- 1Institut des Sciences de l’Évolution de Montpellier (ISEM), EPHE, PSL University, Université de Montpellier, CNRS, IRD, Montpellier, France
- 2Institut de Recherche sur les Forêts (IRF), Université du Québec en Abitibi-Témiscamingue, Rouyn-Noranda, QC, Canada
- 3Centre d’Étude de la forêt (CEF), Université du Québec à Montréal, Montréal, QC, Canada
- 4Natural Resources Canada, Canadian Forest Service, Laurentian Forestry Centre, Sainte-Foy, QC, Canada
- 5Département de Géographie, Université de Montréal, Montréal, QC, Canada
Wildland fire is the most important disturbance in the boreal forests of eastern North America, shaping the floral composition, structure and spatial arrangement. Although the long-term evolution of the frequency and quantity of burned biomass in these forests can be estimated from paleo-ecological studies, we know little about the evolution of fire sizes. We have therefore developed a methodological approach that provides insights into the processes and changes involved over time in the historical fire-vegetation-climate environment of the coniferous forests (CF) and mixedwood forests (MF) of eastern boreal North America, paying particular attention to the metric of fire size. Lacustrine charcoal particles sequestered in sediments from MF and CF regions were analyzed to reconstruct changes in estimated burned biomass, fire frequency, and their ratio interpreted as fire size (FS-index), over the last 7,000 years. A fire propagation model was used to simulate past fire sizes using both a reference landscape, where MF and CF compositions over time were prescribed using pollen reconstructions, and climate inputs provided by the HadCM3BL-M1 snapshot simulations. Lacustrine charcoals showed that Holocene FS-indices did not differ significantly between MF and CF because of the high variability in fire frequencies. However, the estimated burned biomass from MF was always lower than that from CF, significantly so since 5,000 BP. Beyond the variability, the FS-index was lower in MF than CF throughout the Holocene, with slight changes in both forests from 7,000 to 1,000 BP, and simultaneous increases over the last millennium. The fire model showed that MF fires were consistently smaller than CF fires throughout the Holocene, with larger differences in the past than today. The fire model also highlighted the fact that spring fires in both forest types have always been larger than summer fires over the last 7,000 years, which concurs with present-day fire statistics. This study illustrates how fire models, built and used today for forecasting and firefighting, can also be used to enhance our understanding of past conditions within the fire-vegetation-climate nexus.
Introduction
Wildland fire is the most important disturbance in boreal forests, shaping the composition, structure and spatial arrangement of the flora. Its occurrence is the result of complex interactions between climate, weather, topography, and vegetation (Whelan, 1995). Under global warming scenarios, it is anticipated that the frequency, intensity and size of fires will increase as a result of increasing dryness (Flannigan et al., 2009). However, it is also recognized that, to some extent, vegetation composition and structure, as well as fragmented environments, could dampen such a trajectory (Pechony and Shindell, 2010; Chaste et al., 2019). To sustain the future usage and services of the boreal forest, since 1995 Canada has been implementing an ecosystem-based management system (Burton et al., 2003; Gauthier et al., 2009, 2015) in which the logging regime is inspired by natural disturbance. For example, the distribution of harvested area across the landscape over time, and the type of logging (total or selective clear-cutting within stands), is based on fire and insect outbreak history maps built using dendrochronological studies; coarse woody debris and a few snags are left in situ for the benefit of biodiversity; and practices mimicking the impact of disturbances on humus and soil conditions are used to enhance regeneration. For the management regimes to mimic disturbances and their impacts, the natural variability in disturbance that forests have sustained over time (i.e., fire history) is quantified using paleo-ecological reconstructions (Hennebelle et al., 2018). These reconstructions, combined with modeling experiments, allow us to understand the processes involved in the interplay between climate, vegetation, and disturbance. Such an approach also allows the future to be viewed from a long-term perspective, and helps guide sound management of forested landscapes (Waito et al., 2015).
In eastern North America, the closed-crown boreal forest encompasses two ecological regions roughly divided at 49°N, with coniferous forest (CF) to the north, and mixedwood forest (MF) to the south (Rowe, 1972). The historical fire regimes of these areas are among the most documented within the boreal biome (Gauthier et al., 2009). To date, the data held covers all aspects relating to temporal and spatial trajectories in terms of (i) estimated burned biomass, which has been shown to correlate with area burned and fire severity in several North American boreal forest regions (e.g., Higuera et al., 2011; Ali et al., 2012; Kelly et al., 2013; Hennebelle et al., 2020); (ii) fire frequency; and (iii) the spatial distribution of fires within both MF and CF (Girardin et al., 2013a,b, 2019; Blarquez et al., 2015a). Inferences have been made from a suite of fire data proxies, including fire-scars recovered from trees (e.g., Girardin et al., 2006; Le Goff et al., 2008), stand-replacing fire-histories (e.g., Bergeron et al., 2001; Lauzon et al., 2007), and charcoal records collected from soils (Payette, 1992), peat (e.g., van Bellen et al., 2011) and lake sediments (e.g., Carcaillet et al., 2001; Ali et al., 2012; Remy et al., 2017). These research efforts have revealed that, in coniferous-dominated forests, the estimated burned biomass (i.e., amount of charcoal produced) and fire frequency (i.e., number of fires per unit of time) have decreased over the past 4,500 years BP, in parallel with a reduction in solar radiation at high latitudes and the estimated fire-prone season length for these forests (Hély et al., 2010b). However, since the decrease in burned biomass is less pronounced than the decrease in fire frequency, their ratio, interpreted as a fire size index, has increased (Ali et al., 2012). These fire trajectories differ from those reported for locations further south, in MF, where the estimated burned biomass has increased since ca. 4,500 BP (Blarquez et al., 2015a), with no apparent change in fire frequency (Girardin et al., 2013a). According to pollen records (Carcaillet et al., 2001), the cooler and moister conditions in MF are not favorable for broadleaf species, and subsequently have contributed to an increase in fire-prone coniferous species (i.e., increased pollen influx of conifer taxa) and their relative proportion (Blarquez and Aleman, 2016), which has stimulated fire activity over the last 1,500 years (Carcaillet et al., 2001; Girardin et al., 2013a).
Although the long-term evolution of the frequency and estimated quantity of burned biomass in these two forest types is relatively well known, our knowledge of the evolution of fire sizes is limited. However, such information could be of value for forest management practices that take inspiration from natural disturbances (e.g., Hirsch et al., 2001), notably for setting targets related to the size of harvestable area (e.g., Delong and Tanner, 1996; Bergeron et al., 2002; Belleau et al., 2007). It is recognized that contemporaneous CF and MF, under the same climatic conditions, differ in terms of fire size and fire severity (Bergeron et al., 2004). Notably, smaller and less severe fires tend to be prevalent in MF. The lower flammability of broadleaf fuels in MF supports such an interpretation (Forestry Canada Fire Danger Group, 1992; Wotton et al., 2009), although conditions for spreading fires are often high before leaf-out, when direct sunlight reaches and dries the dead fine fuels on the surface. Therefore, while present-day lightning activity tends to peak later in summer (July–August), effective lightning ignitions in boreal MF tend to be more active in the spring.
Based on existing historical information (i.e., charcoal and pollen), and assuming that burned biomass reflects the compounded effects of fire frequency, size and severity, our aim was to test whether MF fires, as well as CF fires, were smaller prior to 4,500 BP (when the estimated levels of burned biomass and fire frequency were lower) than after 4,500 BP (when the estimated levels of burned biomass were higher but fire frequency remained almost unchanged). Given that, within a paleo-historical context, there is no direct indicator of fire size, and fire size and fire severity may be confounded in terms of charcoal biomass sequestered in recent sediments or even deposited on surficial lacustrine traps (Kelly et al., 2013; Hennebelle et al., 2020), we used a fire propagation model to test our fire size hypothesis.
Several modeling tools describing mechanistic functions, with varying degrees of complexity representing the fire-vegetation-climate environment, currently exist for studying fire responses to vegetation and climatic changes (e.g., Krawchuk et al., 2012; Michetti and Zampieri, 2014; Fisher et al., 2018). These models are often used for projecting future fire behavior in response to climatic and anthropogenic changes (Pechony and Shindell, 2010; Boulanger et al., 2017; Chaste et al., 2019). We have developed a complementary methodological approach that provides insights into the processes and changes involved over time in the historical fire-vegetation-climate environment of the CF and MF of eastern boreal North America. By populating a spatially explicit probabilistic fire propagation model (Anderson, 2010) with pollen information and paleo-climate simulations, we have simulated fire size during the Holocene. By comparing simulated fire sizes over time and between forests with those observed and archived by provincial agencies for both forest types, we can provide a more applied perspective for the current situation in both forests. Boreal MF and CF are thought to be delimited by both fire size and fire severity (Bergeron et al., 2004), and the proposed approach will facilitate greater insight into the fire size component. Our working hypotheses were as follows.
(i) The size of simulated fires has been significantly smaller in MF, compared with CF, since deglaciation 8,000 years ago, i.e., the onset of vegetation differentiation between southern MF and northern CF.
(ii) Because of the desiccation of fuels before leaf-out, spring fires should account for almost all of the total annual burned area in MF.
(iii) The contemporaneous increase in proportion of needleleaf species in MF with early fire-season start over the last 1,500 years may have offset the generally less fire-prone conditions that had developed since the onset of the Neoglacial climate 4,500 years ago, leading to larger fires than before.
Materials and Methods
Study Area
The study area is located in the eastern Canadian boreal CF and MF, from approximately 46–51°N to approximately 73–88°W (Figure 1). MF and CF are located south and north of 49°N (Rowe, 1972), respectively, with the transition shifting slightly further northward toward the west (Figure 1). The climate of the study area is continental, with cold winters and warm summers, while soil patterns are homogeneous at the macroscopic regional scale: the topography in CF is generally flat, and the most important surficial deposits is organic soil, followed by clay deposit, while MF has a more rolling topography and clay deposits are dominant, followed by organic deposits (Bergeron et al., 2004). Conversely, the forest composition mosaic is heterogeneous between the forest types. CF is naturally dominated by two needleleaf species (black spruce and jack pine), while MF is dominated by a mixture of broadleaf and needleleaf species (Supplementary Material S1), the relative proportions depending mainly on post-fire stand succession ages (Dansereau and Bergeron, 1993). Pollen reconstructions have shown that the proportions of needleleaf species in MF have increased significantly over the last 1,500 years (Richard, 1980; Liu, 1990; Carcaillet et al., 2001), although all tree species have been present since the drainage of the proglacial Lake Ojibway at ca. 7,900 BP (Richard, 1980). In contrast, the vegetation composition of CF has remained the same over the last 7,000 years, i.e., mostly dominated by black spruce (Carcaillet et al., 2001).
