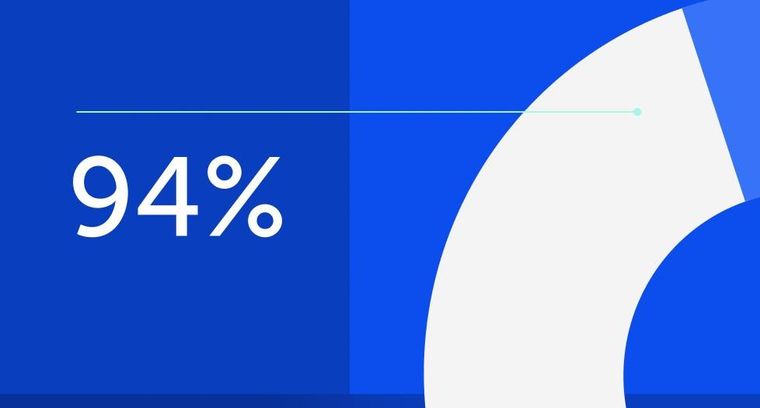
94% of researchers rate our articles as excellent or good
Learn more about the work of our research integrity team to safeguard the quality of each article we publish.
Find out more
ORIGINAL RESEARCH article
Front. For. Glob. Change, 12 January 2021
Sec. Forest Ecophysiology
Volume 3 - 2020 | https://doi.org/10.3389/ffgc.2020.601046
This article is part of the Research TopicAdaptation of Forest Species to DroughtView all 4 articles
Springtime bud-break and shoot development induces substantial carbon (C) costs in trees. Drought stress during shoot development can impede C uptake and translocation. This is therefore a channel through which water shortage can lead to restricted shoot expansion and physiological capacity, which in turn may impact annual canopy C uptake. We studied effects of drought and re-hydration on early season shoot development, C uptake and partitioning in five individual 10-year old Picea mariana [black spruce] trees to identify and quantify dynamics of key morphological/physiological processes. Trees were subjected to one of two treatments: (i) well-watered control or (ii) drought and rehydration. We monitored changes in morphological [shoot volume, leaf mass area (LMA)], biochemical [osmolality, non-structural carbohydrates (NSC)] and physiological [rates of respiration (Rd) and light-saturated photosynthesis (Asat)] processes during shoot development. Further, to study functional compartmentalization and use of new assimilates, we 13C-pulse labeled shoots at multiple development stages, and measured isotopic signatures of leaf respiration, NSC pools and structural biomass. Shoot water potential dropped to a minimum of −2.5 MPa in shoots on the droughted trees. Development of the photosynthetic apparatus was delayed, as shoots on well-watered trees broke-even 14 days prior to shoots from trees exposed to water deficit. Rd decreased with shoot maturation as growth respiration declined, and was lower in shoots exposed to drought. We found that shoot development was delayed by drought, and while rehydration resulted in recovery of Asat to similar levels as shoots on the well-watered trees, shoot volume remained lower. Water deficit during shoot expansion resulted in longer, yet more compact (i.e., with greater LMA) shoots with greater needle osmolality. The 12C:13C isotopic patterns indicated that internal C partitioning and use was dependent on foliar developmental and hydration status. Shoots on drought-stressed trees prioritized allocating newly fixed C to respiration over structural components. In conclusion, temporary water deficit delayed new shoot development and resulted in greater LMA in black spruce. Since evergreen species such as black spruce retain active foliage for multiple years, impacts of early season drought on net primary productivity could be carried forward into subsequent years.
Current global climate models not only predict increases in temperature due to climate change, but also altered precipitation regimes, potentially with increased frequency, duration and intensity of drought periods (IPCC, 2013; Spinoni et al., 2018). The combination of changing temperature and precipitation patterns will alter net ecosystem carbon assimilation, especially in the northern boreal ecosystems (Mäkipää et al., 1999; Way et al., 2013) where warming is expected to be particularly acute (IPCC, 2013). In addition to altered precipitation regimes, temperature-driven changes in vapor pressure deficit will increase plant water demand (Ficklin and Novick, 2017; Grossiord et al., 2020), which could exacerbate reductions in boreal ecosystem productivity by amplifying drought conditions and enhancing mortality (Kirschbaum, 2000). Warming has direct consequences for dormancy, bud break, development of photosynthetic capacity and growth (Way and Sage, 2008; Dhuli et al., 2014; Lee et al., 2014; Richardson et al., 2018). Further, the confounding effect of drought during periods of critical physiological development such as spring bud break and shoot expansion can affect seasonal, and for key boreal conifers such as Picea that retain foliage for several years, multi-annual rates of carbon assimilation. Since most of the global terrestrial carbon pool is stored in boreal ecosystems (Dixon et al., 1994), investigating the effects of climate change on dominant boreal species is central to understanding the net-carbon balance of these critical ecosystems.
In boreal forests, annual canopy development is essential for maintaining plant vigor and competitive status. For evergreen species in particular, new shoot development is a significant carbon sink that will provide multiple years of photosynthetic carbon (C) gain. Development of new shoots early in the growing season requires substantial investment, altering the within canopy source-sink relationships, and tapping into stored C reserves (Turgeon, 1989; Kozłowski, 1992; Kozlowski and Pallardy, 2002). Expanding leaves or needles begin as heterotrophic organs that rely on C allocated from other sources inside the plant (Turgeon, 1989; Sprugel, 2002). The specific C investment associated with foliar development is dependent on when the developing shoot becomes self-sufficient [i.e., the sink-source transition when C assimilation becomes greater than the C demands of respiration and growth (Turgeon, 1989)]. Therefore, fast and complete development of an efficient photosynthetic apparatus is critical for maximizing a plant's C-economy.
For boreal evergreen conifers, spring and early summer entails large changes in C allocation within the canopy. Highly mobile non-structural carbohydrates (NSC) play a central role during this period of bud break and shoot development. NSCs can be re-allocated between different sinks, such as storage, defense, osmoregulation, respiration or growth, and dynamics are highly variable during springtime (Chapin et al., 1990; Schädel et al., 2009; Fløistad and Granhus, 2010; Woodruff and Meinzer, 2011; Martínez-Vilalta et al., 2016; Furze et al., 2018).
Elevated air and soil temperatures initiate de-hardening of overwintered foliage and photosynthesis in older needle cohorts (Vegis, 1964; Goodine et al., 2008; Fløistad and Granhus, 2010). Stored NSCs are remobilized and accumulated in needles and buds during bud break (Mandre et al., 2002) and are the main support for early growth of current-year shoots (Fischer and Höll, 1991). This initial accumulation of NSCs may persist if bud break and shoot development is restricted for example by water deficit or low temperatures. NSCs are stored in wood, roots, and buds, and in evergreen trees also in overwintering foliage (Richardson et al., 2013; Furze et al., 2018; Schiestl-Aalto et al., 2019). While some deciduous species have shown significant decreases in starch reserves at distant locations (i.e., roots and stems) during bud-break (Bonhomme et al., 2005; Alves et al., 2007), only small changes of NSC were found in branch sapwood of Picea abies and Pinus sylvestris (Schädel et al., 2009). This suggests that stored NSCs in nearby older needles contribute substantially to the developing shoots, either through export of newly fixed C or older stored NSCs.
The effects of environmental factors such as temperature, photoperiod length, and CO2 concentration on bud break and shoot development have been studied extensively in various Picea species (see for example Slaney et al., 2007; Fløistad and Granhus, 2010; Karst and Landhäusser, 2014; De Barba et al., 2016). However, much less is known on the effects of drought (see references in Way et al., 2013). Here we focus on Picea mariana (black spruce), which is widely distributed across the boreal zone in North America and is thought to be sensitive to future elevated summer temperatures (Dang and Lieffers, 1989; Way and Sage, 2008; Dymond et al., 2019). In addition, P. mariana has a relatively shallow root system, especially on mesic and wet sites (Tarroux et al., 2014; Iversen et al., 2018), making it sensitive even to periods of mild water deficiency (Warren et al. under review). As in many boreal conifer species, bud and shoot maturation of P. mariana is relatively slow. We have earlier shown that new shoot development, including the maturation of the photosynthetic apparatus, is a prolonged process of several weeks (Jensen et al., 2015). The combination of a shallow root system and a long shoot and needle maturation period also increases the likelihood of a drought occurring while shoots are developing. Furthermore, as current-year shoots start out as net C sinks, delayed shoot development due to temperature-, or drought-stress, will likely affect net carbon assimilation patterns, respiration rates, and structural development. On a seasonal basis, current-year shoots contribute less to overall carbon assimilation than older needle cohorts (Jensen et al., 2015, 2019). Thus, it is likely that a restriction in early shoot development will affect their primary function (carbon assimilation) during the current and subsequent growing seasons. This is particularly relevant in light of the fact that P. mariana retains its foliage for up to 7 years.
