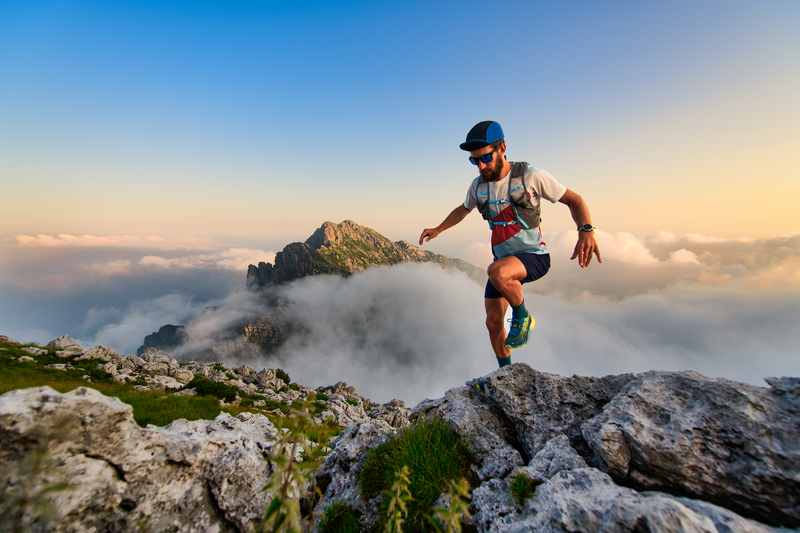
95% of researchers rate our articles as excellent or good
Learn more about the work of our research integrity team to safeguard the quality of each article we publish.
Find out more
ORIGINAL RESEARCH article
Front. For. Glob. Change , 24 September 2019
Sec. Temperate and Boreal Forests
Volume 2 - 2019 | https://doi.org/10.3389/ffgc.2019.00058
We studied the seasonal dynamics of xylem and phloem formation in four boreal tree species that differed in leaf phenology (evergreen vs. winter-deciduous) and wood anatomy (angiosperms vs. gymnosperms). We sampled branch cuttings of balsam poplar (Populus balsamifera), trembling aspen (Populus tremuloides), lodgepole pine (Pinus contorta), and Siberian larch (Larix siberica) in bi-weekly intervals from the beginning to the end of the growing season. Cross sections were stained with astra blue and safranin in order to assess the width of the current year's phloem and to distinguish between the current year's mature and enlarging/wall-thickening xylem. After enzymatic clearance of cytoplasmic components, we used scanning electron microscopy to observe seasonal patterns of callose deposition in sieve plates of balsam poplar. The sieve plate pores were not blocked by callose in April (prior to the start of the growing season) and in July. In October, after the end of the growing season, we found only minor callose accumulation, and only in a subset of samples. In three of the four species studied here, phloem formation in spring began before the start of xylogenesis, but the lag was shorter than what was previously reported for temperate environments with longer growing seasons. New xylem cells were first produced in trembling aspen, followed by lodgepole pine, balsam poplar and Siberian larch. Most of the xylem was produced in June and July, and all cells were mature in early September. Phloem production was mostly completed by early August. Balsam poplar had the shortest growing season in terms of cambial activity and leaf presence, suggesting a risk avoidance strategy with regards to frost damage.
The growth of boreal tree species is limited by their short growing season. Differences among species can be observed in leaf phenology and seasonal dynamics of cold hardiness (Sakai and Weiser, 1973; Morin et al., 2009; Chuine, 2010). Within species, susceptibility to late and early frost events differs with latitude of the seed source (e.g., Montwé et al., 2018). Winter temperatures in the boreal forest often exceed −40°C and at the same time can frequently rise above 0°C for short periods (Schreiber et al., 2013b). Such freeze-thaw events expose boreal tree species to significant mechanical stress in their wood. Frost damage often affects the wood in the form of cankers and frost-cracked stems as well as through dieback when living cells are permanently damaged by ice formation in the protoplasm (Burke et al., 1976; Zalasky, 1976). Woody trees in the boreal forest, however, can cope with this through various physiological adaptations such as the timing of bud break and leaf senescence (Howe et al., 2003) as well as by dehydrating living cells to prevent ice formation (Burke et al., 1976).
