- 1Soil Science and Soil Protection, Institute of Agricultural and Nutritional Sciences, Martin Luther University Halle-Wittenberg, Halle (Saale), Germany
- 2Department System Ecotoxicology, Helmholtz Centre for Environmental Research, Leipzig, Germany
- 3Soil Biogeochemistry, Institute of Agricultural and Nutritional Sciences, Martin Luther University Halle-Wittenberg, Halle (Saale), Germany
- 4Department Soil Ecology, Faculty for Biology, Chemistry and Earth Sciences, University of Bayreuth, Bayreuth, Germany
In forests, where the supply of bioavailable phosphorus (P) from easily weatherable primary minerals is small, plants are thought to recycle P efficiently by uptake of P released from decomposing forest floor material. Yet a share of the P is leached into the subsoil, where it is strongly adsorbed onto the reactive surfaces of pedogenic Fe and Al oxides. This raises the question of whether P leached into subsoil is also recycled. To investigate the mobilization of P bound to hydrous Fe oxides, we conducted a mesocosm experiment in a greenhouse. Beech saplings were grown for 14 months in subsoil material (Bw horizon from the P-poor Lüss beech forest) with added goethite-P adsorption complexes, in either inorganic (orthophosphate) or organic (phytate) form. Four types of control mesocosms were run: soil only and soil mixed with either dissolved orthophosphate or dissolved phytate or goethite. At the end of the experiment, neither total P mass in trees nor P contents in leaves differed between the treatments. According to leaf nutrient contents, plant growth was strongly limited by P in all treatments. Yet total P mass in trees did not increase over the course of the experiment. Thus, despite its P demand, beech was not able to acquire P from goethite surfaces within two vegetation periods. Also P added in dissolved form to the soil before transplanting as well as native soil P were not available. This suggests that, once inorganic and organic P is bound to pedogenic metal oxides in mineral soil, it is not or hardly recycled, which can be an explanation for field data demonstrating quantitatively significant stocks of P in the subsoil of P-deficient forests.
Introduction
Recent research interest in phosphorus (P) nutrition of European beech (Fagus sylvatica L.) forests is triggered by continued decreases in foliar P concentrations over the last decades (Jonard et al., 2015; Talkner et al., 2015). The decrease was attributed to continuing nitrogen (N) deposition and increasing atmospheric carbon (C) dioxide concentrations, which accelerate plant growth and might thereby induce P limitation (Jonard et al., 2015; Talkner et al., 2015). A conceptual framework for P nutrition of beech forests is provided by Lang et al. (2017), who tested if P acquisition strategies of plants and microbes differ across beech forests with different parent material and soil P stocks using a geosequence approach. In particular, their work suggests that, in forests with young soils, plants and soil organisms mobilize P mainly from primary minerals, and P losses are high (“acquiring strategy.” open P cycles), and in forests with mature soils, roots and microorganisms sustain their P demand mainly from the forest floor and soil horizons rich in organic matter, and P losses are low (“recycling strategy,” tight P cycles). Their large data set supports the hypothesis of Odum (1969) on the nutrition strategies of vegetation, which presumes that P cycles “tighten” during succession, meaning that P losses due to exports become lower due to an efficient uptake and recycling of P (Odum, 1969). Nevertheless, also in beech forests classified as “P recycling systems” by Lang et al. (2017), a share of the P is leached into subsoil, where it is strongly adsorbed onto reactive surfaces of pedogenic Fe and Al oxides, the most important sorbents for P at low pH (Walker and Syers, 1976; Hinsinger, 2001). This raises the question of whether P in subsoil is also recycled or if it is unavailable for beech.
It is still a matter of debate to what extent oxide-bound P is available to plants. On the one hand, physicochemical research finds that hydrous Fe and Al oxides can bind P forms very strongly via inner sphere complexes (Hinsinger, 2001). The process has been hypothesized as a main cause for the prevalent P limitation of plant growth (Javaid, 2009). On the other hand, it was demonstrated that plants, bacteria, and fungi are able to release P from mineral surfaces for plant uptake (Hinsinger et al., 2018). Beech trees live in symbiosis with ectomycorrhizal fungi, which supply them with P and, in return, receive photosynthates from the plant. One strategy of the plant–microbe system to release P from minerals is dissolution of the mineral by decreasing soil solution pH and thereby coreleasing P. This can be achieved by exudation of protons or low molecular weight organic acids (Hinsinger et al., 2001). Another strategy is exudation of low molecular weight organic acids, siderophores, or exopolysaccharides, which desorb P from the mineral phases by ligand exchange or complex metal cations involved in P sorption, such as Fe or Al, at low pH (Jones, 1998; Yi et al., 2008; Marschner et al., 2011; Ordoñez et al., 2016; Hinsinger et al., 2018). Thereby, soil exploitation by roots or mycorrhizal hyphae and, more specifically, their surface is crucial for P acquisition as processes are localized in the rhizosphere (Hinsinger et al., 2001; Lambers et al., 2008).
There is also experimental evidence that oxide-associated P can be available to plants, whereby availability also depends on the P form (Andrino et al., 2019; Klotzbücher et al., 2019; D’Amico et al., 2020). Soil P includes inorganic and organic P; the presumably most persistent P-monoester is phytate (Darch et al., 2014), which might, therefore, predominate in soil. As plants can take up P only as orthophosphate (Vance et al., 2003), organic P must be enzymatically cleaved before it is available to plants (Li et al., 1997). Therefore, plants, bacteria, and fungi exudate phosphatase enzymes, which serve to cleave a phosphate group from its substrates (Margalef et al., 2017). Yet enzymatic hydrolysis of organic P might be hampered by adsorption of organic phosphorylated compounds to mineral phases (George et al., 2016). In addition, the inorganic P that results from the hydrolysis might also adsorb to mineral surfaces, which hampers its uptake by plants.
Recently, D’Amico et al. (2020) exposed mesh bags filled with goethite associated with orthophosphate or phytate for 13 months to P-poor soils of the Italian Alps. Phosphorus was lost from the mesh bags to a larger extent than Fe, suggesting that P was directly released from mineral surfaces. The P release was attributed to ectomycorrhizal activity as fungal growth on the mineral surfaces was detected. The extent of organic P release was smaller than that of inorganic P release. In a mesocosm experiment, Andrino et al. (2019) showed that arbuscular mycorrhiza in symbiosis with tomato were able to acquire orthophosphate and phytate from associations with goethite. Again, more P was plant-available in the treatment with inorganic P. This finding is consistent with the observation that more goethite-associated phosphate than phytate was extractable by an anion exchange resin in HCO3– form, which mimics a plant root taking up P (Klotzbücher et al., 2019). In a hydroponic experiment, palisade grass and ruzigrass took up orthophosphate that was associated with goethite or amorphous Al oxide (Merlin et al., 2016). The authors hypothesized that this was due to exudation of organic acids or enzymes by the plants. Also ryegrass was able to take up goethite-associated phosphate in a pot experiment, in which P-loaded goethite was mixed with sand (Parfitt, 1979).
We conducted a mesocosm experiment to test if P from subsoil of the Lüss forest, which was classified as a “P recycling system” by Lang et al. (2017) is available to beech. In more detail, we were interested (i) if P adsorbed to goethite, which is by far the most abundant Fe oxide in soil (Cornell and Schwertmann, 2003), is available to beech, and (ii) if adsorbed organic P is less available than adsorbed inorganic P. Thus, we produced two different goethite-P adsorption complexes using orthophosphate as an inorganic P source and phytate as an organic P source. We mixed the produced adsorption complexes with Lüss subsoil and grew beech saplings for 14 months in experimental pots in a greenhouse. We hypothesized that (i) P from Lüss subsoil and from the goethite-P adsorption complexes is available to beech and that (ii) organic P adsorbed to goethite is less available than adsorbed inorganic P because it not only needs to be desorbed but also mineralized (e.g., by enzymatic cleavage) before it can be taken up by plants.
Materials and Methods
Preparation of Goethite-P Adsorption Complexes
Goethite was loaded with inorganic P (orthophosphate) or organic P (phytate) to serve as P source for beech saplings in the mesocosm experiment. Solutions containing 1 g P l–1 were prepared using KH2PO4 or K-phytate. The pH was adjusted to 4.0 using HCl or KOH. Goethite (Bayferrox 920 Z, Lanxess, Köln, Germany) was weighed in portions of 100 g into 1-l bottles and filled with 800 ml of P solutions. Suspensions were shaken overhead for at least 16 h to equilibrate and were afterward centrifuged at 5050 rpm for 45 min at 5°C. Supernatants were withdrawn by suction and discarded. To remove all dissolved P, goethite was resuspended in deionized water by shaking the bottles on a horizontal shaker at 275 rpm for 10 min, and then, they were placed in an ultrasonic bath for 3 min and shaken again in a horizontal shaker at 275 rpm for 10 min. Thereafter, bottles were centrifuged and supernatants were discarded. These steps were repeated until the supernatant had reached an electric conductivity < 100 μS. The produced P-loaded goethite was agitated in deionized water and frozen at −20°C. After a few days, samples were freeze-dried, pestled, and sieved < 250 μm. All replicates of one treatment were homogenized. To determine the amounts of adsorbed P, aliquots of 50 mg P-loaded goethite were dissolved in 100 ml HCl (32%) for 48 h at 50°C. Concentrations of P and Fe in extracts were determined using inductively coupled plasma-optical emission spectroscopy (ICP-OES, Ultima 2, Horiba Jobin-Yvon, Longjumeau, France). The produced goethite-P adsorption complexes contained 1.17 and 1.84 mg P g–1 goethite–1 for orthophosphate and phytate loadings, respectively.
Mesocosm Experiment
Experimental Setup
Beech saplings of 35–40 cm height were excavated during bud break from the nursery Billen Forst in Bösinghausen, Germany. The nursery was chosen as no mineral fertilizer was applied to saplings; thus, internal P storage of the saplings was assumed not to be too high to render P uptake from soil during the experiment unnecessary. Saplings were stored in darkness at 5°C until transplantation on May 23, 2017. Roots were visibly mycorrhized and additionally inoculated directly before transplanting using a water extract of the organic layer from Lüss forest. The inoculum was prepared by mixing equal masses of material from all organic horizons and extracting it for 48 h in distilled water (soil:solution ratio 1:2) at 4°C. Before inoculation, the extract was diluted 1:1 with deionized water to obtain enough volume; then, all roots were dipped into the extract before each sapling was transplanted into one pot.