While progressive drought as a whole may have lasting impacts on forest composition, dynamics, and C balance (Smith, 2011; Doughty et al., 2015; Anderegg et al., 2018; Trugman et al., 2018), the effects are first seen on individual trees. Drought constrains plant physiology and productivity (Allen et al., 2010), for example by restricting growth during bud break, as cells are not able to expand. During periods of water deficiency plants are often not able to maintain sufficient cell turgor pressure to allow for optimal cell wall expansion (Hsiao, 1973). This can lead to tissues with shorter and smaller cells (see Le Gall et al., 2015). Upon re-hydration, the already developed secondary cell wall tissue may not be able to further expand (Lockhart, 1965). Growth reduction can be especially detrimental during bud break and shoot development for boreal conifers with determinate growth patterns producing only a single flush per season, since this is when new photosynthetic organs develops. By contrast, the impact of early season drought would be reduced for southern conifers that can have multiple flushes, making compensation possible. Early season drought can restrict total annual aboveground growth for conifers (Swidrak et al., 2013), which has implications for forest development and seasonal patterns of net primary production at the ecosystem level. Assessing the impact of drought on shoot development in P. mariana can therefore give important insights into the future of boreal forests.
Against this background, the objective of this study was to quantify the impact of temporary drought followed by re-hydration on internal carbon dynamics and shoot development and maturation in P. mariana trees. We hypothesized that drought during this period would lead to reduced C uptake and impact shoot morphology. Specifically, we expect that water deficit would: (i) delay needle and shoot development, (ii) lower photosynthetic capacity and (iii) increase NSC content. Finally, (iv) we predict that drought will entail shifts in allocation and use of newly fixed carbon, prioritizing osmoregulation. To meet these objectives, we conducted a controlled experiment where we monitored shoot development, physiological development (Asat and Rd) and NSC dynamics from early stages of bud and shoot development, to full shoot maturation in trees exposed to a springtime drought period. The drought treatment consisted of a 40-day water deficit period, where the relative soil water content was kept at 80%. Further, we 13C-pulse labeled developing individual branches during different stages of shoot development, to study functional compartmentalization of newly fixed photosynthates by tracing respiration 13CO2 efflux, and 13C concentrations in non-structural carbohydrates and in structural tissues.
We use two main analytical methods to study the effect of drought on carbon use and partitioning within developing shoots: Quantifying the natural abundance of tissue δ13C and pulse labeling with 13CO2. Starting with the former method, the natural abundance of δ13C is a result of photosynthetic 13C-discrimination. The degree of 13C-discrimination is dependent on the ratio of leaf internal to ambient CO2 concentrations (Ci:Ca), which functions as a proxy for plant water relations and environmental effects. For example, low Ci:Ca ratios show that stomata are closed and will result in decreased discrimination against 13C (Farquhar et al., 1989). The second method, 13C pulse labeling, is a powerful tool to trace newly assimilated C through trees. The aim of this could e.g., be to better understand within-tree circulation, or to analyze plant-plant/plant-microbes interactions (Epron et al., 2012; Warren et al., 2012) and leverage enriched 13C, in order to bypass the discrimination process so that new and old carbon can be separated. This makes the method especially useful to resolve C investment and use of newly fixated carbon.
Six 10-year old Picea mariana (Mill.) B.S.P. trees were obtained from a commercial nursery outside Minneapolis, Minnesota, USA. The trees were 160–170 cm tall with a stem diameter at ground level of 7–10 cm. The trees had been grown in full light in an open field (sandy loam). In December 2012, the dormant trees were excavated, burlapped and stored for > 30 days outdoors in the shade until shipped to Oak Ridge National Laboratory, Oak Ridge, Tennessee, USA. Upon arrival (2-days after shipping) the trees were put into pots (~30 L), keeping the root system as intact as possible using a mixture of sandy loam and peat moss. The trees were dormant for at least 8 weeks prior to the current growth chamber experiment. To induce bud break, the trees were grown the first week under 18/8°C (day/night) in a walk-in growth chamber (BDW, Controlled Environments Ltd., Winnipeg Manitoba Canada). The five trees, were randomly moved between two growth chambers once a week. For the duration of the experiment (18 weeks) trees were grown at 14h days with a 30 min ramping up to full light (500 μmol photons m–2 s–1) at air temperatures and relative humidity of 25/8°C and 40/80% (day/night), respectively. Due to growth-chamber problems half of the trees (of both treatments) experienced 15 h. with higher temperatures (33/20°C). Although not desirable in this growth chamber experiment, temperatures like these are not uncommon in P. mariana's natural habitat (Hanson et al., 2015, see public data in Hanson et al., 2017).
Prior to treatments, the trees were watered to field capacity every 2–3 days. Six weeks into the experiment, trees were randomly assigned to either a temporary drought treatment or a well-watered control treatment. Specifically, three trees were assigned to the temporary drought treatment (n = 3), and two trees were assigned to the well-watered control treatment (n = 2). Tree number 1, 2, and 3 were assigned the temporary drought treatment and tree number 4 and 6 the watered control treatment (tree number 5 died just after potting). Thereafter, all trees stayed in their assigned treatment for the rest of the experiment. Trees in the drought treatment followed an irrigation regime (watered every 3–5 days) with a target of 20% reductions in soil water content compared to the well-watered control treatment (averaging around 13 cm3 water cm3 volume). The drought treatment lasted for 40 days, and thereafter the droughted trees were rehydrated in a single irrigation event, here after all trees followed the same irrigation regime. Soil volumetric water content was measured using 5TM soil moisture sensors (Decagon Devices Inc., WA, USA).
What is the motivation behind choosing our particular drought treatment? From a plant perspective, the severity of a given drought depends on factors such as the intensity, i.e., how much water that is available to the plant (precipitation and soil moisture) in relation to the specific plant's water demand, the duration of the drought and the phenological timing (i.e., which phenological stage the plant is in) (Bréda et al., 2006; Yan et al., 2016; Banks et al., 2019). The drought we imposed in our experiment can be characterized as relatively mild in terms of intensity. Using, for example, the classification from Yan et al. (2016), where the severity of drought intensities is defined as relative soil water content between 56–70% (mild), 41–55% (moderate), and 0–40% (severe), our drought with a relative soil water content of 80% would perhaps best be categorized as very mild. In terms of duration and phenological timing, however, our imposed drought was more severe, lasting 40 days and taking place during the shoot development.
An important motivation behind choosing a water deficit treatment that is mild in terms of intensity is that this is the most common intensity experienced by P. mariana trees during spring in their natural habitat, as the spring thaw of ice and snow largely buffers more severe droughts. In the American boreal-temperate transition zone, mild to moderate drought occurs most frequently (U.S. Drought Portal; www.drought.gov/drought/states/minnesota), although moderate and severe drought intensities do also occur. While the drought in our experiment was mild in terms of intensity, it was more severe in terms of the phenological timing. Since shoot development is the time when the plant is building up its future photosynthetic capacity, a drought at that stage poses bigger problems for the plant than if it occurred, say, mid growth season. Thus, even though we studied a mild intensity drought, it nevertheless occurred at a, for the plant, phenologically critical time.
We measured shoot water potential (Ψ) and needle sap osmolality for all trees at 06:00 (pre-dawn) and 18:00 (pre-sunset), every 2nd to 3rd day throughout the experiment. Shoot water potential was measured using a pressure chamber (PMS Instruments, USA) and needle sap osmolality was determined using a Vapor Pressure Osmometer 5520 (Wescor, EliTechGroup, USA). For the experimental time line, see Supplementary Table 1.
Bud and shoot development were classified as belonging to one of six stages: (1) dormant buds, (2) swollen buds, (3) bud break (i.e., separation of scales with visible needles), (4) shoot elongation without needle separation (5) shoot elongation with needle separation, and (6) stagnation of shoot elongation and fully mature needles (Supplementary Figure 1). We monitored bud and shoot development over time for three to five terminal first-order shoots on three branches per tree and treatment. To ensure a similar light environment, branches were selected in the top of the canopy. Once a week shoot development, growth, and gas exchange were measured. We measured shoot diameter and length of the leading bud/shoot every 2nd to 3rd day on the same upper-canopy branch. Thus, the same bud/shoot was repeatedly measured throughout the experiment. Diameter and length were measured using a caliper, and shoot volume calculated (shoot volume = length * diameter * π). After 19 weeks (stage 6) shoots were harvested to determine final shoot mass, and projected needle area (PA). Shoots were defoliated and PA was estimated using WinRHIZO (Regent Instruments Canada Inc., Canada). Needles and twigs were dried at 70°C for dry mass determination.