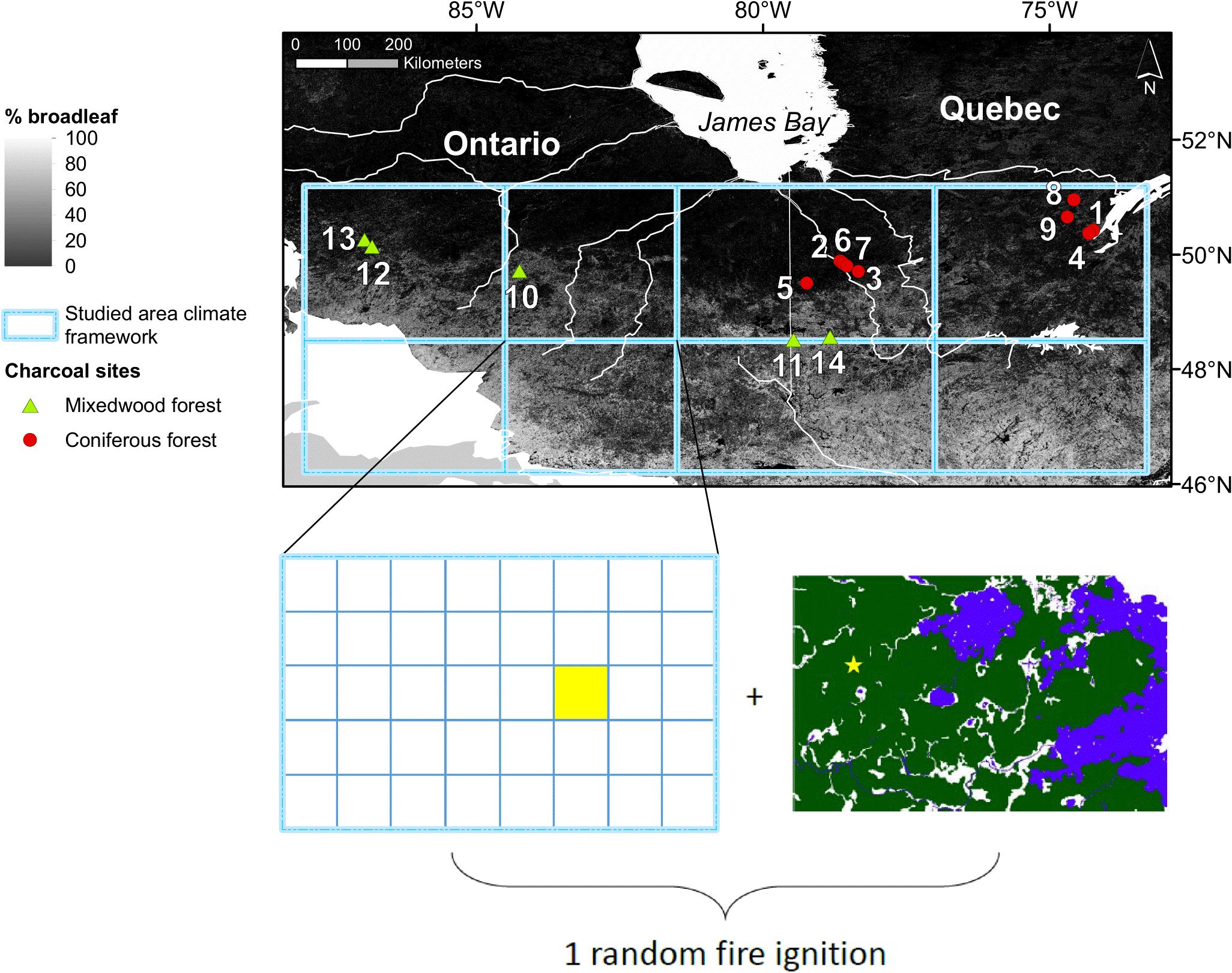
Figure 1. Location of the eastern Canadian study region and its characteristics in terms of sampled lakes (triangles and circles), percentage of broadleaf tree cover [background map from Natural Resource Canada (2008)], and the climate framework (blue rectangle) used to extract Holocene climate snapshot datasets simulated by the HadCM3 climate model (Valdes et al., 2017) over the last seven millennia BP. Five lakes (green triangles) are located in the mixedwood boreal forest, south of the modern transition zone, while nine (red circles) are in the coniferous boreal forest, north of the transition zone (see Supplementary Table S1 and Supplementary Material S1 for lake and regional characteristics, respectively). We used the 48.5°N parallel (i.e., the boundary pixel nearest to 49°N from the HadCM3 climate model) in association with three meridians (77°W, 81.5°W, and 84.5°W) to split the study area (46–51°N, 73–88°W) into eight subregion replicates (blue rectangles). In each subregion, for each Holocene millennium and each forest type present in the region at that time (see Figure 2), we simulated a set of 120 fires, with 20 fires per month from April to October. Each fire simulated by PFAS had a random starting date in the given month, a random projection time-length [i.e., computed from the potential length remaining from ignition date to fire-season end (October 31)], and a double random ignition point location: one within the subregion (yellow 0.5°-pixel providing climatic inputs) and one for the ignition location (yellow star) within the 11,000-ha landscape mosaic.
Observed Fire History
Holocene Fire History Reconstructions From Lacustrine Charcoals
Charcoal samples from fourteen previously published lacustrine cores (Figure 1 and Supplementary Table S1) were used to reconstruct three fire metrics in each forest type: (i) the regional burned biomass [RegBB; Power et al. (2008); Blarquez et al. (2015a)]; (ii) the regional fire frequency [RegFF; Remy et al. (2017)]; and (iii) the fire size index [FS-index; defined as the RegFF/RegBB ratio (Ali et al., 2012)]. The computation of these fire metrics benefited from recent advances in time-series analyses and statistical charcoal treatments (see Supplementary Material S2 and Supplementary Figures S1,S2, for details about standard charcoal treatments and recent advances). RegBB provides a continuous record of the time-dependent evolution of the rate of charcoal accumulation in lacustrine cores for a given forest type. It provides an indication of the average amount of charcoal production (i.e., estimated burned biomass) within landscapes. Studies using charcoals extracted from boreal forest lakes have established that burned biomass, represented by charcoal accumulation rates, correlates well with the area burned and fire severity estimated from remote sensing images (Kelly et al., 2013; Hennebelle et al., 2020), stand-replacing fire maps (Ali et al., 2012) and fire-scar records (Higuera et al., 2011). In our study region, the functional form between burn rate estimates (equivalent to area burned) and RegBB since 1770 CE is close to linear (Ali et al., 2012), and the Spearman rank correlation between the two series is 0.73, with a 95% bootstrap confidence interval (BCI; 0.30, 0.89). RegFF is computed from the number of significant charcoal peaks per unit of time recorded in lacustrine cores, and is thus an indicator of the typical fire frequency within a landscape. Finally, as in Ali et al. (2012), we used the ratio between RegBB and RegFF (the FS-index) to characterize the temporal changes in estimated fire size at a regional scale. FS-index values <1 would be indicative of a lower mean burned biomass per fire as a result of smaller fire sizes, and vice versa (Ali et al., 2012). While Hennebelle et al. (2020) showed that in northern CF, encompassing closed- and open-canopy black spruce forests, charcoal accumulation in traps was related to both fire size and fire severity, to our knowledge no such relationship information exists for boreal MF. Moreover, the fire model used in the present study did not include information about fire severity. Therefore, changes in FS-index values were interpreted as fire size changes and compared with simulated fire size outputs from the fire propagation model (see below).
Characteristics of Recent Fires From the Quebec Fire Agency Database
The lightning-ignited fire database from the Société de Protection des forêts contre le feu (SOPFEU, F. Lefebvre, pers. comm.; period 1994–2008) was used to set reference conditions for the simulation work. Based on the Quebec stand-fuel map (Pelletier et al., 2009) and the SOPFEU database, fires in the boreal CF include those ignited in fuel stand types C1 (lichen black spruce stands), C2 (feather moss black spruce stands) and C3 (jack pine stands), while fires in the MF include those ignited in fuel stand types D (deciduous stands) and M1–M4 [healthy mixed stands with spring (M1) and summer (M2) phenology, and spruce budworm outbreak-impacted stands with spring (M3) and summer (M4) phenology]. The number of fires, mean fire size and seasonal fire proportions were computed for each forest type and three fire classes (Table 1). The first fire class included all recorded ignitions, with fires potentially stopped before spreading. The second class only included fires larger than 1 ha, which were assumed to have resulted from efficient propagation. The third class included the same fires as those in the second class, but the largest fires were constrained to 11,000-ha, which was the size of the landscape mosaic used within our methodological approach (see section “Forested Landscape Settings Over the Last 7,000 Years”). The 95% BCI for the mean fire sizes was computed separately for each fire class and each forest type using the R Boot library (Canty and Ripley, 2016), with a resampling procedure and 10,000 iterations.
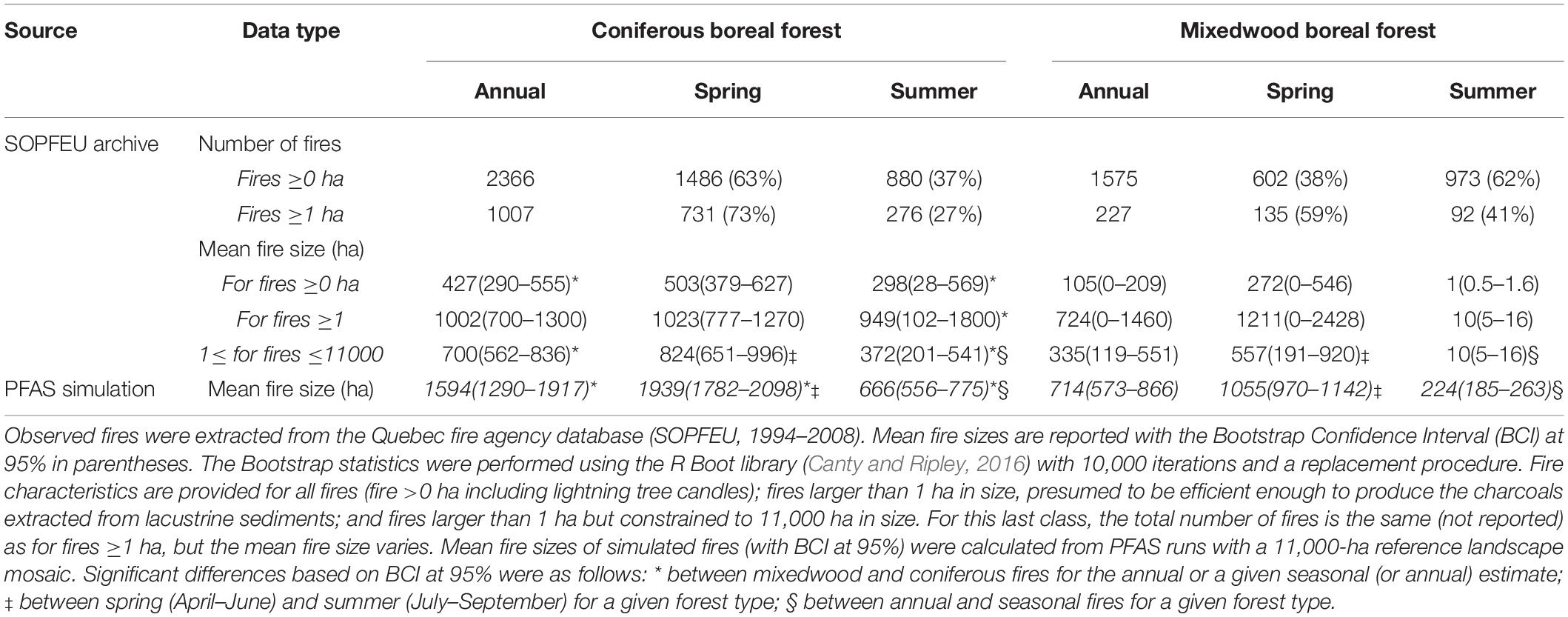
Table 1. Observed vs. simulated annual and seasonal characteristics of fires in the boreal coniferous and mixedwood forests.
The Probabilistic Fire Analysis System and Its Settings for the Holocene
Holocene fire sizes in each forest were simulated using the Probabilistic Fire Analysis System (hereafter PFAS; Anderson, 2010), which is based on the Canadian Forest Fire Behavior Prediction System (Wotton et al., 2009) and has been applied previously to different Canadian regions (Anderson, 2010; Hély et al., 2010a; Ouarmim et al., 2016). PFAS computes the probable fire extent over a spatially explicit landscape from the probabilities of fire spread and fire extinction, which are based on elliptical fire growth in eight compass directions, a 30-year time-series of fire weather normal, and Duff Moisture Code (DMC) computations. The default DMC value of 20 units in PFAS was retained and used as the humidity threshold value beyond which the fire could no longer spread (Anderson, 2010).
