- 1Center for Tree Science, The Morton Arboretum, Lisle, IL, United States
- 2College of Arts & Sciences, Valparaiso University, Valparaiso, IN, United States
Predicting the ecological consequences of shifting forest composition requires an understanding of how tree species alter the soils beneath them. However, given the huge number of tree species on earth, it is critical to assess the extent to which easily observable traits facilitate the prediction of soil biogeochemistry. Both aboveground and belowground traits are important drivers of forest function. The objective of this study was to assess the relative importance of leaf habit (evergreen or deciduous) and root mycorrhizal association (arbuscular [AM] or ectomycorrhizal ECM]) on biogeochemistry. The relative importance of these two traits for carbon (C) and nitrogen (N) dynamics have proven difficult to disentangle as most deciduous tree species associate with AM fungi and most evergreen tree species associate with ECM fungi. Using planted, single-species forestry plots at The Morton Arboretum (DuPage County, IL), we found that mycorrhizal association better predicted tree effects on soil than leaf habit. While both leaf habit and mycorrhizal association drove variation in soil C:N ratios, mycorrhizal association alone predicted differences in soil pH, available N pools, extracellular enzyme activities, and C and N cycling rates. Surprisingly, ammonium concentrations were higher and net nitrification rates were faster in ECM plots than in AM plots. Greater N-degrading enzyme activities in ECM plots and intrinsically high soil pH across the garden likely drove more rapid N cycling in ECM plots. Overall, this study supports the inclusion of mycorrhizal association in terrestrial biosphere models, but suggests that the effects of mycorrhizal association on N dynamics may be site-dependent.
Introduction
Anthropogenic disturbances—including climate change, air pollution, invasive forest pests, and harvesting regimes—are altering the distribution of tree species and the composition of forest communities, with consequences for ecosystem functioning (Crowley et al., 2016; Crowley and Lovett, 2017). For instance, increases in temperature and precipitation have shifted the northern hardwood-boreal ecotone upward in elevation (Beckage et al., 2008), and climate-induced shifts in temperate forest community composition drive changes in soil biogeochemistry (Rollinson et al., 2012; McDaniel et al., 2013, 2014). As such, it is critical to characterize the effects of trees on soils in order to better predict the consequences of changing forest composition. Tree species differ in their influences on soil carbon (C) and nutrient cycling, in part, due to differences in the quantity and chemistry of their organic matter inputs (e.g., leaf and root litters and root exudates). However, given that there are over 60,000 known tree species (Beech et al., 2017), a species-level approach is impractical for both data collection and modeling applications. Instead, plant functional groups, which reflect suites of traits correlated through eco-evolutionary trade-offs (Reich, 2014), offer a practical solution for predicting consequences of tree distribution and forest composition shifts.
Leaf habit (evergreen or deciduous) and mycorrhizal association (arbuscular mycorrhizal [AM] or ectomycorrhizal [ECM]) are two traits commonly used to bin tree species into functional groups. Indeed, both leaf habit and mycorrhizal association have proven useful for predicting range shifts in response to climate change; deciduous forests are predicted to expand in response to climate change while the trailing range edge of AM trees constricts more rapidly that of ECM trees in response to climate change (Cramer et al., 2001; Lankau et al., 2015). Both classification schemes also distinguish tree nutritional strategies, with consequences for soil biogeochemistry. For instance, compared to deciduous trees, evergreen trees exhibit greater nitrogen (N) use efficiency and lower litter decomposition rates owing to greater litter recalcitrance (e.g., higher litter lignin:N ratios) (Aerts, 1995; Scott and Binkley, 1997; Silver and Miya, 2001; Reich and Oleksyn, 2004). Subsequently, soils under evergreen trees commonly have lower pH, wider C:N, and slower N cycling rates than those under deciduous trees (Binkley, 1995; Vesterdal et al., 2008; Cools et al., 2014; Augusto et al., 2015; Mueller et al., 2016). Similarly, due to a combination of litter chemistry and mycorrhizal nutrient acquisition strategies, stands dominated by AM-associated trees (AM stands) and stands dominated by ECM-associated trees (ECM stands) also have distinct soil pH, C:N, and N “economies” (Read, 1991; Cornelissen et al., 2001; Phillips et al., 2013; Averill et al., 2014; Lin et al., 2017; Zhu et al., 2018; Keller and Phillips, 2019). Specifically, relative to those under ECM-associated trees, soils under AM-associated trees tend to have higher pH, narrower C:N, greater inorganic N availability, and faster N mineralization and nitrification rates (Phillips et al., 2013; Averill et al., 2014; Lin et al., 2017; Zhu et al., 2018).
While the effects of both leaf habit and mycorrhizal association on soil processes have been considered separately, evaluating their relative importance has proven challenging due, in part, to correlations among traits in commonly studied clades. For instance, in a recent analysis of fine root decomposition rates of AM and ECM trees, 60% of broadleaf ECM species were from the order Fagales and 90% of the conifers were ECM (See et al., 2019). Few studies account for this phylogenetic autocorrelation (but see Averill et al., 2019). Similarly, most previous studies on leaf habit effects have represented evergreen trees with Pineaceae (Augusto et al., 2015). Pinaceae are also associated with ECM fungi, while most angiosperms associate with AM fungi (Averill et al., 2019), making it difficult to identify whether leaf habit or mycorrhizal association drives differences between these groups. Disentangling leaf habit and mycorrhizal association effects is even more challenging in soil biogeochemistry studies given that dominant ECM species in the wild are primarily from two clades—Fagales and Pinaceae—and AM deciduous trees are often represented by Acer (Zhu et al., 2018; Keller and Phillips, 2019). Considering these biases, extra care must be taken when interpreting the effects of leaf habit and mycorrhizal association on plant traits and ecosystem functions (summarized in Tedersoo and Bahram, 2019). Capturing broad phylogenetic breadth is paramount for robustly evaluating the impacts of mycorrhizal association and leaf habit on soil biogeochemistry.
Additionally, teasing apart tree effects on soils vs. soils as a driver of tree community composition has remained a challenge. Hans Jenny, in his seminal work, referred to this conundrum as “a real bugberrer” (Jenny, 1941). For example, sites with less soil N have high concentrations of evergreen trees, implying that deciduous trees out-compete evergreen trees in sites with high soil N (Binkley and Giardina, 1998; Lusk et al., 2003). Similarily, sites with less soil N tend to contain larger concentrations of trees associating with ECM fungi than with AM fungi, suggesting that AM-associated trees are also superior competitors in N-rich sites (Zhu et al., 2018; Jo et al., 2019). In contrast, in planted common garden experiments, tree effects on soil are isolated from tree colonization preferences. Common garden studies occasionally reveal unexpected patterns (Binkley, 1995; Mueller et al., 2016). In fact, in a meta-analysis of leaf habit effects on soil N mineralization, Mueller et al. (2016) found higher rates of N mineralization under deciduous trees compared to evergreen trees, but only when naturally occurring stands were included; when their analysis was limited to common garden studies, they found no differences in N mineralization rates between deciduous and evergreen stands. Thus, an open question remains—to what extent do trees with different leaf habits and/or mycorrhizal associations create sites with distinct soil properties? In this study, we used nearly 100-year-old tree plantations spanning a broad phylogenetic breadth to evaluate the relative importance of mycorrhizal association and leaf habit on soil C and N dynamics.
Methods
Site Description
This study was conducted in the forestry plots, a planted experiment similar to a common garden, at The Morton Arboretum in Lisle, Illinois (41.81°N, 88.05°W). The region has a continental climate with temperatures as low as −6°C in January and up to 22°C in July, with 800–1,000 mm mean annual precipitation. Soils found here are deep and moderately- to poorly-drained Alfisols formed from a thin layer of loess (0.3–1 m) underlain by glacial till and Mollisols formed from alluvium. The major soil series are Ashkum, Beecher, and Ozaukee silt loams and Sawmill silty clay loam (Soil Survey Staff and Natural Resources Conservation Service United States Department of Agriculture, 2019). Prior to European settlement, The Morton Arboretum consisted mainly of burr and white oak savannah in addition to prairie and oak-dominated deciduous forest (Bowles et al., 1994). Following settlement, “high grading” or selective cutting of large diameter trees occurred, followed by some clear cutting in 1917 (Wilhelm, 1991).
Beginning in 1922, monoculture plots ranging from half an acre to four acres (0.2–1.6 hectares) in size were established with the goal of demonstrating the practical value of reforestation in the Midwestern U.S. By extension, the plots were also established to test and study “all the timber trees of the world which might come under consideration for reforestation purposes in this part of the country” (Morton Arboretum Staff, 1929). Currently, 38 of the initial plots remain intact and viable for examining tree effects on soil properties (Table 1). Plots are distributed throughout the 1,700 acre (688 hectare) property of The Morton Arboretum (Figure 1A). Plots with only 3–4 stems remaining were deemed too small and excluded from our study; plots were also excluded if the understories consisted of frequently mowed turf grass or mulch. These remaining plots include 28 species and range in size from 265 m2 to 3.4 acres.