Net assimilation (Asat) and respiration rates (Rd) were measured at ambient CO2 concentration (400 ppm) at photosynthetic photon flux density (PPFD) of 700 (day-time) and 0 μmol m–2 s–1 (night-time) during bud and shoot development using a portable infrared gas analyzer (LI6400XT, LI-COR, NE, USA). Cuvette humidity and temperatures were set to 25/8°C and 40/80% (day/night). During early stages of bud development (stages 1 and 2, bud length 1–5 mm), several buds from a single branch were selected and gas exchange measured using a mesh bag inserted in a 2 × 3 cm chamber. When these shoots became bigger Asat and Rd were measured while still attached to the branch using a 2 × 3 cm (stage 3 and 4, shoot length 5–40 mm) chamber and an Opaque conifer (stage 5 and 6, shoot length 40–110 mm) chamber. In order to isolate the new tissue within the gas exchange chamber, prior to measurements, older needles were carefully removed without damaging the twigs. In addition to the 60 shoots that were measured over time, at each developmental stage buds and shoots were collected from adjacent branches to determine: Volume (VShoot), mass (MShoot), leaf area, and non-structural carbohydrate (NSC) concentration. Shoot volume was calculated based on shoot length and diameter assuming a cylindrical shape of the shoot. Rates of CO2 exchange are expressed on MShoot basis. When MShoot could not be obtained, VShoot was converted to MShoot using the following relationships derived from measurements of clipped tissues: Trees assigned to control treatment, MShoot = 8.87 + 23.44* VShoot + (−0.3303)* (R2 = 0.992) and trees in the drought treatment, Mshoot = 24.22 + 34.55* VShoot + (−0.5038)* V2Shoot, (R2 = 0.992), see Supplementary Figure 2.
Water-soluble organic matter (WSOM) and non-soluble organic matter (NSOM) was extracted from needles and twigs of developing (Y0) and 1-year old (Y1) shoots as follows. First, we took samples, each weighing 8–16 mg and dried and homogenized, and added 1.6 ml deionized water (dH20) to each sample. The sample was then steamed at >100°C for 90 min and centrifuged (15 min at 1,3000 rpm) to separate the soluble fraction (supernatant) from the non-soluble fraction (pellet). We separated the two factions and evaporated the water at 70°C and analyzed the delta δ13C and C content, see above. We assume that the WSOM faction contains a mixture of sugars, organic acids and amino acids all with high turnover rates (Brandes et al., 2006).
We analyzed non-structural carbohydrate content from both WSOM and NSOM using an enzymatic technique according to Woodruff and Meinzer (2011). In summary, we added 1.6 ml dH20 to 6–16 mg of dry homogenized plant material. Samples were steamed (>100°C) for 90 min, to extract water soluble sugars allocated into two factions for determination of sucrose, fructose, glucose and starch. Sucrose was converted into glucose and fructose units via invertase, while starch was converted to glucose units via amyloglucosidase. Subsequently the samples were reacted with a glucose hexokinase solution based on a commercial glucose assay reagent (G3293, MilliporeSigma, St.Louis, MO, USA) and phosphoglucose isomerase resulting in glucose-6P. Glucose equivalents could be estimated based on accumulation of the NADH cofactor by analyzing the reaction products at 340 nm using a spectrophotometer (SpectraMax Plus 384, Molecular Devices, San Jose, CA).
To track newly assimilated carbon 13C-pulse labeling of individual branches was conducted at shoot-developmental stages 3–6. The first 13C-labeling event was just after the net assimilation had become positive (Asat > 0), and the last was when the terminal shoot had matured (Asat stabilized, shoot developmental stage 6). The targeted branch tip was covered with a plastic bag (Teflon® FEP fluorocarbon film, DuPont, DE, USA), closed up at the base of the branch. Typically, 5–7 terminal buds or developing shoots were enclosed by the bag. To avoid labeling older needles, and potential influx of fresh 13C-enriched assimilates from older needles to the developing buds and shoots, all older needles were either covered with tinfoil or carefully clipped from the branch (Supplementary Figure 3). Depending on stage, the branches were labeled by adding lactic acid in excess to 12–25 mg 13C-enriched (99 atom % 13C) bicarbonate (Sigma-Aldrich, USA), through the plastic bag using a syringe. The branches were labeled for 90 min with a fan mixing the air inside the bag. Bag temperatures were 2–4°C higher than the chamber air temperature (25°C). We monitored the [13CO2], [12CO2], and δ13CO2 of the growth chambers and labeling bag using a Picarro G1101-i Isotopic CO2 Analyzer (Picarro Inc., Sunnyvale, USA). The isotope mass spectrometer was calibrated against CO2 gas standards (Scotty Specialty Gas Calibration Standards, USA). The δ13C in the growth chamber air and inside the labeling bags after 90 min were −9.18 and 570–4,081, respectively. At each labeling event, two branches—one on a well-watered tree and one on a branch on a droughted tree—were labeled. This happened at 8 different times: 61, 64, 68, 70, 75, 77, 117, and 119 days into the experiment. In total, we therefore labeled 16 individual branches.
We monitored respiration and its 13CO2 efflux at 5, 15, and 30 min, then 3, 6, 12, 24, 48 h, and 5-days after each 13C labeling event on the same terminal developing shoot using a portable infrared gas analyzer (LI6400XT, LI-COR, NB, USA) with settings as above. Although CO2 efflux by the shoot likely contains xylem dissolved CO2 originating from other organs (Teskey et al., 2008), we were not able to differentiate between CO2 sources; therefore, we assume that CO2 efflux measured in this study originate only from the developing shoot's respiration. CO2 efflux was collected by attaching a gas sample bag (Chemware, NC, USA) to the chamber air exhaust outlet and the [13CO2] measured using a Picarro G1101-i Isotopic CO2 Analyzer (Picarro Inc., Sunnyvale, CA, USA). Shoot volume development was recorded and at the final measurement point (5-days) the shoot was harvested. In addition, current-year (developing) and 1-year old shoots were collected 5, 30 min and 5 days after the labeling event. Plant tissues were separated into needles and twigs. Ground samples were 13C analyzed using an Integra CN isotope ratio mass spectrometer (SerCon Ltd, Crewe, UK). An internal working glucose standard (δ13C = −10.2) was used for isotope analysis. Our glucose standard was calibrated against reference material from the National Institute of Standards and Technology (NIST 8542, sucrose).
We calculated the isotope ratios, expressed as δ13Csample () using the Pee Dee Belemnite (PDB) standard: δ13C = (Rsample/Rstandard −1), where R is the molar ration of 13C:12C for the sample and the standard. The excess δ13C was calculated as δ13CEcxcess = (δ13CSample − δ13CBackground), using δ13C values for specific LI-COR CO2 cylinders used (from −30.133 to −22.303) and non-labeled developing needles (−25.140 ± 0.967) as background for respiration 13CO2 efflux [δ13CO2Rd_Excess ()] and plant tissues [δ13CO2Mass_Excess, ()], respectively. Exponential decay models were fitted to individual leaf respiration isotopic release patterns through time according to Zang et al. (2014). In contrast to mature tissues, we observed a 2nd peak around 14:00–18:00 (500–700 min) depending on treatment and shoot developmental stage. Therefore, only points prior to this 2nd peak was used to fit:
where δ13Ct () is the enrichment level at time t, δ13Ct0 () initial level, and k the estimated decay constant (min–1). This allowed us to calculate mean residence time (MRT) of the 13C label by MRT = 1/k. To adjust for different 13C concentrations in the labeling bags between pulse-labeling events we calculated the relative δ13C of the efflux from the respiration in relation to values of respiratory CO2 δ13C immediately after the labeling had stopped at each given labeling event. Bulk δ13C in sugars and biomass were also adjusted using the changes in δ13CO2 efflux at each individual labeling event.
Treatment effects on soil water, shoot water potential, shoot osmolality, and natural abundance of δ13C pellet and supernatant were tested using an ANOVA model. The effects of treatment and time (day of experiment) were analyzed using a linear mixed model fitted using maximum likelihood and Satterthwaite's method, with random effects for every individual tree. The dependent variables in the linear mixed models were Asat (nmol g–1 s–1), Rd (nmol g–1 s–1), shoot volume (cm3), shoot surface (cm3) and LMA (g m–2), total NSC (mg g–1), starch (mg g–1), sucrose (mg g–1), fructose and glucose (mg g–1), natural abundance of δ13C pellet (), natural abundance of δ13C supernatant (), the relative δ13C of respiratory CO2 efflux (30 min, 12 h and 5 days after the 13C pulse-labeling), δ13C of soluble sugars [pellet and supernatant (30 min, and 5 days after the 13C pulse-labeling)], δ13C of needles and twigs [(30 min, and 5 days after the 13C pulse-labeling)] and MRT (h). Non-linear regression models were used to analyze relationship between MRT and Rd, Rd and day of experiment, Asat and day of experiment. Non-linear regression models were also used for the relationship between shoot volume and shoot length and dry mass. All treatment of data and statistical analysis were done in R 3.0.0.
