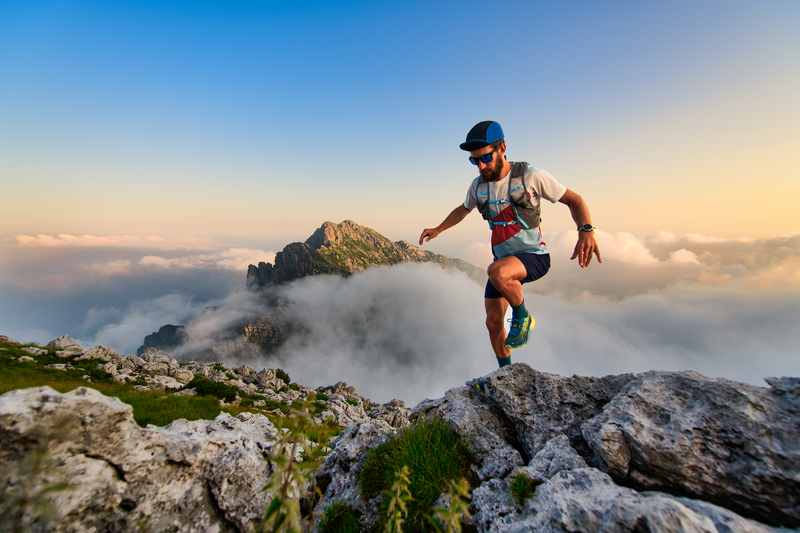
95% of researchers rate our articles as excellent or good
Learn more about the work of our research integrity team to safeguard the quality of each article we publish.
Find out more
ORIGINAL RESEARCH article
Front. For. Glob. Change , 24 June 2020
Sec. Forest Soils
Volume 3 - 2020 | https://doi.org/10.3389/ffgc.2020.00077
This article is part of the Research Topic Changes in Forest Ecosystem Nutrition View all 25 articles
Soil appears to play a key role in the response of the forest ecosystems to N deposition. Twenty years of experimental moderate N addition in a sub-alpine forest increased nitrate leaching, but the soil immobilized most of the N input, gradually decreasing the C:N ratio. Exchangeable and microbial N were only slightly affected, but denitrification and N2O production were increased and soil respiration tended to be reduced while soil microbial communities were remarkably resistant. It is assumed that these changes at the process level are related to the soil microbiome, but soil microbial communities have not been assessed so far at lower taxonomical resolution in this long-term experiment. The aim of this study is to understand the underlying causes of the results obtained so far by assessing how N treatment affects the soil microbiome at different soil depths. We analyzed bacterial and fungal diversity and community structures using Illumina MiSeq sequencing and quantified the responses of the N cycling communities to elevated N loads by quantitative PCR. The microbial functions were assessed by respiration, N mineralization, and potential nitrification. Bacterial and fungal α-diversity, observed richness and Shannon diversity index, remained unchanged upon N addition. Multivariate statistics showed shifts in the structures of fungal but not bacterial communities with N load, while the changes were minor. Differences in the community compositions associated with the N treatment were mainly observed at a lower taxonomical level. We found several fungal OTUs in particular genera such as the ectomycorrhizal fungi Hydnum, Piloderma, Amanita, and Tricholoma that decreased significantly with increased N-loads. We conclude that long-term moderate N addition at this forest site did not strongly affect the soil microbiome (which remained remarkably resistant) and its functioning.
As a result of human activities, forests are exposed to unprecedented levels of N inputs (Bobbink et al., 2010; Schmitz et al., 2019). Biologically reactive N is mainly emitted by fossil fuel combustion or intensification of agriculture, leading to atmospheric deposition over all types of ecosystems even at long distance from sources (Galloway et al., 2008; Simpson et al., 2014). Because N is a key element in ecosystem processes (Nadelhoffer et al., 1999; Galloway et al., 2004), the effects of increased N loads on temperate forests have been intensively studied over the last three decades (e.g., Dise and Wright, 1995; Bredemeier et al., 1998; Emmett et al., 1998; Aber, 2002).
In temperate and boreal forests, reactive N inputs have been shown to alter tree growth and understory biomass, sometimes positively, sometimes negatively, depending on the N status, and other site factors (Solberg et al., 2009; Thomas et al., 2010; Gundale et al., 2014; Forstner et al., 2019a). N deposition can further accelerate soil acidification and base cation loss (Carnol et al., 1997; Högberg et al., 2006; Forstner et al., 2019a), increase N leaching (Carnol et al., 1997; Moldan and Wright, 2011; Schleppi et al., 2017), favor forest nutritional imbalances (Mooshammer et al., 2014; Zechmeister-Boltenstern et al., 2015; Forstner et al., 2019b) and affect the cycling and storage of soil organic C (Treseder, 2008; Janssens et al., 2010; Maaroufi et al., 2015). N deposition can also accelerate microbial soil processes that are part of the N cycle, such as nitrification and denitrification (Gundersen et al., 2012). However, N deposition often reduces the overall soil biological activity as measured by respiration (Janssens et al., 2010; Zhang et al., 2019) or extracellular enzyme activities (Saiya-Cork et al., 2002; Sinsabaugh et al., 2002; DeForest et al., 2004). Frey et al. (2014) reported that organic horizon SOC pools increased by 33 and 52% in hardwood and pine stands, respectively, whereas in mineral horizons SOC pools did not respond to 20 years of N addition treatment (50 kg ha−1 y−1). In two Norway spruce stands, Forstner et al. (2019a) also found an SOC increase in the organic layer, but compensated by a decrease in the mineral soil. The main mechanisms behind the observed increases in topsoil SOC appear to be higher litter inputs to soil via stimulated tree productivity and a slower decomposition of soil organic matter (SOM). There are, however, contradictory reports on the effect of N deposition specifically on the decomposition of less decomposable, recalcitrant SOM (Janssens et al., 2010; Forstner et al., 2019a).
Earlier reports on soils from various forests revealed that N addition reduces microbial biomass and activity (Bowden et al., 2004; Frey et al., 2004; Wallenstein et al., 2006; Demoling et al., 2008; Treseder, 2008). Shifts in fungal:bacterial (F:B) biomass ratios (decrease in fungal biomass with little change in bacterial biomass) indicate alterations in the composition of the microbial community (Wallenstein et al., 2006). The decline in fungal biomass was largely attributed to a decrease in ectomycorrhiza, which form associations with trees, suggesting that fungi are more sensitive to long-term N fertilization than bacteria. Most previous research focused on soil bacterial communities in forests (Shen et al., 2010; Turlapati et al., 2013), and we are aware of only a few studies that examined changes in soil fungal community composition following increased N loads (Boxman et al., 1998; Allison et al., 2007; Edwards et al., 2011; Maaroufi et al., 2019).
Soil microorganisms, regulated by soil N availability, are able to change the terrestrial C cycling by decomposition and formation of soil organic matter (SOM) (Uroz et al., 2016; Baldrian, 2017; Llado et al., 2017). For example, organic substrate with a high N content can be rapidly decomposed by microorganisms at the initial stage, resulting in large accumulation of microbial products and concomitant formation of stable SOM. In contrast, for substrate with a low N content, more C tends to be respired rather than stored as stable SOM (Cotrufo et al., 2013). However, it remains unclear how moderate N addition regulates soil microbial biomass and community composition in forest ecosystems, which constrains our understanding of soil C cycling in response to N deposition. Fungi dominate in the decomposition of the SOM with a low nutrient content, because their nutrient demand and metabolic activity are low compared to bacteria (Mooshammer et al., 2014; Zechmeister-Boltenstern et al., 2015; Zhou et al., 2017). Consequently, N addition may decrease the F:B ratio. Meanwhile, soil acidification induced by N addition is likely to increase the F:B, because fungi are better adapted to environments with high H+ concentration than bacteria (Högberg et al., 2006; Rousk et al., 2010). However, the effect of N addition on soil microbial biomass and community composition remains unclear and the current fragmentary knowledge needs to be improved across temperate forest ecosystems (Forstner et al., 2019b).
The effects of N deposition on forests largely depend upon soil processes such as immobilization, mineralization, nitrification, and denitrification. At the time scale of decades, soils tend to accumulate most of the N inputs (Nadelhoffer et al., 1999), which is reflected in a gradually decreasing soil C:N ratio (Gundersen et al., 1998; Morier et al., 2010; Moldan and Wright, 2011). While these overall processes are well-understood and somehow quantified, the effects on the forest soil microbiome in the context of increased atmospheric N deposition remain very poorly studied, even if microorganisms are the main actors in nutrient cycling and are likely affected by N deposition. Shifts in microbial community diversity and composition through anthropogenic stressors (e.g., atmospheric N deposition) may lead to unpredictable alterations in critical ecosystem processes.
The present study builds on an existing long-term (>20 years) N addition field experiment where atmospheric N deposition is artificially increased by adding small but recurrent amounts of ammonium nitrate (NH4NO3) dissolved in water. This experiment is carried out in the Alptal valley, central Switzerland, and it simulates a moderately increased N deposition (Schleppi et al., 2017). The site is stocked by old Norway spruce trees and the soil type is a relatively nutrient-rich Gleysol. From the previous results of this field study, the soil appears to play a key role in the reaction of the ecosystem to N deposition. The experimental N addition increased nitrate leaching, but, as in the comparable studies mentioned above, the soil immobilized most of the N input, progressively decreasing its C:N ratio (Schleppi et al., 2004). Denitrification and production of N2O were increased and soil respiration tended to be reduced (Mohn et al., 2000; Krause et al., 2013). The abundance and biodiversity of Collembola (feeding mainly on microbes) decreased due to the N treatment (Xu et al., 2009). All these effects are likely linked to modifications of the forest soil microbiome. As one of the few of this kind and duration worldwide, the experiment offers a unique chance to study how N deposition affects microbial communities in natural forest soils in the long term.