Soil was sampled from the Bw horizon of the Hyperdystric Folic Cambisol (Arenic, Loamic, Nechic, Protospodic) of the Lüss Forest (Table 1, Lang et al., 2017). After sampling, soil was air dried, sieved to < 2 mm, and homogenized. To estimate the total content of Fe in pedogenic Fe oxide phases and associated P, the hot dithionite-citrate-bicarbonate (DCB) extraction was applied as outlined by Mehra and Jackson (1960). To estimate contents of Al and Fe in short range-ordered forms and organic complexes and associated P, extraction with ammonium oxalate at pH 3.0 and 2 h shaking in the dark was carried out according to Schwertmann (1964). Plant-available P was estimated by extraction with sodium bicarbonate (Olsen et al., 1954). The concentrations of extracted Fe, Al, and P were determined by ICP-OES. Results were as follows: FeDCB: 1144 mg kg–1, PDCB: 28 mg kg–1, Feoxalate: 146 mg kg–1, Aloxalate: 569 mg kg–1, Poxalate: 11 mg kg–1, POlsen: 2 mg kg–1.
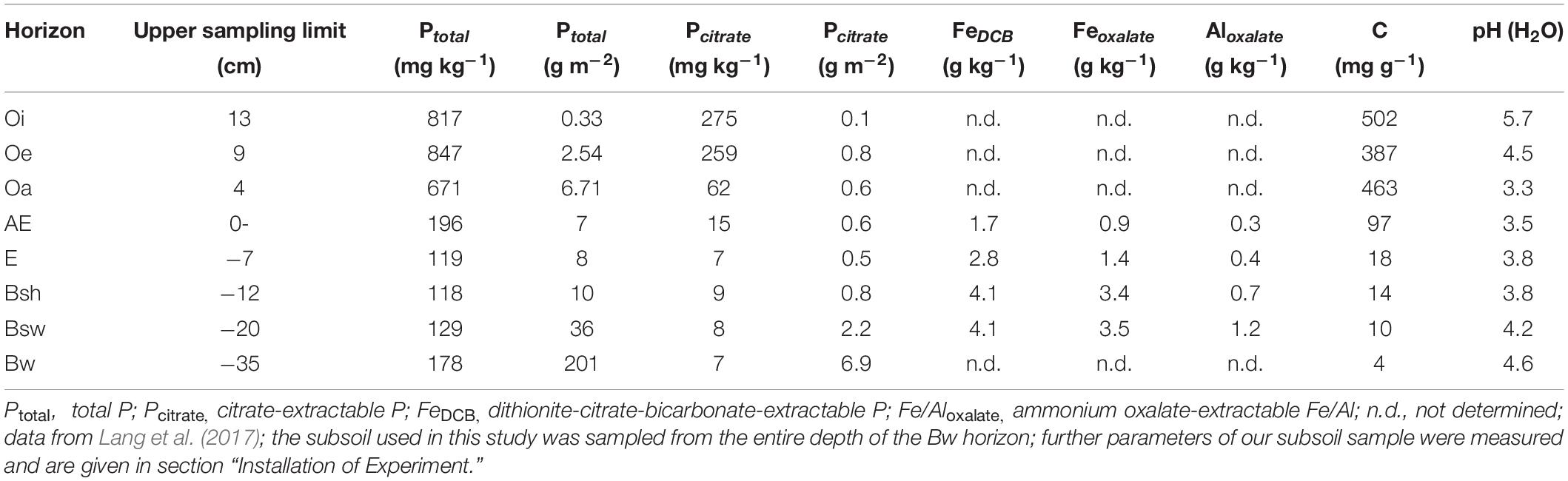
Table 1. Soil characteristics of the Hyperdystric Folic Cambisol (Arenic, Loamic, Nechic, Protospodic) in the Lüss Forest (the Bw horizon extends from 35 to 106 cm soil depth).
Each pot contained 7 kg of the subsoil. Six different treatments were installed in 10 replicates each. For the treatments receiving goethite-P adsorption complexes, soil was either mixed with the goethite preloaded with inorganic P (Goethite Pinorg) or with the goethite preloaded with organic P (Goethite Porg). In the corresponding control treatments, either pure goethite (Goethite) or only orthophosphate (Pinorg) or only phytate (Porg) were added to the soil. Another treatment without any addition to the soil (Control) was installed (Table 2). For all four treatments receiving P (Goethite Pinorg, Goethite Porg, Pinorg, Porg), the additions were normalized to 20 mg P pot–1 (2.9 mg P kg–1 soil). This amount was chosen as it was more than twice of the P that beech saplings contained at start of the experiment (8.5 ± 1.9 mg P per tree), thus assumed to be enough to cover P demand of beech during the experiment. The added P also represented more than 100% of the bicarbonate-extractable P in the native soil. For the treatment Goethite Pinorg, 17.2 g of the adsorption complex (containing 1.17 mg P g–1 goethite–1) and for the treatment Goethite Porg, 10.9 g of the adsorption complex (1.84 mg P g–1 goethite–1) were homogenized with the soil of one pot. For the treatment Goethite, the average amount of those two treatments (14.1 g goethite pot–1) was homogenized with the soil. For the treatments receiving P without goethite (Pinorg and Porg), corresponding amounts of KH2PO4 or K-phytate (20 mg P pot–1) were added in dissolved form to the soil in pots before saplings were transplanted. To test to what extent both P sources adsorb to the soil, sorption isotherms were produced, suggesting that both P forms were immediately immobilized (Figure 1).
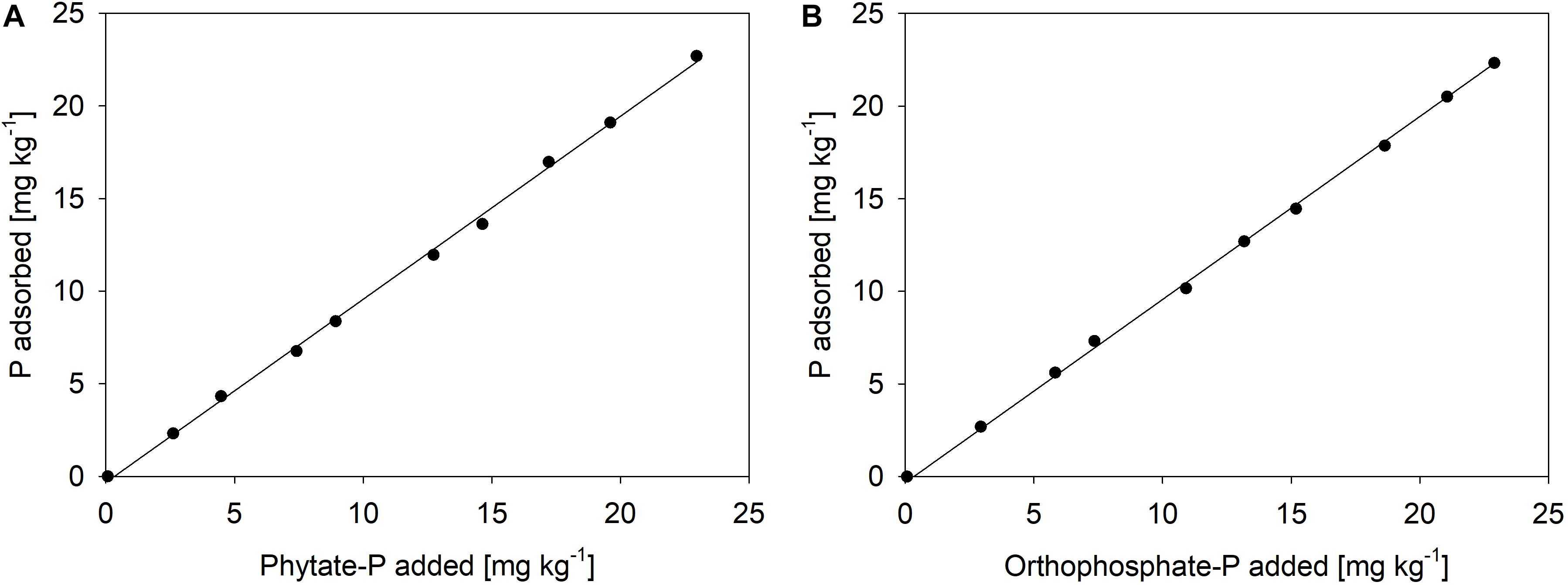
Figure 1. Sorption isotherms of (A) phytate-P and (B) orthophosphate-P to the Lüss Bw horizon, both at pH 4.5 in 1 μM KClO4 solution as a background electrolyte (ca. 100 μS cm– 1); the amount of P fertilized in the mesocosm experiment equals the second dot (2.8 mg kg– 1) in both graphics.
Before transplanting, all pots were watered with 1.5 l of a nutrient solution, which contained all essential nutrients except P. Nutrient concentrations in the solution were calculated based on data of soil leachates from the Lüss Forest (unpublished data) by doubling maximum measured concentrations of macronutrients. No data were available for micronutrients; instead concentrations corresponding to those of Hoagland solution were used. The resulting concentrations were 7 mg N l–1, 11 mg K l–1, 6 mg Ca l–1, 2 mg Mg l–1, 2 mg S l–1, 0.5 mg B l–1, 0.01 mg Mo l–1, 0.5 mg Mn l–1, 0.05 mg Zn l–1, and 0.02 mg Cu l–1.
Growing Conditions
The mesocosm experiment (Figure 2) was conducted in a greenhouse covering almost two vegetation periods (14 months). During the vegetation periods, temperature was kept between 20 and 25°C, and light intensity was reduced by shading the glass roof. In winter during bud break, temperature in the greenhouse was between 5 and 10°C. Pots were assembled randomly in the greenhouse and were periodically rotated to avoid edge effects in terms of wind from the cooling system and exposition to sunlight. During the first vegetation period, plants were irrigated regularly with the nutrient solution, and thereby volumetric water content was kept between 10 and 20%, which is in range of field capacity of a sandy soil as measured with a time domain reflectometry probe. During bud break and during the second vegetation period, plants were regularly watered using deionized water, and nutrient solution was only added in irregular intervals.