Reduced water availability delayed photosynthetic development and general shoot-maturation (Figure 1, Supplementary Figure 2). Overall mean values of light saturated photosynthesis (Asat) were significantly negatively affected by the drought treatment, and became greater as shoots matured (Table 1, Supplementary Table 3, Figure 1A). The negative effect of drought was particularly pronounced toward the end of the drought period. In fact, Asat became positive 14 days later than it did in well-watered trees (Figure 1A). Typically, self-sufficiency (Asat > 0 nmol g–1 s–1) occurred after initial needle elongation but before the needles had reached 5 mm length. The rate of respiration declined exponentially over time for trees in both treatments as the shoot matured. In well-watered trees it changed from mean Rd values of 272 to 12 nmol g–1 s–1. In drought-exposed trees it changed from 213 to 13 nmol g–1 s–1 (Figure 1B). Rates of respiration were significantly negatively affected by the water deficit (Table 1, Figure 1B). Shoot expansion, expressed here as shoot volume, followed a sigmoidal growth function (Figure 1C). While the shape of the growth responses over time were similar between treatments, overall shoot development was delayed and restricted in trees assigned to the drought treatment (Table 1, Figure 1C), resulting in shoots with significantly smaller (~32%) volume, and greater LMA after 120 days (Table 1, Figure 1C, Supplementary Figure 2). For example, in trees assigned to the drought treatment, shoot development stagnated in stages three and four during the drought (Figure 1C, Supplementary Figure 1). By contrast, we found no evidence of delayed shoot development in the trees assigned to the well-watered treatment. Further, we also observed that a single tree, and even a single branch, sometimes experienced more than one developmental stage at the same time. However, this variation has not been quantified.
Figure 1. Developmental changes in (A) light saturated assimilation rates (Asat), (B) respiration rates (Rd), and (C) shoot volume in well-watered (black symbols) and drought (red symbols) 10-year old P. mariana trees. Variables were measured at different shoot developmental stages: stage 2 swollen bud with the scales still covering the new needles (open circles), stage 3 bud scales diverging but no elongation of the shoot (half-filled circles), stage 4 elongation of twig and needles without needle spreading (left side filled circles), stage 5 elongation of twig needles and needles spread (3/4 filled circles), and stage 6 stagnation of shoot elongation and fully mature needles (filled circles). The gray area indicates the period of water deficiency and the eight vertical dotted lines the 13C pulse-labeling events. In (B) relationships between day of experiment (x) and Rd (y) are y = 4* 107 * x−3.183 (R2 = 0.946) and y = 2*106* x−2.486, (R2 = 0.919) for the well-watered and droughted trees, respectively. (C) Relationships between day of experiment (x) and shoot volume (y) are y = (44.004 + 1.369)/[1 + exp (78.328–x)/4.52] and y = (30.351 + 1.225)/[1 + exp(81.612–x)/5.43] for the well-watered and droughted trees, respectively. Mean ± 1SD, n = 2–3 trees.
During the drought period that lasted 40 days, volumetric soil water content was ~20% lower in the drought treatment than in the well-watered treatment. Soil water generally declined during the day, as soil dried up, with on average greater values pre-dawn (at 06:00) (Supplementary Table 2). This was the case for trees in both treatments. Following rehydration, soil water content was similar both between treatment, and across time of day and day of experiment (Supplementary Table 2). Prior to the drought period, average shoot water potential (Ψ) in 1-year old twigs was −0.36 and −0.59 MPa at 06:00 and 18:00, respectively (similar between treatments, results not shown). The temporary drought treatment significantly decreased values of Ψ 2–3-fold (at 06:00) and 2–4-fold (at 18:00) compared to well-watered trees, with some droughted shoots dropping below −1.8 MPa by the end of the day (Supplementary Table 2, Figure 2, Supplementary Figure 4). Re-hydration of droughted trees returned Ψ to pre-treatment values with no significant difference between treatments (Supplementary Table 2). Both during and after the temporary drought treatment, Ψ was greater at 06:00 than at 18:00. The temporary drought increased the needle sap osmolality of developing shoots, whereas re-hydration returned values similar to the shoots on well-watered trees. Although restricted by a low number of observations, the drought treatment increased needle sap osmolality of 1-year old shoots (Supplementary Table 2, Figure 2). Needle sap osmolality in developing shoots declined as shoot water potential took values closer to 0 (Figure 2).
Figure 2. Relationship between shoot water potential (Ψ) and needle sap osmolality in needles of well-watered (black symbols) and droughted (red symbols) P. mariana trees. Individual observations from current year (Y0) shoots in different development stages: Stage 2 (open circles), stage 3 (half-filled circles), stage 4 (left side filled circles), and stage 6 (filled circles). Values for 1-year old (Y1) shoots are showed as filled diamonds. Significant relationships between Ψ (x) and needle sap osmolality (y) for developing shoots on well-watered trees (black line, y = 537.4–390.7x, R2 = 0.356, p < 0.001) and droughted trees (red line, y = 697.2–146.6x, R2 = 0.256, p < 0.001).
Depending on the type of sugar, its concentrations measured in developing- and 1-year old shoots varied between treatment, tissue age and day of experiment (Table 2, Figures 3A,B,D,E). Most of the soluble sugars were starch, with concentrations ranging on average between 2.81–8.41 mg g–1, and 2.89–10.24 mg g–1 in new developing shoots and fully mature 1-year old shoots, respectively (Figure 3B). Day of experiment had a significantly positive effect on total NSC in developing shoots but a negative effect in 1-year old shoots (Table 2, Supplementary Table 4, Figure 3A). End of dormancy, bud break or photosynthetic resumption generally entailed a reduction in starch and sucrose, a pattern not observed for fructose and glucose (Figures 3B,D,E). In current year shoots, starch and sucrose concentration decreased as the shoot developed, to increase again after full maturation was achieved (Figures 3B,D). In developing shoots drought had a significant positive effect on concentrations of total NSC, starch, fructose, and glucose, whereas the concentrations of sucrose were unaffected (Table 2, Figures 3A–E). This effect also persisted after rehydration, when both treatments' shoots had reached full maturation. It is possible that the observed large variation is related to the limited number of trees.
Table 2. The effect of the drought treatment and day of experiment on concentrations of the soluble sugars in developing current year shoots and 1-year old shoots of black spruce.
Figure 3. (A,B,D,E) Non-structural carbohydrate concentrations and (C–F) their natural abundance of 13C, in black spruce for current-year (Y0) and 1-year old shoots (Y1) when trees are well-watered (black symbols) or exposed to a temporary drought period (red symbols). Developmental stages: 1 (stars), 2 (open circles), 3 (half-filled circles), and 6 (filled circles). One-year old shoots were all classified as stage 6 (filled diamonds). For the non-structural sugars, the pellet is a proxy for starch whereas the supernatant contains highly soluble sugars as sucrose, fructose, and glucose. The blue color indicates the 40-day long drought period. Mean ± 1SD, n = 2–3 trees.
Drought had a significantly positive effect on the natural abundance of δ13C in the pellet (a proxy for starch), whereas drought had a significant negative effect on δ13C in the supernatant (a proxy for sucrose, fructose and glucose) (Table 2, Figures 3C,F). As the shoots matured, δ13C of the pellet significantly increased but not δ13C of the supernatant (Table 2, Figures 3C,F). At the end of the experiment [when trees were rehydrated and the shoots where fully matured (stage 6)] the natural abundance of δ13C in the pellet where similar between treatments, whereas natural abundance of δ13C in the supernatant were still significantly lower in the droughted trees (ANOVA: F = 5.2, p = 0.042). In non-stressed trees with fully mature needles, leaf-level isotopic discrimination resulted in greater values of natural abundance δ13C in the supernatant than the pellet. The reverse pattern was observed when trees were exposed to drought stress (Supplementary Figure 5).
When we 13C-pulse-labeled the developing shoots, the 13C followed a classical decay function in the CO2 efflux. Relative δ13C from respiration was significantly greater in the droughted trees than in the well-watered trees 30 min and 12 h, however not 5 days, after the labeling event (Table 3, Figure 4A). Note that in Figure 4A, points above the 1:1 line indicate that the plant prioritized C allocation toward respiration. Shoot maturation (i.e., time), on the other hand, significantly positively influenced the relative δ13C in the efflux 30 min after labeling. However, there was no effect at either 12 h or 5 days after labeling (Table 3). MRT was negatively nonlinearly related to the rate of respiration, with significantly greater values of shoot MRT in drought-stressed trees than in well-watered trees [(Mixed model (estimate (SE), p-value): ln (MRT) = ln (Rd) (−0.85 (0.11)), 1.25e–5, treatment (0.75 (0.18), 0.002), intercept (3.72 (0.12), 5.57e–12)), number of observations 16 in four groups], Figure 5. For both treatments MRT, declined over time as trees were re-watered and shoots matured. The temporary drought had no significant short- or long-term effect on the δ13C of the soluble sugars. However, shoot maturation significantly increased δ13C both in the supernatant [a proxy for easily soluble sugars (i.e., sucrose, fructose and glucose)] and in the pellet [a proxy for less soluble sugars (i.e., starch), Table 3, Figure 4B]. The label was detected in both needles and twigs 30 mins and 5 days after the 13C label stopped. Carbon-13 in needle and twigs samples was significantly negatively affected by the temporary drought (so in terms of Figure 4C, these points are below the 1:1 line). However, it was positively affected by day of the experiment (Table 3, Figure 4C), likely as a result of greater CO2 assimilation capacity (Table 1, Figure 1A). Across developmental stages values of δ13C were somewhat greater in needles than in the twigs (Table 3, Supplementary Table 5, Figure 4C). In summary, results suggest that drought-stressed trees prioritized C allocation into respiratory metabolism over new structural matter, while NSC pools remain unaffected.