Here, we analyzed the soil microbiome in different soil horizons. More specifically, we studied the effects of the long-term N addition on (i) the soil microbial biomass (by fumigation/extraction and as the abundance of bacterial and fungal marker genes), (ii) the microbial diversity, and community structure, and (iii) various aspects of ecosystem functions such as respiration potential, N mineralization and nitrification potential, fine root traits, together with quantifying key functions of the microbiome to increased N loads by quantitative polymerase chain reactions (qPCR). Genes encoding for enzymes catalyzing major processes during N fixation (nifH), nitrification (bacterial amoA, archaeal amoA, nxrB), and denitrification (nirS, nosZ) were targeted. We specificially asked: (1) Do long-term N addition change the microbial communities?; (2) Are these changes evident in different soil layers?; (3) Are community structure shifts represented in altered microbial functions in terms of C and N cycling processes. We hypothesized that chronic N inputs to forest soils alter the soil microbiota compared to soils subjected to ambient deposition only, whereas fungal communities react more strongly than bacterial ones, with symbiotrophs more sensitive than saprotrophs. We further hypothesized that chronic but low N loads induce significant changes in N cycling gene abundances, which might therefore alter nutrient availability of the forest systems.
The present study was conducted in a subalpine Norway spruce forest (Picea abies (L.) Karst.) of the Alptal valley, in Central Switzerland (47°03 N, 8°43 E), at an elevation of 1,200 m a.s.l. Beside Norway spruce, the tree layer features about 15% silver fir (Abies alba Mill). In the herb layer, Vaccinium spp. (L.), Carex spp. (L.), Petasites albus [(L.) Gaertn.], Caltha palustris (L.), Knautia dipsacifolia [(Host) Kreutzer], Chaerophyllum hirsutum (L.), and Lycopodium annotitum (L.) make up most of the biomass. The climate is cool and wet, with a mean air temperature of 6°C and a mean annual precipitation of 2,300 mm. Bulk N deposition measured at the site is 12 kg ha−1 y−1, equally partitioned between and . Throughfall N deposition amounts to 17 kg ha−1 y−1 (Schleppi et al., 1998). According to the scale of Mellert and Göttlein (2012) for foliar concentrations, the nutrition of the Norway spruce can be considered as latent deficient for N and P. For K, Ca, and Mg, concentrations are in the normal range. The parent rock material of the site is Flysch, composed of sedimentary conglomerates with clay-rich schists. The slope is about 20% with a west aspect. Soils are very heavy Gleysols. Because of the high clay content (on average 48%), they have a high cation-exchange capacity but a low permeability. A water table is present throughout the year, on average at a depth of 23 cm (Krause et al., 2013). The pH of the mineral soil increases with depth, from 4.6 to 5.9 on the mounds and from 5.4 to 6.9 in the depressions. Net nitrification rates (measured for of the upper 15 cm) are either positive at some locations, negative at others (N immobilization). The net overall rate is overall not significantly different from zero (Hagedorn et al., 2001b). More details about the experimental site were given previously (Schleppi et al., 2017).
The N addition experiment at Alptal started in 1995. It combines a paired-catchment design (Schleppi et al., 2017) with a replicated plot design (Mohn et al., 2000; Hagedorn et al., 2001a; Xu et al., 2009; Krause et al., 2013). The ten 20-m2 plots of this latter design were sampled for this study. Each of five N-treated plots was paired with a nearby control plot of similar micro-topography and vegetation. Nitrogen was added to treated plots by sprinkling of NH4NO3 dissolved in rainwater (Schleppi et al., 2017) during precipitation events. Hence, N addition varied annually with local precipitation regime, amounting to an average of 22 kg N ha−1 y−1 (Schleppi et al., 2017). Control plots received the same amount of unaltered rainwater. In winter, automatic irrigation was replaced by the occasional application of concentrated NH4NO3 solution on top of the snowpack using a backpack-sprayer.
We sampled soils in 2014 (September) and 2015 (June, September). Three intact soil cores, 5 cm in diameter and 25 cm deep, were taken from each plot, placed on ice and transported to the laboratory. Within 24 h, each core was sectioned into three soil horizons and these horizons were pooled over the three cores from a single plot. From the top to the bottom of the cores, these layers were on average 4.5 (A horizon), eight (oxic B horizon), and nine (reduced B horizon) cm in thickness. The litter layer (L) was separated. At the time of sampling (September), before the main needle fall of the spuce trees and before the decay of the annual plant species, there was few litter (0.06 g cm−2 on average) on the ground. In this season, most of the litter is almost decomposed and integrated in the A horizon. For this reason, the L layer was not further considered in the present study. This sampling scheme thus yielded a total of 30 samples (2 treatments × 3 horizons × 5 replicates). Samples were homogenized by passing through a 2-mm sieve. Fine roots (<2 mm) were removed from sieved soils using forceps. Aliquots of field-moist soil were stored at 4°C up to 2 weeks for analysis of microbial activities. Subsamples were stored at −18°C until analysis of DNA. Gravimetric water content was determined from subsamples dried to constant mass at 65°C. Soil C and N concentrations were measured from milled soil material using an elemental analyzer (Euro-EA, Hekatech GmbH, Germany) coupled to a continuous flow isotope ratio mass spectrometer (Delta-V Advanced IRMS, Thermo GmbH, Germany).
After removing fine roots from soils, they were washed under rinsing demineralized water and stored in plastic bags at 4°C up to 2 weeks for analysis of fine-root traits. Firstly, the fine roots were scanned using a scanner, and the scanned pictures were analyzed using the WinRHIZO software package (version 4.1c, Regent Instruments Inc., Québec, Canada) for morphological and architectural traits such as length, average diameter, and number of tips. Then the fine roots were dried at 60°C for 3 days, weighed, and milled. Fine-root biomass was calculated per soil volume to obtain comparable data. Specific root length (SRL; m g−1) was calculated by combining scanned data with biomass data, and tip frequency (cm−1) was calculated from scanned data (Brunner et al., 2019). Fine-root C and N concentrations were measured from milled root material using an elemental analyzer-continuous flow isotope ratio mass spectrometer (Euro-EA, Hekatech GmbH, Germany, interfaced with a Delta-V Advanced IRMS, Thermo GmbH, Germany).
Potential nitrification was determined using the shaken slurry method (Hart et al., 1994). 10 g of field-moist, sieved soil were shaken in 100 ml of a solution (pH 7.2) containing 1 mM and 15 mM at 22°C in the dark. These conditions ensured a maximum production rate with minimal N immobilization and denitrification. Homogenized sub-samples (15 ml of the slurry) were taken at 2, 6, 23.5, 26, and 29 h after the start of the incubation. These sub-samples were filtered and stored at −20°C until analysis. -N was determined colorimetrically with a continuous flow analyzer (Auto-Analyzer 3, BranLuebbe, Germany). Nitrification rates were calculated by linear regression of -N concentrations over time.
Net nitrogen mineralization was measured through a 28-days aerobic laboratory incubation of 15 g soil at constant temperature (22°C) in the dark (Hart et al., 1994). At the beginning and at the end of the incubation, inorganic N was extracted with a 1 M KCl solution (1:5 w:v) (Allen, 1989) and analyzed as described above. The mineralization rate was calculated from the net increase in inorganic N (-N and -N) during the incubation period.
The respiration potential was measured according to Robertson et al. (1999) as the CO2 accumulation in the headspace (250 ml) of an amber bottle containing 20 g fresh soil, at 22°C in the dark after an overnight pre-incubation. Gas samples (4 ml) were taken at 0, 120, 150, and 180 min with an airtight syringe and analyzed with an infrared gas analyzer (EGM-4, PP Systems, UK). The respiration rate was estimated by linear regression of these measurements against time.
Soil microbial biomass C and N (MBC, MBN) were determined by the chloroform fumigation extraction method (Vance et al., 1987). Fumigations were carried out for 3 days in a vacuum desiccator with alcohol-free chloroform, followed by 0.5 M K2SO4 extraction. Dissolved organic C was measured with a Total Organic Carbon Analyzer (Labtoc, Pollution and Process Monitoring Ltd, UK) and total N was measured colorimetrically as described above. Soil MBC and MBN were calculated from the difference of total extract between fumigated and unfumigated samples, with a conversion factor of 0.45 for MBC (Jenkinson et al., 2004) and 0.54 for MBN (Joergensen and Mueller, 1996).
Water-soluble C and N were extracted from 10 g fresh sieved (4 mm) soil, following Ghani et al. (2003). First, readily soluble C and N (water soluble C and N) were extracted at room temperature with 30 ml distilled water. After 30 min agitation at 2 Hz, the suspension was centrifuged for 10 min (3,000 min−1) and the supernatant filtered at 0.45 μm (GN-6 Metricel, Pall Corporation, US). Total organic C was measured with a Total Organic Carbon analyzer (Labtoc, Pollution and Process Monitoring Ltd, UK). Total N, N, and -N were measured colorimetrically as described above. Organic N was calculated by subtracting -N and -N from total N. After this first extraction step, labile components of soil C were extracted at 80°C (hot water extractable C and N). The centrifuge tube with the remaining wet soil was weighted to calculate the remaining water volume, and a further 60 ml distilled water was added. Samples were shaken for 30 min to re-suspend the soil, closed and placed in a pre-heated oven at 80°C for 16 h. The samples were then shaken again for 30 min, centrifuged, filtered, and analyzed as explained above. As water soluble fractions contains mineral N initially present in soils, water soluble C:N ratios were calculated as water-soluble C divided by water-soluble organic N. As initially present mineral N is mostly removed in the first extraction step, and as in hot water extracts may result from the hydrolysis of organic N (Gregorich et al., 2003), hot water C:N ratios were calculated as hot-water-soluble C divided by hot-water-soluble total N.
Total DNA was extracted from ~0.5 g soil using the PowerSoil DNA Isolation Kit (Qiagen). DNA was quantified using the high sensitivity Qubit assay (Thermo Fisher Scientific). The V3–V4 region of the bacterial small-subunit (16S) rRNA gene and the internal transcribed spacer region 2 (ITS2) of the eukaryotic (fungal groups, some groups of protists, and green algae) ribosomal operon were PCR amplified using primers previously described by Frey et al. (2016). PCR amplification was performed with 20 ng soil DNA and the HotStar Taq amplification kit (Qiagen, Hilden, Germany) in a final volume of 50 μL per samples (16S: 15 min at 95°C/30 cycles: 40 s at 94°C, 40 s at 58°C, 1 min at 72°C/10 min at 72°C; ITS-2: 15 min at 95°C/36 cycles: 40 s at 94°C, 40 s at 58°C, 1 min at 72°C/10 min at 72°C). PCRs were run in triplicates, pooled, and purified using Agencourt Ampure XP beads (Beckman Coulter). Bacterial and fungal amplicon pools were sent to the Génome Québec Innovation Center at McGill University (Montreal, Canada) for barcoding using the Fluidigm Access Array technology and paired-end sequencing on the Illumina MiSeq v3 platform (Illumina Inc.). Raw sequences have been deposited in the NCBI Sequence Read Archive under the BioProject accession number PRJNA595488.