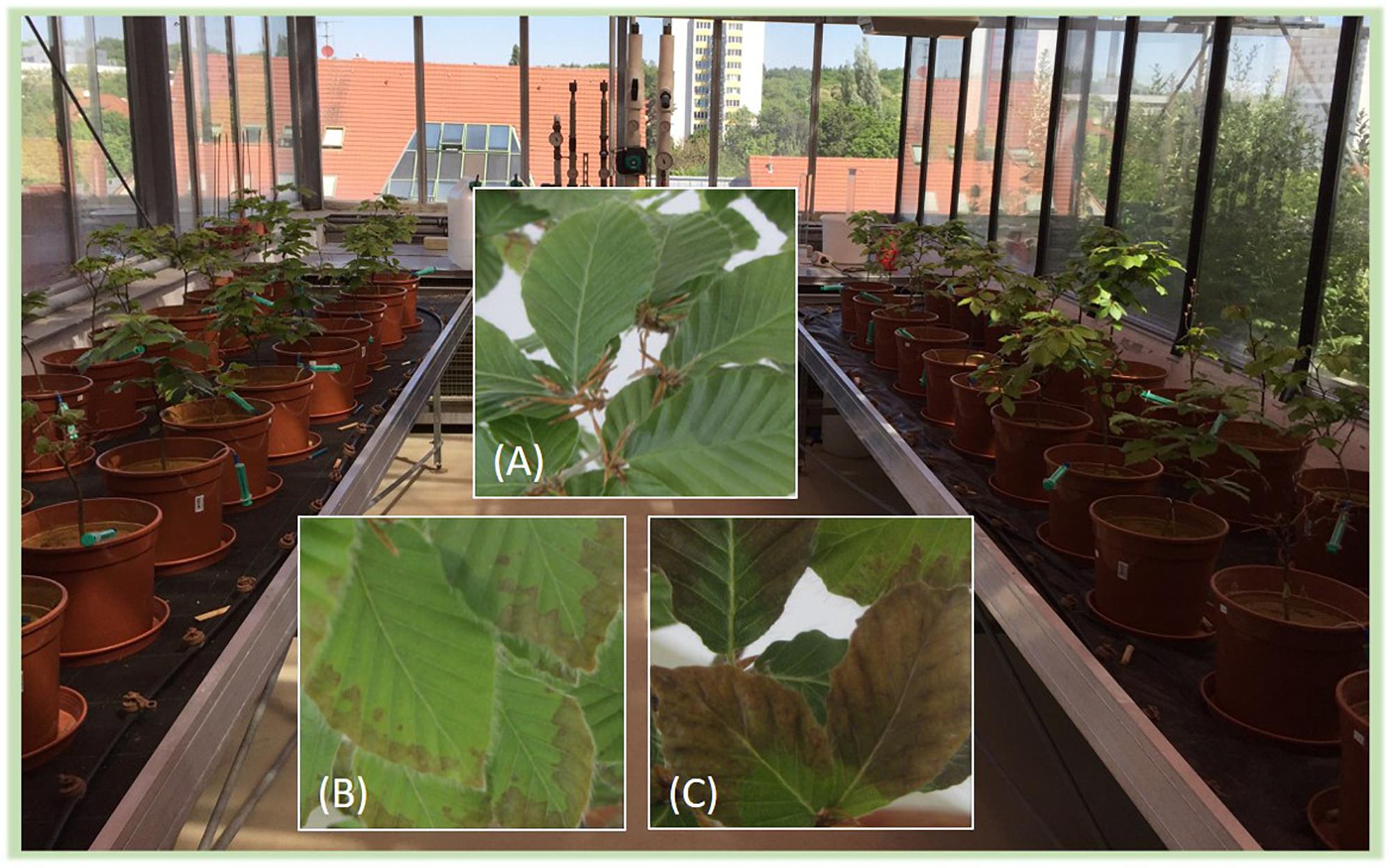
Figure 2. Picture of the mesocosm experiment and of leaves (A) without symptoms, (B) with moderate symptoms, and (C) with severe symptoms supposedly caused by P deficiencies.
Two weeks after transplanting, it turned out that beech saplings were infected with wooly beech scales (Cryptococcus fagisuga). To reduce this infection, all trees were regularly sprayed with Imidacloprid, a systemic P-free insecticide (Confidor, Bayer, Leverkusen, Germany). Despite this, the number of replicates was reduced from 10 to 4–8 depending on the treatment. The intensity of damage was not attributed to be a treatment effect as pest outbreak occurred shortly after transplanting. More likely, mortality was controlled by the vitality of individual trees.
Plant and Soil Sampling and Sample Preparation
To determine biomass and nutrient content of the beech saplings at the start of the experiment, five extra beech saplings were separated into aboveground biomass (stem and branches) and roots, dried at 60°C for 1 week, and stored until the end of the experiment for analyses.
Senesced leaves that fell from trees during both vegetation periods were collected, dried at 60°C for 1 week, and stored until the end of the experiment for analyses.
Trees were harvested after 14 months of growth on July 26, 2018, by cutting stems directly above the soil surface. Soil that stuck to the roots was sampled by carefully removing the root ball from the soil and shaking it within a plastic bag to collect the soil (here referred to as rhizosphere soil). Rhizosphere soil samples were stored at 4°C for a few days until phosphatase activity was analyzed. Trees were separated into leaves, branches, stem, and roots. Plant fractions were dried at 60°C for 1 week.
Dried plant samples were weighed for mass balance calculations. Thereafter, they were first chopped (if necessary) using either a rotor mill or loppers and then ground to fine powder using a vibratory disc mill for nutrient analyses.
Plant Analyses
To determine concentrations of P, K, Ca, Mg, Mn, B, Fe, Zn, and Cu, 50 mg of each sample were digested in two replicates using a mixture of 4 ml concentrated HNO3, 1 ml H2O2 (30%), and 1 ml deionized water for 45 min in a microwave-assisted digestion chamber (MARS 5, CEM, Kamp-Lintfort, Germany). Solutions were filtered through 0.45 μm syringe filters (hydrophobic PTFE, Millex, Merck, Darmstadt, Germany) before measurement by ICP-OES.
Nitrogen content of leaves was analyzed by using a setup of a coupled elemental analyzer (Carlo Erba NC 2500, Thermo Finnigan, Bremen, Germany) and an isotope ratio mass spectrometer (DeltaPlus, Thermo Finnigan, Bremen, Germany), connected by a Conflo III interface (Thermo Finnigan, Bremen, Germany).
Calculation of Mass Balances
Change of total P (K, Ca, and Mg) mass in trees from the start of the experiment until the end of the experiment, including P (K, Ca, and Mg) lost by leaf fall during both vegetation periods, were calculated as follows:
with
and
and
Data from the “start” of the experiment refer to the five extra beech saplings that were used to determine biomass and nutrient content of beech saplings at start of the experiment. Data from the “end” of the experiment refer to the individual beech saplings grown in pots. “Leaves 2017” refers to all leaves of the first vegetation period, and “leaves 2018” refers to all leaves of the second vegetation period.
Analysis of Phosphatase Activity in Rhizosphere Soil
Phosphatase activity was determined using the fluorogenic substrate 4-methylumbelliferyl-phosphate according to Marx et al. (2001); German et al. (2011), and Herold et al. (2014). One g of moist rhizosphere soil was homogenized in 50 ml of sterile water by shaking for 20 min. The soil was then pipetted into black 96-well microplates (Brand GmbH und Co., KG, Wertheim, Germany), diluted with 50 μl sterile deionized water, and 100 μl substrate solution was added. Samples were preincubated in the dark at 15°C for 30 min and subsequently measured fluorometrically after 0, 60, 120, and 180 min using a microplate reader (Infinite 200 PRO, Tecan, Männedorf, Switzerland). Fluorescence was corrected for quenching of the soil as well as for the fluorescence of substrate and soil (German et al., 2011). Enzyme activity was calculated from the slope of net fluorescence over incubation time.
Statistical Analyses
Statistical analyses were performed using the package ggpubr of R version 3.4.4. Data were tested for normal distribution by quantile–quantile plots and the Shapiro-Wilk test. In case of parametric data sets, analysis of variance followed by multiple student’s t-tests were performed; number of comparisons were corrected after Holm (1979). In the case of non-parametric data sets, a Kruskal-Wallis test was performed, and post hoc analysis was conducted with a Wilcoxon-rank-sum test; number of comparisons were corrected after Holm (1979). A significance level of α = 0.05 was applied.
Results
Biomass Production
During the experimental period of 14 months, biomass of the trees increased on average by 154% from 9.0 ± 2.1 g (standard error, SE) to 23.0 ± 1.2 g, including fallen leaves during both vegetation periods. At the end of the experiment, biomass of all plant fractions (leaves, stem, and roots) did not significantly differ between the treatments (Table 3).
Phosphorus Uptake
At the end of the experiment, P contents of leaves did not significantly differ between the treatments; on average, it was 0.52 ± 0.01 (SE) mg g–1 and, thus, far below the threshold value of 0.95 mg g–1 for deficiency symptoms of beech (Figure 3) as reported by Göttlein et al. (2015). During the second vegetation period, P content of leaves was significantly lower than during the first vegetation period, decreasing on average by 19% (Table 4). The P content of stems also decreased during the experiment on average by 77% and that of roots by 68%.