Forested Landscape Settings Over the Last 7,000 Years
The 11,000-ha natural landscape of Lake Duparquet (Harvey, 1999) in the Clay Belt region (Figure 1) was selected as a temporally static landscape reference map in terms of topography (aspect, elevation) and water body locations. Based on pollen information (Carcaillet et al., 2001), past aboveground tree biomass estimated from a network of pollen records (Blarquez et al., 2015a), and previously published maps of forest composition mosaics (Hély et al., 2010a), Holocene landscape mosaic scenarios were built for CF (i.e., with a 100% black spruce mosaic throughout the last 7,000 years) and MF (i.e., a 70% broadleaf dominance for 8,000–2,000 BP declining to only 30% for 1,000–0 BP, Figure 2). Note that present-day broadleaf stands cover 37% of the Duparquet Lake natural mosaic (Hély et al., 2010a), which is very close to the scenario used here for the most recent period (1,000–0 BP). Using the Canadian forest fire behavior prediction system (van Wagner, 1987; Wotton et al., 2009), each selected mosaic scenario was transformed into a fuel stand mosaic and then rasterized at a scale of 625 m2 per grid cell (25 m × 25 m).
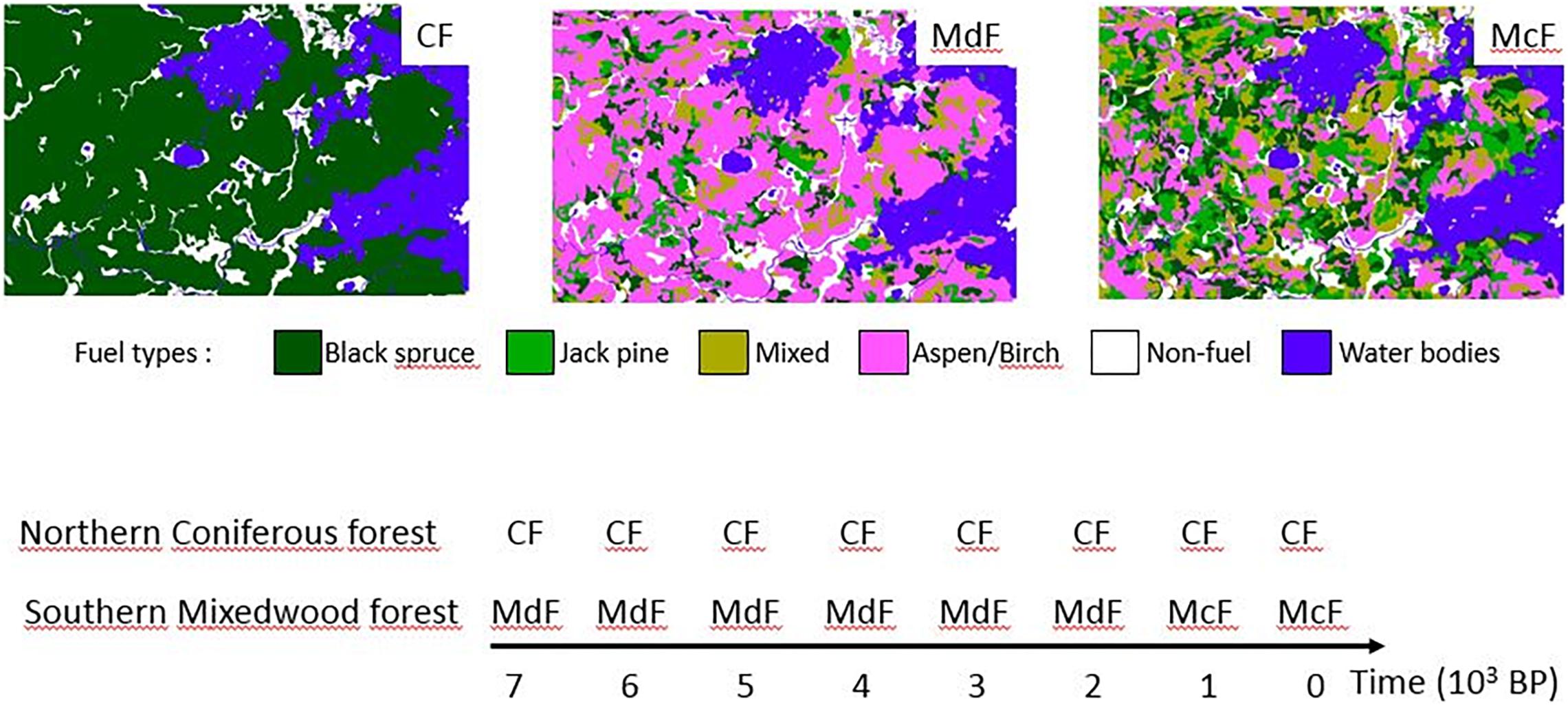
Figure 2. Forest composition scenarios used to run the PFAS fire model (top) and schematic vegetation changes over time (bottom). The boreal coniferous forest (CF) landscape is representative of the northern black spruce forest, with a stable composition since the end of the last deglaciation. The boreal mixedwood forest landscape, dominated by broadleaf deciduous species (MdF), either as pure deciduous stands or as mixed stands in which deciduous species dominate (i.e., a total deciduous percentage of 70%), is representative of the southern part of the study region during most of the Holocene (i.e., 8,000–2,000 years BP). The boreal mixed forest landscape, dominated by coniferous species (McF), either as pure coniferous stands or as mixed stands in which the coniferous species dominated (70%), is representative of the late Holocene (1,500–0 years BP), when it replaced MdF. Such a compositional shift (from MdF to McF) arose from the Neoglacial period that started around 3,000 years BP, reflecting the vegetation response to cooler and moister conditions than those prevailing earlier in the Holocene. Local and regional pollen analyses (Carcaillet et al., 2001; Blarquez et al., 2015a) show that this was a two-step response, with a decrease in deciduous species abundance that in turn provided space for coniferous abundance to increase. Both MdF and McF represent the boreal mixed forest (MF) for comparison with CF.
Climate Datasets
Monthly mean temperature and precipitation at each millennium changeover for the last 7,000 years (i.e., at 7K, 6K, …, 1K BP, respectively) were obtained from the HadCM3BL-M1 climate model (Valdes et al., 2017). Anomalies relative to the pre-industrial period (0 BP equates to AD 1750) were calculated, then downscaled to a 0.5°-spatial resolution (Supplementary Material S3) and applied to the Climate Research Unit climatology dataset TS 2.1 (Mitchell and Jones, 2005). Thirty-year long monthly time-series were then computed based on statistical distributions [Normal and Gamma distributions (New et al., 2002) for temperature and precipitation, respectively] and transformed into daily times-series, using the Richardson (1981) weather generator (Supplementary Material S3) as PFAS inputs. Each millennium changeover time-series was also used independently to compute daily values for the Drought Code (DC) (i.e., moisture content of the deepest humus layer, van Wagner, 1987), and then to assess fire-season length based on the 80-unit DC threshold (Hély et al., 2010b) and its deviation (i.e., spring and summer days to be added or removed) compared with the present-day reference fire-season length (Supplementary Material S4 and Supplementary Figure S3). The difference in spring (summer) fire-season length for each millennium was used to calculate proportionally the number of spring (summer) fires to be randomly selected within that millennium’s simulated fire dataset, fires from both seasons building a representative annual data set per millennium (see section “Statistical Analyses of Fire Sizes”).
PFAS Simulation Settings and Outputs
The study area was subdivided into eight subregions (Figure 1), considered as replicates in terms of climatic conditions (Bergeron et al., 2004). A set of 120 fires (20 fires per month from April to September) was simulated by PFAS in each subregion for each millennium and each forest type, with its prescribed vegetation composition (Figure 2). Each fire simulation was based on a random starting date, a random projected time-length, and a double random ignition point location (Figure 1). The final fire size for each simulated fire was the 80–100% probable extent class from PFAS, as suggested for CF in a previous study of the region and assumed to be valid for MF (Hély et al., 2010a). This fire extent class was used for MF and CF to validate our approach by comparing the 0 BP-simulated fire sizes with present-day observed fires, and to reconstruct changes in simulated fire sizes over the last 7,000 years.
Statistical Analyses of Fire Sizes
To assess Holocene changes in annual and seasonal fire sizes between forest types and millennia, we compared the forest types for each millennium, and the fire size for each forest type over time. The total number of simulated fires for each millennium was adjusted based on its departure from the 0 BP referenced fire-season length (Supplementary Figure S3). Using the R Boot library (Canty and Ripley, 2016) with 10,000 iterations and a replacement procedure, we randomly selected a subset of 100 fires among the 960 simulated fires available for the 0 BP period for each forest type, respecting the present-day proportions of spring- (i.e., April, May, and June) and summer- (i.e., July, August, and September) observed ignitions from the SOPFEU database (fires >1 ha, Table 1). We then used the fire-season length departures in spring and summer for each millennium over the 7,000–1,000 BP period (Supplementary Figure S3) to adjust the seasonal number of fires to be added or subtracted to the theoretical number of 100 fires to be representative for each millennium. This procedure was applied 10,000 times for each millennium fire dataset, and the mean and 95% BCI for spring, summer and annual (i.e., combining spring and summer) fire size distributions for each Holocene millennium calculated. As fire sizes are not normally distributed, we also performed a series of ANOVAs on ranked fire sizes (Conover and Iman, 1981) to analyze the variance partitioning between mosaic composition and climate as well as the likely interaction between these two factors. Finally, in order to allow a comparison of fire size estimates between forest types and between methods, we transformed the FS-index and simulated fire size values into z-scores. For each method, we calculated the mean and standard deviation from the Holocene dataset for the two forest types.
Results
Holocene Fire Activity Reconstructions From Fossil Charcoals
The RegBB reconstructions showed significantly higher charcoal production from fires in CF than in MF over the last 5,500 years (on average 2.4 times more, Figure 3A). The CF RegBB increased over the 7,000–4,000 BP period before reaching a stable state between 4,000 and 2,000 BP, and then declined during the next 2,000 years to values similar to those of 6,000 BP. The RegBB was marked by very high variability during the first 2,000 years, probably as a result of the post-deglaciation afforestation that took place differentially in CF around the studied lakes. The MF RegBB increased over the 7,000–2,000 BP period before reaching a plateau with slight oscillations during the last 2,000 years.
