissions from stopping deforestation and forest degradation, globally. Glob. Chang. Biol. 24, 350–359. doi: 10.1111/gcb.13876
Hudiburg, T. W., Law, B. E., Moomaw, W. R., Harmon, M. E., and Stenzel, J. E. (2019). Meeting GHG reduction targets requires accounting of all forest emission sectors. Environ. Res. Lett. 14:095005. doi: 10.1088/1748-9326/ab28bb
Intergovernmental Panel on Climate Change (2018). “Summary for Policymakers,” in Global warming of 1.5∘C An IPCC Special Report on the impacts of Global Warming of 1.5∘C Above Pre-industrial Levels and Related Global Greenhouse Gas Emission Pathways, in the Context of Strengthening the Global Response to the Threat of Climate Change, Sustainable Development, and Efforts to Eradicate Poverty, eds V. Masson-Delmotte, P. Zhai, H. O. Pörtner, D. Roberts, J. Skea, P. R. Shukla, et al. (Geneva: World Meteorological Society).
Intergovernmental Panel on Climate Change (2019). “Summary for Policymakers,” in Global Warming of 1.5∘C,” In An IPCC Special Report on the Impacts of Global Warming of 1.5∘C above Pre-industrial Levels And Related Global Greenhouse Gas Emission Pathways, in the Context of Strengthening the Global Response to the Threat of Climate Change, Sustainable Development, and Efforts to Eradicate Poverty, eds V. Masson-Delmotte, P. Zhai, H.-O. Pörtner, D. Roberts, J. Skea, P. R. Shukla, et al. (Geneva: World Meteorological Society).
Intergovernmental Science-Policy on Biodiversity and Ecosystem Services (2019). in Global Assessment Report on Biodiversity and Ecosystem Services, eds E. S. Brondizio, J. Settele, S. Díaz, and H. T. Ngo (Bonn: IPBES).
Karjalainen, E., Sarjala, T., and Raitio, H. (2010). Promoting human health through forests: overview and major challenges. Environ. Health Prev. Med. 15, 1–8. doi: 10.1007/s12199-008-0069-2
Keeton, W. S., Whitman, A. A., Mcgee, G. C., and Goodale, C. L. (2011). Late-successional biomass development in northern hardwood-conifer forests of the Northeastern United States. Forest Sci. 57, 489–505.
Kershner, B., and Leverett, R. T. (2004). The Sierra Club Guide to the Ancient Forests of the Northeast. San Francisco, CA: Sierra Club Books.
Law, B. E., Hudiburg, T. W., Berner, L. T., Kent, J. J., Buotte, P. C., and Harmon, M. E. (2018). Land use strategies to mitigate climate change in carbon dense temperate forests. Proc. Natl. Acad. Sci. U.S.A. 115, 3663–3668. doi: 10.1073/pnas.1720064115
Leturcq, P. (2020). GHG displacement factors of harvested wood products: the myth of substitution. Sci. Rep. 10:20752.
Leverett, R. T., and Bertolette, D. (2014). American Forests Champion Trees Measuring Guidelines Handbook [Online]. Available online at: https://www.americanforests.org/wp-content/uploads/2014/12/AF-Tree-Measuring-Guidelines_LR.pdf (accessed 2021).
Leverett, R. T., Ruskin, D. N., and Masino, S. A. (2020). Direct measurement of trunk volume in forest trees: Focus on white pine and comparison to a statistical method. bioRxiv [Preprint] doi: 10.1101/2020.03.18.995985
Lindenmayer, D. B., and Laurance, W. F. (2016). The unique challenges of conserving large old trees. Trends Ecol. Evol. 31, 416–418. doi: 10.1016/j.tree.2016.03.003
Liu, X., Trogisch, S., He, J.-S., Niklaus, P. A., Bruelheide, H., Tang, Z., et al. (2018). Tree species richness increases ecosystem carbon storage in subtropical forests. Proc. Biol. Sci. 285:20181240. doi: 10.1098/rspb.2018.1240
Lutz, J. A., Furniss, T. J., Johnson, D. J., Davies, S. J., Allen, D., Alonso, A., et al. (2018). Global importance of large-diameter trees. Glob. Ecol. Biogeogr. 27, 849–864.