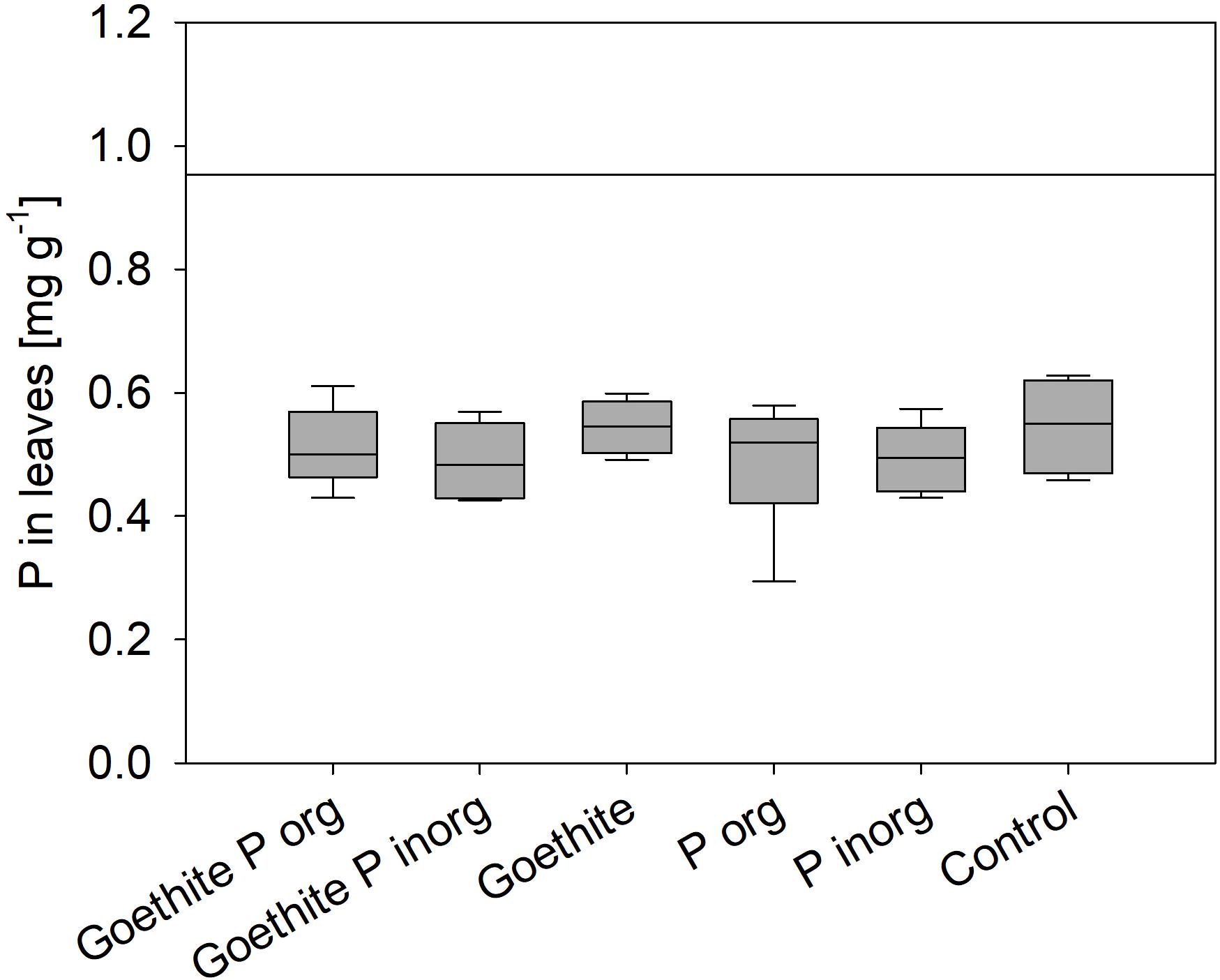
Figure 3. Phosphorus content of leaves at the end of the experiment; error bars represent standard errors; no statistical differences between treatments were detected. Black line represents threshold value for P deficiency symptoms (Göttlein et al., 2015).
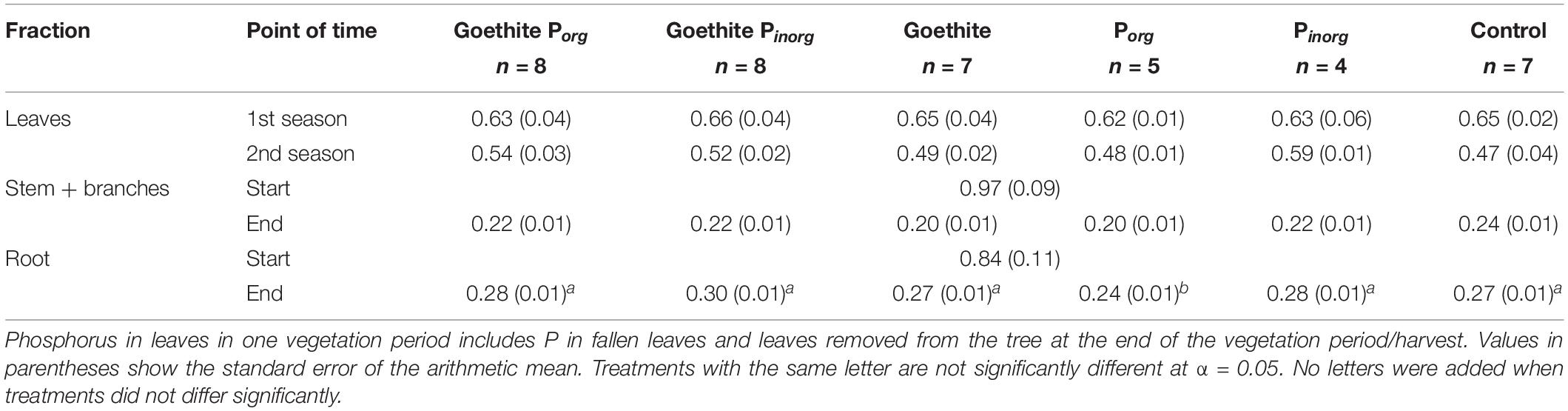
Table 4. Phosphorus contents of beech fractions (mg g–1) at the start (n = 4 because one sample was lost during nutrient analysis) and end of the experiment.
Phosphorus contents did not differ between the treatments for leaves of the first vegetation period, leaves of the second vegetation period (sum of fallen leaves and leaves removed from trees at the end of the experiment), and stems at the end of the experiment (Table 4). Only for roots at the end of the experiment, P content was significantly higher in the treatment Porg than in all other treatments (Table 4).
The results suggest that, during the experiment, no P was taken up from soil in any of the treatments because the sum of total P stored in plants at the end of the experiment plus the P lost with fallen leaves during both vegetation periods did not significantly differ from total P in the trees at the start of the experiment. The sum of total P stored in plants at the end of the experiment plus the P lost with fallen leaves during both vegetation periods did also not significantly differ between the treatments (Figure 4).
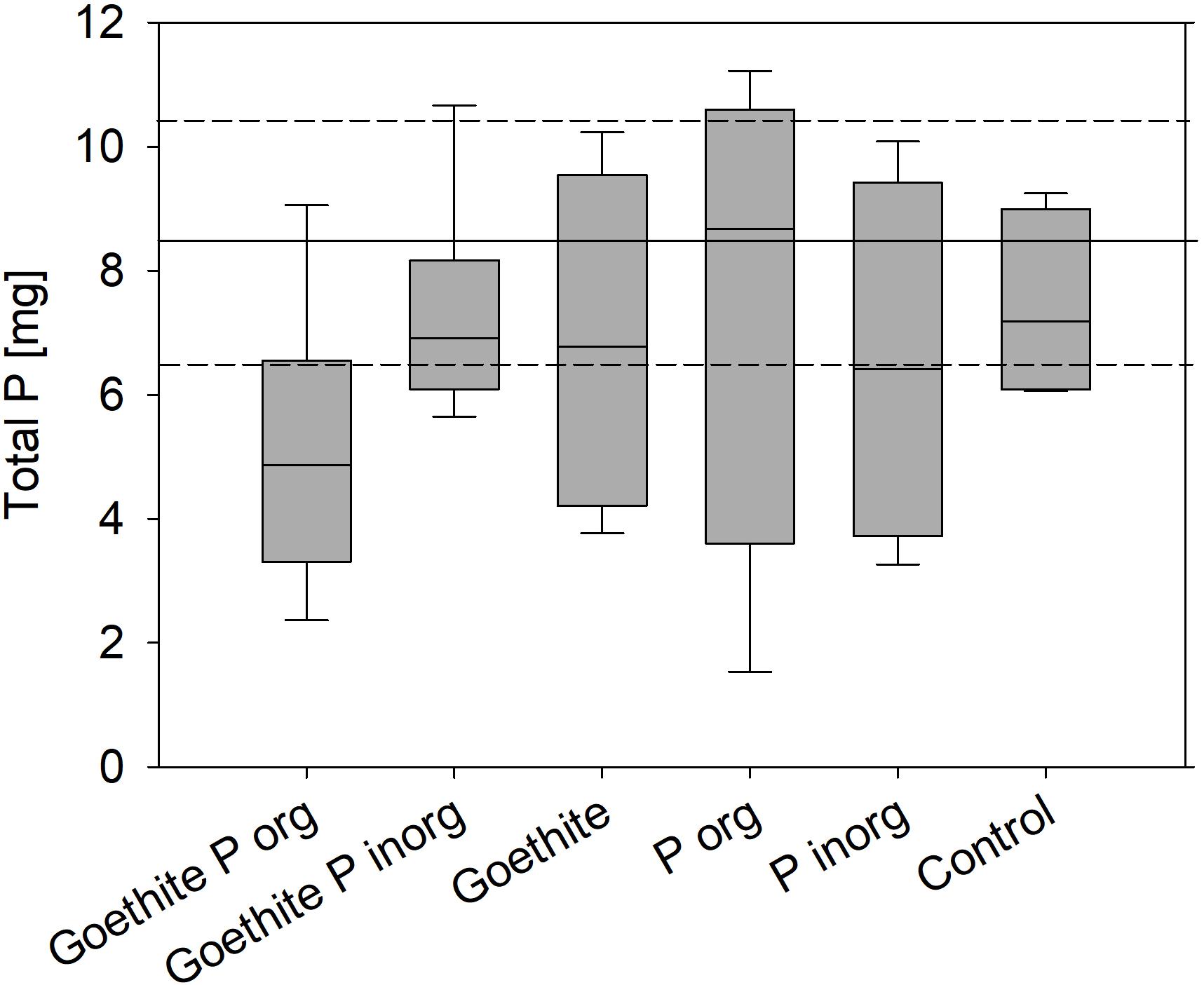
Figure 4. Boxplots represent the sum of total P in trees at the end of the experiment and P lost with leaves during the first and second vegetation period; error bars represent standard errors; no statistical differences between treatments were detected. Black line marks total P in saplings at the start of the experiment; dashed lines represent standard error.