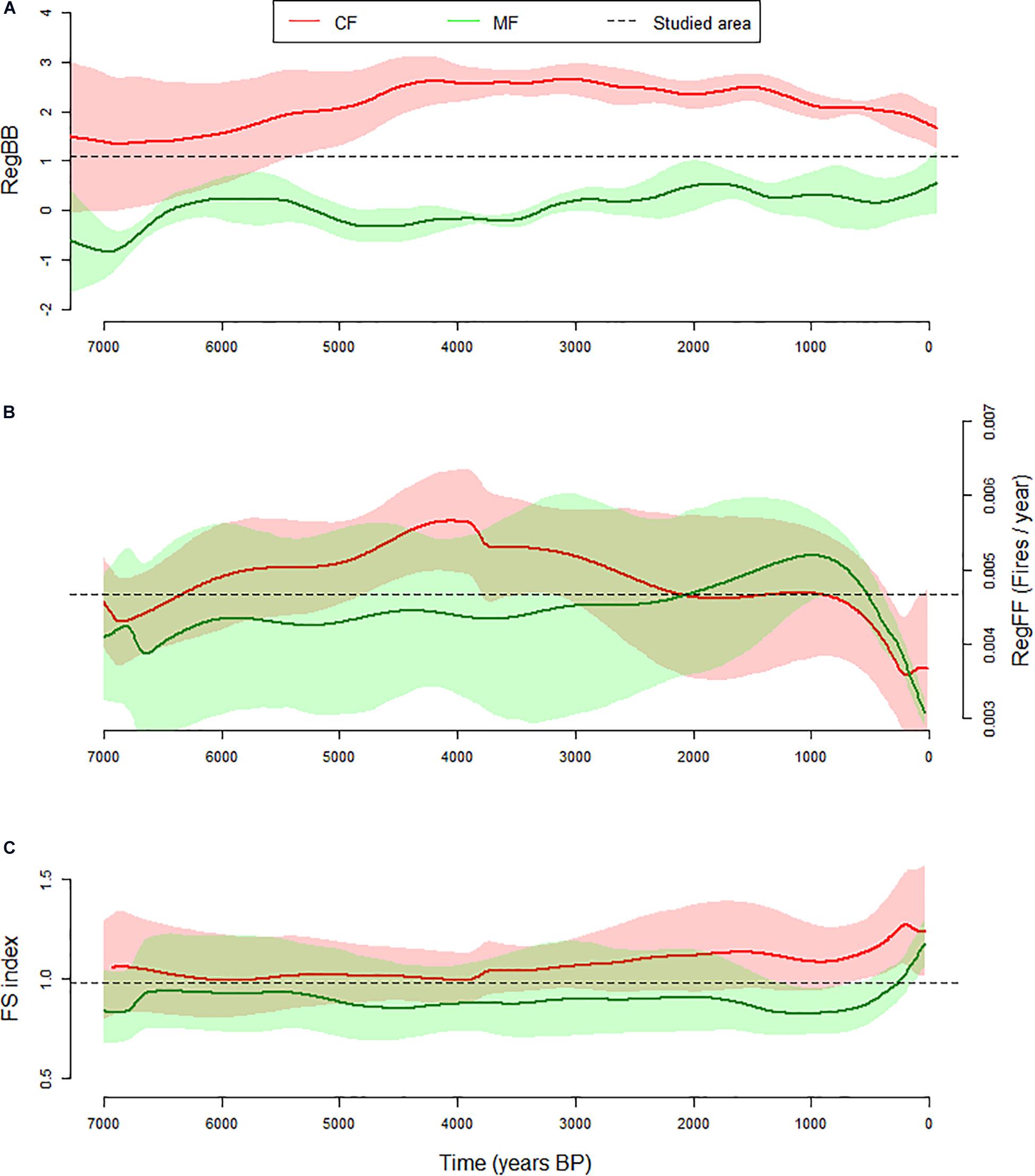
Figure 3. Reconstructed Holocene fire activity changes in coniferous (CF in red) and mixedwood (MF in green) boreal forests based on lacustrine charcoals. Regional biomass burning (RegBB, A) is unitless and refers to the average total charcoal biomass accumulated in sediments, while regional fire frequency (RegFF, B) refers to the number of fires estimated per year using the CharAnalysis pre-treatment peak signal (Higuera et al., 2007). The Fire Size index (FS, C) is the RegBB/RegFF ratio, which is also unitless and captures differences in the charcoal signal for periods when fires were frequent but rather small (FS < 1) versus rare but rather large (FS > 1, Ali et al., 2012). In each panel, the overall regional signal including all lacustrine cores from both forest types is indicated by a dashed line, and for each forest type the envelop represents the 95% Bootstrap Confidence Intervals (BCI) computed using the 2.5% and 97.5% percentiles.
The RegFF reconstructions showed no significant difference between CF and MF because of the high variability among lakes within each forest type (Figure 3B). The CF RegFF increased over the 7,000–4,000 BP period, followed by a decrease until present-day, reaching an unprecedented Holocene low mean value. The MF RegFF showed the same general trend, but with a decrease that started later during the Holocene, around 1,000 BP. The CF RegFF variability was smaller from 7,000 to 3,000 BP compared with the last 2,000 years, whereas the MF RegFF variability was higher from 7,000 to 2,000 BP compared with the last 1,000 years.
The regional fire size (FS-index) reconstructions showed similar and superimposed trends overall between the forest types throughout the Holocene as a result of very high variability (Figure 3C): a relatively stable mean FS-index for the 7,000–2,000 BP period, followed by a slight decrease for a few centuries before increasing again up to 0 BP, the increase being steeper for MF than for CF. Taking just the means into account, there was more contrast over time between the two forest types (Supplementary Figure S4): the FS-index increased in CF over the 6,000–2,000 BP period, followed by a continuous and steeper slope over the 4,000–2,000 BP period, but it was more variable for MF, with a lower increasing slope over the 4,000–2,000 BP period, and an earlier start to the decrease. The FS-index values were always lower on average, and displayed less variability, for MF than CF (Figure 3C). The FS-index for the last millennium and few centuries, for CF and MF, respectively, reached unprecedented Holocene high mean values (Figure 3C and Supplementary Figure S4). Differences between the forest types and over time were also evident when the FS-index values were transformed into z-scores (See Supplementary Figure S5, top left panel).
Holocene Fire Sizes Simulated by the PFAS Model
Mean annual fire sizes (i.e., combining spring and summer fires) simulated in the CF landscape mosaic were two to seven times (five times in average) larger than those simulated in MF during the Holocene (Figure 4). For both forest types, simulated spring fires resulted in larger fire sizes than simulated summer fires, and spring fire sizes were more representative of the average annual fire size and Holocene trends than the summer fire sizes. Annual and spring fires simulated in CF were relatively stable (Figure 4), with the largest sizes occurring during the 7,000–6,000 BP period and at 1,000 BP, and the smallest sizes occurring at 5,000 BP. The trend for CF summer fire sizes was slightly different, with the largest sizes occurring at 6,000 BP and the present-day, and the smallest sizes occurring at both 7,000 and 4,000 BP. CF composition did not change throughout the Holocene (Figure 2) and therefore changes in fire sizes were a direct result of the prescribed climate changes. CF spring, summer and annual fire size averages, computed over the 7,000 years, were representative of all the CF fires (i.e., same order of magnitude), in contrast to those computed for the MF fires (Figure 4).
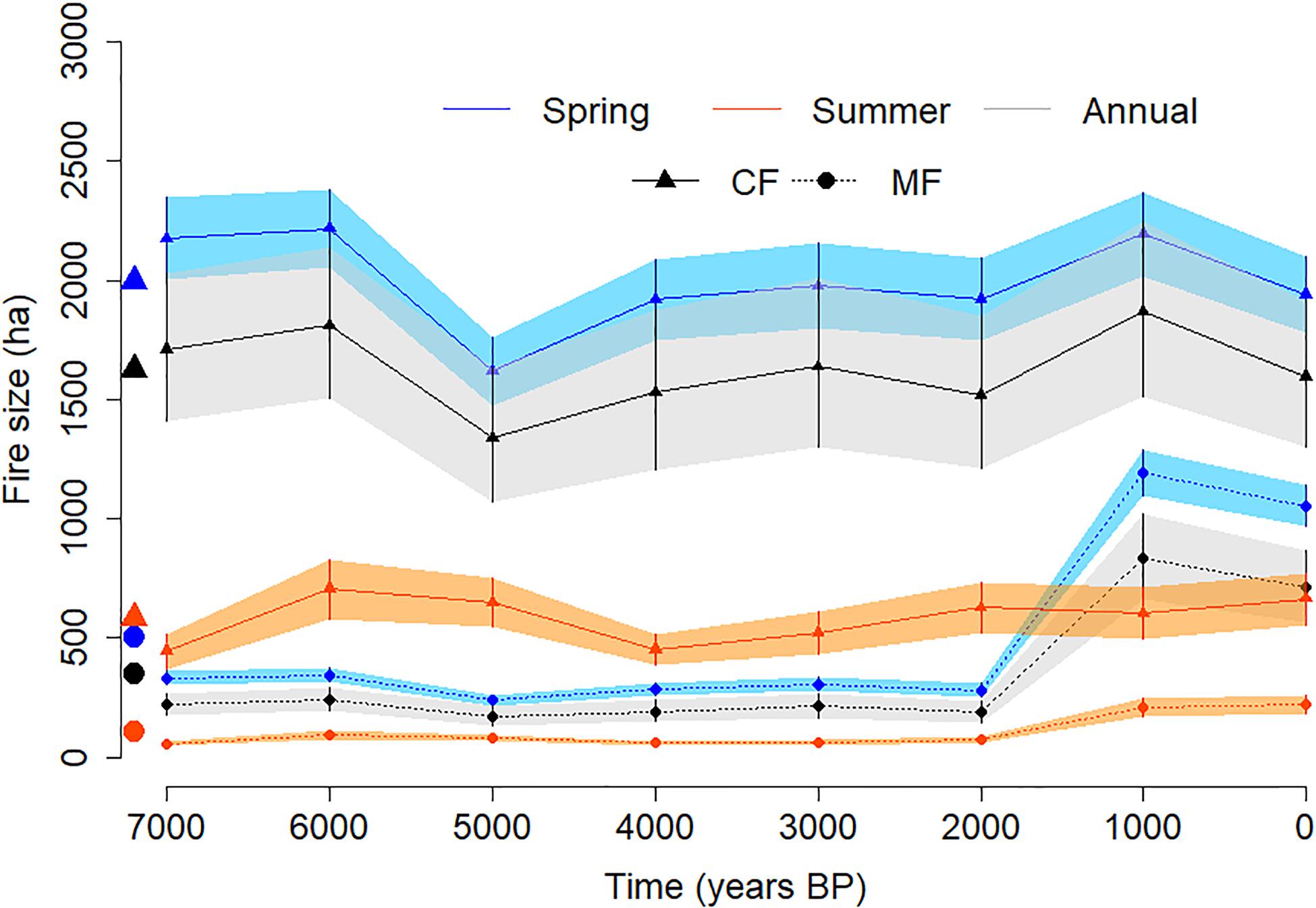
Figure 4. Seasonal and annual fire sizes simulated over the last 7000 years for the coniferous and mixedwood boreal forests (CF and MF, respectively) of eastern Canada using the PFAS fire model. Colors refer to fire seasons: spring (April–June) in blue, summer (July–September) in orange, and annual (April–September) in gray. Symbols associated with error bars [2.5% and 97.5% Bootstrap Confidence Intervals (BCI)] refer to snapshot simulation times (centered on each millennium changeover), while the largest symbols near the y-axis represent mean simulated seasonal or annual Holocene fire sizes, respectively, computed over all millennia.
Simulated fire sizes in the MF showed the same general trends as CF from 7,000 to 2,000 years BP (Figure 4). However, the last two millennium changeovers (at 1,000 and 0 years cal. BP) highlighted a combined effect, resulting from both climate change (as for CF) and vegetation composition change (i.e., an increase in the proportion of conifers, Figure 2), that significantly enhanced fire propagation and created larger fires (three to four times larger) than earlier changeovers in the Holocene. Using the one-way ANOVA results for the Holocene (See Supplementary Material S5 and Supplementary Figures S6,S7) and the two-way ANOVA for the restricted 2,000 and 1,000 years BP (Figure 5), we characterized and quantified the additive effect of mosaic change over climate change. The results of the bootstrap ANOVA procedure (see Supplementary Material S5 Series3) showed that the Mosaic factor was significant in all ANOVAs (100%), while the Climate factor was significant in 55% of the ANOVAs and their interaction in only 2% of the ANOVAs. For ANOVAs of Series3, in which both Climate and Mosaic were significant (Figure 5, top and middle panels), the Climate factor explained between 5 and 50% of the fire size variance, while the Mosaic factor explained between 45 and 94%. As a consequence, while within factor differences were significant, the difference in fire size between Mosaic levels was significantly more important than that resulting from Climate levels (Figure 5, middle panel). When the interaction was significant, it explained between 7 and 17% of the fire size variance, with the Climate factor explaining between 1 and 33%, and the Mosaic factor between 56 and 88%. Both factors revealed the same trend without the interaction. The TukeyHSD results for factor interaction (Figure 5, bottom panel) indicated that, among the six pairs available, the strongest difference in fire size between the two changeovers involved the change from 2,000 years BP in the MdF mosaic to 1,000 years BP in the McF mosaic, refering to the temporal change recorded by pollen assemblages. Throughout the Holocene, the composition of the MF only changed once (from 2,000 to 1,000 years BP); apart from that transition period, most of the changes in simulated fire sizes seen in Figure 4 and tested for both forest types were a result of climate change (see Supplementary Material S5 Series4 and Supplementary Figure S8 for CF).