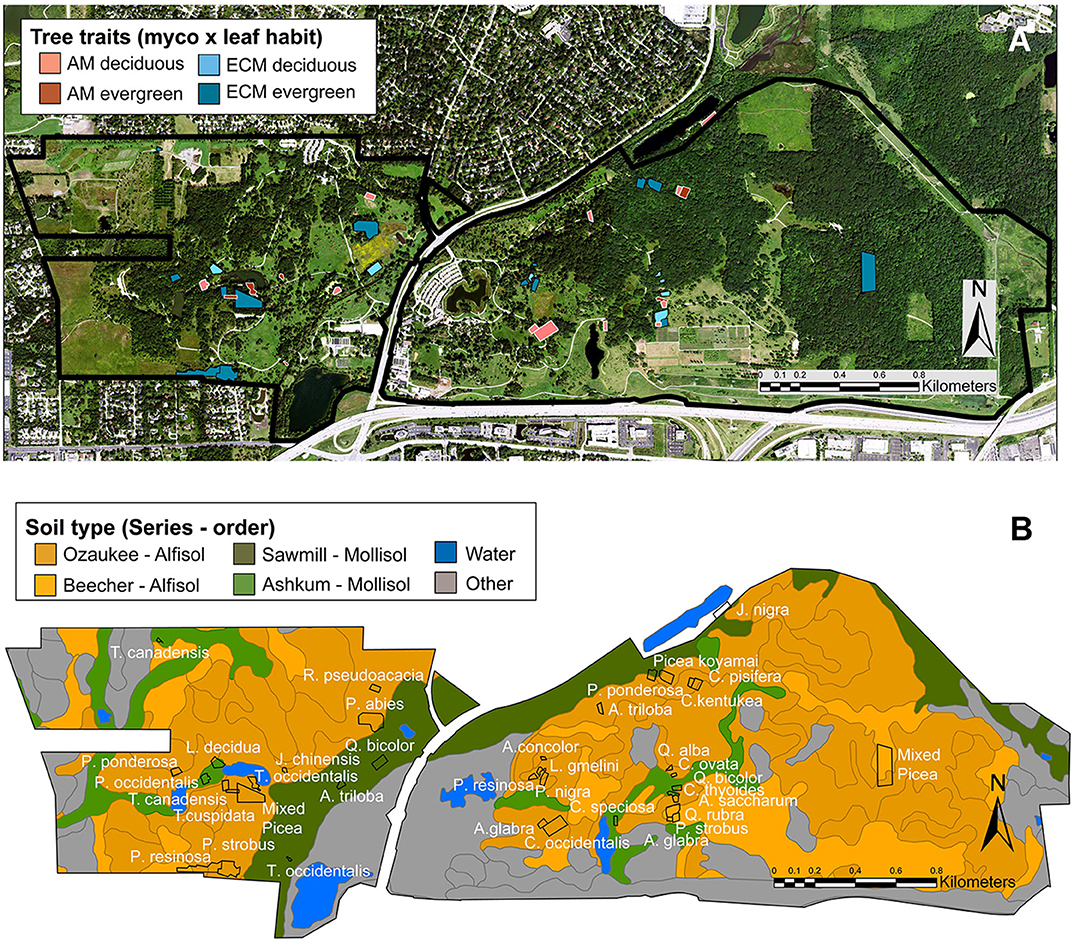
Figure 1. Locations of forestry plots at The Morton Arboretum. Trees varying in mycorrhizal association (AM or ECM) and leaf habit (deciduous or evergreen) (A) were planted as monoculture plots on Alfisols (Ozaukee and Beecher soil series) and Mollisols (Sawmill or Ashkum soil series) (B). In (A), pink, AM plots; blue, ECM plots; light hues, deciduous plots; dark hues, evergreen plots. In (B), dark orange, Ozaukee series (Alfisol); light orange, Beecher series (Alfisol); dark green, Sawmill series (Mollisol); light green, Ashkum series (Mollisol); blue, water; gray, other soil series.
Living trees in the plots were planted between 1922 and 1948 according to accession records (http://bol.mortonarb.org); library records and published bulletins indicate that most plots were planted in the 1920's. While five plots contain no accessioned trees and two contain accessioned trees with unknown planting dates, three of these plots were planted in the 1920's according to published bulletins, and one additional plot is clearly marked on a 1942 Evergreen trail map (Watts, 1942), indicating it was planted in the 1930's, at the latest. Only four plots have no accession or other published planting records. As such, it is likely that all plots were at least 70 years old at the time of sampling with most between 85 and 95 years old. One exception is a Robinia pseudoacacia plot that has no accessioned trees or planting records. Tree cores indicate the oldest trees in this plot were established in 1978 (Christine Rollinson, personal communication).
Tree species included in our study span broad phylogenetic and geographical-origin space with all combinations of mycorrhizal associations and leaf habits represented (Table 1). As such, the species present in our study deviate from those commonly examined in other studies. There are 11 AM deciduous, 6 AM evergreen, 7 ECM deciduous, and 14 ECM evergreen plots. Mycorrhizal association assignments were made following Tedersoo and Brundrett (2017), Brundrett and Tedersoo (2019), Akhmetzhanova et al. (2012), and Wang and Qiu (2006). Species-level mycorrhizal associations were not available for Taxus cuspidata or Cladrastis kentukea, so mycorrhizal assignments were based on genus (Taxus cuspidata) and family (Cladrastis kentukea) information. All evergreen tree species are gymnosperms, and most deciduous tree species are angiosperms. Two Larix species are deciduous gymnosperms. All plots were planted as monocultures of individual tree species except for two Picea plots that contain a mixture of Picea abies, Picea glauca, and Picea pungins. Though soil series and orders vary among the plots (Figure 1B; Table 1), there are no systematic differences in soil properties underlying trees with varying leaf habits or mycorrhizal associations (X2 (df = 1, N = 38) ≥ 0.173; P ≥ 0.356).
Soil Sampling and Processing
To evaluate the effects of tree traits on soil biogeochemistry, we collected soil samples four times during the 2018 growing season: in early May, mid-June, mid-August, and late October. Thirty-two of the 38 plots were sampled in May, 37 were sampled in June, and all 38 were sampled in August and October. After removing the litter layer, we collected four cores from the top 15 cm of the mineral soil from each plot with a 5 cm diameter stainless steel soil corer. Organic horizons in these soils are thin or absent due to relatively high soil pH and nutrient status. Coring sites were purposefully located in the center of a triangle consisting of three dominant stems of the species of interest. The four coring sites were distributed evenly throughout a plot, and we took care to avoid edge effects; cores were collected at least 5 m from the edge of a given plot with edge borders defined by the outermost trees in a plot.
Upon returning to the laboratory, we separated cores into the upper 0–5 cm and lower 5–15 cm layers of soil. We aggregated layers for each plot and sieved them through a 2 mm sieve to homogenize the soil and remove rocks and roots. We stored sieved soils at 4°C overnight before extracting C and N pools, conducting C mineralization assays, and initiating N cycling incubations. We conducted enzyme assays and chloroform fumigations within 12 months of collection. We stored soils for enzyme analyses at −80°C prior to analyses; soils for microbial biomass fumigations at −20°C prior to extraction; and all solutions at −20°C prior to analyses.
Sample Analyses
We measured the water and organic matter content, as well as pH, of our samples using standard laboratory techniques. We dried subsamples of soil (5 g) at 105°C for 24 h to determine gravimetric soil moisture. We subsequently measured organic matter (OM) content by ashing dry soils in a muffle furnace at 450°C for 16 h. We measured soil pH in 0.01 M CaCl2 solutions (5 g soil in 40 mL of solution) using a benchtop electrode pH meter (Orion 5 Star, Thermo Scientific, Beverly, Massachusetts).
To assess tree effects on soil C and N pools, we quantified total C and N, extractable inorganic N, extractable organic C and total N, and microbial biomass C and N concentrations. To measure total C and N, we dried soil samples at 55°C and ground them to fine powders. We used the dry combustion method to measure total C and N concentrations (Vario El III, Elementar, Lengenselbold, Germany). To evaluate inorganic N pools, we extracted inorganic N (ammonium and nitrate) from 4 g soil samples with 2M KCl. We quantified ammonium concentrations using the salicylate-nitroprusside method (Sims et al., 1995) and measured absorbance at 660 nm on a microtiter plate reader (Synergy HTX, Biotek, Winooski, VT). We quantified nitrate concentrations using the VCl3/Griess method (Hood-Nowotny et al., 2010) and measured absorbance at 540 nm on a microtiter plate reader. Total inorganic N is the sum of ammonium and nitrate. We extracted organic C and total N from 10 g soil samples with 0.5M K2SO4 and quantified total organic C and total N concentrations in extracts with high-temperature oxidation and chemiluminescence detection (TOC-L and TNM-L TOC-TN analyzer, Shimadzu, Kyoto, Japan). We calculated extractable organic N as the difference between total extractable N and total inorganic N. We determined soil microbial biomass C and N concentrations by quantifying changes in 0.5M K2SO4-extractable pools of C and N after 4 days of chloroform fumigation (Vance et al., 1987). We adjusted our microbial biomass C values to reflect an extractability of 45% (Beck et al., 1997) and our microbial biomass N values to reflect an extractability of 54% (Brookes et al., 1985).
To evaluate C and N fluxes, we conducted extracellular enzyme activity assays and lab incubations. We measured the potential activities of five extracellular enzymes: β-1,4-glucosidase (BG [EC: 3.2.1.21], a labile C-degrading enzyme which hydrolyzes cellobiose into glucose), N-acetyl-β-D-glucosamidase (NAG [3.1.6.1], a N-degrading enzyme which aids in degrading chitin), acid phosphomonoesterase (AP [3.1.3.2], a phosphorus (P)-degrading enzyme which cleaves phosphate from organic matter), and peroxidase and polyphenol oxidase (PER [1.11.1.7] and PPO [1.10.3.2], respectively, which degrade complex C compounds). We suspended samples (1.5–2 g) in 100 mL of sodium acetate buffer (pH 5.0) and dispensed aliquots into 96-well microplates. We incubated microplates in the dark at 23°C for 2 h (NAG and AP), 5 h (BG), or 4 h (PER and PPO). Immediately prior to measuring BG, NAG, and AP activities, we added 20 μL of 1 M NaOH to improve fluorescence (German et al., 2011). The activities of BG, NAG, and AP were measured with methylumbelliferone-linked substrates using a microplate fluorometer with 365 nm excitation and 450 nm emission filters, while PPO and PER activities were measured spectrophotometrically with absorbance at 460 nm using L-3,4-dihydroxy phenylalanine (L-DOPA) as the substrate (Saiya-Cork et al., 2002). For PER assays, all wells additionally received 20 mL of 0.3% hydrogen peroxide.