Uptake of Further Essential Nutrients
At the end of the experiment, contents of K, Ca, Mg, Mn, B, Fe, Zn, and Cu in leaves were also compared to threshold values for deficiency symptoms (Table 5) reported by Göttlein et al. (2015). For Ca, measured contents were slightly below the threshold (i.e., on average, 3.7 ± 0.1 mg g–1 compared to 4.0 mg g–1); for all other nutrients, measured contents were above the threshold.
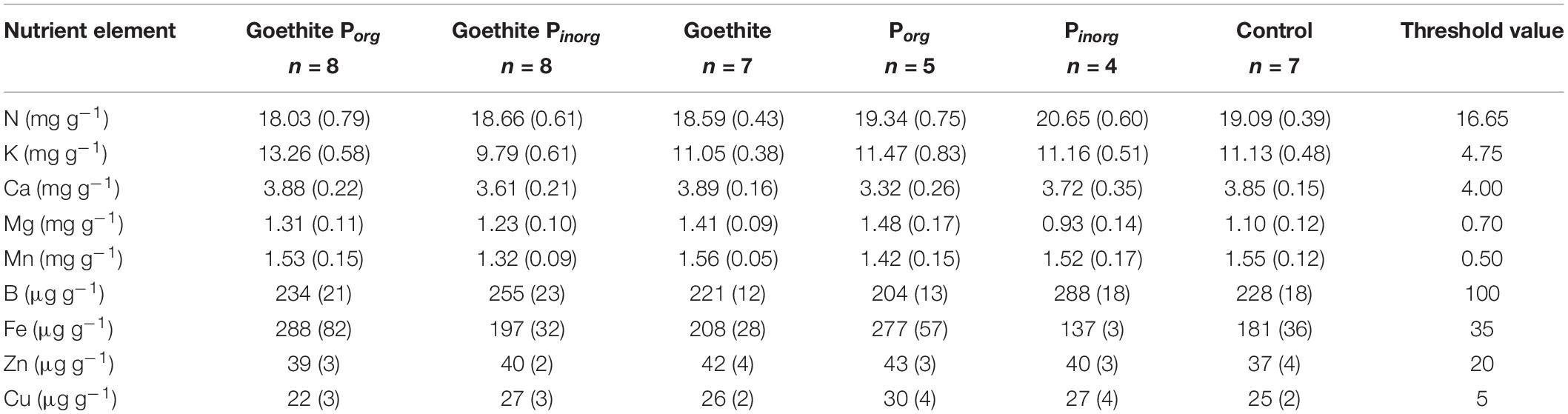
Table 5. Contents of essential nutrient elements in leaves at the end of the experiment in comparison to threshold values for deficiency level (Göttlein et al., 2015).
In contrast to P, total amounts of K, Ca, and Mg in trees increased strongly during the experiment (Figure 5); total K mass in trees increased by 255%, Ca mass by 829%, and Mg mass by 36%. Thus, uptake of Ca and K was faster than biomass production (Figure 5).
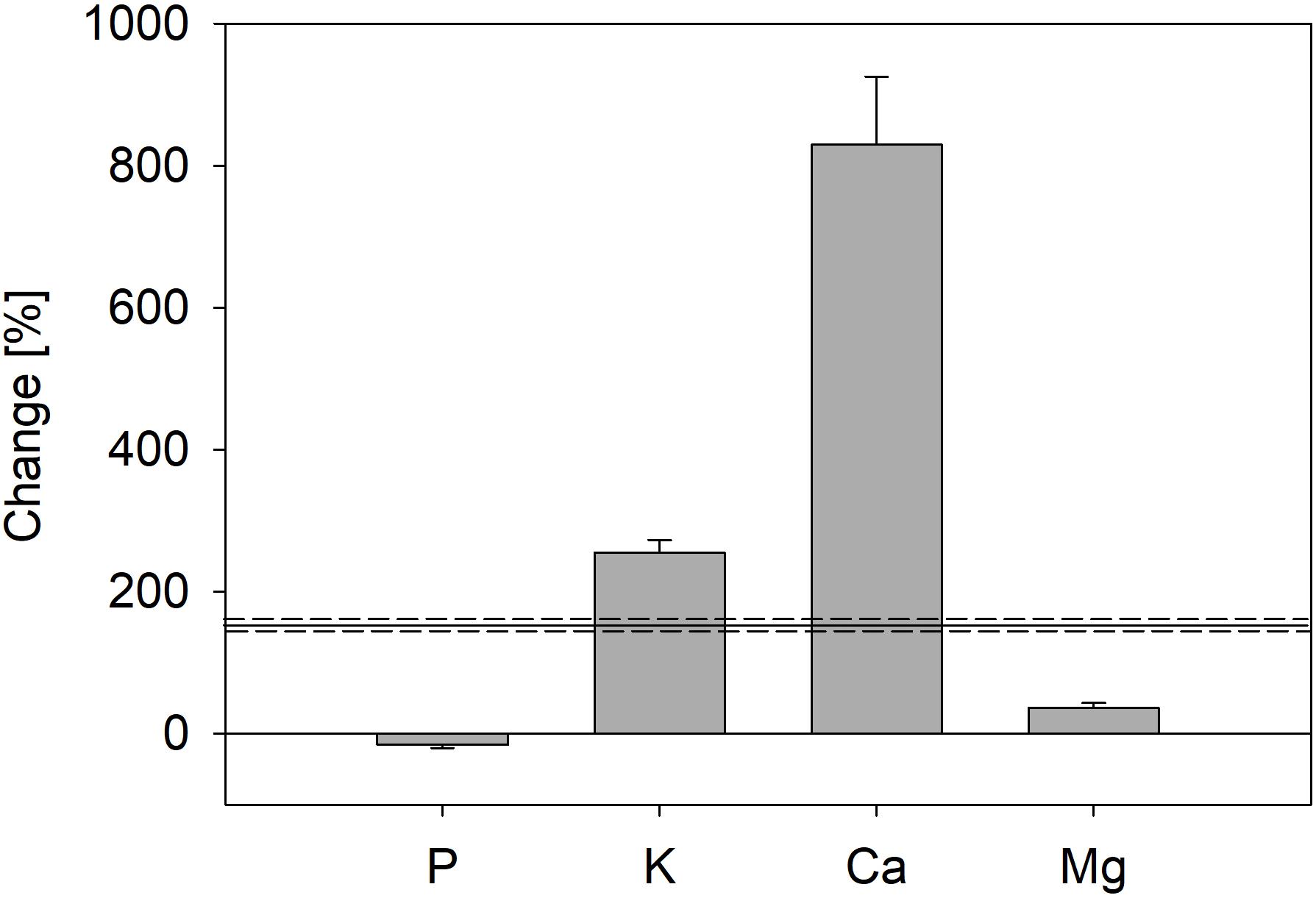
Figure 5. Change of total nutrient mass in trees from the start (four saplings) until end of the experiment, including nutrients lost by leaf fall during both vegetation periods (trees of all treatments were pooled); error bars represent standard errors. Black line marks increase of total biomass during the experiment; dashed lines represent standard error.
Phosphatase Activity
Mean phosphatase activity in the rhizosphere at the end of the experiment was 96 nmol g–1 soil–1 h–1 across all treatments. Mean values of the treatments ranged from 48 to 191 nmol g–1 soil–1 h–1 in the Goethite Porg and Porg treatments, respectively. Overall, phosphatase activity in rhizosphere at the end of the experiment did not differ significantly between treatments (Figure 6).
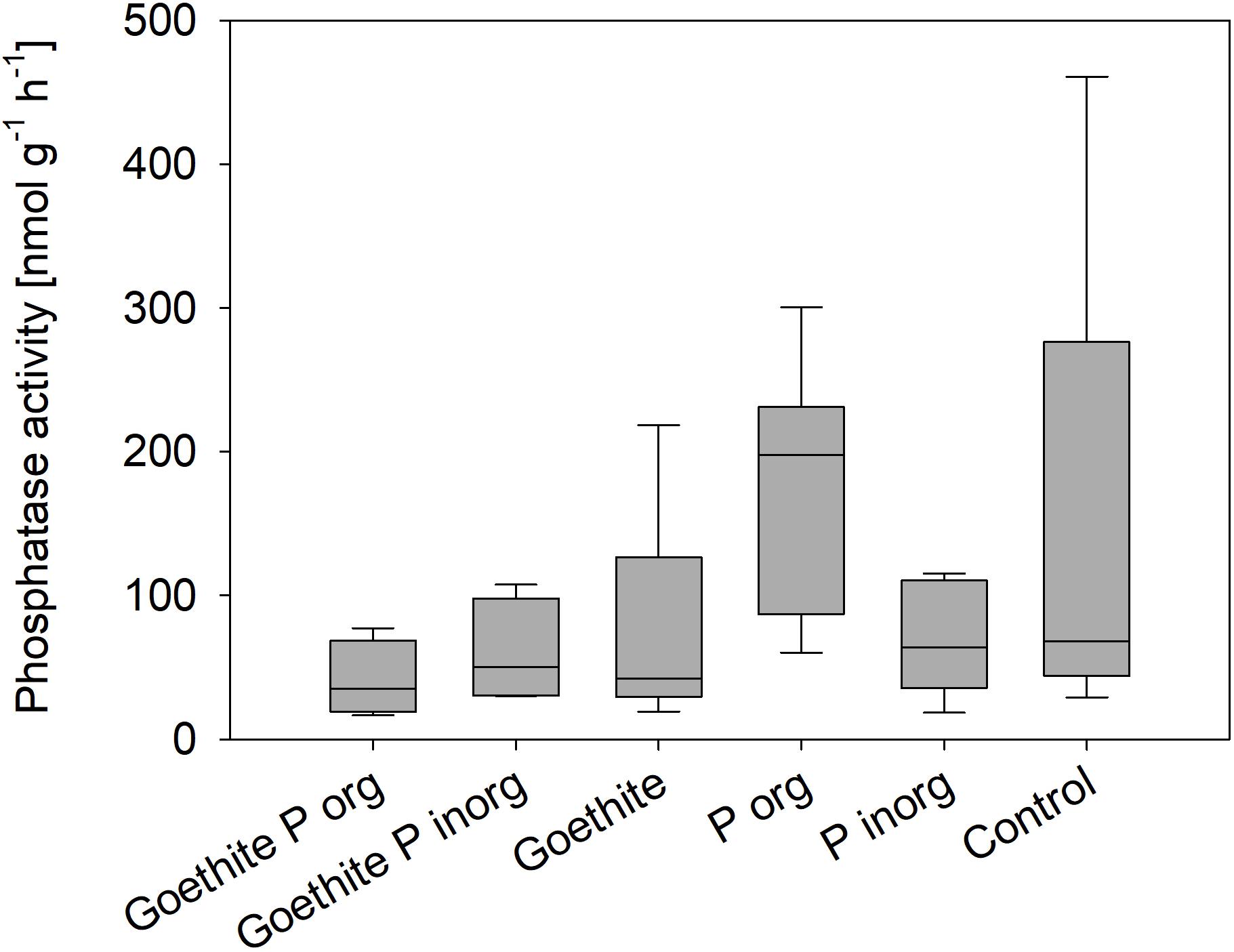
Figure 6. Phosphatase activity in the rhizosphere at the end of the experiment; no statistical differences between treatments were detected.