Mackey, B., Dellasala, D. A., Kormos, C., Lindenmayer, D., Kumpel, N., Zimmerman, B., et al. (2015). Policy options for the world’s primary forests in multilateral environmental agreements. Conserv. Lett. 8, 139–147. doi: 10.1111/conl.12120
McMullin, R. T., and Wiersma, Y. F. (2019). Out with OLD growth, in with ecological continNEWity: new perspectives on forest conservation. Front. Ecol. Environ. 17:176–181. doi: 10.1002/fee.2016
Mildrexler, D. J., Berner, L. T., Law, B. E., Birdsey, R. A., and Moomaw, W. R. (2020). Large trees dominate carbon storage in forests east of the Cascade crest in the U.S. Pacific Northwest. Front. For. Glob. Change 3:594274. doi: 10.3389/ffgc.2020.594274
Mitchard, E. T. A. (2018). The tropical forest carbon cycle and climate change. Nature 559, 527–534. doi: 10.1038/s41586-018-0300-2
Moomaw, W. R., Masino, S. A., and Faison, E. K. (2019). Intact forests in the United States: Proforestation mitigates climate change and serves the greatest good. Front. Forests Global Change 2:27. doi: 10.3389/ffgc.2019.00027
Moose, R. A., Schigel, D., Kirby, L. J., and Shumskaya, M. (2019). Dead wood fungi in North America: an insight into research and conservation potential. Nat. Conserv. 32, 1–17. doi: 10.3897/natureconservation.32.30875
National Council for Air Stream Improvement (2020). United States Department of Agriculture Forest Service. Cole, IL: Carbon on Line Estimator.
Native Tree Society (2021). Available online at: https://www.nativetreesociety.org/, https://www.ents-bbs.org (accessed 2021).
Nature Needs Half (2021). Available online at: https://natureneedshalf.org/ (accessed 2021).
Nicodemus, M. A., and Williams, R. A. (2004). “Quantifying aboveground carbon storage in managed forest ecosystems in Ohio,” in Proceedings of the 14th Central Hardwoods Forest Conference GTR-NE-316, Newtown Square, PA.
Nunery, J. S., and Keeton, W. S. (2010). Forest carbon storage in the northeastern United States: Net effects of harvesting frequency, post-harvest retention, and wood products. Forest Ecol. Manag. 259, 1363–1375. doi: 10.1016/j.foreco.2009.12.029
Oswalt, S. N., Smith, W. B., Miles, P. D., and Pugh, S. A. (2019). “Forest Resources of the United States, 2017: a technical document supporting the Forest Service 2020 update of the RPA Assessment,” in Gen. Tech. Rep. WO-97 ed. F. S. (Washington, DC: United States Department of Agriculture).
Pugh, T. A. M., Lindeskog, M., Smith, B., Poulter, B., Arneth, A., Haverd, V., et al. (2019). Role of forest regrowth in global carbon sink dynamics. Proc. Natl. Acad. Sci. U.S.A. 116, 4382–4387. doi: 10.1073/pnas.1810512116
Riitters, K., Potter, K., Iannone, B., Oswalt, C., Guo, Q., and Fei, S. (2018). Exposure of protected and unprotected forest to plant invasions in the eastern United States. Forests 9:723. doi: 10.3390/f9110723
Ripple, W. J., Wolf, C., Newsome, T. M., Barnard, P., and Moomaw, W. R. (2020). World scientists’ warning of a climate emergency. BioScience 70, 8–12.
Ruddat, J. (2020). Documenting and Protecting New England’s Old-Growth Forests [Online]. Available online at: https://digitalcommons.wpi.edu/iqp-all/5763 (accessed 2021).
Schulz, F., Alteio, L., Goudeau, D., Ryan, E. M., Yu, F. B., Malmstrom, R. R., et al. (2018). Hidden diversity of soil giant viruses. Nat. Commun. 9:4881.
Seymour, R. (2011). Influence of age on Productivity and Carbon Sequestration of Eastern white pine. Northeastern States Research Cooperative. Available online at: https://nsrcforest.org/project/influence-age-productivity-and-carbon-sequestration-eastern-white-pine (accessed 2021).
Seymour, R. (2016). Managing an Aging Resource: Influence of age on Leaf Area Index, Stemwood Growth, Growth Efficiency, and Carbon Sequestration of Eastern White Pine. Northeastern States Research Cooperative. Available online at: https://nsrcforest.org/sites/default/files/uploads/seymour11full.pdf (accessed 2021).
Simmons, C. T., and Matthews, H. D. (2016). Assessing the implications of human land-use change for the transient climate response to cumulative carbon emissions. Environ. Res. Lett. 11:035001. doi: 10.1088/1748-9326/11/3/035001
Sperduto, D. D., Nichols, W. F., Crowley, K. F., and Bechtel, D. A. (2000). Black Gum (Nyssa sylvatica Marsh) in New Hampshire. Concord, NH: New Hampshire Natural Heritage Inventory.