Table 3. Summary of linear mixed models, quantifying effects of drought, and day of experiment of δ13C of respiratory CO2, soluble sugars (pellet and supernatant), needles, and twigs from current year developing shoots of black spruce.
Figure 4. Relationship between δ13C in well-watered and droughted P. mariana trees in (A) CO2 efflux from the respiration, (B) non-structural-carbohydrates and (C) needles and twigs, 30 min (blue squares), 12 h (purple stars), and 5 days (green diamonds) after 13C-pulse labeling. In panel a, values are relative to values of respiratory CO2 δ13C immediately after the labeling had stopped. In (B,C) values of δ13C are adjusted according to labeling strength. Values are individual branches [in (B,C) error bars indicate the variation (SD) from 3 to 4 shoots on that particular branch] from different 13C-pulse labeling events, thus in different developmental stages. The black line is the one-to-one relationship.
Figure 5. Relationship between rates of mean residence time (MRT) of the 13C label in respiratory CO2 efflux and respiration (Rd) in well-watered (black symbols) and droughted (red symbols) trees. Regression lines between Rd (x) and MRT (y) are y = 55.24 x−1.01, adj. R2 = 0.971, p < 0.001, for the well-watered trees (full black line) and y = 25.94 x−0.69, adj. R2 = 0.916, p < 0.001 (red broken line). One observation is a individual shoot from an individual tree at a specific 13C pulse-labeling event. Please note that due to the destructive nature of the sampling, individual shoots was only studied once, so different shoots were studied at different 13C pulse-labeling events.
When 10-year old P. mariana trees were exposed to a temporary water deficit during the critical developmental stages of bud break and shoot development, we observed morphological, physiological, and biochemical responses that affect both short-, and potentially, long-term carbon assimilation, use, and partitioning.
We found that drought significantly decreased Asat and Rd during shoot development. After rehydration, and upon full maturation, values became similar to those observed in well-watered trees. The reduction in Asat, and the fact that the shoot became self-sufficient 14 days later in the drought treatment than in the well-watered treatment, may be a result of reduced translocation and loading during drought (Sevanto, 2018). Even though photosynthesis is not one of the first processes to be affected by water deficit, the maturation process of the photosynthetic apparatus in the shoot may slow down as the import of sugars from older organs slows down or stops, see review by McDowell (2011). At the same time, the tissue is in one of its most active growth stages, with high rates of respiration, which means that the shoot is a strong sink organ for the plant. In mature leaves, drought can initially decrease respiration, as a consequence of inhibition of growth and cellular maintenance (see Flexas et al., 2005 and references herein), thus the reduction in respiration rate observed here is likely a good indicator of both reduced maturation and growth in droughted shoots. After rehydration, the rate of C-assimilation was similar between treatments, implying a fast recovery of the photosynthetic apparatus and maturation processes.
What are the implications of this drought-induced delay in carbon uptake? In the current experiment we only observed a temporary effect on Asat and Rd during the drought treatment. Since the delay in photosynthetic maturation, and the reduction in C-uptake are likely small compared to the shoot's annual C-uptake upon full maturation, the overall consequences for the plant in terms of short-term C-uptake may be limited, although importantly, the significant reduction in photosynthetic area may amplify the impact of the drought. This is underscored by the fact that the current-year shoot of P. mariana typically contributes relatively little to the plant's total carbon uptake, which is dominated by uptake from older cohorts of needles (Jensen et al., 2019).
While temporary drought during the shoot development does not necessarily indicate negative effects on the current year's carbon uptake at the tree level, there may be longer-term effects. Even after 47 days of recovery, shoots on trees that had experienced drought had significantly smaller shoot volume and greater LMA, and therefore low light interception and C-uptake. This suggests that the developing shoot may not have been able to maintain sufficient turgor—despite clear evidence that both osmoregulation and a build-up of NSC occurred—resulting in fewer and smaller cells than the cells of developing shoots from well-watered trees. Therefore, the observed greater shoot density may be a function of more cell wall per area than if the trees had not been water-stressed. If this is the case, it would have implications for the within-needle CO2-diffusion pathway, decreasing mesophyll conductance and possibly increasing CO2 re-assimilation (see for example Tan et al., 1992; Stewart et al., 1995; Eckert et al., 2020). On the other hand, having needles with greater LMA may be an advantage during summer droughts, a trade-off between drought avoidance and desiccation recovery observed in arid species (Fallon and Cavender-Bares, 2018).
The effects of temporary drought on shoot density is key to understanding why the long-term consequences on C-uptake may be quite substantial. Even though the drought-damaged current-year shoot limitation on C-uptake may not be a major problem for the plant in the first year, it may have greater implications for subsequent years, when the current year's shoots would normally contribute more to C uptake (Jensen et al., 2019). Needles in black spruce are retained for up to 7 years. Therefore, the accumulated negative long-term consequences for the tree's overall C-uptake could be quite substantial.
Lastly, it is important to note that the drought in our experiment had a long duration. Even though the intensity of the drought was mild, the treatment lasted for a fairly long time (40 days). With such a long treatment, there is enough time for the cell size to set. Had the duration of the drought been shorter, it is possible that the LMA effect would have been smaller, or even that the rehydration could result in a complete re-expansion of the cells. With this long duration, however, the effects are likely irreversible. Much emphasis has previously been given to the intensity of water deficits and in particular droughts with a severe intensity (see for example McDowell, 2011). Our results illustrated that even a relatively mild intensity drought (even with relatively few trees in the experiment) can have substantial implications for the needle and canopy morphology if the duration is substantial, or the drought occurs at critical stages of shoot development.
The dynamics of NSC concentration over time are driven by the growth and maturation process in developing shoots, whereas for the overwintering 1-year old shoots, the reduction in NSC is likely due to energy- and C-requiring processes of deharding, such as photosynthetic resumption (Bergh and Linder, 1999; Bigras and Bertrand, 2006; Wallin et al., 2013). In addition, members of Picea have been shown to store NSC in their needles, especially starch, over the winter (Schädel et al., 2009; Dhuli et al., 2014). Thus, the observed drop in total NSC and starch in older needles may also be a result of stored carbon from older foliage being reallocated to other sink organs such as new and developing needles/shoots. The greater values of total NSC, starch, fructose, and glucose in developing shoots on trees exposed to drought, may be a result of osmoregulation. In mature organs exposed to water stress, growth declines before photosynthesis, resulting in an NSC surplus (Kozlowski and Pallardy, 2002; Hummel et al., 2010). However, in our study on juvenile foliage, photosynthetic rates were low (often below the breakeven point), thus making such a drought-driven NSC build-up unlikely. Carbon translocation is often disrupted by severe drought (McDowell, 2011; Sevanto, 2018). In our study, however, the drought treatment did not affect δ13C of the soluble sugars after the 13C pulse-labeling events. This implies that the carbon used for osmoregulation was most likely reallocated old carbon from other organs. Although the shoots exposed to water deficit were able to osmoregulate, tissue density increased, which suggests that there was not a complete disruption but rather a restriction in C-translocation.
Our experiment confirmed that in both treatments, bud break and shoot maturation resulted in a reduction in total NSC, driven by a reduction in starch concentration. A similar reduction in total NSC and starch was observed in 1-year old shoots, as shoots broke dormancy. Both these results are in line with previous research (see for example Schädel et al., 2009). Water deficit further increased the concentrations of total NSC. This has also been found in some previous studies (see references in Hartmann and Trumbore, 2016), but typically in mature tissues.
While the primary aim of the paper was to understand the impact of temporary drought and re-hydration on shoot development and carbon investment in P. mariana, we also analyzed the underlying mechanisms. Pulse-13C labeling, showed that developing shoots exposed to water deficit prioritized newly assimilated C toward respiration over structural tissues.
In spite of having relatively few experimental trees, we also found that MRT decreased with increasing rates of dark respiration and that the MRT was greater in the shoots that experienced drought. To the best of our knowledge, these are novel results. Lower respiration rates and water-stress induced reduction in growth likely slowed C use by the droughted shoot, which was reflected by the increase in MRT of labeled C. In addition, the shifts in recent photosynthate partitioning suggest that temporary periods of water deficiency reduce C translocation from older organs and that the imported C is used for osmoregulation. Often, under non-stressed conditions, the older organs feed key cellular processes such as mitochondrial respiration. Although water deficit lowers mitochondrial respiration with on average 23 nmol g–1 s–1, the rates are still relatively high (~150–210 nmol g–1 s–1) compared to values in mature non-expanding black spruce needles (Jensen et al., 2015, 2019). Rates of respiration, measured here as CO2 efflux, were high for shoots in stages 2–4. Although this was expected considering their active metabolic stage, some of the CO2 could also originate from older organs being transported in the expanding bud/shoot (a strong sink) (Turgeon, 1989; Kozłowski, 1992; Kozlowski and Pallardy, 2002; Teskey et al., 2008). Thus, results from the 13C pulse-labeling suggest that newly fixed carbon is allocated to respiration and away from structural components, whereas soluble sugars used as osmolites likely are imported.