Discussion
Phosphorus From Lüss Subsoil and From the Added Adsorption Complexes Is Not Available to Beech
Our first hypothesis that P from Lüss subsoil and from the goethite-P adsorption complexes is available to beech was not confirmed in our experiment. The three main findings supporting rejection of the hypothesis are (i) addition of goethite-P adsorption complexes did not affect P uptake by plants although leaf P contents were far below a deficiency level, (ii) addition of dissolved P did not enhance P uptake by beech as it was rapidly immobilized in soil by adsorption to minerals, and (iii) native P from the subsoil was not available to beech as, despite P limitation, beech leaves were only supplied with P by P redistribution within the plant tissue. In the following, these reasons are explained in detail.
(i) The addition of goethite-P adsorption complexes did not affect P uptake by plants although P content of leaves was far below a deficiency level for beech of 0.95 mg g–1 reported by Göttlein (2015). In our experiment, for the given leaf biomass, only 1.1 ± 0.1 mg P would have been required by a tree of each treatment to reach the threshold value for leaf P content. Even though this amount accounts for only 5.5% of the added P (20 mg P pot–1), plants were not able to use the supplied P. For comparison, leaf P contents of adult trees in the Lüss Forest were 1.2 ± 0.1 mg g–1 (Lang et al., 2016), and mature leaves of young trees from the Lüss Forest had similar P contents (Zavišic and Polle, 2018). In a mesocosm experiment in which saplings from Lüss were grown in Lüss soil from the A horizon and the forest floor, leaf P contents of only 0.7 ± 0.3 mg g–1, thus below the deficiency level, were observed, but saplings did take up P from the soil (Hofmann et al., 2016). Meller et al. (2019) conducted a cross-growth experiment, in which soil and beech saplings from sites with the lowest and highest P contents along the geosequence (Lang et al., 2017), Lüss and Bad Brückenau, were used. Lowest leaf P contents of 0.94 ± 0.06 mg g–1 were reported for beech saplings from the Lüss Forest grown in pots with soil from Bad Brückenau. The authors found that internal P allocation within the tree is a better indicator for P availability in soil than leaf P contents. Nevertheless, in none of the cited experiments were leaf P contents as low as in our experiment with a mean of all treatments of 0.52 ± 0.1 mg g–1. Another hint for P limitation of trees was that all saplings showed signs of stress in both vegetation periods (Figure 2). Leaf tips browned and/or brown spots appeared on the leaf area; then signs expanded to the whole leaf and some leaves crinkled and fell off. Even if no descriptions of P limitation symptoms were found in literature for deciduous trees, similar symptoms were described for P deficiency of plants in general (Zorn et al., 2016).
(ii) The reason for the lack of uptake of dissolved P is likely the strong sorption by the used subsoil material. In accordance with the considerable contents of Fe and Al oxides in the used subsoil, the sorption isotherms for orthophosphate and phytate of the Lüss subsoil (Figure 1) suggest nearly complete adsorption of the supplied P by mineral surfaces. The sorption isotherms indicate that sorption even at the eightfold P input was 92–99%. Thus, in the mesocosm experiment, only a small portion of the free sorption sites was necessary to retain virtually all added P. In comparison with experiments in which beech saplings from Lüss were grown in mesocosms with either forest floor and topsoil horizon (Spohn et al., 2018) or with a mixture of soil from the A and B horizons (Hofmann et al., 2016), beech was able to take up added orthophosphate in dissolved form. This can be explained by a lower number of sorption sites for P in soils of those experiments than in soil from the Bw horizon used in our mesocosm experiment. Rapid adsorption of added orthophosphate in Lüss soil might also have taken place in a mesocosm experiment by Hauenstein et al. (2018). In this experiment, radioactive 33P-labeled orthophosphate was applied to beech saplings in pots filled with Lüss soil from the top three mineral horizons with or without the organic layer. In the treatment with the organic layer, radioactivity in the xylem sap of beech was higher than in the treatment without an organic layer, probably because P immediately adsorbed to oxide surfaces in the mineral soil horizons but remained available in the organic layer. Hence, sorption onto oxide surfaces is a process that competes with plants for the supplied P; i.e., in soils rich in sorption sites, P availability remains low even after large inputs of potentially available P forms.
(iii) Data on P allocation across plant parts suggest that native P from the subsoil horizon (Control) was likewise not bioavailable. We noticed that beech leaves of all treatments were only supplied with P by redistribution within plant tissue during the experiment as indicated by decreasing P contents in stems, branches, and roots from the start to the end of the experiment. Improvement of the internal P recycling was shown to be a strategy of trees to cope with low P in the environment (Côté et al., 2002; Hofmann et al., 2016; Netzer et al., 2017; Hauenstein et al., 2018; Meller et al., 2019). In our experiment, initial internal P storage of beech was so low that beech was not able to compensate for low P availability by P redistribution only, but additional P from soil would have been required to cover the P demand. Surprisingly, leaf biomass increased from the first to the second vegetation period even if it was shown that growth reduction is another strategy of beech trees to cope with low P supply (Yang et al., 2016; Zavišic and Polle, 2018; Meller et al., 2019). Increasing P deficiency from the first to the second vegetation period might also be attributed to a dilution effect of P in plant tissue by biomass increase. Biomass increase was larger for roots than for stems and branches; the rapid root growth might have been one attempt (without effect) of beech to access more P by increasing the root/shoot ratio and the root/soil interface. The total root surface area of plants is one major factor limiting P uptake besides P concentration at the absorbing root surface and the distribution of roots in the soil (Lambers et al., 2008).
The factors limiting P uptake depicted by Lambers et al. (2008) might also explain why our results differ from results of Merlin et al. (2016) and Klotzbücher et al. (2019). In the study of Klotzbücher et al. (2019) it was shown that 43% of goethite-associated phosphate and 6% of goethite-associated phytate were extracted by an anion exchange resin in HCO3– form, which mimics a plant root taking up P. The resin was placed together with P-loaded goethite in ultrapure water. Phosphorus release was driven by the chemical gradient of P between goethite and solution, and an equilibrium state was permanently changed by P removal by the resin. Compared to this experiment, in our mesocosm experiment, the P concentration in the solid phase and, therefore, also in the soil solution was much lower. Also P diffusion was probably much slower in the pot experiments than in the solution with the resin as P diffusion is known to be slow in soil (Barber, 1995). Further, rooting of the pots and thus the absorbing root surface was very limited (when removing the roots from the pots, we realized that root balls did not fill the whole pot volume). In the study of Merlin et al. (2016), goethite-associated P (and Al oxide-associated P) was available for palisade grass and ruzigrass. As their experiments were conducted in nutrient solutions (without soil), results fit those of P extraction from solution by the resin.
Our results also differ from those of Parfitt (1979); Andrino et al. (2019), and D’Amico et al. (2020), who showed that P associated with goethite was at least partly bioavailable in studies using soil or solid substrates instead of nutrient solutions. In the study of Andrino et al. (2019), arbuscular mycorrhiza associated with tomato plants were able to acquire goethite-associated P. On the one hand, this finding might differ from our results because different types of plants and mycorrhiza were studied. On the other hand, also spatial P distribution in the substrates differed between the experimental setups. In the experiment of Andrino et al. (2019), either pure orthophosphate-loaded goethite was applied or phytate-loaded goethite mixed with pure goethite (same substrate volumes, normalized to P) was offered in a chamber accessible for fungal hyphae only. In our experiment, the P-loaded goethite accounted for only 0.2% of the substrate although P-loadings of goethite were similar. Thus, P concentrations at the absorbing hyphae surfaces (and, in our experiment, also root surfaces) were much higher in the experiment of Andrino et al. (2019). D’Amico et al. (2020) conducted (to our knowledge) the only published experiment in which goethite-P associations were exposed to P-poor surroundings in the field. The study sites are located in the Italian Alps, where European Larch is grown. Goethite-P associations were exposed in mesh bags mixed with quartz sand. High P losses of the mesh bags in combination with ectomycorrhizal colonization were attributed to P release by mycorrhizal fungi. However, due to the experimental setup, it was not possible to test if mobilized P was taken up by trees or if it was readsorbed to mineral surfaces in soil. In the study of Parfitt (1979), ryegrass was able to take up goethite-associated phosphate in a pot experiment in which adsorption complexes were mixed with sand. Probably, P uptake was possible because the lack of other adsorption sites for P in the sand. Taken together, the results of the discussed studies and our mesocosm experiment suggest that P adsorbed to goethite can (at least partly) be released by a plant-microbe system. In substrates without abundant free adsorption sites for P, the released P is plant-available after release from oxides; however, when many free adsorption sites are abundant, such as in our experiment, these are more competitive than plants regarding the desorbed P.
Results of our mesocosm experiment fit to those of Pastore et al. (2020), who showed that goethite-associated phosphate is hardly available to microorganisms especially at low concentrations of easily degradable organic C, which is an important driver for P release by microorganisms (Brucker et al., 2020). In our mesocosm experiment, C-limitation by microbes might, thus, be a further reason why microbes did not release sufficient P from goethite in our C-poor subsoil.