To determine C mineralization rates, we incubated 5 g soil samples for 3 h at 23°C and quantified C mineralization rates as the change in CO2 concentrations over the course of the incubation (LI-6200, Li-Cor Incorporated, Lincoln, Nebraska). We determined net N mineralization rates by quantifying the change in 2M KCl-extractable pools of ammonium and nitrate in 4 g subsamples after an aerobic 14 day laboratory incubation at 23°C. We measured net nitrification rates by quantifying the change in nitrate over the same time period. Ammonium and nitrate concentrations were quantified as above.
Statistical Analyses
We used mixed linear models to evaluate the effects of mycorrhizal association, leaf habit, and their interaction on soil biogeochemical properties. Our mixed linear models included mycorrhizal association (AM or ECM), leaf habit (evergreen or deciduous), and their interaction as fixed effects, plot ID as a random effect, and the above variables of interest as dependent variables to determine the impact of tree traits on soil properties. We ran separate sets of models for the two soil layers (0–5 cm and 5–15 cm). In addition to examining the above variables scaled by soil dry weights, we also evaluated the effects of mycorrhizal association, leaf habit, and their interaction on total soil C:N, microbial biomass C:N and the proportion of extractable N in inorganic forms. We also assessed tree trait effects on several enzyme ratios (BG:NAG, BG:AP, and NAG:AP). Enzyme ratios integrate relative microbial C and nutrient demand and availability, often varying with mycorrhizal association (Midgley and Phillips, 2016; Cheeke et al., 2017). We conducted all statistical analyses using the lmerTest package for mixed linear models in R (Kuznetsova et al., 2017; R Core Team, 2019). Results were considered significant if they had P-values < 0.05.
Results
Across all analyses, there were no statistically significant interactions between mycorrhizal association and leaf habit (P ≥ 0.075). In addition, mycorrhizal association effects on soil properties in the 5–15 cm soil layer were generally consistent with those in the 0–5 cm soil layer or dampened (Tables 2, 3). Hence, we largely highlight the results from the main effects of mycorrhizal association and leaf habit on soil biogeochemistry in the 0–5 cm soil layer.
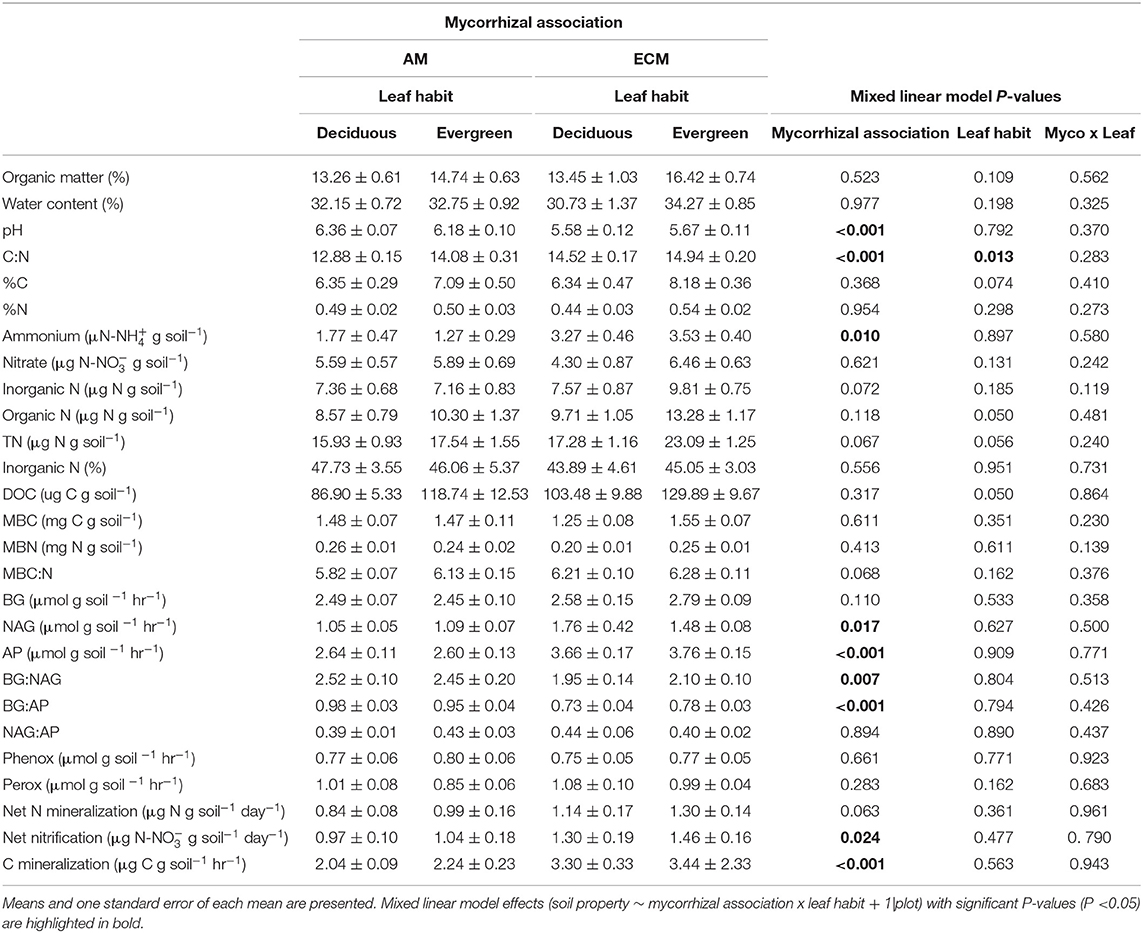
Table 2. Properties of 0–5 cm soils in AM deciduous, AM evergreen, ECM deciduous, and ECM evergreen forestry plots at The Morton Arboretum.
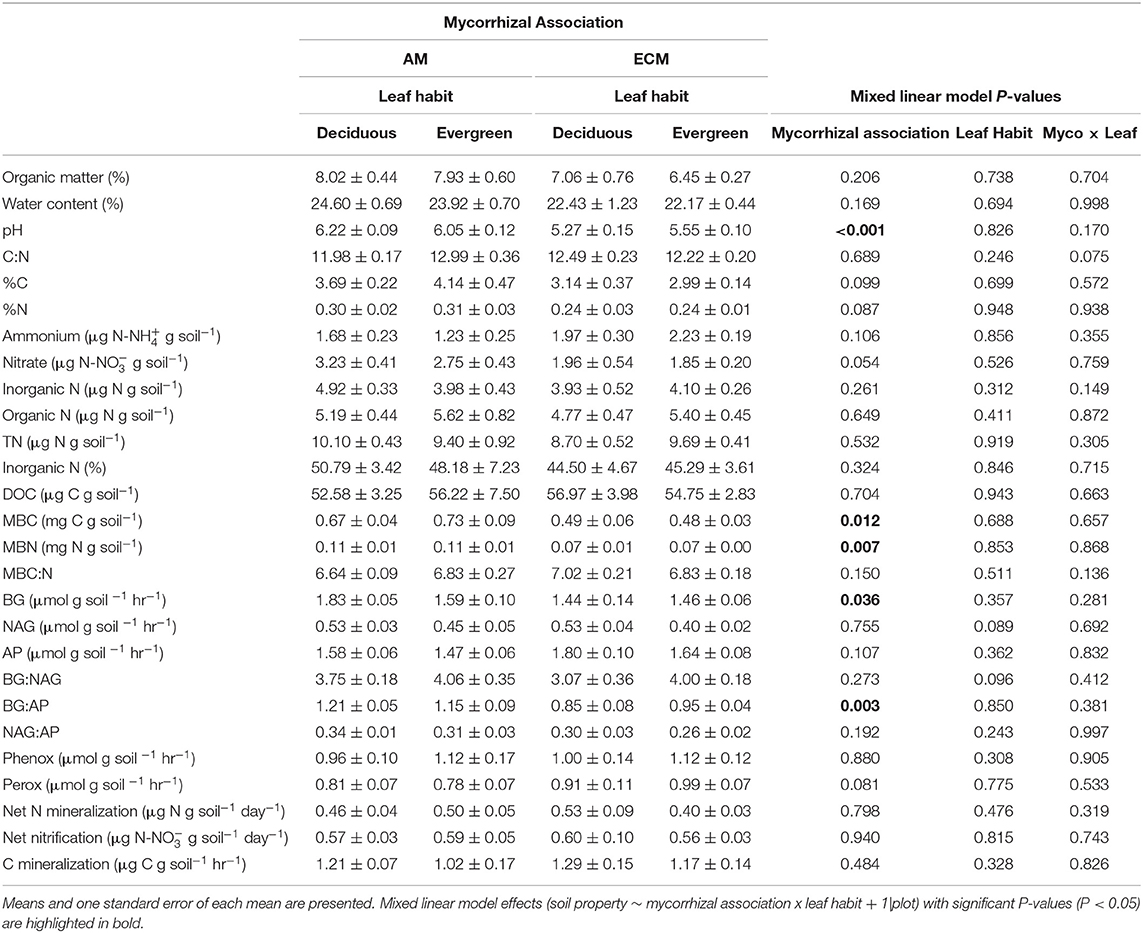
Table 3. Properties of 5–15 cm soils in AM deciduous, AM evergreen, ECM deciduous, and ECM evergreen forestry plots at The Morton Arboretum.