Quality filtering, clustering into operational taxonomic units (OTUs) and taxonomic assignments were performed as previously described in Frey et al. (2016). In brief, a customized pipeline largely based on UPARSE (Edgar, 2013; Edgar and Flyvbjerg, 2015) implemented in USEARCH (v9.2; Edgar, 2010) was used. Filtered reads were de-replicated and singleton reads removed prior to clustering. Sequences were clustered into OTUs at 97% sequence identity (Edgar, 2013). For taxonomic classification of the OTUs, corresponding centroid sequences were queried against selected reference databases using the naïve Bayesian classifier (Wang et al., 2007) and a minimum bootstrap support of 80%. Prokaryotic sequences were queried against the SILVA database (v132; Quast et al., 2013). Eukaryotic ITS2 sequences were first queried against a custom-made ITS2 reference database retrieved from NCBI GenBank, and sequences assigned to fungi were subsequently queried against the fungal ITS database UNITE (v8.0; Abarenkov et al., 2010). Prokaryotic centroid sequences identified as originating from organelles (chloroplast, mitochondria), as well as eukaryotic centroid sequences identified as originating from soil animals (Metazoa), plants (Viridiplantae, except green algae), or of unknown eukaryotic origin, were removed prior to data analysis.
Relative abundances of the bacterial 16S rRNA genes, fungal ITS, and various C- and N-cycling genes were determined as previously by quantitative PCR as previously described (Frey et al., 2011; Rime et al., 2016) using an ABI7500 Fast Real-Time PCR system (Applied Biosystems). The same primers (without barcodes) and cycling conditions as for the sequencing approach were used for the 16S and ITS (Frossard et al., 2018). For qPCR analyses 2.5 ng DNA in a total volume of 25 μl containing 0.5 μM of each primer, 0.2 mg of BSA ml−1, and 12.5 μl of QuantiTect SYBR Green PCR master mix (Qiagen, Hirlen, Germany) were used. Abundances of C- and N-cycling genes were quantified using primers and thermocycling conditions as reported in Table S1. Functional marker genes encoding for enzymes catalyzing major processes during methanogenesis (mcrA) nitrogen fixation (nifH), nitrification (bacterial amoA, archaeal amoA, nxrB), and denitrification (nirS, nosZ) are targeted. The specificity of the amplification products was confirmed by melting-curve analysis, and the expected sizes of the amplified fragments were checked in a 1.5% agarose gel stained with ethidium bromide. Three standard curves per target region (correlations ≥ 0.997) were obtained using tenfold serial dilutions (10−1-10−9 copies) of plasmids generated from cloned targets (Frey et al., 2011). Data was converted to represent average copy number of targets per μg DNA.
All statistical analyses were performed using R (v.3.6.0; R Core Team, 2017). Variables were tested for normality and homogeneity of variances using Shapiro-Wilk and Levene's tests, respectively. In case of non-normality and/or heteroscedasticity, the data were transformed by either by taking the natural logarithm or by using the Box-Cox family of power transformations. We used analysis of variance (ANOVA) to test for effects of N addition treatment, soil horizon, and their interaction on univariate response variables. Post-hoc differences between soil horizons were assessed with Tukey's HSD tests, and Dunnett's tests were used to check for treatment effects within horizons.
For analysis of bacterial and fungal α-diversities, observed richness (number of OTUs) and Shannon diversity index were estimated based on OTU abundance matrices rarefied to the lowest number of sequences using the R package phyloseq (v1.28.0; McMurdie and Holmes, 2013). To assess the main and interactive effects of N treatment and soil horizon on α-diversities a two-way ANOVA was performed. Pairwise comparisons of significant effects were conducted using Tukey's HSD post-hoc tests.
Bray-Curtis dissimilarities were calculated based on square root transformed relative abundances of OTUs (Hartmann et al., 2017). The effects of N treatment, soil horizon, and interactive effects on microbial community structures (ß-diversities) were assessed by conducting a permutational ANOVA (PERMANOVA, number of permutations = 9,999) with the function adonis implemented in the vegan package (v2.5.5; Oksanen et al., 2017). Canonical analysis of principal coordinates (CAP) ordinations of microbial community structures were calculated using the ordinate function implemented in the R package phyloseq.
Changes in the relative abundances of the most abundant phyla (classes, orders) were assessed by conducting a two-way analysis of variance (ANOVA). Differences were considered significant at p < 0.05 unless mentioned otherwise. To identify microbial genera that were significantly different between N amended and control samples we first agglomerated OTUs to the genus level, and generated subsets for each soil horizon. Differential abundance analysis by applying a negative binomial generalized linear model to the OTU count data using the DESeq2 package (v.1.24.0; Love et al., 2014) was performed. Genera were considered significantly different (Wald test) between N-treated and control samples if the false discovery rate (adjusted p-value) was < 0.05. Fungal functional guilds were assigned within the six most abundant guilds, namely ectomycorrhizal fungi, arbuscular mycorrhizal, endophyte, undefined saprotrophs, animal pathogens, and plant pathogens, using an open annotation tool (FUNGuild) according to Nguyen et al. (2016). Only the guild assignment with “highly probable” confidence rankings was accepted.
Overall, soil properties and processes showed only a small impact of two decades of N addition. The total C and N concentrations clearly declined with depth but were not significantly affected by the treatment (Table 1). The N addition, however, significantly decreased the soil C:N ratio by about two in all three layers. Hot water extractable C and N (Figure 1) were also very similar in the N-addition plots compared to the control plots, and concentrations of both elements clearly decreased with depth. C:N ratios in the extractable organic matter showed no effects at all, except that the ratio was lower in the hot-water than in the cold-water extract. Water soluble and hot water extractable was not significantly affected either, even if the cumulated addition of -N amounted to 220 g m−2, which would represent 250 mg g−1 if it would be homogenously mixed into the 25 cm depth of the soil cores. There was a significant interaction of N addition treatment and horizon on water soluble and hot water extractable , with higher values under N inputs in the A-horizon (p < 0.001). In lower layers, the tendency was even opposite pointing to reducing conditions in lower layers.
Table 1. Biotic and abiotic site characteristics of soil horizons (A, Bo, Br) in the control and N-added plots of the Alptal N addition experiment.
Figure 1. Extractable C and N concentrations in the horizons of the mineral soil in the control and N-addition plots. A first extraction was done with cold water and a subsequent one with hot water. All results are from three cores per plot and given per dry soil mass as averages ± standard error (n = 5; A, A horizon; Bo, oxic B horizon; Br, reduced B horizon).
Microbial biomass C and N (Table 1) decreased with depth (p < 0.001) with a tendency of less (p = 0.062) microbial biomass C in N-treated plots, resulting in a lower microbial C:N ratio in the top soil layer (0–4.5 cm) with N addition (interaction N × horizon: p = 0.007). Respiration and N mineralisation (Table 1) strongly decreased with soil depth (p < 0.001). No effect of the N treatment could be detected, neither if process rates were expressed per soil dry mass nor per total or extractable C or N. We also found no significant interactions between horizons and N addition. Potential nitrification did not change with N addition nor with soil depth (Table 1).
In contrast to microbial biomass C, DNA content was significantly lower under N treatment (p = 0.003) and decreased with soil depth (p < 0.001; Table 1). Bacterial and fungal abundance (16S and ITS copy numbers) as well as the seven C- and N-cycling genes were not affected by the N addition (Table 1, Figure 2). Similarly, we found that the abundance of fungal biomass relative to bacterial (F:B ratio) did not change with the addition of N. There was a tendency of higher nrxB (p = 0.06) and nifH (p = 0.08) genes in plots with N inputs. Three functional genes (nrxB, nirS, and nifH) significantly increased with soil depth (Table 1, Figure 2).
Figure 2. DNA content and abundances of bacterial (16S), fungal (ITS2), and C- and N-cycling genes in the horizons of the mineral soil in the control and N-addition plots (n = 5; A, A horizon; Bo, oxic B horizon; Br, reduced B horizon). Gene copies (as log10) were given per μg DNA.
N addition had no effect on fine root traits of Norway spruce. Fine root biomass, morphology (specific root length, tips, and diameter) and root chemistry (C, N) did not respond significantly to N addition (Table 1).
Since the results of the community analyses were similar for the three sampling times (with no or only minor changes in the soil microbiome), we decided to present only the analyses from the last sampling (September 2015). The overall microbial community analysis has been documented in the Supplementary Results. Bacterial α-diversity indices in control and N-added soils were unchanged (p = 0.9; Table 2, Figure 3). Bacterial α-diversity was also similar between different horizons under control and experimental N deposition (Table 2, Table S2). In contrast, we found a significant decline (p = 0.05) of fungal α-diversity indices (Richness and Shannon Index) with soil depth (Table 2, Figure 3), while neither effects of treatment (N addition) nor interactions (N × depth) on fungal α-diversity indices were recorded (Table 2). Among the fungal populations we found that animal pathogen (35%), ectomycorrhizal (30%), saprotroph (12%), arbuscular mycorrhizal (10%), endophyte (8%), and plant pathogen (2%) were the dominant functional guilds with a “highly probable” classification (Nguyen et al., 2016). Overall, there was a decrease of richness with N addition with strongest (p < 0.001; data not shown) impact on ectomycorrhizal, arbuscular mycorrhizal fungi, and endophytes (Figure 4). PERMANOVA analysis revealed a weak (p = 0.06) but not significant difference in fungal ß-diversities with N addition (Table 2) as also shown by canonical analysis of principal coordinates (CAP) based on the Bray-Curtis dissimilarities (Figure 5). In contrast, bacterial community structures were not influenced by N addition and soil depth.