Stephenson, N. L., Das, A. J., Condit, R., Russo, S. E., Baker, P. J., Beckman, N. G., et al. (2014). Rate of tree carbon accumulation increases continuously with tree size. Nature 507, 90–93.
Sterman, J. D., Siegel, L., and Rooney-Varga, J. N. (2018). Reply to comment on ‘Does replacing coal with wood lower CO2 emissions? Dynamic lifecycle analysis of wood bioenergy’. Environ. Res. Lett. 13:128003. doi: 10.1088/1748-9326/aaf354
Thom, D., Golivets, M., Edling, L., Meigs, G. W., Gourevitch, J. D., Sonter, L. J., et al. (2019). The climate sensitivity of carbon, timber, and species richness covaries with forest age in boreal-temperate North America. Glob. Chang. Biol. 25, 2446–2458. doi: 10.1111/gcb.14656
Tomasso, L. P., and Leighton, M. (2014). The impact of land use change for greenhouse gas inventories and state-level climate mediation policy: a GIS methodology applied to Connecticut. J. Environ. Protec. 5:52176.
United States Climate Alliance (2021). Available online at: http://www.usclimatealliance.org/ (accessed 2021).
United States Department of Agriculture (2009). Specific Gravity and Other Properties of Wood and Bark for 156 Tree Species Found in North America [Online]. Available online at: https://www.fs.usda.gov/treesearch/pubs/34185 (accessed 2021).
United States Environmental Protection, and Agency (2018). Sources of Greenhouse Gas Emissions [Online]. Available online at: https://www.epa.gov/ghgemissions/sources-greenhouse-gas-emissions (accessed 2021).
United States Forest Service (2019). EVALIDator Version 1.8.0.01 [Online]. Available online at: https://apps.fs.usda.gov/Evalidator/evalidator.jsp (accessed 2021).
United States Forest Service (2020). Forest Inventory Analysis [Online]. Available online at: https://www.fia.fs.fed.us/ (accessed 2021).
United States Forest Service (2021). Ecosystem Services [Online]. Available online at: https://www.fs.fed.us/ecosystemservices/ (accessed 2021).
Watson, J. E. M., Evans, T., Venter, O., Williams, B., Tulloch, A., Stewart, C., et al. (2018). The exceptional value of intact forest ecosystems. Nat. Ecol. Evol. 2, 599–610.
Whitney, G. G. (1996). From Coastal Wilderness to Fruited Plain: A History of Environmental Change in Temperate North America from 1500 to the Present. Cambridge: Cambridge University Press.
Wilson, E. O. (2016). Half-Earth: Our Planet’s Fight for Life. New York, NY: Liveright Publishing Corp.
World Wildlife Federation (2020). Living Planet Report 2020 [Online]. Available online at: https://livingplanet.panda.org/en-us/ (accessed 2021).
Keywords: proforestation, intact forest, ecological resilience, carbon accumulation, chronoseqeuence, old-growth and second-growth forest, tree volume, ecological integrity
Citation: Leverett RT, Masino SA and Moomaw WR (2021) Older Eastern White Pine Trees and Stands Accumulate Carbon for Many Decades and Maximize Cumulative Carbon. Front. For. Glob. Change 4:620450. doi: 10.3389/ffgc.2021.620450
Received: 22 October 2020; Accepted: 07 April 2021;
Published: 13 May 2021.
Edited by:
Alexandra C. Morel, University of Oxford, United KingdomReviewed by:
Matthew Brolly, University of Brighton, United KingdomCopyright © 2021 Leverett, Masino and Moomaw. This is an open-access article distributed under the terms of the Creative Commons Attribution License (CC BY). The use, distribution or reproduction in other forums is permitted, provided the original author(s) and the copyright owner(s) are credited and that the original publication in this journal is cited, in accordance with accepted academic practice. No use, distribution or reproduction is permitted which does not comply with these terms.
*Correspondence: William R. Moomaw, V2lsbGlhbS5tb29tYXdAdHVmdHMuZWR1
Disclaimer: All claims expressed in this article are solely those of the authors and do not necessarily represent those of their affiliated organizations, or those of the publisher, the editors and the reviewers. Any product that may be evaluated in this article or claim that may be made by its manufacturer is not guaranteed or endorsed by the publisher.
Research integrity at Frontiers
Learn more about the work of our research integrity team to safeguard the quality of each article we publish.