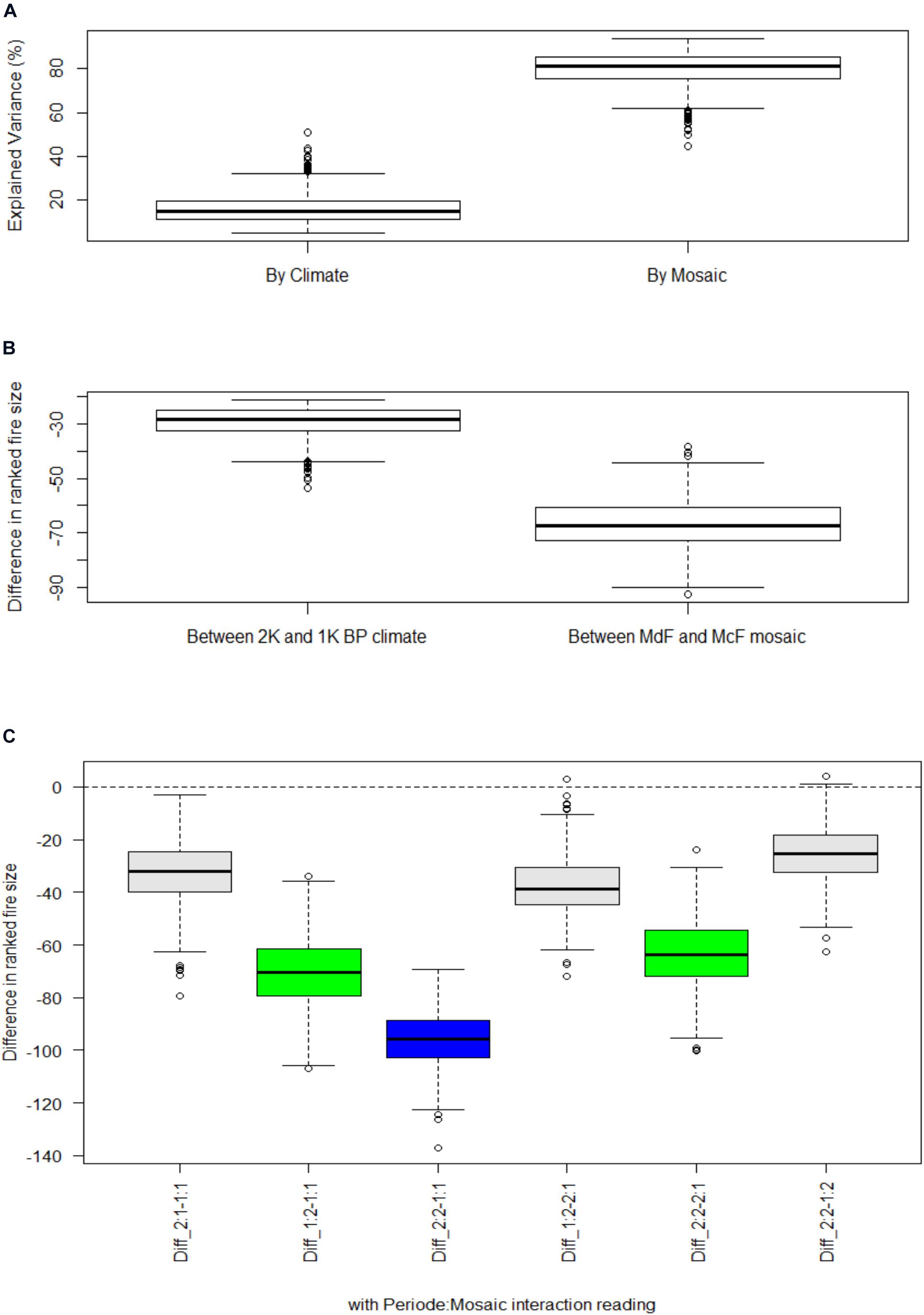
Figure 5. Results of the significant two-way ANOVA encompassing the 2K–1K BP millennium changeovers. (A,B) refer to the 55% of ANOVAs for which both Climate and Mosaic had significant (additive) effects, with the variance partition between factors (A) and the differences in fire sizes between levels of a given factor (TukeyHSD test, B). For the few ANOVAs in which the Climate:Mosaic interaction was significant (C), McF is coded 1 and MdF is coded 2. Among the six pairs of differences in fire sizes analyzed using the TukeyHSD test, the most significant and important difference was found for the change from the 2K BP MdF mosaic to the 1K BP McF mosaic (blue boxplot), which represented palynological changes. The two green boxplots are representative of differences found in the first two series (see Supplementary Figures S6,S7), but only for 2K–1K BP differences.
Based on these statistical analyses, mean fire sizes simulated in MF over the last two millennium changeovers (1,000 and 0 BP) were significantly higher than the previous millennium changeovers, and higher than the average size computed for the Holocene. This result was replicated when fire size datasets were transformed into z-scores (Supplementary Figure S5, bottom left panel), suggesting an important change in the fire-vegetation-climate interaction.
Comparison Between Present-Day Archived and Simulated Fires
Except for summer fires in MF, present-day fire sizes simulated by PFAS were systematically about twice as large as the observed fires in each forest type (Table 1). Despite this, we felt the reconstructed Holocene trends could be analyzed and compared between the forest types because the overestimation factor was constant whatever the forest type or the fire season, and because significant differences between seasons or between seasonal and annual observed fire sizes were preserved in the simulated datasets for both CF and MF.
Over the 1994–2008 period, the SOPFEU agency has observed 50% more fires (all sizes included) in CF than in MF (Table 1), and CF has mainly been subject to spring fires whereas MF has been subject to summer fires. By restricting the analysis to efficient fires (i.e., size >1 ha), both forest types showed the same seasonal pattern (i.e., more numerous fires in spring than in summer) but diverged more in terms of overall number of fires. CF had almost 350% more fires than MF. In terms of fire size, CF was subject to larger fires than MF at both annual and summer scales for most fire size datasets (Table 1). When the SOPFEU’s largest fire sizes were constrained to 11,000 ha for comparison with the simulations, spring fires were significantly larger, twice and fifty times, respectively, than summer fires in CF and MF. Therefore, consistent with the PFAS simulation results, spring fire sizes were more representative of annual fire sizes than summer fire sizes.
Discussion
MF Versus CF Fire Size Hypothesis Confirmed
The charcoal records indicated that MF had significantly lower burned biomass (RegBB) than CF throughout the Holocene. This, combined with similar regFF values for both forest types, systematically resulted in a lower FS-index for MF than for CF. In parallel, and independently from fire reconstructions based on charcoal, the PFAS simulations indicated that fires in MF were always smaller in size than those in CF throughout the Holocene, confirming that, until the present-day, regardless of climate change, MF has been less prone than CF to fires propagated over large areas, even at the last two millennium changeovers, when its needleleaf evergreen species proportion significantly increased (Carcaillet et al., 2001, 2010). Both reconstructed and simulated fire sizes also showed that the differences observed today between MF and CF are a legacy dating back to earlier in the Holocene, when conditions were more contrasted between the two forest types. We used z-scores for the primary purpose of comparing Holocene fires in the two forest types using both lake charcoal and fire simulations (Supplementary Figure S5, left panels). The similar general trend found for MF (except for 1,000 years BP), although amplified for charcoal, shows potential for paleo-ecological research dedicated to fire size. However, the greater disparity between z-score trends for CF raises more questions. This disparity could have several origins, among which the moving 500-year time window size and the methodological choice for treating end-effects in paleo-fire time-series are the most likely. Nonetheless, differences in terms of estimated biomass burning or fire size have gradually subsided between the forest types throughout the Holocene, particularly over the last 2,000 years (Figures 3,4). The present study data therefore confirms all the tested hypotheses and that of Bergeron et al. (2004) related to fire size differences between the forest types.
Beyond the Uncertainties, the Importance of Spring Fires
By using a spatially explicit fire model originally designed for applied research in fire management (Anderson, 2010), this study has shown that spring fires have always been larger in size than summer fires for both forest types. This was confirmed by the analysis of all fires larger than 1 ha in the lightning-fire database. Many studies deliberately focus on fires larger than 200 ha (e.g., Amiro et al., 2001; Girardin et al., 2013a), as these fires represent 3% of fire occurrences but are responsible for 97% of the total annual area burned (Stocks et al., 2003). We recognize the importance of these larger fires and have previously attempted to decipher larger from smaller fires based on charcoal records (Hély et al., 2010b). However, there is a priori no possibility of knowing whether peaks in charcoal records from lacustrine sediments are related to fires larger or smaller than 200 ha, nor of assessing real fire sizes through FS-index computation. At best, it has been shown that the quantity and size of charcoal particles are representative of the proximity of fires to the studied lakes (Brossier et al., 2014; Oris et al., 2014). However, uncertainties remain, as experiments conducted over recent years point to significant relationships between the quantity of charcoal trapped annually on lake surfaces and the fire severity or areas with fires located up to 30-kms from the lakes (Oris et al., 2014; Hennebelle et al., 2020). Except in rare cases of annually laminated lake sediments, the time resolution of sediment samples is usually longer than 10–15 years. Thus, the annually resolved relationships identified by Hennebelle et al. (2020) are not directly applicable to long sediment cores and would need to be adapted in a sedimentary context to estimate the same metrics (fire severity and distance to fire) accurately. Based on all these uncertainties, we chose to compute the number and mean size of fires from areas larger than 1 ha, as we conservatively assumed that such fires would be large enough to produce charcoals that would be transported to the vicinity of the lakes. The importance of spring in relation to the total annual burned area, as highlighted by our simulations, is consistent with statistics from the last few decades, showing that the years with the largest burned areas recorded very large spring fires (Ali et al., 2012). Using the 10-ha fire size threshold to compute the lightning-fire database statistics would have resulted in the same seasonal distribution of fire ignitions and mean fire size (not shown) as the 1-ha minimum threshold.