A likely implication of this redirection of newly fixed C in drought-stressed trees is delayed shoot maturation. This can occur through two mechanisms: (i) limited shoot capacity to osmoregulate, which will confine shoot ability to reach the optimal/potential capacity for cell turgor and thus cell size and (ii) less shoot investment of newly fixed C in structural tissues. This implies higher LMA and smaller needles, and potentially a lower area based photosynthetic capacity (potentially restricting net annual C- uptake for several years).
Results from natural abundance of δ13C measurements showed that drought affected 13C discrimination. Specifically, we observed a positive drought effect on the natural abundance of δ13C in the pellet but a negative effect in the supernatant. It should be noted that the δ13C of the growth chamber air was similar to outside air. This result partly agrees with earlier observations of black spruce tree ring δ13C, where the aggregated 13C signal in wood xylem declined (i.e., the natural abundance of 13C became more abundant) with increasing moisture limitation due to reduced stomata conductance (see Walker et al., 2015). For example, (Warren et al. under review) compared multiple conifers and found a negative relationship between Ψ and δ13C in stem wood. However, of relevance for this paper, they also point out that the relationship was weak during spring, i.e., at budbreak, likely due to mixing of old and new C. This may, together with our new empirical results, suggest that the positive relationship between drought and δ13C is much less general than what was previously thought. This would be worthwhile testing in the field at a larger scale.
One possible explanation for our mixed relationships between droughts and δ13C is the following. Short periods of water deficit often induce reductions of the stomatal conductance simply because the mature needles close their stomata. However, during development, stomata are most likely not fully developed (and references herein Casson and Gray, 2008) and thus not fully functional. Thus, when the drought occurs during development stages, the relationship between drought and δ13C shifts. Another possible explanation for the negative drought effect found in our study is that we analyzed a different level of aggregation than what has typically been the case. We focused on soluble sugars, i.e., water-soluble organic matter at the shoot level, whereas other studies, such as Walker et al. (2015) aggregate at organ level without pool separation.
If co-existing species react differently to drought, this likely shapes species composition and may therefore strongly influence ecosystem function (Beck et al., 2011; Peng et al., 2011). Our finding that even a mild intensity drought has substantial negative effects on shoot size, and thus canopy development may for the individual tree entail a reduced net annual carbon uptake, potentially lasting for several years. For evergreen conifer species like P. mariana this may result in loss of competition capacity compared to deciduous conifer or broadleaved trees that do not retain a drought-reduced canopy for several years after a particular drought. If spring droughts become more frequent in a future warmer climate, the difference between functional groups may become even greater. Whether or not this will shift species composition and ecosystem function at the stand level is unclear as other members of the community will have been negatively affected by the drought as well. At a large landscape scale study in interior Alaska, Sullivan et al. (2017) found that radial growth in P. mariana trees was sensitive to even mild reductions in moisture availability. However, they did not observe the expected reduction in growth, suggesting that the rising temperature may offset the negative impact of water deficit.
To summarize, our study shows that drought events during early development of P. mariana shoots affect the current year's carbon partitioning and shoot morphology, with potential long-term effects on whole plant carbon uptake. We found that developing shoots experiencing drought allocated carbon away from structural components and toward respiratory and osmoregulation processes. While rates of photosynthesis recovered after the drought was terminated, shoot volume remained low, an effect that could potentially impact P. mariana productivity in the following years. Thus, if extensive spring drought occurs during budbreak, there are significant implications for net ecosystem exchange and climate feedbacks within the boreal forest biome.
The raw data supporting the conclusions of this article will be made available by the authors, without undue reservation.
This article has been authored by UT-Battelle, LLC under Contract No. DE-AC05-00OR22725 with the US Department of Energy. The United States Government retains and the publisher, by accepting the article for publication, acknowledges that the United States Government retains a non-exclusive, paid-up, irrevocable, world-wide license to publish, or reproduce the published form of this article, or allow others to do so, for United States Government purposes. The Department of Energy will provide public access to these results of federally sponsored research in accordance with the DOE Public Access Plan (http://energy.gov/downloads/doe-public-access-plan).
AJ and JW conceived the initial research questions. AJ, KC, MP, and JW were responsible for the collection and statistical analysis of the data. AJ, MP, DE, JW, and KC contributed to the writing of the article. All authors contributed to the article and approved the submitted version.
Research was sponsored by the U.S. Department of Energy, Office of Science, Office of Biological and Environmental Research. Oak Ridge National Laboratory is managed by UT-Battelle, LLC, for the U.S. Department of Energy under contract DE-AC05-00OR22725. AJ and DE were funded also by Swedish Research Council (VR), grant 2015-05083 as well as Linnaeus University.
The authors declare that the research was conducted in the absence of any commercial or financial relationships that could be construed as a potential conflict of interest.
The authors appreciate fieldwork and data analysis from Joanne Childs, Ingrid Slette, Jeffery Riggs, Deanne Brice, and Colleen Iversen.
The Supplementary Material for this article can be found online at: https://www.frontiersin.org/articles/10.3389/ffgc.2020.601046/full#supplementary-material
Allen, C. D., Macalady, A. K., Chenchouni, H., Bachelet, D., McDowell, N., Vennetier, M., et al. (2010). A global overview of drought and heat-induced tree mortality reveals emerging climate change risks for forests. For. Ecol. Manage. 259, 660–684. doi: 10.16/j.foreco.2009.09.001
Alves, G., Decourteix, M., Fleurat-Lessard, P., Sakr, S., Bonhomme, M., Améglio, T., et al. (2007). Spatial activity and expression of plasma membrane H+-ATPase in stem xylem of walnut during dormancy and growth resumption. Tree Physiol. 27, 1471–1480. doi: 10.93/treephys/27.10.1471
Anderegg, W. R. L., Konings, A. G., Trugman, A. T., Yu, K., Bowling, D. R., Gabbitas, R., et al. (2018). Hydraulic diversity of forests regulates ecosystem resilience during drought. Nature 561, 538–541. doi: 10.38/s41586-018-0539-7
Banks, J. M., Percival, G. C., and Rose, G. (2019). Variations in seasonal drought tolerance rankings. Trees 33, 1063–1072. doi: 10.07/s00468-019-01842-5
Beck, P. S. A., Juday, G. P., Alix, C., Barber, V. A., Winslow, S. E., Sousa, E. E., et al. (2011). Changes in forest productivity across alaska consistent with biome shift. Ecol. Lett. 14, 373–379. doi: 10.1111/j.1461-0248.2011.01598.x
Bergh, J., and Linder, S. (1999). Effects of soil warming during spring on photosynthetic recovery in boreal Norway spruce stands. Glob. Chang. Biol. 5, 245–253. doi: 10.46/j.1365-2486.1999.00205.x
Bigras, F. J., and Bertrand, A. (2006). Responses of Picea mariana to elevated CO2 concentration during growth, cold hardening and dehardening: phenology, cold tolerance, photosynthesis and growth. Tree Physiol. 26, 875–888. doi: 10.93/treephys/26.7.875
Bonhomme, M., Rageau, R., Lacointe, A., and Gendraud, M. (2005). Influences of cold deprivation during dormancy on carbohydrate contents of vegetative and floral primordia and nearby structures of peach buds (Prunus persica L. batch). Sci. Hortic. 105, 223–240. doi: 10.16/j.scienta.2005.01.015