Both mycorrhizal association and leaf habit drove variation in basic soil properties. While soil organic matter and water content did not vary between AM and ECM or deciduous and evergreen plots (Figure 2A), both mycorrhizal association and leaf habit drove differences in soil C:N—soil C:N was wider in ECM plots than in AM plots and wider in evergreen plots than in deciduous plots (Figure 2C). While soil C content tended to be higher in evergreen plots than in deciduous plots (P = 0.074), neither %N nor %C clearly drove C:N patterns. However, mycorrhizal association alone predicted differences in soil pH—pH was higher in AM plots than in ECM plots (Figure 2B). These pH patterns also manifested in the 5–15 cm soil layer.
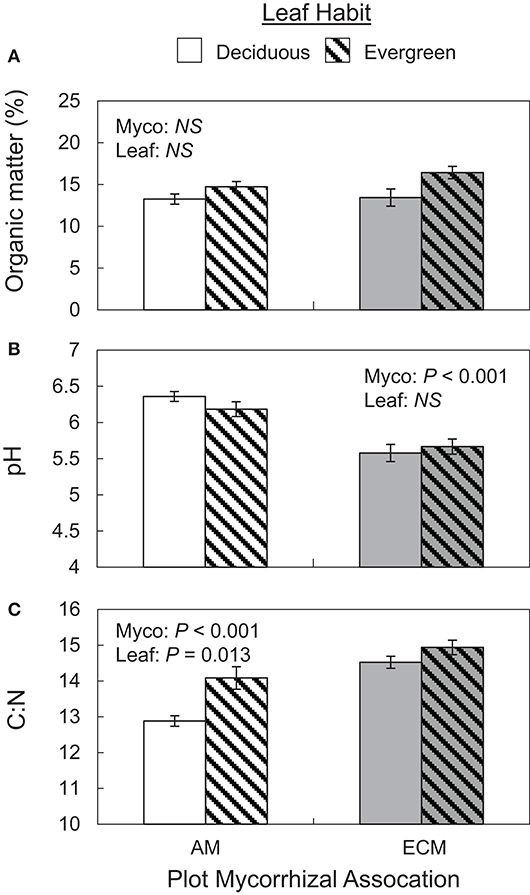
Figure 2. Soil organic matter content (A), pH (B), and C:N ratios (C) in forestry plots at The Morton Arboretum. Bars are mean ratios; one standard error of the mean is presented. Open bars, deciduous plots; hatched bars, evergreen plots; white bars, AM plots; gray bars, ECM plots.
Differences in extractable N pools were largely limited to ammonium concentrations in AM and ECM plots. Ammonium concentrations were higher in ECM plots than in AM plots, though there were no significant differences in nitrate concentrations between AM and ECM plots (Figure 3A). On average, nitrate concentrations were nearly two times greater than ammonium concentrations; as such, inorganic N concentrations were not significantly different but tended to be higher in ECM plots than in AM plots (P = 0.072). Organic N and total extractable N concentrations also exhibited little difference between AM and ECM plots. Total extractable N concentrations tended to be higher in ECM plots than in AM plots (P = 0.067), and both organic and extractable N concentrations tended to be higher in evergreen plots than in deciduous plots (TN: P = 0.056; DON: P = 0.050). Overall, the proportion of inorganic N in the extractable N pool did not vary with plot mycorrhizal association or leaf habit; inorganic N was 46 ± 2% of the total extractable N pool across all plots (Figure 3B).
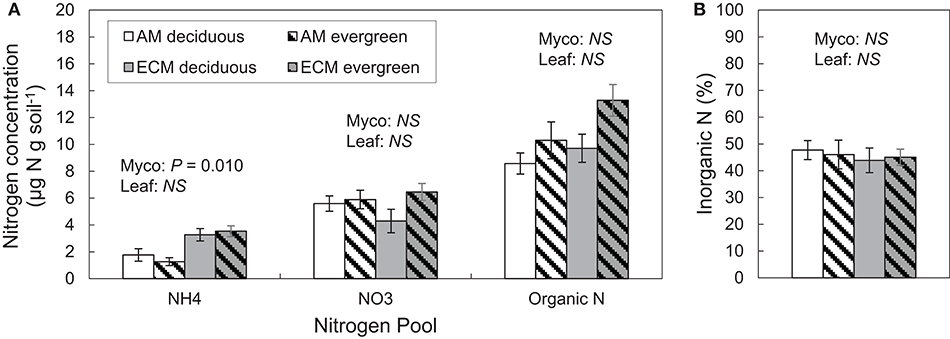
Figure 3. Soil nitrogen pools (A) and the proportion of inorganic N in the extractable N pool (B) in forestry plots at The Morton Arboretum. Bars are mean ratios; one standard error of the mean is presented. Open bars, deciduous plots; hatched bars, evergreen plots; white bars, AM plots; gray bars, ECM plots.
Similarly, neither mycorrhizal association nor leaf habit drove variation in extractable organic C, microbial biomass C and microbial biomass N pools, or in microbial biomass C:N ratios, though microbial biomass C:N tended to be wider in ECM plots than in AM plots (P = 0.068), consistent with total soil C:N. However, in the 5–15 cm soil layer, microbial biomass C and N were both higher in AM plots than in ECM plots; we found no effects of mycorrhizal association on microbial biomass C:N in the 5–15 cm soil layer.
Extracellular enzyme activities and their ratios exclusively varied with plot mycorrhizal association. While BG and oxidative enzyme activities did not vary between AM and ECM plots (Figure 4A), both NAG and AP activities were greater in ECM plots than in AM plots (Figures 4B,C). As such, BG:NAG and BG:AP ratios were wider in AM plots than in ECM plots. However, we found no differences in NAG:AP ratios between AM and ECM plots. In the 5–15 cm soil layer, BG activities were higher in AM plots than in ECM plots, which drove wider BG:AP ratios in AM plots relative to ECM plots, consistent with BG:AP ratios in the 0–5 cm soil layer.
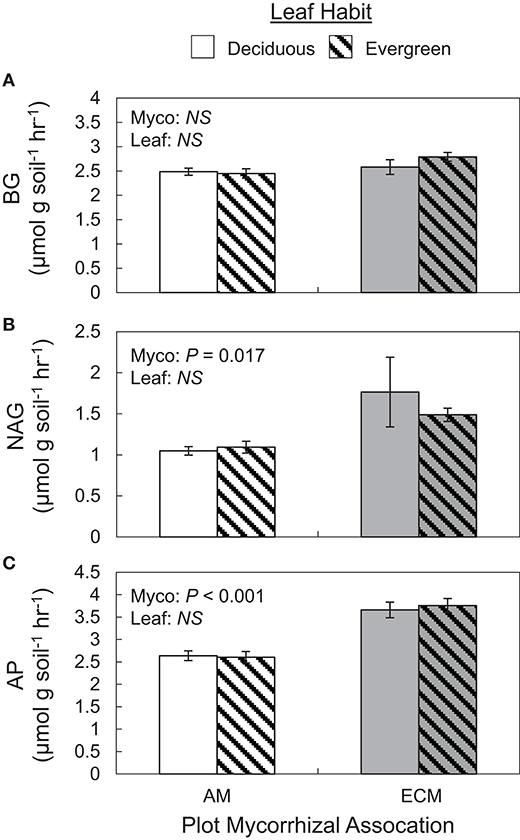
Figure 4. Potential soil extracellular enzyme activities of β-1,4-glucosidase (BG) (A), N-acetyl-β-d-glucosamidase (NAG) (B), acid phosphatase (AP) (C) in forestry plots at The Morton Arboretum. Bars are mean ratios; one standard error of the mean is presented. open bars, deciduous plots; hatched bars, evergreen plots; white bars, AM plots; gray bars, ECM plots.
Both C and N flux rates varied with plot mycorrhizal association, but not leaf habit (Figure 5). While N mineralization rates only tended to be faster in ECM plots relative to AM plots (P = 0.063), nitrification rates were faster in ECM plots than in AM plots. On average, nitrification rates were also faster than N mineralization rates, indicating that both initially available ammonium and N mineralized during the incubation were largely nitrified (Figure 5A). Carbon mineralization rates were also faster in ECM plots than in AM plots (Figure 5B).
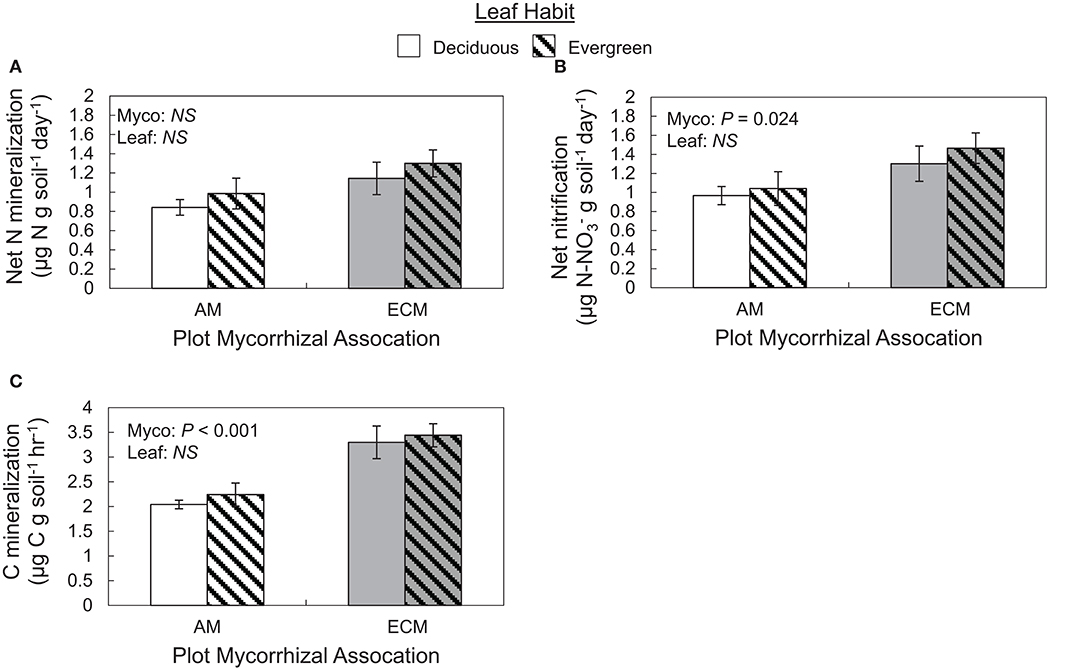
Figure 5. Rates of ex situ net nitrogen mineralization (A), net nitrification (B), and carbon mineralization (C) in soils collected from forestry plots at The Morton Arboretum. Bars are mean ratios; one standard error of the mean is presented. Open bars, deciduous plots; hatched bars, evergreen plots; white bars, AM plots; gray bars, ECM plots.