Climate-Vegetation-Fire Interplay
The trends in simulated fire sizes for both forest types were similar to those of change in fire-season length reconstructed from climate model simulations, and particularly to those of spring onset departure rather than summer termination departure. This confirms that, regionally, annual fire size is positively related to earlier fire-season onset induced by high spring radiative insolation, which has been driving warmer spring conditions in the region since before ca. 4,000 BP (Hély et al., 2010b). Present-day fire-season onsets that are earlier and warmer than normal are usually responsible for large fire years as a result of dry fuel conditions and greater fuel availability coinciding with earlier seasonal ice thaw (Westerling et al., 2006; Ali et al., 2012). Cooler and wetter overall annual climatic conditions compared with those before 3,000 BP are therefore less favorable to fire ignition and fire propagation, partly explaining the straightforward decreasing trends in both RegFF and RegBB records for CF. Conversely, these climatic conditions, which are more unfavorable for broadleaf species, first led to a decrease in broadleaf tree biomass in MF and then to an increase in needleleaf species and their proportion over the last two millennium changeovers (Carcaillet et al., 2001; Blarquez et al., 2015a). This compositional change in MF compensated for climatic conditions that were less prone to fires. The MF humus, because of the absence of leaves on the deciduous trees in the spring, was drying out more quickly than in CF. Moreover, with the increase in conifer abundance (Blarquez et al., 2015a), fuel within MF became more flammable than previously and fire probably spread easily through the better quality fuel available (Hély et al., 2000, 2001). This interplay between spring climate and vegetation composition explains why MF recorded both rather stable RegBB and slightly higher RegFF than CF over the last 3,000 years, advancing our knowledge compared with previous reconstructions for MF (Carcaillet et al., 2001; Girardin et al., 2013a; Blarquez et al., 2015a).
PFAS: A Valuable Fire Model for Insights Into the Paleo-Ecological Climate-Vegetation-Fire Interplay
The good agreement between Holocene trends in reconstructed and simulated fire sizes confirms the accuracy and effectiveness of PFAS for providing insights into past fire patterns and the climate-vegetation-fire interplay. More importantly, by using PFAS we have revealed and confirmed three key processes operating within the boreal forest: (i) the importance of spring fires in shaping the mean annual fire size in CF and MF over the last 7,000 years BP, which previously had only been suggested for CF, based on fire archives and June temperature records for the last few decades (Ali et al., 2012); (ii) the positive feedback between the increase in fire-prone species abundance in MF over the 1,000–0 BP period and the increase in both spring and annual fire size trends, which had been suggested previously only in terms of larger fire size occurrences (Girardin et al., 2013a; Terrier et al., 2013); and (iii) the effect of fire-season length shortening (Supplementary Figure S3), induced by less fire-prone climatic conditions, which has been offset by vegetation change toward a more fire-prone composition for MF. Termination earlier in the summer does not affect spring conditions known to be more favorable to fires in MF (Wotton et al., 2009). While Girardin et al. (2013a) suggested a positive feedback between MF vegetation composition and fire frequency, we have confirmed it for all three fire regime variables studied, namely RegBB, RegFF, and fire size (FS-index).
As most paleo-ecological reconstructions from lacustrine sediments have a multi-year resolution, it is impossible to know the fire number for any given sediment sample or significant charcoal peak. In contrast, PFAS can simulate individual fires up to a daily resolution. The assumption of a linear relationship between past fire-season length and proportion of seasonal fires is strong, but there is currently no means available to justify a more realistic pattern. By using a large number of simulations over several fire seasons representative of a given period (equivalent to “fire normals”), a distinction between spring and summer fires is statistically possible within an assessment of annual fire size distribution and change over time. Such a seasonal resolution can be obtained from tree-ring-based fire-scar studies, where it is possible to record the position of each scar within its annual ring. However, this approach is constrained by both tree longevities and decay rates, because fire scars are commonly collected from trees that are already dead, and therefore the time frame represented is usually only a few centuries (Pitkänen et al., 1999; Girardin et al., 2006; Le Goff et al., 2008).
The Last 1,000 Years: Likely a Mix of Signal Treatment Limits, Global Change, and Human Activity
The increase in FS-index over the last millennium in both forest types could indicate an overall regional influence, such as climate change. However, several interactions create sufficient uncertainties that prevent a straightforward conclusion. The first issue arises from potential changes in boreal forest equilibrium induced by anthropogenic activities over the last few centuries (Bergeron et al., 2004). The use of a multi-century moving window in charcoal metrics and statistical analyses (Higuera et al., 2010; Kelly et al., 2013; Blarquez et al., 2015b) spreads the footprint of European colonization over at least the last millennium for our charcoal analyses (indicated by both continuous trends and z-score box plots over time), and probably introduces some discrepancies. Intensive European colonization associated with large clearance burnings for the transcontinental railroad first occurred in MF in 1910–1920, and was followed by forestry and agricultural activities (Bergeron et al., 2004). While these activities created a lot of charcoal that was sequestered in the lacustrine sediments over a very short period of time, they may also have induced a regional landscape fragmentation that was less favorable for efficient fires (Niklasson and Granström, 2000; Bowman et al., 2011; Blarquez et al., 2018). However, such fragmentation was not included in the reference mosaic used with PFAS, as that mosaic refers to a protected landscape with natural dynamics (Harvey, 1999). Therefore, lakes within MF may have recorded several fires that spread across natural and unfragmented landscapes, producing high biomass burning and inducing a higher FS-index than expected from the managed surroundings. PFAS simulations performed for the same period also resulted in larger fire sizes in MF than earlier in the Holocene, as a result of warmer springs with earlier fire-season onset and higher coniferous abundance than in previous millennia, but still with well-connected forest stands because of the protected natural mosaic of Lake Duparquet. Moreover, a fire suppression strategy has always been more active in populated areas, which correlates with the MF in the south, compared with the northern CF, which is considered to have been virgin forest until the late 1970s (Bergeron et al., 2004). The fire suppression effect could also partly explain the smaller number of fires (all sizes and fires >1 ha) recorded in the lightning-fire database for MF than for CF. These trends, extracted from the 1994 to 2008 period, are the opposite of those reconstructed from older archives (i.e., 1945–1998 period, Bergeron et al., 2004), which may also indicate that current global change (climate warming and human activities) has already started to change fire regime trends.
The MF represents a sensitive ecosystem in the context of global change as its distribution could shrink as a result of several factors, such as the northward migration of southern temperate forest species with enhanced growing seasons, and probably the counter-intuitive southward migration of coniferous species, although available values of migration rate are quite low and only represent a short instrumental period (Boisvert-Marsh et al., 2014). MF could also come under threat from the many insect pests that attack broadleaf and coniferous tree species over large areas (Gray, 2013), producing heavy dead fuel loads in a warming environment, which in turn could exacerbate several components of the fire regime.
Conclusion
This study has illustrated how a spatially explicit fire model, developed for present-day applications, can help paleo-ecological studies by providing independent process-based datasets for comparisons with reconstructions. This is the first regional reconstruction of paleo-fires illustrating Holocene changes for three fire regime metrics (fire frequency, biomass burning, and fire size) simultaneously for both coniferous and mixedwood boreal forests. Compared with the variability in fire metrics recorded for CF, the MF data has highlighted different periods with relatively high variability. The combined paleo-ecological analyses of fire (charcoal reconstructions and PFAS simulations) suggest that fires in the boreal MF have been significantly smaller than those in the boreal CF over the last 7,000 years. Warmer spring conditions combined with overall annually cooler and wetter conditions since 3,000 BP have favored less frequent but larger fires in both forest types, especially in the MF because of an increase in coniferous species abundance. Over the last century, climate warming and human activities may have significantly modified the distribution of fire size in MF, probably toward larger spring fires, therefore reducing the difference between the two forest types. Today the boreal MF is being reshaped by temperate forest species migrating northward, northern coniferous species migrating southward, and insect outbreaks attacking broadleaf and coniferous tree species over large areas, producing heavy dead fuels and modifying the fire hazards. The quantification of natural variability is important for current forest management because it provides the range, and probably the limits, of the natural variability each forest type can withstand. It should also allow projections of future fire regimes in IPCC scenarios to be placed within an historical context and used as comparanda. Further research could enable an assessment of the sustainability of forest resilience in the context of change in future fire regimes and forest management practices to be added to the methodological approach presented here.
Data Availability Statement
The datasets generated for this study are available on request to the corresponding author.
Author Contributions
CH conceived the ideas and performed the fire simulations. AA and CR organized the charcoal database. CH, EC, MG, and CR performed the statistical analyses. CH wrote the first draft of the manuscript with input and review from EC, MG, YB, and OB. All authors contributed to manuscript revisions, and read and approved the submitted version.
Funding
This research was funded by the Natural Sciences and Engineering Research Council of Canada (NSERC Strategic grant and Discovery Grant), the European FP7-PEOPLE IRSES NEWFORESTS program, the PREREAL program (Belmont Forum), and the Cold Forests GDRI program supported by the Centre National de la Recherche Scientifique (CNRS-INEE, France), the École Pratique des Hautes Études, and the University of Montpellier.
Conflict of Interest
The authors declare that the research was conducted in the absence of any commercial or financial relationships that could be construed as a potential conflict of interest.
Acknowledgments
We thank Prof. Paul Valdes from the University of Bristol for providing us with the HadCM3BL-M1 climate simulations for the Holocene. We also thank the SOPFEU for providing us with the lightning-ignited fire database. The paper has been language edited by Sees-editing Ltd.
Supplementary Material
The Supplementary Material for this article can be found online at: https://www.frontiersin.org/articles/10.3389/ffgc.2020.511901/full#supplementary-material
MATERIAL S1 | Biophysical and forest features of the study area, throughout the Holocene.
MATERIAL S2 | Fire reconstruction improvements, including charcoal peak detection and bootstrap confidence interval computation.
MATERIAL S3 | Holocene climate datasets.
MATERIAL S4 | Computation of the Canadian Drought Code index (DC) and fire-season length over the last 7,000 years.
MATERIAL S5 | ANOVA and variance partitioning analysis of the simulated forest fire sizes to test the effects of climatic and forest compositional changes.
FIGURE S1 | CharAnalysis output checks for all charcoal extracted from lakes located in the coniferous boreal forest. For each lake, (A) presents the charcoal influx, with associated charcoal background (mean noise in gray) and its confidence intervals (in red) from which significant charcoal peaks (crosses) were retrieved over time. Similarly, (B) reports the Signal-to-Noise Index (SNI) with its changes over time, to check when SNI values fall below the threshold value of 3 (dashed line), as suggested by Kelly et al. (2011), which would indicate that a fire frequency reconstruction had been overestimated as a result of false fire events. Over the last 1,000 years, SNI values lower than 3 only occurred temporarily for three of the nine lake records (Pessière, Twin, and Profond Lakes, respectively).
FIGURE S2 | CharAnalysis output checks for all charcoal extracted from lakes located in the mixedwood boreal forest. The same legend as in Supplementary Figure S1 applies here. Among these lakes, only one (Jack Pine Lake) showed SNI values lower than 3 over the last few centuries.
FIGURE S3 | Holocene changes in average fire-season length over the study region (46–51°N and 73–88°W from HadCM3BL-M1 pixels), encompassing the transition between the mixedwood and coniferous boreal forests in eastern Canada. Fire-season length as well as spring and summer anomalies (departures for onset and termination dates, respectively) compared with present-day (0 BP) values were computed following Hély et al. (2010b) for each Holocene millennium. Fire-season length and onset and termination dates were based on a 30-year time-series of the Drought Code index (DC) (van Wagner, 1987) reconstructed from HadCM3BL-M1 monthly climate simulation anomalies in temperature and precipitation applied to the 0.5°CRU climate normal dataset and combined with the Richardson (1981) weather generator (see Supplementary Material S3).
FIGURE S4 | Changes in average Fire Size index (FS-index) computed from lacustrine charcoal over the last 7,000 years in the mixedwood (green) and coniferous (red) boreal forests of western Quebec. The dashed black line represents the Holocene average FS-index for the entire study area encompassing both the mixedwood and coniferous boreal forests.