Much of the carbon produced by trees is transported in the phloem and is then used by the vascular cambium to produce vascular tissue, primarily wood. Since the cambium is a large carbon sink in trees, it requires the delivery of carbohydrates from leaves and/or ray cells. Utilizing reserve carbohydrates, proteins, and lipids from ray cells is especially important in spring when buds open and leaves unfold (Zamski and Zimmermann, 1979; Sauter and van Cleve, 1994; Sauter, 2000; Savidge et al., 2000; Sanz-Perez et al., 2009; Plavcová and Jansen, 2015). Leaf phenology, vascular transport, and cambial activity are all linked, not just via photosynthesis and the subsequent transport and consumption of carbohydrates, but also by the hormonal signals that are originating in expanding buds and leaves. Auxin in particular is likely to play a key role in synchronizing leaf and cambial development (Hacke et al., 2017; Johnson et al., 2018).
Here, we study the seasonal dynamics of vascular tissue production in four boreal tree species that differ in leaf phenology (deciduous vs. evergreen) and vascular tissue anatomy (i.e., sieve cells and tracheids in conifers vs. sieve tubes and vessels in angiosperms). Previous studies focused on xylogenesis (e.g., Rossi et al., 2011), while much less is known about the seasonal dynamics of phloem production. This is surprising, given the importance of the phloem in delivering photosynthates to the cambium and the role of the phloem in distributing signaling molecules across plant organs (Lucas et al., 2013). Much of what we know about seasonal phloem development comes from the pioneering studies of Evert and co-workers (Evert, 1990, and literature cited therein); only recently have researchers focused their attention on the phloem again (e.g., Prislan et al., 2018).
Seasonal changes of xylem transport capacity in trees have been measured in many studies (Sperry et al., 1988, 1994; Hacke and Sauter, 1996), providing us with a fairly thorough understanding of trade-offs and transport challenges. By contrast, questions remain about the seasonal dynamics of phloem transport. In many temperate woody angiosperm species, the sieve elements “function only during the season in which they are formed, becoming [irreversibly] non-functional in the fall” (Evert, 2006, p. 392). In other woody angiosperms, sieve elements may function for two or more seasons, becoming dormant in late fall and becoming reactivated in spring (Evert, 1990, 2006).
Callose deposition on sieve plates has a major influence on phloem transport rates (Stanfield et al., 2018), so it would follow that this may be an indication of transport cessation. To date, few studies have demonstrated changes in callose deposition on sieve plates on a seasonal basis (but see Davis and Evert, 1970; Prislan et al., 2018). Given that seasonal callose deposition remains poorly documented, many questions remain regarding the functionality of callose deposition on sieve plates. For example, at what time of year is callose deposited on sieve plates? How does this deposition coincide with the halt of seasonal phloem development, and do overwintered sieve tubes become reactivated by removing callose from plates? Despite its potential importance, we currently lack a comprehensive understanding of the plant-internal and environmental cues that may trigger callose formation in sieve plates at the end of the growing season. A good first step is to understand when this formation begins.
In the present study, we collected young branches from four boreal tree species at regular intervals throughout the 2018 growing season to study the development of the xylem and phloem and to assess the phenology of the leaves. We studied two angiosperms, balsam poplar (Populus balsamifera) and trembling aspen (Populus tremuloides; “aspen” from here on), and two conifers, lodgepole pine (Pinus contorta), and Siberian larch (Larix siberica). Balsam poplar and aspen both have a wide distribution across North America (Peterson and Peterson, 1992). While the northern limits are similar for both species, aspen's distribution extends into Mexico, while balsam poplar only reaches into the northern United States. Lodgepole pine is an evergreen conifer and occurs across western North America, separated into three subspecies. We studied the interior subspecies, P. contorta subsp. latifolia, which has a northern distribution limit in the Yukon Territory (Little, 1971). Siberian larch is a deciduous conifer; it originates from central Russia and has been introduced to Canada mainly as an ornamental plant (Shuman et al., 2011).