Table 2. Treatment (N addition) and spatial (horizon) effects on α-diversity (Richness and Shannon Index) and ß-diversity (Bray-Curtis dissimilarities) in the Alptal N addition experiment.
Figure 3. Variation in Richness and Shannon index of bacterial (A) and fungal (B) communities in the horizons of the mineral soil in the control and N-addition plots (n = 5; A, A horizon; Bo, oxic B horizon; Br, reduced B horizon).
Figure 4. Patterns of α-diversity (Richness) of the different fungal guilds in the horizons of the mineral soil in the control and N-addition plots (n = 5; A, A horizon; Bo, oxic B horizon; Br, reduced B horizon). Fungal functional guilds were analyzed by FUNGuild showing the six most abundant guilds. Only the guild assignment with “highly probable” confidence rankings was accepted.
Figure 5. Canonical analysis of principal coordinates (CAP) based on the Bray-Curtis dissimilarities for bacterial (A) and fungal (B) communities in the horizons of the mineral soil in the control and N-addition plots (n = 5; A, A horizon; Bo, oxic B horizon; Br, reduced B horizon). The variation in microbial community structures was explained by each CAP axis (for bacteria: CAP 1, 5.2%; CAP 2, 3.9%; for fungi: CAP 1, 15.5%; CAP 2, 9.6%).
The relative abundance of bacterial phyla did not change between the ambient and experimental N deposition. No bacterial phyla and classes exhibited a significant change in relative abundance with the N treatment. However, we found significant (p < 0.05) depth effects in the relative abundance of OTUs attributable to Anaerolineae (Table 3). Within fungi, the phylum Basidiomycota significantly decreased in relative abundance with N (Table 3). The other fungal phyla did not exhibit significant changes with the experimental N deposition treatment. A few fungal classes and orders were affected by the experimental N deposition treatment. Relative abundance of Agaricomycetes, Agaricales, and Cantharellales decreased (p < 0.05) whereas the relative abundance of Heliotales increased (p < 0.1) under experimental N addition.
Table 3. Treatment (N addition) and spatial (horizon) effects on the relative abundance of bacteria and fungi in the Alptal N addition experiment.
As bacterial and fungal phyla consist of various heterogeneous groups, we also investigated the changes in the differential abundance of the most common bacterial and fungal genera in response to N treatment among the three soil horizons. Since we did not find any taxa with significant (p < 0.05) log2 fold change in bacteria, we report on those that responded weakly (p < 0.1) to N addition (Figure S1). The majority of genera that had positive log2 fold changes upon N treatment belonged to the phylum Proteobacteria. Within this phylum, Rhodoblastus and the sulfur-oxidizing genus Sulfurifustis exhibited the strongest positive response to N (increase with N addition). In addition, the methane-producing Methanocella and Methanolinea showed higher positive log2 fold changes, indicating anaerobic soil conditions (Figure S1). The greatest negative log2 fold change in response to N addition was exhibited by genera of the phylum Acidobacteria such as the acidophilic Granulicella and Acidipila. Genera of the phylum Proteobacteria also decreased with N deposition, with Rickettsiella and Inquilinus having overall the greatest negative log2 fold change (Figure S1).
Within the fungal kingdom, the majority of genera that exhibited either positive or negative log2 fold changes were members of the phyla Ascomycota and Basidiomycota (Figure 6, Figure S2). The genera Clavulina and Physisporinus had the strongest positive responses to the N addition (Figure 6). Clavulina is an ectomycorrhizal fungus (Basidiomycota) with some saprophytic lifestyle and Physisporinus is a wood saprotroph (Ascomycota). Interestingly, fungal entomopathogens such as Metarhizium and Trichoderma (both Ascomycota) showed negative log2 fold changes upon treatment with N. Genera belonging to Basidiomycota, such as the ectomycorrhizal fungi Membranomyces, Thelephora, Hydnum, Piloderma, and Amanita were found to decrease in N-treated samples (Figure 6, Figure S2). Membranomyces had the largest negative log2 fold change followed by Thelephora. Ectomycorrhizal fungi showed a mixed response with Thelephora declined significantly with N fertilization, while Clavulina increased.
Figure 6. Differently abundant fungal genera (statistically different p < 0.05) which respond to N treatments across all soil horizons with a log2-fold change > 1.0. Negative values (blue) refer to Control-upregulated genera, positive values (red) to N-Addition-upregulated genera. Bar diagrams on the right shows the relative abundance.
There are only a few long-term (20 years and more) N addition experiments in forest ecosystems worldwide. They all have one result in common: from an annual up to a decadal scale, added N is mainly sequestered into the soil (Cheng et al., 2019). Higher deposition rates therefore lead to an accumulation of N in the soil, with potentially effects on soil organisms and the biochemical processes that they drive. Such effects are not limited to the N cycle, but also affect C and other elements.
In the present study we did not find any significant effect of the treatment or interaction thereof with the mineral soil layers. Similarly, for soils from the same site, Forstner et al. (2019a) also reported no significant differences in C mineralization rates with N treatment. Some studies showed an increase in forest soil respiration as a result of N addition (Hasselquist et al., 2012; Zhang et al., 2019), but in other reports there was no effect (e.g., Haynes and Gower, 1995; Bowden et al., 2000; Liu et al., 2017). Based on 5 years measurements with static chambers, Krause et al. (2013) found only a weak tendency of soil respiration rates to be reduced by N addition. At our site, more C tended to accumulate in the organic layer of N-addition plots, but C was decreased in the mineral soil (Forstner et al., 2019a). At the same time, the input of litter remained in the same range as for control (Krause et al., 2013). This means that neither the soil C pool nor its main fluxes in and out of the soil were significantly affected by the N treatment. Therefore, despite theoretically large potential effects, our results can only confirm that N deposition effects in relation to the C balance of forest soils are minor (Erisman et al., 2011).
At our site, in our results as well as in the previous study of Schleppi et al. (2004), the C:N ratio of the mineral soil significantly decreased as a result of the N addition. As shown by Providoli et al. (2006) using 15 or 15, most of the N from deposition entering the soil is rapidly immobilized and bound to its organic matter. This explains why the extractable inorganic N was not increased in the present study. Compared to the control plots, we observed a clear increase of extractable in the A-horizon of the N addition plots. However, in relation to the annual added, this represents only about one-tenth of the total. Due to the high mobility of this ion, this measurement can anyway only be considered as a snapshot. Together with the extractable , it indicates that only very little N as inorganic N is present in the soils (i.e., very little in a form that is directly available to plants and microbes).
Interestingly, no significant effects by long-term moderate N addition on any of the fine root traits of Norway spruce investigated have been observed, which is in accordance with Carnol et al. (1999). This is in contrast to what could be expected when N is added to an ecosystem (Ostonen et al., 2007; Li et al., 2015). Li et al. (2015) observed in a meta-analysis on simulated N deposition an overall significant increase of the total root biomass, with the coarse roots increasing and the fine roots decreasing significantly. In addition, they observed a significant increase of the root N concentration. However, a few fine-roots traits seemed not to be affected by N addition, in particular fine root length and diameter (Li et al., 2015). Ostonen et al. (2007) observed in their meta-analysis a significant decrease of the specific root length, whereas Li et al. (2015) did not observe any significant change of this parameter. In our study, despite the fact that we did not observe any change of biomass or N concentrations in the fine roots, needle dry weight as well as needle N concentration increased significantly from the 4th year of the N addition (Krause et al., 2012). This indicates that some additional N is indeed available to tree roots but that it was transported to aboveground tree compartments. The fact that none of the fine-root traits significantly responded to the N addition is likely related to other factors limiting root growth, especially the anoxic conditions that prevail most of the time in the lower gleyic layers of the soil. Combined with waterlogging, this can indeed strongly limit root growth and, subsequently, the uptake of N (Fan et al., 2017).
Soil microbial communities were remarkably resistant to long-term N addition. Although there have been several studies examining the effects of N deposition on microbial communities across ecosystems (Treseder, 2008; Zhou et al., 2017), ours is one of the few that comprehensively describes the long-term (>20 years) effects of moderate N addition on bacterial and fungal diversity and community structures in forest soils. Overall, the soil microbiome was relatively resistant and responded only weakly to long-term moderate N addition at our forest site. In the long-term soil microbial communities seem to be very resilient to environmental change or disturbance and adapt to new environmental conditions (Hartmann et al., 2014; Frossard et al., 2018). Another reason for the weak response of the soil microbiome might be that the added N is easily available and soluble, so it can either be washed out, or readily taken up by ectomycorrhizal fungi and roots and transported to the aboveground parts or readily immobilized in the soil (Schleppi et al., 2004; Providoli et al., 2006). Since N is not a limiting factor for the belowground communities here, more N cannot be truly used for more growth (microbial biomass). Another factor limiting the responses of N to the microbial communities is certainly the low oxygen available at lower soil depths. Because of their high clay content, Alptal soils feature anaerobic microenvironments. At this forest site a water-impermeable soil layer in the underground (gleyic soils) hinders to alleviate the oxygen limitation. All these mentioned reasons explain why we found no or only minor changes of the soil microbial communities to the moderate long-term N addition at this forest site.
The bacterial and fungal abundance remained unchanged, which is in agreement with others (Peng et al., 2017; Forstner et al., 2019b). Similarly, Hesse et al. (2015) found no change in fungal biomass in a natural maple forest in USA treated with N for 16 years. We assume that the lack of a significant effect on microbial biomass is linked to the fact that the root biomass was not affected and that the effects on C-exudate production did not alter the size of the root-associated microbes. Similarly, the abundance of C and N cycling genes were not affected by N addition.
Fungal α-diversity (Richness and Shannon Index) also remained unchanged, which is consistent with other studies with long-term N addition experiments in forests (Freedman et al., 2015; Hesse et al., 2015; Morrison et al., 2016). Morrison et al. (2016) reported no effect on fungal diversity with the addition of 50 kg N ha−1 yr−1 but an increase of fungal richness with higher N addition rates (150 kg N ha−1 yr−1). A change of fungal richness in response to N-fertilization was reported in 0–2 cm soil depth but not at lower soil depths (5 and 10 cm). Similarly, Haas et al. (2018) showed an increase in fungal diversity with nutrient addition (100 kg N ha−1 y−1), indicating that the effects on fungal diversity dependent on the amount of N added and soil horizon. N addition may affect microbial communities mainly as a nutrient rather than via soil acidification as suggested by Zhou et al. (2017).