Discussion
Overall, mycorrhizal association was a better predictor of tree trait-driven differences in soil C and N dynamics than leaf habit. Specifically, soils in ECM plots had larger ammonium pools, greater N-degrading enzyme activities, and faster C and N flux rates than soils from AM plots. Additionally, soils in AM plots had higher pH than those in ECM plots. In contrast, leaf habit alone did not predict any variable. Soil C:N was wider in evergreen plots than in deciduous plots, but soil C:N was also wider in ECM plots compared to AM plots. Global syntheses have found that mycorrhizal association is a more important trait for predicting tree tissue chemistry and decomposition rate than leaf habit (Zhang et al., 2018; Averill et al., 2019; Keller and Phillips, 2019; See et al., 2019). Here, we show that mycorrhizal association is also more important than leaf habit for predicting soil biogeochemistry. However, our study also complements a growing body of literature demonstrating that mycorrhizal effects on soil processes are not uniform across sites (e.g., Craig et al., 2019). Given the recent push to incorporate mycorrhizal associations into terrestrial biosphere and global climate models (Brzostek et al., 2017; Sulman et al., 2017, 2019), it is imperative for underlying assumptions to be critically considered when inferring model implications.
Soil C and N Dynamics Vary With Mycorrhizal Association
For one soil property—soil C:N—AM deciduous trees and ECM evergreen trees represented end members along a spectrum. The widest soil C:N was found under ECM evergreen trees while the narrowest soil C:N was found under AM deciduous trees. This finding is consistent with a myriad of other studies finding wider C:N in ECM soils than in AM soils (i.e., Averill et al., 2014; Lin et al., 2017; Zhu et al., 2018) and in evergreen soils than in deciduous soils (Vesterdal et al., 2008; Cools et al., 2014; Augusto et al., 2015). Two mechanisms are commonly attributed to C:N differences among stands: the “Gadgil” effect and differences in plant tissue C:N (Gadgil and Gadgil, 1971, 1975; Averill et al., 2014; Cools et al., 2014; Averill and Hawkes, 2016). However, the Gadgil effect—a build-up of soil C in ECM soils due to competition between ECM and saprotrophic fungi for resources—is minimized in sites rich in inorganic N (Fernandez et al., 2019; Maaroufi et al., 2019; Smith and Wan, 2019), such as ours. Furthermore, we found no evidence of increased C concentrations in ECM plots relative to AM plots. As such, Gadgil effects are not likely driving differences in C:N ratios in our study. Instead, differences in soil C:N likely reflect litter C:N. Deciduous leaf litter tends to have a narrower C:N ratio than evergreen leaf litter (Cornwell et al., 2008; Augusto et al., 2015) and ECM leaf litter tends to have a narrower C:N ratio than AM leaf litter (Keller and Phillips, 2019). Thus, our soil C:N ratios are likely correlated with the C:N of the leaf litter than falls on it (Cools et al., 2014).
Carbon dynamics exclusively varied with mycorrhizal association and largely aligned with patterns found at other sites. ECM soils from the 0–5 cm layer exhibited faster C mineralization rates than AM soils, and AM soils from the 5–15 cm layer had greater BG activities than ECM soils. In contrast, mycorrhizal association did not drive variation in oxidative enzyme activities. Similarly, across a series of sites in southern Indiana, soils from ECM stands had faster C mineralization rates and lower BG activities than AM stands, and there were no consistent differences in oxidative enzyme activities (Brzostek et al., 2015; Midgley and Phillips, 2016; Cheeke et al., 2017). While other recent studies have detected faster heterotrophic respiration and soil respiration rates in AM soils or in AM-dominated stands compared to ECM soils and stands (Taylor et al., 2016; Wang and Wang, 2018; Lang et al., 2019), these discrepancies are likely due to differences in methodologies and calculations. For instance, while Taylor et al. (2016) found that heterotrophic respiration (per unit C) was faster from AM soils than from ECM soils, AM soils also had lower C concentrations. Additionally, in situ soil respiration rate measurements encompass both root and heterotrophic respiration; faster respiration rates from AM stands were largely driven by higher soil temperatures in a recent study at the Hubbard Brook Experimental Forest (Lang et al., 2019), while in our lab incubations, temperature was held constant. Overall, our C dynamic patterns are not unusual, though drivers of these patterns and their relationships with soil respiration remain to be explored.
Based on previous studies, we expected soil NAG activities to be higher, and soil inorganic N pools and fluxes to be lower, in ECM plots relative to AM plots—in other words, ECM plots would have an “organic N economy” (Phillips et al., 2013). While soil NAG activities were higher in ECM plots than in AM plots, inorganic N pools (ammonium, in particular) and nitrification rates were actually larger and faster in ECM plots than in AM plots. In contrast, N mineralization and nitrification rates are commonly slower in ECM stands than in AM stands (Phillips and Fahey, 2006; Phillips et al., 2013; Midgley and Phillips, 2016; Lin et al., 2017). Faster inorganic N cycling rates and availability in ECM plots are especially surprising given the wider C:N and lower pH soils found in ECM plots compared to AM plots; N mineralization and nitrification rates are often negatively correlated with C:N and positively and positively correlated with pH (Scott and Binkley, 1997; Finzi et al., 1998a,b; Gundersen et al., 1998; Lovett et al., 2004; Hobbie et al., 2006). Perhaps most surprisingly, we found no evidence of differences in the N “economies” of AM and ECM plots; the relative abundance of soil inorganic N was similar between AM and ECM plots.
Drivers of Mycorrhizal Effects on N Dynamics
What underlies these surprising N dynamics? While ECM fungi and their hosts may have co-evolved in low N environments (Arnoldi et al., 2019; Lu and Hedin, 2019), the characteristics that enable them to persist in low N environments (i.e., N acquisition abilities and N use efficiency) may enhance N availability in high N environments, as we found in this study. In fact, in a uniform N environment, ECM trees had faster root exudation rates than AM trees (Liese et al., 2018), indicating that faster exudation rates from ECM trees are not simply a phenotypic response to low N environments. ECM trees and fungi enhance N-rich compound degradation via root exudate priming and N-degrading enzyme production (Read and Perez-Moreno, 2003; Talbot et al., 2008; Yin et al., 2014; Lin et al., 2018), and ECM trees take up relatively more organic N than and have higher N use efficiency than AM trees (Liese et al., 2018; Zhang et al., 2018). Thus, despite generally high soil N availability, ECM fungi and trees still produce high concentrations of N-degrading enzymes in our ECM plots, and the ECM trees may continue to be efficient in their N use. These mechanisms account for the build-up of inorganic N, ammonium in particular, in ECM plots.
Faster nitrification rates in ECM plots relative to AM plots are the likely product of higher ammonium concentrations in ECM plots and the generally high soil pH at our site. As is commonly found across other sites, AM plots had higher soil pH than ECM plots (Phillips et al., 2013; Taylor et al., 2016; Chen et al., 2018), likely owing to the production of organic acids by roots and litter-degrading microbes. Nitrification is often suppressed in lower pH sites (Ste-Marie and Paré, 1999), and this may contribute to slower ECM nitrification rates found in other studies comparing AM and ECM stands (Phillips et al., 2013; Midgley and Phillips, 2016). However, the soil pH at our site was generally high−5.6 in ECM sites and 6.3 in AM sites, in the 0–5 cm soil layer. As such, despite the acidifying effects of ECM trees and fungi, soil pH did not mediate nitrification rates. Given the abundant ammonium and high pH under ECM trees at our site, subsequently higher nitrification rates in ECM soils are to be expected.
Stepping back, these seemingly surprising N dynamics are not particularly unusual. For instance, a study from Whitehall Forest in Georgia found no differences in inorganic N pools under AM and ECM trees (Taylor et al., 2016). Their pH ranges and averages were similar to ours (ECM = 5.6; AM = 6.1), and their nitrate pools were an order of magnitude larger than ammonium pools. Similarly, under naturally-occurring trees in northeast China, soils under ECM trees tended to have faster N mineralization rates and higher NAG enzyme activities relative to those under AM trees (Chen et al., 2018). Again, ECM trees had soils with an average pH of ~5.5 compared to AM trees that had an average soil pH ~6. In contrast, examples of AM and ECM stands with distinct N “economies” are largely from the same series of forests in southern Indiana, which have inherently low pH (AM = 5.2 and ECM = 4.3 at Moores Creek; [Phillips et al., 2013]). In a cross-site study, nitrate decreased as ECM dominance increased in low pH sites, but this relationship did not persist at higher pH sites (Craig et al., 2019). Similarly, in a study from Denmark that evaluated the effects of several tree species on soil biogeochemistry, there were no detectable effects of tree species on soil N dynamics at a higher pH site with an agricultural land-use history while at two previously forested sites with lower pH, there were clear “spruce effects” (Ribbons et al., 2018). In short, tree species effects, and AM and ECM tree effects, in particular, are modulated by site conditions, and inherent soil pH may be a particularly important factor mediating inorganic N pools and fluxes.