Our first objective was to assess whether species had different patterns of vascular tissue production over the growing season. We expected to find differences between the two conifer species due to their contrasting leaf phenology. The production of new leaves may bind resources and may therefore cause a delay in the production of xylem and phloem. Our second objective was to better understand how phloem production coincides with xylogenesis. Based on previous work on diffuse-porous species (Tucker and Evert, 1969) and conifers (Alfieri and Evert, 1968; Swidrak et al., 2014), we hypothesized that phloem cell production would start before xylem cell production. Due to the short growing season in boreal environments, however, we expected that the lag in xylem development would be shorter than what has been described for temperate regions. The third objective was to study if sieve pores in the sieve tubes of the most recent phloem increment would be blocked by callose prior to the start, during, and after the end of the growing season. We hypothesized that sieve pores would be blocked before and after the growing season, but that they would be open during the summer. Due to the time-consuming nature of these measurements, this was only evaluated in one species, balsam poplar.
Seven sampling sites were selected: Three sites represent pairs of balsam poplar and aspen, three sites covered pairs of lodgepole pine and Siberian larch (Supplementary Table 1). Due to limited resources and logistics, one additional site was selected in close proximity to the University of Alberta to infer callose formation over the season in balsam poplar. The sampling sites were within the city limits of Edmonton, Alberta, Canada, located near the edge of the Canadian prairies and the boreal plains. Climate data was retrieved from the University of Alberta Climate Station (Station code: CA00301FFNJ, 53.52N, −113.53E, 668.0 m). The climate of this region is continental, with an average annual temperature of 2.2°C and an average coldest month temperature of −14.7°C. This region is also characterized by a relatively low annual precipitation of 466 mm, although most of this precipitation occurs during summer. At each site, one to five twigs from one tree per species were cut at ~2 m in height. We aimed to standardize sampling as much as possible, targeting 2–3 year old twigs of similar diameter. On average, sampled twigs were 2.92 years old, with a standard error of 0.15. The average twig diameter was 3.93 mm with a standard error of 0.13. A mixed model, implemented with lmerTest (Kuznetsova et al., 2017), with species as a fixed effect and unique tree id as a random effect, did not show significant differences in twig age or twig diameter (p = 0.5754 and p = 0.4773). Trees ranged in approximate age from 30 to 60 years, 8–18 m in height, and 15–30 cm in stem diameter at 1.3 m height. Sampling began prior to flushing, with the first sampling date occurring on April 8th, 2018. Sampling continued every second week until after snowfall, with the last sampling date occurring on September 24th, 2018.
Sampling of five balsam poplars for callose formation assessment occurred three times during the growing season: Prior to leaf flush (April), in the middle of the growing season (July) and after the growing season had finished (October). Since callose may form rapidly after cutting (Evert, 1990; Mullendore et al., 2010; Kalmbach and Helariutta, 2019), these samples were placed in liquid nitrogen for 1 min immediately after cutting. They were then placed in test tubes filled with chilled 100% ethanol, again submerged in liquid nitrogen for 1 min, and transported on ice to the laboratory.
In the laboratory, sections of ~2–3 cm in length were cut from the twigs, placed in tubes filled with isopropanol alcohol and stored under cool conditions (1–2°C) until further processing. Thin cross-sections, between 10 and 20 μm in thickness, were cut from each of these twig sections using a GSL-microtome (Gärtner et al., 2014). These micro-sections were stained with a 50:50 mixture of astra blue and safranin. Astra blue dyes cellulose blue while safranin stains lignified cell walls, and hence, tissue lacking lignin results in a blue stain. After staining, the micro-sections were washed with distilled water and dehydrated first with ethanol and then with isopropanol. After excess isopropanol had evaporated, the sections were embedded using Canada balsam, which was preheated to decrease viscosity for easier application (Isaac-Renton et al., 2018). The glass slides were dried in an oven at 60°C for 12–24 h. Using a Leica DM3000 microscope at 200× magnification, digital images were taken of each micro-section, capturing all cells from the pith to the bark. Single images were stitched with Microsoft Image Composer (Microsoft Corporation).