Long-term N additions showed weak (p = 0.06) changes in the structure of the fungal communities. This response was similar across depths (treatment × depth, p = 0.77). It was observed that elevated N deposition has either a significant influence (Eisenlord et al., 2013; Entwistle et al., 2013; Weber et al., 2013; Hesse et al., 2015; Morrison et al., 2016; van der Linde et al., 2018) or no effect (Freedman et al., 2015) on the fungal community composition. N treatments effects were dependent on soil depth in another study (Weber et al., 2013) and were most evident in very shallow surface horizons. We also expected the strongest effects of N deposition in the first (0–5 cm) soil layer according to the partitioning of N deposition (Hagedorn et al., 2001a; Providoli et al., 2006) but here in our study we did not find treatment × soil depth interactions neither for bacteria nor fungi.
The observed change of fungal community composition with elevated N deposition was mainly driven by a shift of relative abundance of Basidiomycota and Ascomycota. Consistent with previous studies that examined the response of soil fungal community composition to N fertilization (Weber et al., 2013), we recovered increased numbers of Ascomycota sequences and decreased numbers of Basidiomycota sequences (i.e., Agaricales) from N-treated soils in all depth intervals. Reduction in Basidiomycota abundance may alleviate some of the competitive pressures on Ascomycota for resources and thus explain the contrasting changes observed in these phyla (Geisseler and Scow, 2014; Farrer and Suding, 2016; Zhou et al., 2017). This phylum-level composition shift was accompanied by changes on lower taxonomical level that warrant further study. Differential abundant taxa analysis indicated that fungal genera such as the ectomycorrhizal fungi Hydnum, Piloderma, Amanita, and Tricholoma showed decreased recovery of sequences in response to N addition. Treseder (2004) and Li et al. (2015) as well observed in their meta-analysis on simulated N deposition a significant decrease of fungal colonization of roots. Because simulated N deposition supplies directly plant-available N, plant investment in ectomycorrhizal fungi may be minimal and result in a reduction of ectomycorrhizal mycelia growth and production (Sims et al., 2007). A decline in the relative abundance of ectomycorrhizal fungi, in particular members of Amanitaceae, Cortinariaceae, and Russulaceae following long-term N addition has also been reported by others (Burke et al., 2006; Weber et al., 2013; Morrison et al., 2016). However, ectomycorrhizal responses have been shown to be taxon-specific with N enrichment by disfavoring Cortinarius species and most Russula species, while significantly enhancing the relative abundance of Russula vinacea (Morrison et al., 2016). Here, nitrophilic species included ectomycorrhizal as well as saprotrophic fungi. In particular, Laccaria, Hygrophorus, and Pachyphlodes, a truffle-like fungi belonging to the Pezizaceae, all known to be ectomycorrhizal associates of trees, were favored by the addition of N.
In contrast to fungi, bacteria were remarkably resistant and did not show changes due to long-term N addition. While the effects of N addition on the diversity of bacterial communities are not always clear, including negative, positive or no effects, changes in the composition across different ecosystems are the rule (Ramirez et al., 2012; Leff et al., 2015; Haas et al., 2018). Studies in mixed hardwood stands, loblolly pine plantations, and boreal forest have all reported either no effect (Burke et al., 2006) or an increase of bacterial richness and diversity after long-term N-addition (Turlapati et al., 2013; Haas et al., 2018). Twenty years of N-addition in a mixed hardwood stand induced a significant increase in diversity as well as a change in composition in both organic and mineral soils (Turlapati et al., 2013). The increase in diversity was attributable to changes in community structure with significantly higher relative abundance of Acidobacteria, Chlamydiae, and Proteobacteria with N addition. In contrast, in our experiment N addition did not change bacterial community structure neither at the phylum level nor at different soil depths after 20 years. Even at lower taxonomic level (e.g., genus) we did not detect significant (p < 0.05) responses of specific bacterial taxa to long-term N addition.
Within deeper soil depths we found significant more bacterial sequences from the class Anaerolineae of the phylum Chloroflexi, independently on the N addition. Members of the phylum Chloroflexi are slow growing heterotrophic bacteria, that are ubiquitous in natural ecosystems, whereas Anaerolineae become abundant at anaerobic conditions (Yamada and Sekiguchi, 2009; Hartmann et al., 2014). Because of their high clay content, combined with the wet climate of the site, this obviously favors the anoxic classes of Chloroflexi like Anaerolineae (Vos et al., 2013). Moreover, the increased presence of the methane-producing Methanocella and Methanolinea in the gleyic soil layers points to prevailing anaerobic conditions that strongly limits the root growth and most probably the response of the soil microbes to long-term moderate N addition.
From a functional approach, we conclude that long-term moderate N addition at the Alptal forest site did not strongly affect the soil microbiome and its functioning. Changes due to the treatment were small compared to the heterogeneity of the soil and to differences between soil horizons. The present study including DNA analyses largely confirms the conclusion that the soil microbiome is remarkably resistant to such a chronic low-dose N treatment. We can see some shifts that can be ascribed to the surplus of N that accumulates in the soil, mainly in the composition of the fungal community. Even if the soil microbiome appears to retain its functions and trees are still taking advantage of the additional N to build larger needles and increase their stem growth (Krause et al., 2012), N continues to accumulate in the soil of the treated plots. All the small changes seen so far could intensify in the future and possibly lead to much stronger impacts in a non-linear way. At (slowly decreasing) ambient deposition rates, however, it seems that no negative consequences on the soil microbiome and its function should be feared within the next decades.
The datasets generated for this study can be found in the NCBI Sequence Read Archive, PRJNA595488.
PS designed the study and participated in sample collection. IB, MC, BF, and PS contributed to data collection. IB, AD, BF, and PS performed data analysis. IB, BF, and PS wrote the study. All authors commented on previous versions of the manuscript, read and approved the final manuscript.
This study was partly funded by the Swiss National Science Foundation (SNSF) under the grant C16.0052: Linking forest soil food-web patterns with soil organic matter characteristics across five European biogeographical regions.
The authors declare that the research was conducted in the absence of any commercial or financial relationships that could be construed as a potential conflict of interest.
We thank the Central Laboratory, Bernhard Elsner, Simon Baumgartner, and Beat Stierli (Swiss Federal Research Institute WSL) for completing soil analyses and assisting with other laboratory work. We also acknowledge the Genetic Diversity Centre (GDC) of the ETH Zurich and the contribution of scientists at the McGill University and Génome Québec Innovation Center in Montréal, Canada, for performing Illumina MiSeq sequencing.
The Supplementary Material for this article can be found online at: https://www.frontiersin.org/articles/10.3389/ffgc.2020.00077/full#supplementary-material
Abarenkov, K., Henrik Nilsson, R., Larsson, K., Alexander, I. J., Eberhardt, U., Erland, S., et al. (2010). The UNITE database for molecular identification of fungi–recent updates and future perspectives. New Phytol. 186, 281–285. doi: 10.1111/j.1469-8137.2009.03160
Aber, J. D. (2002). “Nitrogen saturation in temperate forest ecosystems: current theory, remaining questions and recent advances,” in Progress in Plant Nutrition: Plenary Lectures of the XIV International Plant Nutrition Colloquium. Developments in Plant and Soil Sciences, eds W. J. Horst, A. Bürkert, N. Claassen, H. Flessa, W. B. Frommer, H. Goldbach, W. Merbach, H.-W. Olfs, V. Römheld, B. Sattelmacher, U. Schmidhalter, M. K. Schenk, and N. von Wirén (Dordrecht: Springer), 179–188.
Allen, S. E. (1989). Chemical Analysis of Ecological Materials, 2nd Edn. Oxford, London: Blackwell Scientific Publications.