Implications and Future Directions
Future studies should identify the penultimate drivers of our observed differences in soil C and N pools and fluxes by examining soil property relationships with continuous tree traits, such as leaf and root litter and root exudate quantity and chemistry (C:N, lignin:N, calcium content, etc.). Stand-level properties including tree productivity and standing biomass, light incidence to the forest floor, and understory community composition may also drive variable C and N dynamics. For instance, if trees or understory plants in our AM stands are more productive than those in our ECM stands, this could account for the lower concentrations of available ammonium in AM plots (Binkley and Giardina, 1998). Studies that have considered both tree species and functional groups (e.g., mycorrhizal association, leaf habit) have often found that variation within these groups is greater than variation between groups (i.e., Taylor et al., 2016; Chen et al., 2018; Sun et al., 2018). As such, identifying the continuous traits that best predict differences among species within these groups will be key to incorporating variation into terrestrial biosphere models. Root properties may be particularly important for soil biogeochemistry. However, while some studies have found differences in exudation rates and root decomposition between AM and ECM trees (Yin et al., 2014; Liese et al., 2018; See et al., 2019), others have not (Brzostek and Finzi, 2011; Sun et al., 2018). Furthermore, the effects of mycorrhizal fungi on C and N dynamics vary markedly across fungal lineages (Lindahl and Tunlid, 2015; Pellitier and Zak, 2018). General understanding of variation in root and fungal characteristics and their relationships with soil C and N dynamics remains limited; this topic clearly merits further investigation.
Our study complements a growing body of research suggesting that mycorrhizal association is a more important trait than leaf habit; as such, mycorrhizal association is an important trait to incorporate into terrestrial biosphere and global climate models (Brzostek et al., 2017; Sulman et al., 2017, 2019). However, intrinsic soil properties may mediate the impacts of trees on soils (Callesen et al., 2013; Ribbons et al., 2018; Craig et al., 2019). While percent clay is often considered (e.g., Averill et al., 2014), we have argued here that soil pH may be important for predicting mycorrhizal effects on N cycling. Soil pH can be predicted by soil and climate (Slessarev et al., 2016), and, thus, could be incorporated into global models. This could assist in identifying where tree-driven changes in pH will or will not lead to differences in soil N dynamics. While mycorrhizal association is increasingly incorporated into global models (Brzostek et al., 2017; Sulman et al., 2019), these are parameterized assuming ECM trees exist in low N environments. Here, we show that this may not be universally true, and we urge future modeling efforts to account for this context-dependency.
Broadly speaking, some mycorrhizal-associated plant traits and ecosystem properties seem to be very stable across sites and studies (e.g., leaf and litter N; soil C:N and pH), others may be context-dependent (leaf litter decomposition; soil inorganic N; rhizosphere effects), and others still may be driven by factors unrelated to tree mycorrhizal associations (root decomposition and other root traits). Our common garden-style design, which captures a broad phylogenetic range of tree species, may better reflect tree effects on soil properties than studies performed in natural settings. New common garden studies, designed to evaluate mycorrhizal effects across broad phylogenetic space and across sites with varying inherent soil properties, are needed to obtain a better picture of AM vs. ECM effects on soils, their interactions with other traits, the mechanisms driving these effects, and their context-dependency.
Data Availability Statement
The datasets generated for this study are available on request to the corresponding author.
Author Contributions
MM formulated the research questions and designed the research. MM and RS collected samples, analyzed samples, and conducted statistical analyses. MM wrote the first draft of the manuscript with significant intellectual and editing input from RS. All authors contributed to manuscript revision, and read and approved the final submission.
Funding
This project was supported by The Morton Arboretum's Center for Tree Science. RS was supported as an Undergraduate Research Fellow (2018) and a Research Experience Extension Fellow (2019) by The Morton Arboretum and Morton Salt.
Conflict of Interest
The authors declare that the research was conducted in the absence of any commercial or financial relationships that could be construed as a potential conflict of interest.
Acknowledgments
We thank Michelle Catania and The Morton Arboretum Soils Lab volunteers for field and lab assistance, as well as, Silvia Alvarez-Clare and Allyson Salisbury for insightful suggestions on data interpretation and presentation. We also thank Kurt Dreisilker and Matt Lobdell for providing access to the forestry plots and the Natural Resources, Landscape, and Collections crews for maintaining the plots.
References
Aerts, R. (1995). The advantages of being evergreen. Trends Ecol. Evol. 10, 402–407. doi: 10.1016/S0169-5347(00)89156-9
Akhmetzhanova, A. A., Soudzilovskaia, N. A., Onipchenko, V., Cornwell, W. K., Agafonov, V. A., Selivanov, I. A., et al. (2012). A rediscovered treasure: mycorrhizal intensity database for 3000 vascular plant species across the former Soviet Union. Ecology 93:689. doi: 10.1890/11-1749.1
Arnoldi, J., Coq, S., Kéfi, S., and Ibanez, S. (2019). Positive plant–soil feedback trigger tannin evolution by niche construction: a spatial stoichiometric model. J. Ecol. 13234, 1365–2745. doi: 10.1101/320572
Augusto, L., De Schrijver, A., Vesterdal, L., Smolander, A., Prescott, C., and Ranger, J. (2015). Influences of evergreen gymnosperm and deciduous angiosperm tree species on the functioning of temperate and boreal forests. Biol. Rev. 90, 444–466. doi: 10.1111/brv.12119
Averill, C., Bhatnagar, J. M., Dietze, M. C., Pearse, W. D., and Kivlin, S. N. (2019). Global imprint of mycorrhizal fungi on whole-plant nutrient economics. Proc. Natl. Acad. Sci. U.S.A. 116, 23163–23168. doi: 10.1073/pnas.1906655116
Averill, C., and Hawkes, C. V. (2016). Ectomycorrhizal fungi slow soil carbon cycling. Ecol. Lett. 19, 937–947. doi: 10.1111/ele.12631
Averill, C., Turner, B. L., and Finzi, A. C. (2014). Mycorrhiza-mediated competition between plants and decomposers drives soil carbon storage. Nature 505, 543–545. doi: 10.1038/nature12901
Beck, T., Joergensen, R. G., Kandeler, E., Makeschin, F., Nuss, E., Oberholzer, H. R., et al. (1997). An inter-laboratory comparison of ten different ways of measuring soil microbial biomass C. Soil Biol. Biochem. 29, 1023–1032. doi: 10.1016/S0038-0717(97)00030-8
Beckage, B., Osborne, B., Gavin, D. G., Pucko, C., Siccama, T., and Perkins, T. (2008). A rapid upward shift of a forest ecotone during 40 years of warming in the green mountains of vermont. Proc. Natl. Acad. Sci. U.S.A. 105, 4197–4202. doi: 10.1073/pnas.0708921105
Beech, E., Rivers, M., Oldfield, S., and Smith, P. P. (2017). GlobalTreeSearch: the first complete global database of tree species and country distributions. J. Sustain. For. 36, 454–489. doi: 10.1080/10549811.2017.1310049
Binkley, D. (1995). “The influence of tree species on forest soils: processes and patterns,” in Proceedings of the Trees and Soil Workshop, eds. D. Mead and I. Cornforth (Canterbury: Lincoln University Press), 1–34.
Binkley, D., and Giardina, C. (1998). Why do tree species affect soils? The warp and woof of tree-soil interactions. Biogeochemistry 42, 89–106. doi: 10.1023/A:1005948126251
Bowles, M. L., Hutchison, M. D., and Mcbride, J. (1994). “Landscape pattern and structure of oak savanna, woodland, and barrens in Northeastern Illinois at the time of European settlement,” in Proceedings of the North American Conference on Savannas and Barrens: Living in the Edge, ed J. Fralish (Normal, IL: Illinois State University), 65–74.