Brandes, E., Kodama, N., Whittaker, K., Weston, C., Rennenberg, H., Keitel, C., et al. (2006). Short-term variation in the isotopic composition of organic matter allocated from the leaves to the stem of Pinus sylvestris: effects of photosynthetic and postphotosynthetic carbon isotope fractionation. Glob. Chang. Biol. 12, 1922–1939. doi: 10.1111/j.1365-2486.2006.01205.x
Bréda, N., Huc, R., Granier, A., and Dreyer, E. (2006). Temperate forest trees and stands under severe drought: a review of ecophysiological responses, adaptation processes and long-term consequences. Ann. For. Sci. 63, 625–644. doi: 10.51/forest:2006042
Casson, S., and Gray, J. E. (2008). Influence of environmental factors on stomatal development. N. Phytol. 178, 9–23. doi: 10.1111/j.1469-8137.2007.02351.x
Chapin, I. S. S., Schulze, E. D., and Mooney, H. A. (1990). The ecology and economics of storage in plants. Annu. Rev. Ecol. Syst. 21, 423–447. doi: 10.1146/annurev.es.21.110190.002231
Dang, Q. L., and Lieffers, V. J. (1989). Climate and annual ring growth of black spruce in some alberta peatlands. Can. J. Bot. 67, 1885–1889. doi: 10.1139/b89-239
De Barba, D., Rossi, S., Deslauriers, A., and Morin, H. (2016). Effects of soil warming and nitrogen foliar applications on bud burst of black spruce. Trees 30, 87–97. doi: 10.07/s00468-015-1152-0
Dhuli, P., Rohloff, J., and Strimbeck, G. R. (2014). Metabolite changes in conifer buds and needles during forced bud break in Norway spruce (Picea abies) and European silver fir (Abies alba). Front. Plant Sci. 5:706. doi: 10.3389/fpls.2014.00706
Dixon, R. K., Brown, S., Houghton, R. A., Solomon, A. M., Trexler, M. C., and Wisniewski, J. (1994). Carbon pools and flux of global forest ecosystems. Science 263, 185–190. doi: 10.1126/science.263.5144.185
Doughty, C. E., Metcalfe, D. B., Girardin, C. A. J., Amézquita, F. F., Cabrera, D. G., Huasco, W. H., et al. (2015). Drought impact on forest carbon dynamics and fluxes in amazonia. Nature 519, 78–82. doi: 10.38/nature14213
Dymond, S. F., D'Amato, A. W., Kolka, R. K., Bolstad, P. V., Sebestyen, S. D., Gill, K., et al. (2019). Climatic controls on peatland black spruce growth in relation to water table variation and precipitation. Ecohydrology 12:e2137. doi: 10.02/eco.2137
Eckert, D., Jensen, A. M., and Gu, L. (2020). The maximum carboxylation rate of rubisco affects CO2 refixation in temperate broadleaved forest trees. Plant Physiol. Biochem. 155, 330–337. doi: 10.16/j.plaphy.2020.06.052
Epron, D., Bahn, M., Derrien, D., Lattanzi, F. A., Pumpanen, J., Gessler, A., et al. (2012). Pulse-labelling trees to study carbon allocation dynamics: a review of methods, current knowledge and future prospects. Tree Physiol. 32, 776–798. doi: 10.93/treephys/tps057
Fallon, B., and Cavender-Bares, J. (2018). Leaf-level trade-offs between drought avoidance and desiccation recovery drive elevation stratification in arid oaks. Ecosphere 9:e02149. doi: 10.02/ecs2.2149
Farquhar, G. D., Ehleringer, J. R., and Hubick, K. T. (1989). Carbon isotope discrimination and photosynthesis. Annu. Rev. Plant Physiol. Plant Mol. Biol. 40, 503–537. doi: 10.1146/annurev.pp.40.060189.002443
Ficklin, D. L., and Novick, K. A. (2017). Historic and projected changes in vapor pressure deficit suggest a continental-scale drying of the United States atmosphere. J. Geophys. Res. 122, 2061–2079. doi: 10.02/2016JD025855
Fischer, C., and Höll, W. (1991). Food reserves of scots pine (Pinus sylvestris L.). Trees 5, 187–195. doi: 10.07/BF00227524
Flexas, J, Galmes, J, Ribas-Carbo, M, and Medrano, H. (2005) ‘The Effects of water stress on plant respiration,’ in Plant Respiration. Advances in Photosynthesis and Respiration (Vol 18), eds H. Lambers, M. Ribas-Carbo (Dordrecht: Springer). doi: 10.1007/1-4020-3589-6_6
Fløistad, I. S., and Granhus, A. (2010). Bud break and spring frost hardiness in picea abies seedlings in response to photoperiod and temperature treatments. Can. J. For. Res. 40, 968–976. doi: 10.1139/X10-050
Furze, M. E., Jensen, A. M., Warren, J. M., and Richardson, A. D. (2018). Seasonal patterns of nonstructural carbohydrate reserves in four woody boreal species. J. Torrey Bot. Soc. 145, 332–339. doi: 10.3159/TORREY-D-18-00007.1
Goodine, G. K., Lavigne, M. B., and Krasowski, M. J. (2008). Springtime resumption of photosynthesis in balsam fir (Abies balsamea). Tree Physiol. 28, 1069–1076. doi: 10.93/treephys/28.7.1069
Grossiord, C., Buckley, T. N., Cernusak, L. A., Novick, K. A., Poulter, B., Siegwolf, R. T. W., et al. (2020). Plant responses to rising vapor pressure deficit. New Phytol. 226, 1550–1566. doi: 10.1111/nph.16485
Hanson, P. J., Riggs, J. S., Forrance, C., Nettles, W. R., and Hook, L. A. (2015). SPRUCE Environmental Monitoring Data: 2010-2016. Oak Ridge, TN: Oak Ridge National Laboratory, U.S. Department of Energy, Carbon Dioxide Information Analysis Center.
Hanson, P. J., Riggs, J. S., Nettles, W. R., Phillips, J. R., Krassovski, M. B., Hook, L. A., et al. (2017). Attaining whole-ecosystem warming using air and deep-soil heating methods with an elevated CO2 atmosphere. Biogeosciences 14, 861–883. doi: 10.5194/bg-14-861-2017
Hartmann, H., and Trumbore, S. (2016). Understanding the roles of nonstructural carbohydrates in forest trees - from what we can measure to what we want to know. New Phytol. 211, 386–403. doi: 10.1111/nph.13955
Hsiao, T. C. (1973). Plant responses to water stress. Annu. Rev. Plant Physiol. 24, 519–570. doi: 10.1146/annurev.pp.24.060173.002511
Hummel, I., Pantin, F., Sulpice, R., Piques, M., Rolland, G., Dauzat, M., et al. (2010). Arabidopsis plants acclimate to water deficit at low cost through changes of carbon usage: an integrated perspective using growth, metabolite, enzyme, and gene expression analysis. Plant Physiol. 154, 357–372. doi: 10.1104/pp.110.157008
IPCC (2013). “Climate change 2013: the physical science basis. Contribution of working group I to the fifth assessment report of the intergovernmental panel on climate change,” in Contribution of Working Group I to the Fifth Assessment Report of the Intergovernmental Panel on Climate Change Cambridge, eds T. F. Stocker, D. Qin, G. K. Plattner, M. Tignor, S. K. Allen, J. Boschung, et al. (New York, NY: Cambridge University Press), 33–119.
Iversen, C. M., Childs, J., Norby, R. J., Ontl, T. A., Kolka, R. K., Brice, D. J., et al. (2018). Fine-root growth in a forested bog is seasonally dynamic, but shallowly distributed in nutrient-poor peat. Plant Soil 424, 123–143. doi: 10.07/s11104-017-3231-z
Jensen, A. M., Warren, J. M., Hanson, P. J., Childs, J., and Wullschleger, S. D. (2015). Needle age and season influence photosynthetic temperature response and total annual carbon uptake in mature Picea mariana trees. Ann. Bot. 116, 821–832. doi: 10.93/aob/mcv115
Jensen, A. M., Warren, J. M., King, A. W., Ricciuto, D. M., Hanson, P. J., and Wullschleger, S. D. (2019). Simulated projections of boreal forest peatland ecosystem productivity are sensitive to observed seasonality in leaf physiology†. Tree Physiol. 39, 556–572. doi: 10.93/treephys/tpy140
Karst, J., and Landhäusser, S. M. (2014). Low soil temperatures increase carbon reserves in Picea mariana and Pinus contorta. Ann. For. Sci. 71, 371–380. doi: 10.07/s13595-013-0344-2
Kirschbaum, M. U. (2000). Forest growth and species distribution in a changing climate. Tree Physiol. 20, 309–322. doi: 10.93/treephys/20.5-6.309
Kozłowski, J. (1992). Optimal allocation of resources to growth and reproduction: implications for age and size at maturity. Trends Ecol. Evol. 7, 15–19. doi: 10.16/0169-5347(92)90192-E
Kozlowski, T. T., and Pallardy, S. G. (2002). Acclimation and adaptive responses of woody plants to environmental stresses. Bot. Rev. 68, 270–334. doi: 10.1663/0006-8101(2002)068[0270:AAAROW]2.0.CO;2
Le Gall, H., Philippe, F., Domon, J. M., Gillet, F., Pelloux, J., and Rayon, C. (2015). Cell wall metabolism in response to abiotic stress. Plants 4, 112–166. doi: 10.3390/plants4010112
Lee, Y. K., Alexander, D., Wulff, J., and Olsen, J. E. (2014). Changes in metabolite profiles in Norway spruce shoot tips during short-day induced winter bud development and long-day induced bud flush. Metabolomics 10, 842–858. doi: 10.07/s11306-014-0646-x