Allison, S. D., Hanson, C. A., and Treseder, K. K. (2007). Nitrogen fertilization reduces diversity and alters community structure of active fungi in boreal ecosystems. Soil Biol. Biochem. 39, 1878–1887. doi: 10.1016/j.soilbio.2007.02.001
Baldrian, P. (2017). Forest microbiome: diversity, complexity and dynamics. FEMS Microbiol. Rev. 41, 109–130. doi: 10.1093/femsre/fuw040
Bobbink, R., Hicks, K., Galloway, J., Spranger, R., Alkemade, M., Ashmore, M., et al. (2010). Global assessment of nitrogen deposition effects on terrestrial plant diversity: a synthesis. Ecol. Appl. 20, 30–59. doi: 10.1890/08-1140.1
Bowden, R. D., Davidson, E., Savage, K., Arabia, C., and Steudler, P. (2004). Chronic nitrogen additions reduce total soil respiration and microbial respiration in temperate forest soils at the Harvard Forest. For. Ecol. Manag. 196, 43–56. doi: 10.1016/j.foreco.2004.03.011
Bowden, R. D., Rullo, G., Stevens, G. R., and Steudler, P. A. (2000). Soil fluxes of carbon dioxide, nitrous oxide, and methane at a productive temperate deciduous forest. J. Environ. Qual. 29, 268–276. doi: 10.2134/jeq2000.00472425002900010034x
Boxman, A. W., Blanck, K., Brandrud, T. E., Emmett, B. A., Gundersen, P., Hoger-Vorst, R. F., et al. (1998). Vegetation and soil biota response to experimentally-changed nitrogen inputs in coniferous forest ecosystems of the NITREX project. For. Ecol. Manag. 101, 65–79. doi: 10.1016/S0378-1127(97)00126-6
Bredemeier, M., Blanck, K., Xu, Y.-J., Tietema, A., Boxman, A. W., Emmett, B., et al. (1998). Input-output budgets at the NITREX sites. For. Ecol. Manag. 101, 57–64. doi: 10.1016/S0378-1127(97)00125-4
Brunner, I., Herzog, C., Galiano, L., and Gessler, A. (2019). Plasticity of fine-root traits under long-term irrigation of a water-limited Scots pine forest. Front Plant Sci. 10:701. doi: 10.3389/fpls.2019.00701
Burke, D. J., Kretzer, A. M., Rygiewicz, P. T., and Topa, M. A. (2006). Soil bacterial diversity in a loblolly pine plantation: influence of ectomycorrhizas and fertilization. FEMS Microbiol. Ecol. 57, 409–419. doi: 10.1111/j.1574-6941.2006.00125.x
Carnol, M., Cudlin, P., and Ineson, P. (1999). Impacts of (NH4)2SO4 deposition on Norway spruce (Picea abies [L.] Karst) roots. Water Air Soil Pollut. 116, 111–120. doi: 10.1023/A:1005250710017
Carnol, M., Ineson, P., Anderson, J. M., Beese, F., Berg, M. P., Bolger, T., et al. (1997). The effects of ammonium sulphate deposition and root sinks on soil solution chemistry in coniferous forest soils. Biogeochemistry 38, 255–280. doi: 10.1023/A:1005875505591
Cheng, S. J., Hess, P. G., Wieder, W. R., Thomas, R. Q., Nadelhoffer, K. J., Vira, J., et al. (2019). Decadal fates and impacts of nitrogen additions on temperate forest carbon storage: a data-model comparison. Biogeosciences 16, 2771–2793. doi: 10.5194/bg-16-2771-2019
Cotrufo, M. F., Wallenstein, M. D., Boot, C. M., Denef, K., and Paul, E. (2013). The microbial efficiency-matrix stabilization (MEMS) framework integrates plant litter decomposition with soil organic matter stabilization: do labile plant inputs form stable soil organic matter? Glob. Change Biol. 19, 988–995. doi: 10.1111/gcb.12113
DeForest, J. L., Zak, D. R., Pregitzer, K. S., and Burton, A. J. (2004). Atmospheric nitrate deposition and the microbial degradation of cellobiose and vanillin in a northern hardwood forest. Soil Biol. Biochem. 39, 1878–1887. doi: 10.1016/j.soilbio.2004.02.011
Demoling, F., Nilsson, L. O., and Bååth, E. (2008). Bacterial and fungal response to nitrogen fertilization in three coniferous forest soils. Soil Biol. Biochem. 40, 370–379. doi: 10.1016/j.soilbio.2007.08.019
Dise, N. B., and Wright, R. F. (1995). Nitrogen deposition and leaching from European forests. For. Ecol. Manag. 71, 153–162. doi: 10.1016/0378-1127(94)06092-W
Edgar, R. C. (2010). Search and clustering orders of magnitude faster than BLAST. Bioinformatics 26, 2460–2461. doi: 10.1093/bioinformatics/btq461
Edgar, R. C. (2013). UPARSE: highly accurate OTU sequences from microbial amplicon reads. Nat. Methods. 10, 996–998. doi: 10.1038/nmeth.2604
Edgar, R. C., and Flyvbjerg, H. (2015). Error filtering, pair assembly and error correction for next-generation sequencing reads. Bioinformatics 31, 3476–3482. doi: 10.1093/bioinformatics/btv401
Edwards, I. P., Zak, D. R., Kellner, H., Eisenlord, S. D., and Pregitzer, K. S. (2011). Simulated atmospheric N deposition alters fungal community composition and suppresses ligninolytic gene expression in a northern hardwood forest. PLoS ONE 6:e20421. doi: 10.1371/journal.pone.0020421
Eisenlord, S. D., Freedman, Z., Zak, D. R., Xue, K., He, Z., and Zhou, J. (2013). Microbial mechanisms mediating increased soil C storage under elevated atmospheric N deposition. Appl. Environ. Microbiol. 79, 1191–1199. doi: 10.1128/AEM.03156-12
Emmett, B. A., Boxman, D., Bredemeier, M., Gundersen, P., Kjønaas, O. J., Moldan, F., et al. (1998). Predicting the effects of atmospheric nitrogen deposition in conifer stands: evidence from the NITREX ecosystem-scale experiments. Ecosystems 1, 352–360. doi: 10.1007/s100219900029
Entwistle, E. M., Zak, D. R., and Edwards, I. P. (2013). Long-term experimental nitrogen deposition alters the composition of the active fungal community in the forest floor. Soil Sci. Soc. Am. J. 77, 1648–1658. doi: 10.2136/sssaj2013.05.0179
Erisman, J. W., Galloway, J., Seitzinger, S., Bleeker, A., and Butterbach-Bahl, K. (2011). Reactive nitrogen in the environment and its effect on climate change. Curr. Opin. Environ. Sustain. 3, 281–290. doi: 10.1016/j.cosust.2011.08.012
Fan, Y., Miguez-Macho, G., Jobbágy, E. G., Jackson, R. B., and Otero-Casal, C. (2017). Hydrologic regulation of plant rooting depth. Proc. Natl. Acad. Sci. U. S. A. 114, 10572–10577. doi: 10.1073/pnas.1712381114
Farrer, E. C., and Suding, K. N. (2016). Teasing apart plant community responses to N enrichment: the roles of resource limitation, competition and soil microbes. Ecol. Lett. 19, 1287–1296. doi: 10.1111/ele.12665
Forstner, S. J., Wechselberger, V., Müller, S., Keiblinger, K. M., Díaz-Pinés, E., Wanek, W., et al. (2019a). Vertical redistribution of soil organic carbon pools after twenty years of nitrogen addition in two temperate coniferous forests. Ecosystems 22, 379–400. doi: 10.1007/s10021-018-0275-8
Forstner, S. J., Wechselberger, V., Stecher, S., Müller, S., Keiblinger, K. M., Wanek, W., et al. (2019b). Resistant soil microbial communities show signs of increasing phosphorus limitation in two temperate forests after long-term nitrogen addition. Front. For. Glob. Change 2:13. doi: 10.3389/ffgc.2019.00073
Freedman, Z. B., Romanowicz, K. J., Upchurch, R. A., and Zak, D. R. (2015). Differential responses of total and active soil microbial communities to long-term experimental N deposition. Soil Biol. Biochem. 90, 275–282. doi: 10.1016/j.soilbio.2015.08.014
Frey, B., Niklaus, P. A., Kremer, J., Lüscher, P., and Zimmermann, S. (2011). Heavy-machinery traffic impacts methane emissions as well as methanogen abundance and community structure in oxic forest soils. Appl. Environ. Microbiol. 77, 6060–6068. doi: 10.1128/AEM.05206-11
Frey, B., Rime, T., Phillips, M., Stierli, B., Hajdas, I., Widmer, F., et al. (2016). Microbial diversity in European alpine permafrost and active layers. FEMS Microbiol. Ecol. 92:fiw018. doi: 10.1093/femsec/fiw018
Frey, S. D., Knorr, M., Parrent, J. L., and Simpson, R. T. (2004). Chronic nitrogen enrichment affects the structure and function of the soil microbial community in temperate hardwood and pine forests. For. Ecol. Manag. 196, 159–171. doi: 10.1016/j.foreco.2004.03.018
Frey, S. D., Ollinger, S., Nadelhoffer, K., Bowden, R., Brzostek, E., Burton, A., et al. (2014). Chronic nitrogen additions suppress decomposition and sequester soil carbon in temperate forests. Biogeochemistry 121, 305–316. doi: 10.1007/s10533-014-0004-0
Frossard, A., Donhauser, J., Mestrot, A., Gygax, S., and Frey, B. (2018). Long- and short-term effects of mercury pollution on the soil microbiome. Soil Biol. Biochem. 120, 191–199. doi: 10.1016/j.soilbio.2018.01.028
Galloway, J. N., Dentener, F. J., Capone, D. G., Boyer, E. W., Howarth, R. W., Seitzinger, S. P., et al. (2004). Nitrogen cycles: past, present, and future. Biogeochemistry 70, 153–226. doi: 10.1007/s10533-004-0370-0
Galloway, J. N., Townsend, A. R., Erisman, J. W., Bekunda, M., Cai, Z., Freney, J. R., et al. (2008). Transformation of the nitrogen cycle: recent trends, questions, and potential solutions. Science 320, 889–892. doi: 10.1126/science.1136674
Geisseler, D., and Scow, K. M. (2014). Long-term effects of mineral fertilizers on soil microorganisms—a review. Soil Biol. Biochem. 75, 54–63. doi: 10.1016/j.soilbio.2014.03.023
Ghani, A., Dexter, M., and Perrott, K. W. (2003). Hot-water extractable carbon in soils: a sensitive measurement for determining impacts of fertilisation, grazing and cultivation. Soil Biol. Biochem. 35, 1231–1243. doi: 10.1016/S0038-0717(03)00186-X
Gregorich, E. G., Beare, M. H., Stoklas, U., and St-Georges, P. (2003). Biodegradability of soluble organic matter in maize-cropped soils. Geoderma 113, 237–252. doi: 10.1016/S0016-7061(02)00363-4
Gundale, M. J., From, F., Bach, L. H., and Nordin, A. (2014). Anthropogenic nitrogen deposition in boreal forests has a minor impact on the global carbon cycle. Glob. Chang. Biol. 20, 276–286. doi: 10.1111/gcb.12422