Brookes, P. C., Landman, A., Pruden, G., and Jenkinson, D. S. (1985). Chloroform fumigation and the release of soil nitrogen: a rapid direct extraction method to measure microbial biomass nitrogen in soil. Soil Biol. Biochem. 17, 837–842. doi: 10.1016/0038-0717(85)90144-0
Brundrett, M., and Tedersoo, L. (2019). Misdiagnosis of mycorrhizas and inappropriate recycling of data can lead to false conclusions. New Phytol. 221, 18–24. doi: 10.1111/nph.15440
Brzostek, E. R., Dragoni, D., Brown, Z. A., and Phillips, R. P. (2015). Mycorrhizal type determines the magnitude and direction of root-induced changes in decomposition in a temperate forest. New Phytol. 206, 1274–1282. doi: 10.1111/nph.13303
Brzostek, E. R., and Finzi, A. C. (2011). Substrate supply, fine roots, and temperature control proteolytic enzyme activity in temperate forest soils. Ecology 92, 892–902. doi: 10.1890/10-1803.1
Brzostek, E. R., Rebel, K. T., Smith, K. R., and Phillips, R. P. (2017). “Integrating mycorrhizas into global scale models: a journey toward relevance in the Earth's climate system,” in Mycorrhizal Mediation of Soil: Fertility, Structure, and Carbon Storage, eds N. Johnson, C. Gehring, and J. Jansa (Amsterdam: Elsevier), 479–499. doi: 10.1016/B978-0-12-804312-7.00026-7
Callesen, I., Nilsson, L. O., Schmidt, I. K., Vesterdal, L., Ambus, P., Christiansen, J. R., et al. (2013). The natural abundance of 15N in litter and soil profiles under six temperate tree species: N cycling depends on tree species traits and site fertility. Plant Soil 368, 375–392. doi: 10.1007/s11104-012-1515-x
Cheeke, T. E., Phillips, R. P., Brzostek, E. R., Rosling, A., Bever, J. D., and Fransson, P. (2017). Dominant mycorrhizal association of trees alters carbon and nutrient cycling by selecting for microbial groups with distinct enzyme function. New Phytol. 214, 432–442. doi: 10.1111/nph.14343
Chen, X., Ding, Z., Tang, M., and Zhu, B. (2018). Greater variations of rhizosphere effects within mycorrhizal group than between mycorrhizal group in a temperate forest. Soil Biol. Biochem. 126, 237–246. doi: 10.1016/j.soilbio.2018.08.026
Cools, N., Vesterdal, L., De Vos, B., Vanguelova, E., and Hansen, K. (2014). Tree species is the major factor explaining C:N ratios in European forest soils. For. Ecol. Manage. 311, 3–16. doi: 10.1016/j.foreco.2013.06.047
Cornelissen, J., Aerts, R., Cerabolini, B., Werger, M., and van der Heijden, M. (2001). Carbon cycling traits of plant species are linked with mycorrhizal strategy. Oecologia 129, 611–619. doi: 10.1007/s004420100752
Cornwell, W. K., Cornelissen, J. H. C., Amatangelo, K., Dorrepaal, E., Eviner, V. T., Godoy, O., et al. (2008). Plant species traits are the predominant control on litter decomposition rates within biomes worldwide. Ecol. Lett. 11, 1065–1071. doi: 10.1111/j.1461-0248.2008.01219.x
Craig, M. E., Lovko, N., Flory, S. L., Wright, J. P., and Phillips, R. P. (2019). Impacts of an invasive grass on soil organic matter pools vary across a tree-mycorrhizal gradient. Biogeochemistry 144, 149–164. doi: 10.1007/s10533-019-00577-2
Cramer, W., Bondeau, A., Woodward, F. I., Prentice, I. C., Betts, R. A., Brovkin, V., et al. (2001). Global response of terrestrial ecosystem structure and function to CO2 and climate change: results from six dynamic global vegetation models. Glob. Chang. Biol. 7, 357–373. doi: 10.1046/j.1365-2486.2001.00383.x
Crowley, K. F., and Lovett, G. M. (2017). Effects of nitrogen deposition on nitrate leaching from forests of the northeastern United States will change with tree species composition. Can. J. For. Res. 47, 997–1009. doi: 10.1139/cjfr-2016-0529
Crowley, K. F., Lovett, G. M., Arthur, M. A., and Weathers, K. C. (2016). Long-term effects of pest-induced tree species change on carbon and nitrogen cycling in northeastern U.S. forests: a modeling analysis. For. Ecol. Manage. 372, 269–290. doi: 10.1016/j.foreco.2016.03.045
Fernandez, C. W., See, C. R., and Kennedy, P. G. (2019). Decelerated carbon cycling by ectomycorrhizal fungi is controlled by substrate quality and community composition. New Phytol. 226, 569–582. doi: 10.1111/nph.1626
Finzi, A. C., Canham, C. D., and Van Breemen, N. (1998a). Canopy tree-soil interactions within temperate forests: species effects on pH and cations. Ecol. Appl. 8, 447–454. doi: 10.1890/1051-0761(1998)008[0447:CTSIWT]2.0.CO;2
Finzi, A. C., Van Breemen, N., and Canham, C. D. (1998b). Canopy tree-soil interactions within temperate forests: species effects on soil carbon and nitrogen. Ecol. Appl. 8, 440–446. doi: 10.1890/1051-0761(1998)008[0440:CTSIWT]2.0.CO;2
Gadgil, R. L., and Gadgil, P. D. (1971). Mycorrhiza and litter decomposition. Nature 233:133. doi: 10.1038/233133a0
Gadgil, R. L., and Gadgil, P. D. (1975). Suppression of litter decomposition by mycorrhizal roots of Pinus radiata. New Zeal. J. For. Sci. 5, 33–41.
German, D. P., Weintraub, M. N., Grandy, A. S., Lauber, C. L., Rinkes, Z. L., and Allison, S. D. (2011). Optimization of hydrolytic and oxidative enzyme methods for ecosystem studies. Soil Biol. Biochem. 43, 1387–1397. doi: 10.1016/j.soilbio.2011.03.017
Gundersen, P., Callesen, I., and De Vries, W. (1998). Nitrate leaching in forest ecosystems is related to forest floor C/N ratios. Environ. Pollut. 102, 403–407. doi: 10.1016/S0269-7491(98)80060-2
Hobbie, S. E., Reich, P. B., Oleksyn, J., Ogdahl, M., Zytkowiak, R., Hale, C., et al. (2006). Tree species effects on decomposition and forest floor dynamics in a common garden. Ecology 87, 2288–2297. doi: 10.1890/0012-9658(2006)87[2288:TSEODA]2.0.CO;2
Hood-Nowotny, R., Hinko-Najera Umana, N., Inselbacher, E., Oswald-Lachouani, P., and Wanek, W. (2010). Alternative methods for measuring inorganic, organic, and total dissolved nitrogen in soil. Soil Sci. Soc. Am. J. 74, 1018–1027. doi: 10.2136/sssaj2009.0389
Jenny, H. (1941). Factors of Soil Formation: a System of Quantitative Pedology. New York, NY: Dover Publications.
Jo, I., Fei, S., Oswalt, C. M., Domke, G. M., and Phillips, R. P. (2019). Shifts in dominant tree mycorrhizal associations in response to anthropogenic impacts. Sci. Adv. 5:eaav6358. doi: 10.1126/sciadv.aav6358
Keller, A. B., and Phillips, R. P. (2019). Leaf litter decay rates differ between mycorrhizal groups in temperate, but not tropical, forests. New Phytol. 222, 556–564. doi: 10.1111/nph.15524
Kuznetsova, A., Brockhoff, P. B., and Christensen, R. H. B. (2017). lmerTest package: tests in linear mixed effects models. J. Stat. Softw. 82, 1–26. doi: 10.18637/jss.v082.i13
Lang, A. K., Jevon, F. V., Ayres, M. P., and Hatala Matthes, J. (2019). Higher soil respiration rate beneath arbuscular mycorrhizal trees in a northern hardwood forest is driven by associated soil properties. Ecosystems. doi: 10.1007/s10021-019-00466-7
Lankau, R. A., Zhu, K., and Ordonez, A. (2015). Mycorrhizal strategies of tree species correlate with trailing range edge responses to current and past climate change. Ecology 96, 1451–1458. doi: 10.1890/14-2419.1
Liese, R., Lübbe, T., Albers, N. W., and Meier, I. C. (2018). The mycorrhizal type governs root exudation and nitrogen uptake of temperate tree species. Tree Physiol. 38, 83–95. doi: 10.1093/treephys/tpx131
Lin, G., Guo, D., Li, L., Ma, C., and Zeng, D. H. (2018). Contrasting effects of ectomycorrhizal and arbuscular mycorrhizal tropical tree species on soil nitrogen cycling: the potential mechanisms and corresponding adaptive strategies. Oikos 127, 518–530. doi: 10.1111/oik.04751
Lin, G., McCormack, M. L., Ma, C., and Guo, D. (2017). Similar below-ground carbon cycling dynamics but contrasting modes of nitrogen cycling between arbuscular mycorrhizal and ectomycorrhizal forests. New Phytol. 213, 1440–1451. doi: 10.1111/nph.14206
Lindahl, B. D., and Tunlid, A. (2015). Ectomycorrhizal fungi - potential organic matter decomposers, yet not saprotrophs. New Phytol. 205, 1443–1447. doi: 10.1111/nph.13201
Lovett, G. M., Weathers, K. C., Arthur, M. A., and Schultz, J. C. (2004). Nitrogen cycling in a northern hardwood forest: do species matter? Biogeochemistry 67, 289–308. doi: 10.1023/B:BIOG.0000015786.65466.f5
Lu, M., and Hedin, L. O. (2019). Global plant–symbiont organization and emergence of biogeochemical cycles resolved by evolution-based trait modelling. Nat. Ecol. Evol. 3, 239–250. doi: 10.1038/s41559-018-0759-0
Lusk, C. H., Wright, I., and Reich, P. B. (2003). Photosynthetic differences contribute to competitive advantage of evergreen angiosperm trees over evergreen conifers in productive habitats. New Phytol. 160, 329–336. doi: 10.1046/j.1469-8137.2003.00879.x
Maaroufi, N. I., Nordin, A., Palmqvist, K., Hasselquist, N. J., Forsmark, B., Rosenstock, N. P., et al. (2019). Anthropogenic nitrogen enrichment enhances soil carbon accumulation by impacting saprotrophs rather than ectomycorrhizal fungal activity. Glob. Chang. Biol. 25, 2900–2914. doi: 10.1111/gcb.14722
McDaniel, M. D., Kaye, J. P., and Kaye, M. W. (2013). Increased temperature and precipitation had limited effects on soil extracellular enzyme activities in a post-harvest forest. Soil Biol. Biochem. 56, 90–98. doi: 10.1016/j.soilbio.2012.02.026
McDaniel, M. D., Kaye, J. P., Kaye, M. W., and Bruns, M. A. (2014). Climate change interactions affect soil carbon dioxide efflux and microbial functioning in a post-harvest forest. Oecologia 174, 1437–1448. doi: 10.1007/s00442-013-2845-y
Midgley, M. G., and Phillips, R. P. (2016). Resource stoichiometry and the biogeochemical consequences of nitrogen deposition in a mixed deciduous forest. Ecology 97, 3369–3377. doi: 10.1002/ecy.1595
Morton Arboretum Staff (1929). Report on forestry plots. Bull. Pop. Inf. 4, 23–26. Available online at: https://acorn.mortonarb.org/Detail/objects/32509 (accessed June 16, 2020).