FIGURE S5 | Changes in fire size z-scores throughout the Holocene, computed using charcoal (top panel) or simulated fire sizes from the PFAS fire model (bottom panel) for the mixedwood (green) and coniferous (red) boreal forests of western Quebec. The dashed black line is provided to facilitate the comparison of the two types of forest for a given dataset, or between the two datasets (FS-index and PFAS simulations) for a given forest. For each dataset (i.e., charcoal FS-index or PFAS fire size), the z-score computations were based on the overall mean and standard deviation computed using both forest types simultaneously for the 7,000 years. For the FS-index, each millennium boxplot was calculated for a 500-year window. Except for 0 and 7,000 years BP, for which the window was set to other limits (0–500 for 0 BP, and 6,500–7,000 for 7,000 years BP), all windows encompassed 250 years before and 250 years after the millennium changeovers (e.g., for 3,000 years BP, z-scores for 2,750–3,250 years BP were used). In the case of PFAS fire sizes, as for the ANOVAs (see Supplementary Material S5), we computed the z-scores based on annual sets of fire size for each forest type, randomly selecting the fire sizes simulated for a given millennium but respecting the spring and summer numbers of fires to be added to create an annual set representative of that millennium based on its fire-season length (see main text section “Statistical Analyses of Fire Sizes” and Supplementary Figure S3). As the 500-year window for each millennium changeover in the charcoal dataset encompassed 50 values per forest type, the bootstrap procedure for the PFAS fire size dataset was also performed using 50 iterations for each forest type.
FIGURE S6 | Results of one-way ANOVAs (representing 30% of the 1,000-bootstrap repetitions) showing the significant effect of Climate on ranked fire sizes in the MdF mosaic (top left panel) and the related percentage variance explained by Climate (top right panel). Among the 28 pairs of millennia differences tested by TukeyHSD [on significant ANOVAs only (bottom panel)], blue box plots represent differences found to be significant (p-value < 0.05) most of the time, the null difference value, shown by the dotted blue line, being outside the whiskers of that given distribution.
FIGURE S7 | Results of one-way ANOVAs (representing 20% of the 1,000-bootstrap repetitions), showing the significant effect of Climate on ranked fire sizes in the McF mosaic (top left panel) and the related percentage variance explained by Climate (top right panel). Among the 28 pairs of millennia differences tested by TukeyHSD [on significant ANOVAs only (bottom panel)], green box plots represent differences found to be significant (p-value < 0.05) most of the time, the null difference value, shown by the dotted green line, being outside or near the extremity of the whiskers of that given distribution.
FIGURE S8 | Results of one-way ANOVAs (representing 17% of the 1,000-bootstrap repetitions), showing the significant effect of Climate on ranked fire sizes in the CF mosaic (top left panel) and the related percentage variance explained by Climate (top right panel). Among the 28 pairs of millennia differences tested by TukeyHSD [on significant ANOVAs only (bottom panel)], violet box plots represent differences found to be significant (p-value < 0.05) most of the time, the null difference value, shown by the dotted violet line, being outside or near the extremity of the whiskers of that given distribution.
TABLE S1 | Main characteristics of the lakes and surrounding environments studied within the two boreal forest types of eastern Canada. The dominant species were Abies balsamea (Ab), Alnus viridis (Av), Betula papyrifera (Bp), Larix laricina (Ll), Pinus banksiana (Pb), Picea glauca (Pg), Picea mariana (Pm), and Populus tremuloïdes (Pt); CA stands for Cultivated Areas.
References
Ali, A. A., Blarquez, O., Girardin, M. P., Hély, C., Tinquaut, F., El Guellab, A., et al. (2012). Control of the multimillennial wildfire size in boreal North America by spring climatic conditions. Proc. Natl. Acad. Sci. U.S.A. 109, 20966–20970.
Amiro, B. D., Todd, J. B., Wotton, B. M., Logan, K. A., Flannigan, M. D., Stocks, B. J., et al. (2001). Direct carbon emissions from Canadian forest fires, 1959-1999. Can. J. For. Res. 31, 512–525. doi: 10.1139/x00-197
Anderson, K. R. (2010). A climatologically based long-range fire growth model. Intern. J. Wildland Fire 19, 879–894. doi: 10.1071/WF09053
Belleau, A., Bergeron, Y., Leduc, A., Gauthier, S., and Fall, A. (2007). Using spatially explicit simulations to explore size distribution and spacing of regenerating areas produced by wildfires: recommendations for designing harvest agglomerations for the Canadian boreal forest. For. Chron. 83, 72–83. doi: 10.5558/tfc83072-1
Bergeron, Y., Gauthier, S., Flannigan, M. D., and Kafka, V. (2004). Fire regimes at the transition between mixedwood and coniferous boreal forest in northwestern Quebec. Ecology 85, 1916–1932. doi: 10.1890/02-0716
Bergeron, Y., Gauthier, S., Kafka, V., Lefort, P., and Lesieur, D. (2001). Natural fire frequency for the eastern Canadian boreal forest: consequences for sustainable forestry. Can. J. For. Res. 31, 384–391. doi: 10.1139/x00-178
Bergeron, Y., Leduc, A., Harvey, B. D., and Gauthier, S. (2002). Natural fire regime: a guide for sustainable management of the Canadian boreal forest. Silva Fennica 36, 81–95.
Blarquez, O., and Aleman, J. (2016). Tree biomass reconstruction shows no lag in post-glacial afforestation of eastern Canada. Can. J. For. Res. 46, 485–498. doi: 10.1139/cjfr-2015-0201
Blarquez, O., Ali, A. A., Girardin, M. P., Grondin, P., Fréchette, B., Bergeron, Y., et al. (2015a). Regional paleofire regimes affected by non-uniform climate, vegetation and human drivers. Sci. Rep. 5:13356. doi: 10.1038/srep13356
Blarquez, O., Vanniere, B., Marlon, J., Daniau, A. L., Power, M. J., Brewer, S., et al. (2015b). paleofire: an R package to analyse sedimentary charcoal records from the global charcoal database to reconstruct past biomass burning. Comput. Geosci. 72, 255–261. doi: 10.1016/j.cageo.2014.07.020
Blarquez, O., Talbot, J., Paillard, J., Lapointe-Elmrabti, L., Pelletier, N., and St-Pierre, C. G. (2018). Late Holocene influence of societies on thefire regime in southern Quebec temperate forests. Q. Sci. Rev. 180, 63–74. doi: 10.1016/j.quascirev.2017.11.022
Boisvert-Marsh, L., Périé, C., and de Blois, S. (2014). Shifting with climate? Evidence for recent changes in tree species distribution at high latitudes. Ecosphere 5:83.
Boulanger, Y., Taylor, A. R., Price, D. T., Cyr, D., McGarrigle, E., Rammer, W., et al. (2017). Climate change impacts on forest landscapes along the Canadian southern boreal forest transition zone. Landsc. Ecol. 32, 1415–1431.
Bowman, D. M. J. S., Balch, J., Artaxo, P., Bond, W. J., Cochrane, M. A., D’Antonio, C. M., et al. (2011). The human dimension of fire regimes on Earth. J. Biogeogr. 38, 2223–2236. doi: 10.1111/j.1365-2699.2011.02595.x
Brossier, B., Oris, F., Finsinger, W., Asselin, H., Bergeron, Y., and Ali, A. A. (2014). Using tree-ring record to calibrate peak detection in fire reconstruction based on sedimentary charcoal records. Holocene 24, 635–645. doi: 10.1177/0959683614526902
Burton, P. J., Messier, C., Smith, D. W., and Adamowicz, W. L. (2003). Towards Sustainable Management of the Boreal Forest. Ottawa, ON: National Research Council Research Press.
Carcaillet, C., Bergeron, Y., Richard, P. J. H., Frechette, B., Gauthier, S., and Prairie, Y. T. (2001). Change of fire frequency in the eastern Canadian boreal forests during the Holocene: does vegetation composition or climate trigger the fire regime? J. Ecol. 89, 930–946. doi: 10.1111/j.1365-2745.2001.00614.x
Carcaillet, C., Richard, P. J. H., Bergeron, Y., Fréchette, B., and Ali, A. A. (2010). Resilience of the boreal forest in response to Holocene fire-frequency changes assessed by pollen diversity and population dynamics. Intern. J. Wildland Fire 19, 1026–1039. doi: 10.1071/wf09097
Chaste, E., Girardin, M. P., Kaplan, J. O., Bergeron, Y., and Hély, C. (2019). Increases in heat-induced tree mortality could drive reductions of biomass resources in Canada’s managed boreal forest. Landsc. Ecol. 34, 403–426.
Conover, W. J., and Iman, R. L. (1981). Rank transformations as a bridge between parametric and nonparametric statistics. Am. Statist. 35, 124–129. doi: 10.2307/2683975
Dansereau, P. R., and Bergeron, Y. (1993). Fire history in the southern boreal forest of northwestern Quebec. Can. J. For. Res. 23, 25–32. doi: 10.1139/x93-005
Delong, S. C., and Tanner, D. (1996). Managing the pattern of forest harvest: lessons from wildfire. Biodiver. Conserv. 5, 1191–1205. doi: 10.1007/bf00051571
Fisher, R. A., Koven, C. D., Anderegg, W. R. L., Christoffersen, B. O., Dietze, M. C., Farrior, C. E., et al. (2018). Vegetation demographics in earth system models: a review of progress and priorities. Glob. Chang. Biol. 24, 35–54. doi: 10.1111/gcb.13910
Flannigan, M. D., Stocks, B. J., Turetsky, M., and Wotton, M. (2009). Impacts of climate change on fire activity and fire management in the circumboreal forest. Glob. Chang. Biol. 15, 549–560. doi: 10.1111/j.1365-2486.2008.01660.x
Forestry Canada Fire Danger Group (1992). Development and Structure of the Canadian Forest Fire Behavior Prediction System. Ottawa, ON: Forestry Canada.
Gauthier, S., Bernier, P., Kuuluvainen, T., Shvidenko, A., and Schepaschenko, D. G. (2015). Boreal forest health and global change. Science 349:819.
Gauthier, S., Vaillancourt, M.-A., Leduc, A., De Grandpré, L., Kneeshaw, D., Morin, H., et al. (2009). Sustainable Management in the Boreal Forest. Montréal: Presses de l’Université du Québec.