To assess the seasonal development of the xylem and phloem rings of 2018, the width in μm of these tissues was measured in ImageJ on calibrated micrographs. Only the phloem increment of 2018 was measured, without separating the phloem ring into early and late phloem. The phloem increment of 2018 was identified by the presence of large phloem cells being produced after the smaller late phloem cells of the previous year. The xylem ring was separated into mature xylem and a combined category for enlarging/wall-thickening xylem based on the color of the tissue after double staining with safranin and astra blue (Gärtner and Schweingruber, 2013). Following Čufar et al. (2008), we classified xylem cells as mature when they were clear of cell contents and when they had walls that were completely stained red. We assigned tracheids or vessels to the enlarging/wall-thickening xylem when cell content was present in the lumen and/or when the walls were stained partially or fully blue (Figure 1).
Figure 1. Micro-sections of trembling aspen (A) and lodgepole pine (B) branches collected on June 17, 2018. Red stain is from safranin and indicates lignified tissue. Blue stain is from astra blue and indicates tissue that has not (yet) been lignified. In aspen, mature fibers and vessels are stained red while rays and axial parenchyma cells are blue. There was a narrow zone near the cambium in which the color of fiber and vessel walls changed from blue to red; i.e., cell walls underwent lignification. The 2018 phloem increment already had some large, more or less circular sieve tubes and a distinct layer of fibers. By contrast, sieve tubes that were formed in the previous year (2017) looked crushed. In lodgepole pine, there was a very narrow band of enlarging/wall-thickening tracheids near the cambium. Phloem cells produced in 2018 looked functional while phloem formed in the previous year appeared crushed.
At each of the biweekly sampling dates, the leaf development stage was assessed to derive dates for budburst, bud set and leaf senescence following Way (2011) and protocols outlined in Wesolowski and Rowinski (2006). Budburst was defined as the beginning of leaf unfolding. In cases where the leaves had already unfolded, approximate dates of budburst were estimated based on the stage of unfolding. Leaf senescence was defined as the date on which most of the leaves appeared yellow. In cases where trees had turned yellow within the biweekly sampling window, the senescence date was approximated based on the proportion of yellow leaves.
Sieve plates were imaged according to the protocol of Mullendore et al. (2010) to assess seasonal callose formation. After samples were collected they were stored in a −20°C freezer for 24 h. After overnight freezing, stem samples were thawed for 2 h at room temperature. Next, the segments were put into fresh deionized water and allowed to sit for 2 h. Samples were then rinsed twice more in deionized water, and cut into ~1 mm thick transverse sections using a fresh razor blade. The cut segments were then put into a 0.1% proteinase K (Life Technologies, Carlsbad, California, USA) protein digestion solution for 2–3 weeks. Samples were kept on a hot plate at 55°C and shaken at 100 rpm using an orbital shaker. Sample tubes were inverted daily. After this enzyme treatment, samples were washed in 100% ethanol before rinsing in deionized water. Next, samples were put in a 0.1% amylase solution for 24 h at 60°C to digest any starch from the sieve plates. After, samples were freeze-dried overnight, placed upon aluminum stubs and sputter coated with gold for Scanning Electron Microscopy (SEM) using a Zeiss EVO MA 15; accelerating voltage on the microscope was set to 20 kV and accelerating current to 50 pA.