Mueller, K. E., Hobbie, S. E., Oleksyn, J., Reich, P. B., David, M., Mueller, K. E., et al. (2016). Do evergreen and deciduous trees have different effects on net N mineralization in soil? Ecology 93, 1463–1472. doi: 10.1890/11-1906.1
Pellitier, P. T., and Zak, D. R. (2018). Ectomycorrhizal fungi and the enzymatic liberation of nitrogen from soil organic matter: why evolutionary history matters. New Phytol. 217, 68–73. doi: 10.1111/nph.14598
Phillips, R. P., Brzostek, E., and Midgley, M. G. (2013). The mycorrhizal-associated nutrient economy: a new framework for predicting carbon-nutrient couplings in temperate forests. New Phytol. 199, 41–51. doi: 10.1111/nph.12221
Phillips, R. P., and Fahey, T. J. (2006). Tree species and mycorrhizal associations influence the magnitude of rhizosphere effects. Ecology 87, 1302–1313. doi: 10.1890/0012-9658(2006)871302
Read, D. J., and Perez-Moreno, J. (2003). Mycorrhizas and nutrient cycling in ecosystems - a journey towards relevance? New Phytol. 157, 475–492. doi: 10.1046/j.1469-8137.2003.00704.x
Reich, P. B. (2014). The world-wide ‘fast-slow’ plant economics spectrum: a traits manifesto. J. Ecol. 102, 275–301. doi: 10.1111/1365-2745.12211
Reich, P. B., and Oleksyn, J. (2004). Global patterns of plant leaf N and P in relation to temperature and latitude. Proc. Natl. Acad. Sci. U.S.A. 101, 11001–11006. doi: 10.1073/pnas.0403588101
Ribbons, R. R., Kepfer-Rojas, S., Kosawang, C., Hansen, O. K., Ambus, P., McDonald, M., et al. (2018). Context-dependent tree species effects on soil nitrogen transformations and related microbial functional genes. Biogeochemistry 140, 145–160. doi: 10.1007/s10533-018-0480-8
Rollinson, C. R., Kaye, M. W., and Leites, L. P. (2012). Community assembly responses to warming and increased precipitation in an early successional forest. Ecosphere 3, 1–17. doi: 10.1890/ES12-00321.1
Saiya-Cork, K., Sinsabaugh, R., and Zak, D. (2002). The effects of long term nitrogen deposition on extracellular enzyme activity in an acer saccharum forest soil. Soil Biol. Biochem. 34, 1309–1315. doi: 10.1016/S0038-0717(02)00074-3
Scott, N. A., and Binkley, D. (1997). Foliage litter quality and annual net N mineralization: comparison across North American forest sites. Oecologia 111, 151–159. doi: 10.1007/s004420050219
See, C. R., Luke McCormack, M., Hobbie, S. E., Flores-Moreno, H., Silver, W. L., and Kennedy, P. G. (2019). Global patterns in fine root decomposition: climate, chemistry, mycorrhizal association and woodiness. Ecol. Lett. 22, 946–953. doi: 10.1111/ele.13248
Silver, W. L., and Miya, R. K. (2001). Global patterns in root decomposition: comparisons of climate and litter quality effects. Oecologia 129, 407–419. doi: 10.1007/s004420100740
Sims, G. K., Ellsworth, T. R., and Mulvaney, R. L. (1995). Microscale determination of inorganic nitrogen in water and soil extracts. Commun. Soil Sci. Plant Anal. 26, 303–316. doi: 10.1080/00103629509369298.
Slessarev, E. W., Lin, Y., Bingham, N. L., Johnson, J. E., Dai, Y., Schimel, J. P., et al. (2016). Water balance creates a threshold in soil pH at the global scale. Nature 540, 567–569. doi: 10.1038/nature20139
Smith, G. R., and Wan, J. (2019). Resource-ratio theory predicts mycorrhizal control of litter decomposition. New Phytol. 223, 1595–1606. doi: 10.1111/nph.15884
Soil Survey Staff and Natural Resources Conservation Service United States Department of Agriculture (2019). Web Soil Survey. Soil Survey Staff and Natural Resources Conservation Service United States Department of Agriculture.
Ste-Marie, C., and Paré, D. (1999). Soil, pH and N availability effects on net nitrification in the forest floors of a range of boreal forest stands. Soil Biol. Biochem. 31, 1579–1589. doi: 10.1016/S0038-0717(99)00086-3
Sulman, B. N., Brzostek, E. R., Medici, C., Shevliakova, E., Menge, D. N. L., and Phillips, R. P. (2017). Feedbacks between plant N demand and rhizosphere priming depend on type of mycorrhizal association. Ecol. Lett. 20, 1043–1053. doi: 10.1111/ele.12802
Sulman, B. N., Shevliakova, E., Brzostek, E. R., Kivlin, S. N., Malyshev, S., Menge, D. N. L., et al. (2019). Diverse mycorrhizal associations enhance terrestrial C storage in a global model. Global Biogeochem. Cycles 33, 501–523. doi: 10.1029/2018GB005973
Sun, T., Hobbie, S. E., Berg, B., Zhang, H., Wang, Q., Wang, Z., et al. (2018). Contrasting dynamics and trait controls in first-order root compared with leaf litter decomposition. Proc. Natl. Acad. Sci. U.S.A. 115, 10392–10397. doi: 10.1073/pnas.1716595115
Talbot, J. M., Allison, S. D., and Treseder, K. K. (2008). Decomposers in disguise: mycorrhizal fungi as regulators of soil C dynamics in ecosystems under global change. Funct. Ecol. 22, 955–963. doi: 10.1111/j.1365-2435.2008.01402.x
Taylor, M. K., Lankau, R. A., and Wurzburger, N. (2016). Mycorrhizal associations of trees have different indirect effects on organic matter decomposition. J. Ecol. 104, 1576–1584. doi: 10.1111/1365-2745.12629
Tedersoo, L., and Bahram, M. (2019). Mycorrhizal types differ in ecophysiology and alter plant nutrition and soil processes. Biol. Rev. 94, 1857–1880. doi: 10.1111/brv.12538
Tedersoo, L., and Brundrett, M. C. (2017). “Evolution of ectomycorrhizal symbiosis in plants.” in Biogeography of Mycorrhizal Symbiosis, Ecological Studies (Analysis and Synthesis) ed L. Tedersoo (Cham: Springer), 407–467. doi: 10.1007/978-3-319-56363-3_19
Vance, E. D., Brookes, P. C., and Jenkinson, D. S. (1987). An extraction method for measuring soil microbial biomass C. Soil Biol. Biochem. 19, 703–707. doi: 10.1016/0038-0717(87)90052-6
Vesterdal, L., Schmidt, I. K., Callesen, I., Nilsson, L. O., and Gundersen, P. (2008). Carbon and nitrogen in forest floor and mineral soil under six common European tree species. For. Ecol. Manage. 255, 35–48. doi: 10.1016/j.foreco.2007.08.015
Wang, B., and Qiu, Y. L. (2006). Phylogenetic distribution and evolution of mycorrhizas in land plants. Mycorrhiza 16, 299–363. doi: 10.1007/s00572-005-0033-6
Wang, X., and Wang, C. (2018). Mycorrhizal associations differentiate soil respiration in five temperate monocultures in Northeast China. For. Ecol. Manage. 430, 78–85. doi: 10.1016/j.foreco.2018.08.001
Watts, M. T. (1942). Evergreen Trail Map and Work Sheet Planned to Accompany Evergreen Trail Guide Booklet. Lisle, IL. Available online at: https://acorn.mortonarb.org/Detail/objects/24653 (accessed June 16, 2020).
Wilhelm, G. S. (1991). “Implications of changes in floristic composition of the Morton Arboretum's East Woods,” in Proceedings of the Oak Woods Management Workshop, eds G. Burger, J. Ebinger, and G. Wilhelm (Eastern Illinois University, Charleston, IL), 31–54.
Yin, H., Wheeler, E., and Phillips, R. P. (2014). Root-induced changes in nutrient cycling in forests depend on exudation rates. Soil Biol. Biochem. 78, 213–221. doi: 10.1016/j.soilbio.2014.07.022
Zhang, H.-Y., Lü, X.-T., Hartmann, H., Keller, A., Han, X.-G., Trumbore, S., et al. (2018). Foliar nutrient resorption differs between arbuscular mycorrhizal and ectomycorrhizal trees at local and global scales. Glob. Ecol. Biogeogr. 27, 875–885. doi: 10.1111/geb.12738
Keywords: common garden, functional groups, nutrient economy, plant-soil interactions, soil ecology
Citation: Midgley MG and Sims RS (2020) Mycorrhizal Association Better Predicts Tree Effects on Soil Than Leaf Habit. Front. For. Glob. Change 3:74. doi: 10.3389/ffgc.2020.00074
Received: 20 December 2019; Accepted: 21 May 2020;
Published: 30 June 2020.
Edited by:
Peyton Smith, Texas A&M University, United StatesReviewed by:
Ingeborg Callesen, University of Copenhagen, DenmarkNadia Maaroufi, University of Bern, Switzerland
Copyright © 2020 Midgley and Sims. This is an open-access article distributed under the terms of the Creative Commons Attribution License (CC BY). The use, distribution or reproduction in other forums is permitted, provided the original author(s) and the copyright owner(s) are credited and that the original publication in this journal is cited, in accordance with accepted academic practice. No use, distribution or reproduction is permitted which does not comply with these terms.
*Correspondence: Meghan G. Midgley, bW1pZGdsZXkmI3gwMDA0MDttb3J0b25hcmIub3Jn