Lockhart, J. A. (1965). An analysis of irreversible plant cell elongation. J. Theor. Biol. 8, 264–275. doi: 10.16/0022-5193(65)90077-9
Mäkipää, R., Karjalainen, T., Pussinen, A., and Kellomäki, S. (1999). Effects of climate change and nitrogen deposition on the carbon sequestration of a forest ecosystem in the boreal zone. Can. J. For. Res. 29, 1490–1501. doi: 10.1139/x99-123
Mandre, M., Tullus, H., and Klõseiko, J. (2002). Partitioning of carbohydrates and biomass of needles in scots pine canopy. Z Naturforsch C J Biosci. 57, 296–302. doi: 10.1515/znc-2002-3-417
Martínez-Vilalta, J., Sala, A., Asensio, D., Galiano, L., Hoch, G., Palacio, S., et al. (2016). Dynamics of non-structural carbohydrates in terrestrial plants: a global synthesis. Ecol. Monogr. 86, 495–516. doi: 10.02/ecm.1231
McDowell, N. G. (2011). Mechanisms linking drought, hydraulics, carbon metabolism, and vegetation mortality. Plant Physiol. 155, 1051–1059. doi: 10.1104/pp.110.170704
Peng, C., Ma, Z., Lei, X., Zhu, Q., Chen, H., Wang, W., et al. (2011). A drought-induced pervasive increase in tree mortality across canada's boreal forests. Nat. Clim. Chang. 1, 467–471. doi: 10.38/nclimate1293
Richardson, A. D., Carbone, M. S., Keenan, T. F., Czimczik, C. I., Hollinger, D. Y., Murakami, P., et al. (2013). Seasonal dynamics and age of stemwood nonstructural carbohydrates in temperate forest trees. New Phytol. 197, 850–861. doi: 10.1111/nph.12042
Richardson, A. D., Hufkens, K., Milliman, T., Aubrecht, D. M., Furze, M. E., Seyednasrollah, B., et al. (2018). Ecosystem warming extends vegetation activity but heightens vulnerability to cold temperatures. Nature 560, 368–371. doi: 10.38/s41586-018-0399-1
Schädel, C., Blöchl, A., Richter, A., and Hoch, G. (2009). Short-term dynamics of nonstructural carbohydrates and hemicelluloses in young branches of temperate forest trees during bud break. Tree Physiol. 29, 901–911. doi: 10.93/treephys/tpp034
Schiestl-Aalto, P., Ryhti, K., Mäkelä, A., Peltoniemi, M., Bäck, J., and Kulmala, L. (2019). Analysis of the NSC storage dynamics in tree organs reveals the allocation to belowground symbionts in the framework of whole tree carbon balance. Front. For. Glob. Chang. 2:17. doi: 10.3389/ffgc.2019.00017
Sevanto, S. (2018). Drought impacts on phloem transport. Curr. Opin. Plant Biol. 43, 76–81. doi: 10.16/j.pbi.2018.01.002
Slaney, M., Wallin, G., Medhurst, J., and Linder, S. (2007). Impact of elevated carbon dioxide concentration and temperature on bud burst and shoot growth of boreal Norway spruce. Tree Physiol. 27, 301–312. doi: 10.93/treephys/27.2.301
Smith, M. D. (2011). The ecological role of climate extremes: current understanding and future prospects. J. Ecol. 99, 651–655. doi: 10.1111/j.1365-2745.2011.01833.x
Spinoni, J., Vogt, J. V., Naumann, G., Barbosa, P., and Dosio, A. (2018). Will drought events become more frequent and severe in Europe? Int. J. Climatol. 38, 1718–1736. doi: 10.02/joc.5291
Sprugel, D. G. (2002). When branch autonomy fails: milton's law of resource availability and allocation. Tree Physiol. 22, 1119–1124. doi: 10.93/treephys/22.15-16.1119
Stewart, J. D., el Abidine, A. Z., and Bernier, P. Y. (1995). Stomatal and mesophyll limitations of photosynthesis in black spruce seedlings during multiple cycles of drought. Tree Physiol. 15, 57–64. doi: 10.93/treephys/15.1.57
Sullivan, P. F., Pattison, R. R., Brownlee, A. H., Cahoon, S. M. P., and Hollingsworth, T. N. (2017). Limited evidence of declining growth among moisture-limited black and white spruce in interior alaska. Sci. Rep. 7:15344. doi: 10.38/s41598-017-15644-7
Swidrak, I., Schuster, R., and Oberhuber, W. (2013). Comparing growth phenology of co-occurring deciduous and evergreen conifers exposed to drought. Flora 208, 609–617. doi: 10.16/j.flora.2013.09.004
Tan, W., Blake, T. J., and Boyle, T. J. B. (1992). Drought tolerance in faster- and slower-growing black spruce (Picea mariana) progenies: I. Stomatal and gas exchange responses to osmotic stress. Physiol. Plantar. 85, 639–644. doi: 10.1111/j.1399-3054.1992.tb04766.x
Tarroux, E., DesRochers, A., and Girard, J. P. (2014). Growth and root development of black and white spruce planted after deep planting. For. Ecol. Manage. 318, 294–303. doi: 10.16/j.foreco.2014.01.032
Teskey, R. O., Saveyn, A., Steppe, K., and McGuire, M. A. (2008). Origin, fate and significance of CO2 in tree stems. N. Phytol. 177, 17–32. doi: 10.1111/j.1469-8137.2007.02286.x
Trugman, A. T., Detto, M., Bartlett, M. K., Medvigy, D., Anderegg, W. R. L., Schwalm, C., et al. (2018). Tree carbon allocation explains forest drought-kill and recovery patterns. Ecol. Lett. 21, 1552–1560. doi: 10.1111/ele.13136
Turgeon, R. (1989). The sink-source transition in leaves. Annu. Rev. Plant Physiol. Plant Mol. Biol. 40, 119–138. doi: 10.1146/annurev.pp.40.060189.001003
Vegis, A. (1964). Dormancy in higher plants. Annu. Rev. Plant Physiol. 15, 185–224. doi: 10.1146/annurev.pp.15.060164.001153
Walker, X. J., Mack, M. C., and Johnstone, J. F. (2015). Stable carbon isotope analysis reveals widespread drought stress in boreal black spruce forests. Glob. Chang. Biol. 21, 3102–3113. doi: 10.1111/gcb.12893
Wallin, G., Hall, M., Slaney, M., Räntfors, M., Medhurst, J., and Linder, S. (2013). Spring photosynthetic recovery of boreal Norway spruce under conditions of elevated [CO2] and air temperature. Tree Physiol. 33, 1177–1191. doi: 10.93/treephys/tpt066
Warren, J. M., Iversen, C. M., Garten, C. T. Jr., Norby, R. J., Childs, J., Brice, D., et al. (2012). Timing and magnitude of C partitioning through a young loblolly pine (Pinus taeda L.) stand using 13C labeling and shade treatments. Tree Physiol. 32, 799–813. doi: 10.93/treephys/tpr129
Way, D. A., Crawley, C., and Sage, R. F. (2013). A hot and dry future: warming effects on boreal tree drought tolerance. Tree Physiol. 33, 1003–1005. doi: 10.93/treephys/tpt092
Way, D. A., and Sage, R. F. (2008). Elevated growth temperatures reduce the carbon gain of black spruce [Picea mariana (Mill.) BSP]. Glob. Chang. Biol. 14, 624–636. doi: 10.1111/j.1365-2486.2007.01513.x
Woodruff, D. R., and Meinzer, F. C. (2011). Water stress, shoot growth and storage of non-structural carbohydrates along a tree height gradient in a tall conifer. Plant Cell Environ. 34, 1920–1930. doi: 10.1111/j.1365-3040.2011.02388.x
Yan, W., Zhong, Y., and Shangguan, Z. (2016). A meta-analysis of leaf gas exchange and water status responses to drought. Sci. Rep. 6:20917. doi: 10.38/srep20917
Keywords: carbon limitation, stable isotope labeling, CO2 efflux, carbon allocation, pulse labeling
Citation: Jensen AM, Eckert D, Carter KR, Persson M and Warren JM (2021) Springtime Drought Shifts Carbon Partitioning of Recent Photosynthates in 10-Year Old Picea mariana Trees, Causing Restricted Canopy Development. Front. For. Glob. Change 3:601046. doi: 10.3389/ffgc.2020.601046
Received: 31 August 2020; Accepted: 03 December 2020;
Published: 12 January 2021.
Edited by:
Rosana López Rodríguez, Polytechnic University of Madrid, SpainReviewed by:
Roberto L. Salomon, Polytechnic University of Madrid, SpainCopyright © 2021 Jensen, Eckert, Carter, Persson and Warren. This is an open-access article distributed under the terms of the Creative Commons Attribution License (CC BY). The use, distribution or reproduction in other forums is permitted, provided the original author(s) and the copyright owner(s) are credited and that the original publication in this journal is cited, in accordance with accepted academic practice. No use, distribution or reproduction is permitted which does not comply with these terms.
*Correspondence: Anna M. Jensen, YW5uYS5qZW5zZW5AbG51LnNl; orcid.org/0000-0001-5113-5624
†Present address: Anna M. Jensen, Department of Forestry and Wood Technology, Linnaeus University, Växjö, Sweden
Kelsey R. Carter, Earth and Environmental Sciences Division, Los Alamos National Laboratory, Los Alamos, NM, United States
Disclaimer: All claims expressed in this article are solely those of the authors and do not necessarily represent those of their affiliated organizations, or those of the publisher, the editors and the reviewers. Any product that may be evaluated in this article or claim that may be made by its manufacturer is not guaranteed or endorsed by the publisher.
Research integrity at Frontiers
Learn more about the work of our research integrity team to safeguard the quality of each article we publish.