In general, our results suggest that, once P is bound to pedogenic metal oxides in mineral soil, it is no longer recycled, which can be an explanation for field data demonstrating quantitatively significant stocks of P in subsoil of P deficient forests (Lang et al., 2017), such as in Lüss (Table 1). Supposedly, in P recycling systems, P leaching from the forest floor to subsoil is not recycled; i.e., trees entirely rely on P from the organic layer or organic-rich topsoil as suggested by Lang et al. (2017) and confirmed by Leuschner et al. (2006) and Hauenstein et al. (2018). Further, our results are in line with low P availability in highly weathered (thus, Fe and Al oxide-rich) soils under tropical forests (Reed et al., 2010). Generally, in acidic and deeply weathered tropical soils, P-availability is low as a large portion of soil P occurs as bound to and/or occluded in Fe and Al oxides (Jones and Oburger, 2011).
Both Inorganic and Organic P Adsorbed to Goethite Were Not Available to Beech
Our second hypothesis of organic P adsorbed to goethite being less available than adsorbed inorganic P because it requires not only desorption, but also mineralization (e.g., by enzymatic cleavage) before being taken up by plants is not confirmed as none of the two P sources was available. Even though phosphatase activity varied slightly between the treatments, there were no significant differences. A global meta-analysis showed that average acid phosphatase activities in deciduous forests amount up to 14,500 nmol g–1 h–1 (Margalef et al., 2017) – much higher than the phosphatase activities measured in our study. However, studies show that phosphatase activities decrease rapidly with soil depth (Taylor et al., 2002; Zhang et al., 2005; Herold et al., 2014). In subsoil horizons (45-105 cm depth) phosphomonoesterase activity of around 1000 nmol g–1 h–1 in rhizosphere and around 75 nmol g–1 h–1 in bulk soil were measured in an agricultural field (Uksa et al., 2015). Similar to our study, Herold et al. (2014) found phosphatase activities of around 122 nmol g–1 h–1 in the Bw horizon of a nutrient-poor sandy forest soil. An explanation for the low phosphatase activities could be low microbial biomass C concentrations in combination with C-limitation of microbes in this C- and P-poor subsoil (Herold et al., 2014) as well as the plants’ nutrient limitation.
As described in section “Phosphorus From Lüss Subsoil and From the Added Adsorption Complexes Is Not Available to Beech,” organic P adsorbed to goethite was shown to be less available than adsorbed inorganic P in other studies (Andrino et al., 2019; Klotzbücher et al., 2019; D’Amico et al., 2020). This was probably caused by slower desorption of organic P than of inorganic P in the study of D’Amico et al. (2020), in which mycorrhizal colonization of the goethite-P-complexes was measured, and in the study of Klotzbücher et al. (2019), in which desorption induced by a chemical gradient was determined. Desorption rates for organic P from goethite might have been lower than those for inorganic P because of stronger bindings of organic P than of inorganic P to goethite. In the study of Andrino et al. (2019), P uptake by plants was lower for organic than for inorganic P; thus, either slower desorption from goethite and/or the necessity of mineralization of organic P might have caused the difference in availability. However, in our mesocosm experiment, in which even inorganic P was unavailable to beech, both inorganic and organic P were either not released from oxides or rapidly readsorbed to oxides after release.
Conclusion
In the conducted mesocosm experiment, we have shown that beech was not able to take up organic or inorganic P from a subsoil in which P was bound to pedogenic Fe and Al oxides, which additionally provided free adsorption sites. Neither native soil P nor P added in dissolved form nor P from added goethite-P adsorption complexes was taken up by beech saplings. This was attributed to rapid adsorption of dissolved P to hydrous Fe and Al oxides and rapid readsorption of P possibly released by oxides. Thus, oxides were more competitive in taking up and retaining P than plants. Obviously, P from goethite-P adsorption complexes might only be acquired by plant-microbe systems in environments with little other adsorption sites for P. Our results support the view that in P-poor forest ecosystems classified as P recycling systems, trees rely on P from the organic matter and on tree internal P recycling. We conclude for P recycling systems that P leached from decomposing forest floor to subsoil is not or hardly recycled. Further, our results point at a critical view of extractions estimating plant-available P in soil, such as the method using an anion exchange resin in HCO3– form in a water extract because it does not consider additional free adsorption sites for P in soil, which might prevent P uptake by plants due to rapid P adsorption.
Data Availability Statement
The original contributions presented in the study are included in the article, further inquiries can be directed to the corresponding author.
Author Contributions
AK, TK, KK, RM, and BG designed the experiment. AK and FS conducted the experiment. MS and MW analyzed phosphatase activity. FS and AK analyzed all other data. AK wrote the manuscript with contributions from all authors.
Funding
The study was carried out in the framework of the priority program 1685 “Ecosystem Nutrition: Forest Strategies for limited Phosphorus Resources” funded by the German Federal Ministry of Education and Research (DFG, MI 1377/7-2). We further acknowledge the financial support within the funding program “Open Access Publishing” by the DFG.
Conflict of Interest
The authors declare that the research was conducted in the absence of any commercial or financial relationships that could be construed as a potential conflict of interest.
Acknowledgments
We acknowledge Simon Clausing for ordering beech saplings from the nursery and sharing his expertise on mycorrhiza. We thank Sonia Meller for practical advice during planning of the experiment. We thank Prof. Dr. Edgar Peiter for the allowance to use the greenhouse chamber and Dr. Margret Köck for her technical assistance. We are thankful for the scientific and technical support during the greenhouse experiment by Dr. Bastian Meier. We thank Alexandra Boritzki, Christine Krenkewitz, Miriam Kempe, and Christian Treptow for their work in the greenhouse and laboratory.
References
Andrino, A., Boy, J., Mikutta, R., Sauheitl, L., and Guggenberger, G. (2019). Carbon investment required for the mobilization of inorganic and organic phosphorus bound to goethite by an arbuscular mycorrhiza (Solanum lycopersicum x Rhizophagus irregularis). Front. Environ. Sci. 7:26. doi: 10.3389/fenvs.2019.00026
Barber, S. A. (1995). Soil nutrient Bioavailability: A Mechanistic Approach. New York, NY: John Wiley & Sons.
Brucker, E., Kernchen, S., and Spohn, M. (2020). Release of phosphorus and silicon from minerals by soil microorganisms depends on the availability of organic carbon. Soil Biol. Biochem. 143:107737. doi: 10.1016/j.soilbio.2020.107737
Cornell, R. M., and Schwertmann, U. (2003). The Iron Oxides: Structure, Properties, Reactions, Occurrences and Uses. Hoboken, NJ: John Wiley & Sons.
Côté, B., Fyles, J. W., and Djalilvand, H. (2002). Increasing N and P resorption efficiency and proficiency in northern deciduous hardwoods with decreasing foliar N and P concentrations. Ann. For. Sci. 59, 275–281. doi: 10.1051/forest:2002023
D’Amico, M., Almeida, J. P., Barbieri, S., Castelli, F., Sgura, E., Sineo, G., et al. (2020). Ectomycorrhizal utilization of different phosphorus sources in a glacier forefront in the Italian Alps. Plant Soil 446, 81–95. doi: 10.1007/s11104-019-04342-0
Darch, T., Blackwell, M. S., Hawkins, J., Haygarth, P. M., and Chadwick, D. (2014). A meta-analysis of organic and inorganic phosphorus in organic fertilizers, soils, and water: implications for water quality. Crit. Rev. Environ. Sci. Technol. 44, 2172–2202. doi: 10.1080/10643389.2013.790752
George, T. S., Hinsinger, P., and Turner, B. L. (2016). Phosphorus in Soils and Plants–Facing Phosphorus Scarcity. Berlin: Springer.
German, D. P., Weintraub, M. N., Grandy, A. S., Lauber, C. L., Rinkes, Z. L., and Allison, S. D. (2011). Optimization of hydrolytic and oxidative enzyme methods for ecosystem studies. Soil Biol. Biochem. 43, 1387–1397. doi: 10.1016/j.soilbio.2011.03.017
Göttlein, A. (2015). Grenzwertbereiche für die ernährungsdiagnostische Einwertung der Hauptbaumarten Fichte, Kiefer, Eiche, Buche. Allg For. Jagdztg 186, 110–116.