Gundersen, P., Callesen, I., and de Vries, W. (1998). Nitrate leaching in forest ecosystems is related to forest floor C/N ratios. Environ. Pollut. 102, 403–407. doi: 10.1016/S0269-7491(98)80060-2
Gundersen, P., Christiansen, J. R., Alberti, G., Brüggemann, N., Castaldi, S., Gasche, R., et al. (2012). The response of methane and nitrous oxide fluxes to forest change in Europe. Biogeosciences 9, 3999–4012. doi: 10.5194/bg-9-3999-2012
Haas, J. C., Street, N. R., Sjödin, A., Lee, N. M., Högberg, M. N., Näsholm, T., et al. (2018). Microbial community response to growing season and plant nutrient optimisation in a boreal Norway spruce forest. Soil Biol. Biochem. 125, 197–209. doi: 10.1016/j.soilbio.2018.07.005
Hagedorn, F., Bucher, J. B., and Schleppi, P. (2001a). Contrasting dynamics of dissolved inorganic and organic nitrogen in soil and surface waters of forested catchments with Gleysols. Geoderma 100, 173–192. doi: 10.1016/S0016-7061(00)00085-9
Hagedorn, F., Schleppi, P., Bucher, J. B., and Flühler, H. (2001b). Retention and leaching of elevated N deposition in a forested ecosystem with Gleysols. Water Air Soil Pollut. 129, 119–142. doi: 10.1023/A:1010397232239
Hart, S. C., Stark, J. M., Davidson, E. A., and Firestone, M. K. (1994). “Nitrogen mineralization, immobilization, and nitrification,” in Methods of Soil Analysis. Part 2: Microbiological and Biochemical Properties, eds R. Weaver, S. Angle, P. Bottomley, D. Bezdicek, S. Smith, S., A. Tabatabai, et al. (Madison, WI: Soil Science Society of America), 985–1018. doi: 10.2136/sssabookser5.2.c42
Hartmann, M., Brunner, I., Hagedorn, F., Bardgett, R. D., Stierli, B., Herzog, C., et al. (2017). A decade of irrigation transforms the soil microbiome of a semi-arid pine forest. Mol. Ecol. 26, 1190–1206. doi: 10.1111/mec.13995
Hartmann, M., Niklaus, P. A., Zimmermann, S., Schmutz, S., Kremer, J., Abarenkov, K., et al. (2014). Resistance and resilience of the forest soil microbiome to logging-associated compaction. ISME J. 8, 226–244. doi: 10.1038/ismej.2013
Hasselquist, N. J., Metcalfe, D. B., and Högberg, P. (2012). Contrasting effects of low and high nitrogen additions on soil CO2 flux components and ectomycorrhizal fungal sporocarp production in a boreal forest. Glob. Change Biol. 18, 3596–3605. doi: 10.1111/gcb.12001
Haynes, B. E., and Gower, S. T. (1995). Belowground carbon allocation in unfertilized and fertilized red pine plantations in northern Wisconsin. Tree Physiol. 15, 317–325. doi: 10.1093/treephys/15.5.317
Hesse, C. N., Mueller, R. C., Vuyisich, M., Gallegos-Graves, L. V., Gleasner, C. D., Zak, D. R., et al. (2015). Forest floor community metatranscriptomes identify fungal and bacterial responses to N deposition in two maple forests. Front. Microbiol. 6:337. doi: 10.3389/fmicb.2015.00337
Högberg, P., Fan, H., Quist, M., Binkley, D., and Tamm, C. O. (2006). Tree growth and soil acidification in response to 30 years of experimental nitrogen loading on boreal forest. Glob. Change Biol. 12, 489–499. doi: 10.1111/j.1365-2486.2006.01102.x
Janssens, I. A., Dieleman, W., Luyssaert, S., Subke, J.-A., Reichstein, M., Ceulemans, R., et al. (2010). Reduction of forest soil respiration in response to nitrogen deposition. Nat. Geosci. 3, 315–322. doi: 10.1038/ngeo844
Jenkinson, D. S., Brookes, P. C., and Powlson, D. S. (2004). Measuring soil microbial biomass. Soil Biol. Biochem. 36, 5–7. doi: 10.1016/j.soilbio.2003.10.002
Joergensen, R. G., and Mueller, T. (1996). The fumigation-extraction method to estimate soil microbial biomass: calibration of the kEN value. Soil Biol. Biochem. 28, 33–37. doi: 10.1016/0038-0717(95)00102-6
Krause, K., Cherubini, P., Bugmann, H., and Schleppi, P. (2012). Growth enhancement of Picea abies trees under long-term, low-dose N addition is due to morphological more than to physiological changes. Tree Physiol. 32, 1471–1481. doi: 10.1093/treephys/tps109
Krause, K., Niklaus, P. A., and Schleppi, P. (2013). Soil-atmosphere fluxes of the greenhouse gases CO2, CH4 and N2O in a mountain spruce forest subjected to long-term N addition and to tree girdling. Agric. For. Meteorol. 181, 61–68. doi: 10.1016/j.agrformet.2013.07.007
Leff, J. W., Jones, S. E., Prober, S. M., Barberán, A., Borer, E. T., Firn, J. L., et al. (2015). Consistent responses of soil microbial communities to elevated nutrient inputs in grasslands across the globe. Proc. Natl. Acad. Sci. U. S. A. 112, 10967–10972. doi: 10.1073/pnas.1508382112
Li, W., Jin, C., Guan, D., Wang, Q., Wang, A., Yuan, F., et al. (2015). The effects of simulated nitrogen deposition on plant root traits: a meta-analysis. Soil Biol. Biochem. 82, 112–118. doi: 10.1016/j.soilbio.2015.01.001
Liu, X., Yang, Z., Lin, C., Giardina, C. P., Xiong, D., Lin, W., et al. (2017). Will nitrogen deposition mitigate warming-increased soil respiration in a young subtropical plantation? Agric. For. Meteorol. 246, 78–85. doi: 10.1016/j.agrformet.2017.06.010
Llado, S., Lopez-Mondejar, R., and Baldrian, P. (2017). Forest soil bacteria: diversity, involvement in ecosystem processes, and response to global change. Microbiol. Mol. Biol. Rev. 81, e00063–e00016. doi: 10.1128/MMBR.00063-16
Love, M. I., Huber, W., and Anders, S. (2014). Moderated estimation of fold change and dispersion for RNA-seq data with DESeq2. Genome Biol. 15:550. doi: 10.1186/s13059-014-0550-8
Maaroufi, N. I., Nordin, A., Hasselquist, N. J., Bach, L. H., Palmqvist, K., and Gundale, M. J. (2015). Anthropogenic nitrogen deposition enhances carbon sequestration in boreal soils. Glob. Change Biol. 21, 3169–3180. doi: 10.1111/gcb.12904
Maaroufi, N. I., Nordin, A., Palmqvist, K., Hasselquist, N. J., Forsmark, B., Rosenstock, N. P., et al. (2019). Anthropogenic nitrogen enrichment enhances soil carbon accumulation by impacting saprotrophs rather than ectomycorrhizal fungal activity. Glob. Change Biol. 25, 2900–2914. doi: 10.1111/gcb.14722
McMurdie, P. J., and Holmes, S. (2013). Phyloseq: an R package for reproducible interactive analysis and graphics of microbiome census data. PLoS ONE 8:e61217. doi: 10.1371/journal.pone.0061217
Mellert, K. H., and Göttlein, A. (2012). Comparison of new foliar nutrient thresholds derived from van den Burg's literature compilation with established central European references. Euro. J. For. Res. 131, 1461–1472. doi: 10.1007/s10342-012-0615-8
Mohn, J., Schürmann, A., Hagedorn, F., Schleppi, P., and Bachofen, R. (2000). Increased rates of denitrification in nitrogen-treated forest soils. For. Ecol. Manag. 137, 113–119. doi: 10.1016/S0378-1127(99)00320-5
Moldan, F., and Wright, R. F. (2011). Nitrogen leaching and acidification during 19 years of NH4NO3 additions to a coniferous-forested catchment at Gårdsjön, Sweden (NITREX). Environ. Pollut. 159, 431–440. doi: 10.1016/j.envpol.2010.10.025
Mooshammer, M., Wanek, W., Hämmerle, I., Fuchslueger, L., Hofhansl, F., Knoltsch, A., et al. (2014). Adjustment of microbial nitrogen use efficiency to carbon:nitrogen imbalances regulates soil N cycling. Nat. Commun. 5:3694. doi: 10.1038/ncomms4694
Morier, I., Schleppi, P., Saurer, M., Providoli, I., and Guenat, C. (2010). Retention and hydrolysable fraction of atmospherically deposited nitrogen in two contrasting forest soils in Switzerland. Eur. J. Soil Sci. 61, 197–206. doi: 10.1111/j.1365-2389.2010.01226.x
Morrison, E. W., Frey, S. D., Sadowsky, J. J., van Diepen, L. T., Thomas, W. K., and Pringle, A. (2016). Chronic nitrogen additions fundamentally restructure the soil fungal community in a temperate forest. Fungal Ecol. 23, 48–57. doi: 10.1016/j.funeco.2016.05.011
Nadelhoffer, K. J., Emmett, B. A., Gundersen, P., Kjønaas, O. J., Koopmans, C. J., Schleppi, P., et al. (1999). Nitrogen deposition makes a minor contribution to carbon sequestration in temperate forests. Nature 398, 145–148. doi: 10.1038/18205
Nguyen, N. H., Song, Z. W., Bates, S. T., Branco, S., Tedersoo, L., Menke, J., et al. (2016). FUNGuild: an open annotation tool for parsing fungal community datasets by ecological guild. Fungal Ecol. 20, 241–248. doi: 10.1016/j.funeco.2015.06.006
Oksanen, J., Blanchet, F. G., Friendly, M., Kindt, R., Legendre, P., McGlinn, D., et al. (2017). Vegan: Community Ecology Package. R Package Version 2.4–4. Available online at: http://CRAN.Rproject.org/package=vegan
Ostonen, I., Püttsepp, Ü., Biel, C., Alberton, O., Bakker, M. R., Lõhmus, K., et al. (2007). Specific root length as an indicator of environmental change. Plant Biosyst. 141, 426–442. doi: 10.1080/11263500701626069
Peng, Y., Chen, G. S., Chen, G. T., Li, S., Peng, T. C., Qiu, X. R., et al. (2017). Soil biochemical responses to nitrogen addition in a secondary evergreen broad-leaved forest ecosystem. Sci. Rep. 7:2783. doi: 10.1038/s41598-017-03044-w
Providoli, I., Bugmann, H., Siegwolf, R., Buchmann, N., and Schleppi, P. (2006). Pathways and dynamics of 15 and 15 applied in a mountain Picea abies forest and in a nearby meadow in central Switzerland. Soil Biol. Biochem. 38, 1645–1657. doi: 10.1016/j.soilbio.2005.11.019
Quast, C., Pruesse, E., Yilmaz, P., Gerken, J., Schweer, T., Yarza, P., et al. (2013). The SILVA ribosomal RNA gene database project: improved data processing and web-based tools. Nucleic Acids Res. 41, D590–D596. doi: 10.1093/nar/gks1219
R Core Team (2017). R: A Language and Environment for Statistical Computing. Vienna: R Foundation for Statistical Computing.