Girardin, M. P., Ali, A. A., Carcaillet, C., Blarquez, O., Hély, C., Terrier, A., et al. (2013a). Vegetation limits the impact of a warm climate on boreal wildfires. New Phytol. 199, 1001–1011. doi: 10.1111/nph.12322
Girardin, M. P., Ali, A. A., Carcaillet, C., Gauthier, S., Hély, C., Le Goff, H., et al. (2013b). Fire in managed forests of eastern Canada: risks and options. For. Ecol. Manag. 294, 238–249. doi: 10.1016/j.foreco.2012.07.005
Girardin, M. P., Bergeron, Y., Tardif, J. C., Flannigan, M. D., Gauthier, S., and Mudelsee, M. (2006). Two centuries dendroclimatic inferred record of annual forest fire activity for the Boreal Shield of Canada. Intern. J. Wildland Fire 15, 375–388. doi: 10.1071/wf05065
Girardin, M. P., Portier, J., Remy, C., Ali, A. A., Paillard, J., Blarquez, O., et al. (2019). Coherent signature of warming-induced extreme sub-continental boreal wildfire activity 4800 and 1100 years BP. Environ. Res. Lett. 14:ab59c9. doi: 10.1088/1748-9326/ab59c9
Gray, D. R. (2013). The influence of forest composition and climate on outbreak characteristics of the spruce budworm in eastern Canada. Can. J. For. Res. 43, 1181–1195. doi: 10.1139/cjfr-2013-0240
Harvey, B. (1999). The lake Duparquet research and teaching forest: building a foundation for ecosystem management. For. Chron. 75, 389–393. doi: 10.5558/tfc75389-3
Hély, C., Bergeron, Y., and Flannigan, M. D. (2000). Effects of stand composition on fire hazard in mixed-wood Canadian boreal forest. J. Veg. Sci. 11, 813–824. doi: 10.2307/3236551
Hély, C., Flannigan, M. D., Bergeron, Y., and McRae, D. (2001). Role of vegetation and weather on fire behavior in the Canadian mixedwood boreal forest using two fire behavior prediction systems. Can. J. For. Res. 31, 430–441. doi: 10.1139/x00-192
Hély, C., Fortin, M. J., Anderson, K. H., and Bergeron, Y. (2010a). Landscape composition influences local pattern of fire size in the eastern Canadian boreal forest: role of weather and landscape mosaic on fire size distribution in mixedwood boreal forest using the Prescribed Fire Analysis System. Intern. J. Wildland Fire 19, 1099–1109. doi: 10.1071/wf09112
Hély, C., Girardin, M. P., Ali, A. A., Carcaillet, C., Brewer, S., and Bergeron, Y. (2010b). Eastern boreal North American wildfire risk of the past 7000 years: a model-data comparison. Geophys. Res. Lett. 37:2010GL043706. doi: 10.1029/2010GL043706
Hennebelle, A., Aleman, J. C., Ali, A. A., Bergeron, Y., Carcaillet, C., Grondin, P., et al. (2020). The reconstruction of burned area and fire severity using charcoal from boreal lake sediments. Holocene 30, 1400–1409. doi: 10.1177/0959683620932979
Hennebelle, A., Grondin, P., Aleman, J. C., Ali, A. A., Bergeron, Y., Borcard, D., et al. (2018). Using paleoecology to improve reference conditions for ecosystem-based management in western spruce-moss subdomain of Québec. For. Ecol. Manag. 430, 157–165. doi: 10.1016/j.foreco.2018.08.007
Higuera, P. E., Gavin, D. G., Bartlein, P. J., and Hallett, D. J. (2010). Peak detection in sediment-charcoal records: impacts of alternative data analysis methods on fire-history interpretations. Intern. J. Wildland Fire 19, 996–1014. doi: 10.1071/wf09134
Higuera, P. E., Peters, M. E., Brubaker, L. B., and Gavin, D. G. (2007). Understanding the origin and analysis of sediment-charcoal records with a simulation model. Quat. Sci. Rev. 26, 1790–1809. doi: 10.1016/j.quascirev.2007.03.010
Higuera, P. E., Whitlock, C., and Gage, J. A. (2011). Linking tree-ring and sediment-charcoal records to reconstruct fire occurrence and area burned in subalpine forests of Yellowstone national Park, USA. Holocene 21, 327–341. doi: 10.1177/0959683610374882
Hirsch, K. G., Kafka, V., Tymstra, C., McAlpine, R., Hawkes, B., Stegehuis, H., et al. (2001). Fire-smart forest management: a pragmatic approach to sustainable forest management in fire-dominated ecosystems. For. Chron. 77, 357–363. doi: 10.5558/tfc77357-2
Kelly, R., Chipman, M. L., Higuera, P. E., Stefanova, I., Brubaker, L. B., and Hu, F. S. (2013). Recent burning of boreal forests exceeds fire regime limits of the past 10,000 years. Proc. Natl. Acad. Sci. U.S.A. 110, 13055–13060. doi: 10.1073/pnas.1305069110
Kelly, R., Higuera, P., Barrett, C. M., and Hu, F. S. (2011). A signal-to-noise index to quantify the potential for peak detection in sediment-charcoal records. Q. Res. 75, 11–17. doi: 10.1016/j.yqres.2010.07.011
Krawchuk, M., Lisgo, K., Leroux, S., Vernier, P., Cumming, S., and Schmiegelow, F. (2012). “Boreal forest, Canada,” in Climate and Conservation: Landscape and Seascape Science, Planning, and Action, eds J. Hilty, C. C. Chester, and M. S. Cross (Washington, DC: Island Press), 69–79.
Lauzon, E., Kneeshaw, D., and Bergeron, Y. (2007). Reconstruction of fire history (1680-2003) in Gaspesian mixedwood boreal forests of eastern Canada. For. Ecol. Manag. 244, 41–49. doi: 10.1016/j.foreco.2007.03.064
Le Goff, H., Girardin, M. P., Flannigan, M. D., and Bergeron, Y. (2008). Dendroclimatic inference of wildfire activity in Quebec over the 20th century and implications for natural disturbance-based forest management at the northern limit of the commercial forest. Intern. J. Wildland Fire 17, 348–362. doi: 10.1071/wf07080
Liu, K.-B. (1990). Holocene paleoecology of the boreal forest and Great Lakes-St Lawrence forest in northern Ontario. Ecol. Monogr. 60, 179–212. doi: 10.2307/1943044
Michetti, M., and Zampieri, M. (2014). Climate-human-land interactions: a review of major modelling approaches. Land 3, 793–833. doi: 10.3390/land3030793
Mitchell, T. D., and Jones, P. D. (2005). An improved method of constructing a database of monthly climate observations and associated high-resolution grids. Int. J. Climatol. 25, 693–712. doi: 10.1002/joc.1181
New, M. G., Lister, D., Hulme, M., and Makin, I. (2002). A high-resolution data set of surface climate over global land areas. Clim. Res. 21, 1–25. doi: 10.3354/cr021001
Niklasson, M., and Granström, A. (2000). Numbers and sizes of fires: long-tern spatially explicit fire history in a Swedish boreal landscape. Ecology 81, 1484–1499. doi: 10.1890/0012-9658(2000)081[1484:nasofl]2.0.co;2
Oris, F., Ali, A. A., Asselin, H., Paradis, L., Bergeron, Y., and Finsinger, W. (2014). Charcoal dispersion and deposition in boreal lakes from 3 years of monitoring: differences between local and regional fires. Geophys. Res. Lett. 41, 6743–6752. doi: 10.1002/2014GL060984
Ouarmim, S., Paradis, L., Asselin, H., Bergeron, Y., Ali, A. A., and Hély, C. (2016). Burning potential of fire refuges in the mixedwood boreal forest. Forests 7:246. doi: 10.3390/f7100246
Payette, S. (1992). “Fire as controlling process in the North American boreal forest,” in A Systems Analysis of the Global Boreal Forest, ed. H. H. Shugart (Cambrige: Cambrige University Press), 144–169. doi: 10.1017/cbo9780511565489.006
Pechony, O., and Shindell, D. T. (2010). Driving forces of global wildfires over the past millennium and the forthcoming century. Proc. Natl. Acad. Sci. U.S.A. 107, 19167–19170. doi: 10.1073/pnas.1003669107
Pelletier, G., St-Onge, J., Bordeleau, P., De Rainville, P., Bart, F., Aubin, E., et al. (2009). Classification des Peuplements Forestiers en Tant Que Combustibles, Selon La Méthode Canadienne de Prévision du Comportement des Incendies de Forêt. Troisième Programme Décennal D’inventaire Forestier du Québec. Québec: Société de protection des forêts contre le feu et ministère des Ressources naturelles et de la Faune, Direction de l’environnement et de la protection des forêts.
Pitkänen, A., Lethtonen, H., and Huttunen, P. (1999). Comparison of sedimentary microscopic charcoal particle records in a small lake with dendrochronological data: evidence for the local origin of microscopic charcoal produced by forest fires of low intensity in eastern Finland. Holocene 9, 559–567. doi: 10.1191/095968399670319510
Power, M. J., Marlon, J., Ortiz, N., Bartlein, P. J., Harrison, S. P., Mayle, F. E., et al. (2008). Changes in fire regimes since the Last Glacial maximum: an assessment based on a global synthesis and analysis of charcoal data. Clim. Dyn. 30, 887–907. doi: 10.1007/s00382-007-0334-x
Remy, C., Hély, C., Blarquez, O., Magnan, G., Bergeron, Y., Lavoie, M., et al. (2017). Different regional climatic drivers of Holocene large wildfires in boreal forests of northeastern America. Environ. Res. Lett. 12:035005. doi: 10.1088/1748-9326/aa5aff
Richard, P. J. H. (1980). Histoire postglaciaire de la végétation au sud du lac Abitibi, Ontario et Québec. Géogr. Phys. Q. 34, 77–94. doi: 10.7202/1000385ar
Richardson, C. W. (1981). Stochastic simulation of daily precipitation, temperature, and solar radiation. Water Resour. Res. 17, 182–190. doi: 10.1029/wr017i001p00182
Stocks, B. J., Mason, J. A., Todd, J. B., Bosch, E. M., Wotton, B. M., Amiro, B. D., et al. (2003). Large forest fires in Canada, 1959-1997. J. Geophys. Res. 108:JD000484. doi: 10.1029/2001JD000484
Terrier, A., Girardin, M. P., Périé, C., Legendre, P., and Bergeron, Y. (2013). Potential changes in forest composition could reduce impacts of climate change on boreal wildfires. Ecol. Appl. 23, 21–35. doi: 10.1890/12-0425.1
Valdes, P. J., Armstrong, E., Badger, M. P. S., Bradshaw, C. D., Bragg, F., Crucifix, M., et al. (2017). The BRIDGE HadCM3 family of climate models: HadCM3@Bristol v1.0. Geosci. Model Dev. 10, 3715–3743. doi: 10.5194/gmd-10-3715-2017
van Bellen, S., Garneau, M., and Booth, R. K. (2011). Holocene carbon accumulation rates from three ombrotrophic peatlands in boreal Quebec, Canada: impact of climate-driven ecohydrological change. Holocene 21, 1217–1231. doi: 10.1177/0959683611405243
van Wagner, C. E. (1987). Development and Structure of the Canadian Forest Fire Weather Index System. Ottawa, ON: Canadian Forestry Service.
Waito, J., Girardin, M. P., Tardif, J. C., Hély, C., Blarquez, O., and Ali, A. A. (2015). “Fire and climate: using the past to predict the future,” in Handbook of Forest Ecology, eds K. S. H. Peh, R. T. Corlett, and Y. Bergeron (Berlin: Springer), 489–503.
Westerling, A. L., Hidalgo, H. G., Cayan, D. R., and Swetnam, T. W. (2006). Warming and earlier spring increase in Western U.S. forest wildfire activity. Science 313, 940–943. doi: 10.1126/science.1128834
Keywords: fire frequency, biomass burning, fire-season length, Drought Code, charcoals, lake sediments, Probabilistic Fire Analysis System, mixedwood-coniferous forest transition
Citation: Hély C, Chaste E, Girardin MP, Remy CC, Blarquez O, Bergeron Y and Ali AA (2020) A Holocene Perspective of Vegetation Controls on Seasonal Boreal Wildfire Sizes Using Numerical Paleo-Ecology. Front. For. Glob. Change 3:511901. doi: 10.3389/ffgc.2020.511901
Received: 13 November 2019; Accepted: 21 August 2020;
Published: 29 September 2020.
Edited by:
Rachel Loehman, United States Geological Survey (USGS), United StatesReviewed by:
Alan Tepley, University of Montana, United StatesMelissa Lucash, Portland State University, United States
Copyright © 2020 Hély, Chaste, Girardin, Remy, Blarquez, Bergeron and Ali. This is an open-access article distributed under the terms of the Creative Commons Attribution License (CC BY). The use, distribution or reproduction in other forums is permitted, provided the original author(s) and the copyright owner(s) are credited and that the original publication in this journal is cited, in accordance with accepted academic practice. No use, distribution or reproduction is permitted which does not comply with these terms.
*Correspondence: Christelle Hély, Y2hyaXN0ZWxsZS5oZWx5QGVwaGUucHNsLmV1
†These authors share first authorship
‡Present address: Emeline Chaste, Université de Lorraine, AgroParisTech, INRAE, UMR SILVA, Nancy, France; Cécile C. Remy, Department of Biology, The University of New Mexico, Albuquerque, NM, United States