Hauenstein, S., Neidhardt, H., Lang, F., Krüger, J., Hofmann, D., Pütz, T., et al. (2018). Organic layers favor phosphorus storage and uptake by young beech trees (Fagus sylvatica L.) at nutrient poor ecosystems. Plant Soil 432, 289–301. doi: 10.1007/s11104-018-3804-5
Herold, N., Schöning, I., Berner, D., Haslwimmer, H., Kandeler, E., Michalzik, B., et al. (2014). Vertical gradients of potential enzyme activities in soil profiles of European beech, Norway spruce and Scots pine dominated forest sites. Pedobiologia 57, 181–189. doi: 10.1016/j.pedobi.2014.03.003
Hinsinger, P. (2001). Bioavailability of soil inorganic P in the rhizosphere as affected by root-induced chemical changes: a review. Plant Soil 237, 173–195. doi: 10.1023/A:1013351617532
Hinsinger, P., Herrmann, L., Lesueur, D., Robin, A., Trap, J., Waithaisong, K., et al. (2018). Impact of roots, microorganisms and microfauna on the fate of soil phosphorus in the rhizosphere. Annu. Plant Rev. Online 377–407. doi: 10.1002/9781119312994.apr0528
Hofmann, K., Heuck, C., and Spohn, M. (2016). Phosphorus resorption by young beech trees and soil phosphatase activity as dependent on phosphorus availability. Oecologia 181, 369–379. doi: 10.1007/s00442-016-3581-x
Javaid, A. (2009). Arbuscular mycorrhizal mediated nutrition in plants. J. Plant Nutr. 32, 1595–1618. doi: 10.1080/01904160903150875
Jonard, M., Fürst, A., Verstraeten, A., Thimonier, A., Timmermann, V., Potočić, N., et al. (2015). Tree mineral nutrition is deteriorating in Europe. Global Change Biol. 21, 418–430. doi: 10.1111/gcb.12657
Jones, D. L. (1998). Organic acids in the rhizosphere – a critical review. Plant and Soil 205, 25–44. doi: 10.1023/A:1004356007312
Jones, D. L., and Oburger, E. (2011). “Solubilization of phosphorus by soil microorganisms,” in Phosphorus in Action. Biological Processes in Soil Phosphorus Cycling, eds E. K. Bünemann, A. Oberson, and E. Frossard, (Berlin: Springer-Verlag), 169–198. doi: 10.1007/978-3-642-15271-9_7
Klotzbücher, A., Kaiser, K., Klotzbücher, T., Wolff, M., and Mikutta, R. (2019). Testing mechanisms underlying the Hedley sequential phosphorus extraction of soils. J. Plant Nutr. Soil Sci. 182, 570–577. doi: 10.1002/jpln.201800652
Lambers, H., Raven, J. A., Shaver, G. R., and Smith, S. E. (2008). Plant nutrient-acquisition strategies change with soil age. Trends Ecol. Evol. 23, 95–103. doi: 10.1016/j.tree.2007.10.008
Lang, F., Bauhus, J., Frossard, E., George, E., Kaiser, K., Kaupenjohann, M., et al. (2016). Phosphorus in forest ecosystems: new insights from an ecosystem nutrition perspective. J. Plant Nutr Soil Sci. 179, 129–135. doi: 10.1002/jpln.201500541
Lang, F., Krüger, J., Amelung, W., Willbold, S., Frossard, E., Bünemann, E., et al. (2017). Soil phosphorus supply controls P nutrition strategies of beech forest ecosystems in Central Europe. Biogeochemistry 136, 5–29. doi: 10.1007/s10533-017-0375-0
Leuschner, C., Meier, I. C., and Hertel, D. (2006). On the niche breadth of Fagus sylvatica: soil nutrient status in 50 Central European beech stands on a broad range of bedrock types. Ann. For. Sci. 63, 355–368. doi: 10.1051/forest:2006016
Li, M., Osaki, M., Honma, M., and Tadano, T. (1997). Purification and characterization of phytase induced in tomato roots under phosphorus-deficient conditions. Soil Sci. Plant Nutr. 43, 179–190. doi: 10.1080/00380768.1997.10414726
Margalef, O., Sardans, J., Fernández-Martínez, M., Molowny-Horas, R., Janssens, I. A., Ciais, P., et al. (2017). Global patterns of phosphatase activity in natural soils. Sci. Rep. 7, 1–13. doi: 10.1038/s41598-017-01418-8
Marschner, P., Crowley, D., and Rengel, Z. (2011). Rhizosphere interactions between microorganisms and plants govern iron and phosphorus acquisition along the root axis – model and research methods. Soil Biol. Biochem. 43, 883–894. doi: 10.1016/j.soilbio.2011.01.005
Marx, M.-C., Wood, M., and Jarvis, S. C. (2001). A microplate fluorimetric assay for the study of enzyme diversity in soils. Soil Biol. Biochem. 33, 1633–1640. doi: 10.1016/S0038-0717(01)00079-7
Mehra, O. P., and Jackson, M. L. (1960). Iron oxide removal from soils and clay by a dithionite-citrate system buffered with sodium bicarbonate. Clays Clay Minererals 7, 317–327. doi: 10.1016/B978-0-08-009235-5.50026-7
Meller, S., Frossard, E., and Luster, J. (2019). Phosphorus allocation to leaves of beech saplings reacts to soil phosphorus availability. Front. Plant Sci. 10:744. doi: 10.3389/fpls.2019.00744
Merlin, A., Rosolem, C. A., and He, Z. (2016). Non-labile phosphorus acquisition by Brachiaria. J. Plant Nutri. 39, 1319–1327. doi: 10.1080/01904167.2015.1109117
Netzer, F., Schmid, C., Herschbach, C., and Rennenberg, H. (2017). Phosphorus-nutrition of European beech (Fagus sylvatica L.) during annual growth depends on tree age and P-availability in the soil. Environ. Exp. Bot. 137, 194–207. doi: 10.1016/j.envexpbot.2017.02.009
Odum, E. P. (1969). The strategy of ecosystem development. Science 164, 267–270. doi: 10.5822/978-1-61091-491-8_20
Olsen, S. R., Cole, C. V., Watanabe, F. S., and Dean, L. A. (1954). Estimation of Available Phosphorus in Soils by Extraction with Sodium Bicarbonate. Washington, DC: USDA.
Ordoñez, Y. M., Fernandez, B. R., Lara, L. S., Rodriguez, A., Uribe-Vélez, D., and Sanders, I. R. (2016). Bacteria with phosphate solubilizing capacity alter mycorrhizal fungal growth both inside and outside the root and in the presence of native microbial communities. PLoS One 11:e0154438. doi: 10.1371/journal.pone.0154438
Parfitt, R. L. (1979). The availability of P from Phosphate-goethite bridging complexes. Desorption and uptake by ryegrass. Plant Soil 53, 55–65. doi: 10.1007/BF02181879
Pastore, G., Kaiser, K., Kernchen, S., and Spohn, M. (2020). Microbial release of apatite- and goethite-bound phosphate in acidic forest soils. Geoderma 370:114360. doi: 10.1016/j.geoderma.2020.114360
Reed, S. C., Townsend, A. R., Taylor, P. G., and Cleveland, C. C. (2010). “Phosphorus cycling in tropical forests growing on highly weathered soils,” in Phosphorus in Action, eds E. Bünemann, A. Oberson, and E. Frossard, (Berlin: Springer), 339–369. doi: 10.1007/978-3-642-15271-9_14
Schwertmann, U. (1964). Differenzierung der Eisenoxide des Bodens durch Extraktion mit Ammoniumoxalat-Lösung. Zeitschrift für Pflanzenernährung Düngung Bodenkunde 105, 194–202. doi: 10.1002/jpln.3591050303
Spohn, M., Zavišić, A., Nassal, P., Bergkemper, F., Schulz, S., Marhan, S., et al. (2018). Temporal variations of phosphorus uptake by soil microbial biomass and young beech trees in two forest soils with contrasting phosphorus stocks. Soil Biol. Biochem. 117, 191–202. doi: 10.1016/j.soilbio.2017.10.019
Talkner, U., Meiwes, K. J., Potočić, N., Seletković, I., Cools, N., De Vos, B., et al. (2015). Phosphorus nutrition of beech (Fagus sylvatica L.) is decreasing in Europe. Ann. For. Sci. 72, 919–928. doi: 10.1007/s13595-015-0459-8
Taylor, J. P., Wilson, B., Mills, M. S., and Burns, R. G. (2002). Comparison of microbial numbers and enzymatic activities in surface soils and subsoils using various techniques. Soil Biol. Biochem. 34, 387–401. doi: 10.1016/S0038-0717(01)00199-7
Uksa, M., Schloter, M., Kautz, T., Athmann, M., Köpke, U., and Fischer, D. (2015). Spatial variability of hydrolytic and oxidative potential enzyme activities in different subsoil compartments. Biol. Fertil. Soils 51, 517–521. doi: 10.1007/s00374-015-0992-5
Vance, C. P., Uhde-Stone, C., and Allan, D. L. (2003). Phosphorus acquisition and use: critical adaptations by plants for securing a nonrenewable resource. New Phytol. 157, 423–447. doi: 10.1046/j.1469-8137.2003.00695.x
Walker, T. W., and Syers, J. K. (1976). The fate of phosphorus during pedogenesis. Geoderma 15, 1–19. doi: 10.1016/0016-7061(76)90066-5
Yang, N., Zavišić, A., Pena, R., and Polle, A. (2016). Phenology, photosynthesis, and phosphorus in European beech (Fagus sylvatica L.) in two forest soils with contrasting P contents. J. Plant Nutr. Soil Sci. 179, 151–158. doi: 10.1002/jpln.201500539
Yi, Y., Huang, W., and Ge, Y. (2008). Exopolysaccharide: a novel important factor in the microbial dissolution of tricalcium phosphate. World J. Microbiol. Biotechnol. 24, 1059–1065. doi: 10.1007/s11274-007-9575-4
Zavišic, A., and Polle, A. (2018). Dynamics of phosphorus nutrition, allocation and growth of young beech (Fagus sylvatica L.) trees in P-rich and P-poor forest soil. Tree Physiol. 38, 37–51. doi: 10.1093/treephys/tpx146
Zhang, Y.-M., Wu, N., Zhou, G.-Y., and Bao, W.-K. (2005). Changes in enzyme activities of spruce (Picea balfouriana) forest soil as related to burning in the eastern Qinghai-Tibetan Plateau. Appl. Soil Ecol. 30, 215–225. doi: 10.1016/j.apsoil.2005.01.005
Keywords: Lüss forest, mesocosm experiment, goethite-P-association, orthophosphate, P recycling system, phosphorus nutrition, plant-available phosphorus, phytate
Citation: Klotzbücher A, Schunck F, Klotzbücher T, Kaiser K, Glaser B, Spohn M, Widdig M and Mikutta R (2020) Goethite-Bound Phosphorus in an Acidic Subsoil Is Not Available to Beech (Fagus sylvatica L.). Front. For. Glob. Change 3:94. doi: 10.3389/ffgc.2020.00094
Received: 20 May 2020; Accepted: 07 July 2020;
Published: 07 August 2020.
Edited by:
Delphine Derrien, INRA Centre Nancy-Lorraine, FranceReviewed by:
Mark Bakker, Ecole Nationale Supérieure des Sciences Agronomiques de Bordeaux-Aquitaine, FranceFélix Brédoire, University of Wyoming, United States
Jessé Rodrigo Fink, Instituto Federal do Paraná Câmpus Palmas, Brazil
Copyright © 2020 Klotzbücher, Schunck, Klotzbücher, Kaiser, Glaser, Spohn, Widdig and Mikutta. This is an open-access article distributed under the terms of the Creative Commons Attribution License (CC BY). The use, distribution or reproduction in other forums is permitted, provided the original author(s) and the copyright owner(s) are credited and that the original publication in this journal is cited, in accordance with accepted academic practice. No use, distribution or reproduction is permitted which does not comply with these terms.
*Correspondence: Anika Klotzbücher, YW5pa2Eua2xvdHpidWVjaGVyQGxhbmR3LnVuaS1oYWxsZS5kZQ==