Ramirez, K. S., Craine, J. M., and Fierer, N. (2012). Consistent effects of nitrogen amendments on soil microbial communities and processes across biomes. Glob. Change Biol. 18, 1918–1927. doi: 10.1111/j.1365-2486.2012.02639.x
Rime, T., Hartmann, M., and Frey, B. (2016). Potential sources of microbial colonizers in initial soil ecosystem after retreat of an Alpine glacier. ISME J. 10, 1625–1641. doi: 10.1038/ismej.2015.238
Robertson, G. P., Wedin, D., Groffmann, P. M., Blair, J. M., Holland, E. A., Nadelhoffer, K. J., et al. (1999). “Soil carbon and nitrogen availability: Nitrogen mineralization, nitrification, and soil respiration potentials,” in Standard Soil Methods for Long-Term Ecological Research, eds P. Robertson, D. C. Coleman, C. Bledsoe, and P. Sollins (New York, NY: Oxford University Press), 258–271.
Rousk, J., Bååth, E., Brookes, P. C., Lauber, C. L., Lozupone, C., Caporaso, J. G., et al. (2010). Soil bacterial and fungal communities across a pH gradient in an arable soil. ISME J. 4, 1340–1351. doi: 10.1038/ismej.2010.58
Saiya-Cork, K. R., Sinsabaugh, R. L., and Zak, D. R. (2002). The effects of long term nitrogen deposition on extracellular enzyme activity in an Acer saccharum forest soil. Soil Biol. Biochem. 34, 1309–1315. doi: 10.1016/S0038-0717(02)00074-3
Schleppi, P., Curtaz, F., and Krause, K. (2017). Nitrate leaching from a sub-alpine coniferous forest subjected to experimentally increased N deposition for 20 years, and effects of tree girdling and felling. Biogeochemistry 134, 319–335. doi: 10.1007/s10533-017-0364-3
Schleppi, P., Hagedorn, F., and Providoli, I. (2004). Nitrate leaching from a mountain forest ecosystem with Gleysols subjected to experimentally increased N deposition. Water Air Soil Pollut. Focus 4, 453–467. doi: 10.1023/B:WAFO.0000028371.72044.fb
Schleppi, P., Muller, N., Feyen, H., Papritz, A., Bucher, J., and Flühler, H. (1998). Nitrogen budgets of two small experimental forested catchments at Alptal, Switzerland. For Ecol. Manag. 101, 177–185. doi: 10.1016/S0378-1127(97)00134-5
Schmitz, A., Sanders, T., Bolte, A., Bussotti, F., Dirnböck, T., Johnson, J., et al. (2019). Responses of forest ecosystems in Europe to decreasing nitrogen deposition. Environ. Poll. 244, 980–994. doi: 10.1016/j.envpol.2018.09.101
Shen, J., Zhang, L. M., Guo, J. F., Ray, J. L., and He, J. Z. (2010). Impact of long-term fertilization practices on the abundance and composition of soil bacterial communities in northeast China. Appl. Soil Ecol. 46, 119–124. doi: 10.1016/j.apsoil.2010.06.015
Simpson, D., Andersson, C., Christensen, J. H., Engardt, M., Geels, C., Nyiri, A., et al. (2014). Impacts of climate and emission changes on nitrogen deposition in Europe: a multi-model study. Atmos. Chem. Phys. 14, 6995–7017. doi: 10.5194/acp-14-6995-2014
Sims, S. E., Hendricks, J. J., Mitchell, R. J., Kuehn, K. A., and Pecot, S. D. (2007). Nitrogen decreases and precipitation increases ectomycorrhizal extramatrical mycelia production in a longleaf pine forest. Mycorrhiza 17, 299–309. doi: 10.1007/s00572-007-0105-x
Sinsabaugh, R. L., Carreiro, M. M., and Repert, D. A. (2002). Allocation of extracellular enzymatic activity in relation to litter composition, N deposition, and mass loss. Biogeochemistry 60, 1–24. doi: 10.1023/A:1016541114786
Solberg, S., Dobbertin, M., Reinds, G. J., Lange, H., Andreassen, K., Garcia Fernandez, P., et al. (2009). Analyses of the impact of changes in atmospheric deposition and climate on forest growth in European monitoring plots: a stand growth approach. For. Ecol. Manag. 258, 1735–1750. doi: 10.1016/j.foreco.2008.09.057
Thomas, Q. R., Canham, C. D., Weathers, K. C., and Goodale, C. L. (2010). Increased tree carbon storage in response to nitrogen deposition in the US. Nat. Geosci. 3, 13–17. doi: 10.1038/ngeo721
Treseder, K. K. (2004). A meta-analysis of mycorrhizal responses to nitrogen, phosphorus, and atmospheric CO2 in field studies. New Phytol. 164, 347–355. doi: 10.1111/j.1469-8137.2004.01159.x
Treseder, K. K. (2008). Nitrogen additions and microbial biomass: a meta-analysis of ecosystem studies. Ecol. Lett. 11, 1111–1120. doi: 10.1111/j.1461-0248.2008.01230.x
Turlapati, S. A., Minocha, R., Bhiravarasa, P. S., Tisa, L. S., Thomas, W. K., and Minocha, S. C. (2013). Chronic N-amended soils exhibit an altered bacterial community structure in Harvard Forest, MA, USA. FEMS Microbiol. Ecol. 83, 478–493. doi: 10.1111/1574-6941.12009
Uroz, S., Buée, M., Deveau, A., Mieszkin, S., and Martin, F. (2016). Ecology of the forest microbiome: highlights of temperate and boreal ecosystems. Soil Biol. Biochem. 103, 471–488. doi: 10.1016/j.soilbio.2016.09.006
van der Linde, S., Suz, L. M., Orme, C. D. L., Cox, F., Andreae, H., Asi, E., et al. (2018). Environment and host as large-scale controls of ectomycorrhizal fungi. Nature 558, 243–248. doi: 10.1038/s41586-018-0189-9
Vance, E. D., Brookes, P. C., and Jenkinson, D. S. (1987). An extraction method for measuring soil microbial biomass C. Soil Biol. Biochem. 19, 703–707. doi: 10.1016/0038-0717(87)90052-6
Vos, M., Wolf, A. B., Jennings, S. J., and Kowalchuk, G. A. (2013). Microscale determinants of bacterial diversity in soil. FEMS Microbiol. Rev. 37, 936–954. doi: 10.1111/1574-6976.12023
Wallenstein, M. D., McNulty, S., Fernandez, I. J., Boggs, J., and Schlesinger, W. H. (2006). Nitrogen fertilization decreases forest soil fungal and bacterial biomass in three long-term experiments. For. Ecol. Manag. 222, 459–468. doi: 10.1016/j.foreco.2005.11.002
Wang, Q., Garrity, G. M., Tiedje, J. M., and Cole, J. R. (2007). Naïve Bayesian classifier for rapid assignment of rRNA sequences into the new bacterial taxonomy. Appl. Environ. Microbiol. 73, 5261–5267. doi: 10.1128/AEM.00062-07
Weber, C. F., Vilgalys, R., and Kuske, C. R. (2013). Changes in fungal community composition in response to elevated atmospheric CO2 and nitrogen fertilization varies with soil horizon. Front. Microbiol. 4:78. doi: 10.3389/fmicb.2013.00078
Xu, G.-L., Schleppi, P., Li, M.-H., and Fu, S.-L. (2009). Negative responses of Collembola in a forest soil (Alptal, Switzerland) under experimentally increased N deposition. Environ. Pollut. 157, 2030–2036. doi: 10.1016/j.envpol.2009.02.026
Yamada, T., and Sekiguchi, Y. (2009). Cultivation of uncultured Chloroflexi subphyla: significance and ecophysiology of formerly uncultured Chloroflexi “subphylum I” with natural and biotechnological relevance. Microbes Environ. 24, 205–216. doi: 10.1264/jsme2.me09151s
Zechmeister-Boltenstern, S., Keiblinger, K. M., Mooshammer, M., Peñuelas, J., Richter, A., Sardans, J., et al. (2015). The application of ecological stoichiometry to plant-microbial-soil organic matter transformations. Ecol. Monogr. 85, 133–155. doi: 10.1890/14-0777.1
Zhang, H., Liu, Y., Zhou, Z., and Zhang, Y. (2019). Inorganic nitrogen addition affects soil respiration and belowground organic carbon fraction for a Pinus tabuliformis forest. Forests 10:369. doi: 10.3390/f10050369
Keywords: forest ecosystems, nitrogen, ecosystem functioning, soil microbiome, Illumina MiSeq sequencing, fungal communities, FUNGuild
Citation: Frey B, Carnol M, Dharmarajah A, Brunner I and Schleppi P (2020) Only Minor Changes in the Soil Microbiome of a Sub-alpine Forest After 20 Years of Moderately Increased Nitrogen Loads. Front. For. Glob. Change 3:77. doi: 10.3389/ffgc.2020.00077
Received: 09 March 2020; Accepted: 22 May 2020;
Published: 24 June 2020.
Edited by:
Klaus Kaiser, Martin Luther University Halle-Wittenberg, GermanyReviewed by:
Chao Wang, Institute of Applied Ecology (CAS), ChinaCopyright © 2020 Frey, Carnol, Dharmarajah, Brunner and Schleppi. This is an open-access article distributed under the terms of the Creative Commons Attribution License (CC BY). The use, distribution or reproduction in other forums is permitted, provided the original author(s) and the copyright owner(s) are credited and that the original publication in this journal is cited, in accordance with accepted academic practice. No use, distribution or reproduction is permitted which does not comply with these terms.
*Correspondence: Beat Frey, YmVhdC5mcmV5QHdzbC5jaA==
Disclaimer: All claims expressed in this article are solely those of the authors and do not necessarily represent those of their affiliated organizations, or those of the publisher, the editors and the reviewers. Any product that may be evaluated in this article or claim that may be made by its manufacturer is not guaranteed or endorsed by the publisher.
Research integrity at Frontiers
Learn more about the work of our research integrity team to safeguard the quality of each article we publish.