Gompertz functions were fit to the tissue widths averaged over the three individual trees per species (Rossi et al., 2003). The parameters for the Gompertz functions were estimates using the non-linear weighted least square approach as implemented in the nls() function of the R base package. Initial values for the models were estimated with the nls_multstart() function of the nls.multstart package. Critical dates such as the start and end of cambial activity were extracted from the curves, based on threshold values (Rossi et al., 2003; Rathgeber et al., 2018). The start of xylem and phloem formation were estimated as the day of year at which 1% of the curve maximum was exceeded. The end of xylem formation was specified as the day of year at which the width exceeded 90% of the curve maximum. For these critical dates, standard errors were calculated based on the model fits using the Delta method as implemented in the car package for R. Based on these standard errors, z-tests were conducted to test for significant differences among species. P-values were adjusted for the false discovery rate with the Benjamini & Hochberg method (Benjamini and Hochberg, 1995).
Species differed slightly in the start date of wood formation (Figures 2, 3C, Table 1). Lodgepole pine was first to start xylogenesis, followed by aspen and Siberian larch. Balsam poplar took significantly longer to start forming new xylem (Figures 2, 3C, Table 1). Notably, the start of wood formation varied by 13 days among species. The time from the start of wood formation, to the first mature xylem was similar among species. Mature xylem occurred after 12 days in aspen, and 13 days in balsam poplar and larch, and 11 days in pine. Balsam poplar was first to cease wood formation, followed by lodgepole pine and aspen. Siberian larch formed wood latest into the fall. Consequently, species differed in the length of the wood formation period, with balsam poplar having the shortest and Siberian larch having the longest growing season.
Figure 2. Timing of xylem and phloem traits in four boreal tree species by day of year over a growing season inferred through Gompertz fits. The width of total xylem is indicated by the solid lines. Mature xylem that has completed the enlarging and wall-thickening states is indicated as dashed lines. The width of the 2018 phloem increment is indicated by dotted lines. Species are aspen, balsam poplar, Siberian larch, and lodgepole pine. The vertical lines, from left to right, show the time of budburst, bud set, and leaf senescence (not shown for lodgepole pine, which is evergreen).
Figure 3. Climate related to critical dates for leaf phenology, phloem and wood formation. (A) Bars represent daily precipitation sums for the period of 1987–2017 (light blue) and the year 2018 (dark blue). (B) Lines represent 5 days running means of the previous 5 days of maximum, average, and minimum temperature for the period of 1987–2017 (light blue) and the year 2018 (dark blue). (C) Dots indicate the date of activity. The dates are based on Gompertz-fits to biweekly measurements of xylem and phloem development. The dates for leaf phenology are based on biweekly assessments of leaf formation and senescence. Dots are colored by species. Of all four species, balsam poplar had the shortest growing season as defined by the period between the start and end of wood formation and the duration of leaf presence while larch had the longest duration of wood formation and leaf presence. The start of wood formation and the date of bud break were similar in all species. By contrast, the end of phloem formation, wood formation, and the date of bud set were highly variable across species.
Table 1. Multiple comparisons among species in dates (Day of Year) of phloem and wood formation as well as leaf phenology.
Siberian larch and aspen were the first species to produce new phloem cells, followed by lodgepole pine. Balsam poplar started the latest to produce new phloem. Phloem formation started 27 and 19 days earlier than wood formation in larch and aspen, respectively. By contrast, phloem and xylem production started at a similar date in balsam poplar and lodgepole pine (Figures 2, 3). However, these two species differed considerably in the magnitude of phloem production. Lodgepole pine produced new phloem more quickly, with the majority being formed after 31 days.
The time of budbreak was very similar in all species (Figure 3). Nonetheless, significant differences existed within this narrow timeframe, which is mainly attributed to a later start of balsam poplar (Table 1). Lodgepole pine broke bud first, followed by Siberian larch and aspen (Figure 3). Siberian larch set bud latest (Figure 3). Similarly, leaf senescence occurred last for Siberian larch. Balsam poplar lost their leaves first, followed by aspen. After adjustment for multiple comparisons, significant differences were found among species (Table 1). Budbreak and the beginning of wood formation coincided in all species. The beginning of phloem formation was the first developmental process we could document in spring (Figure 3C).
Precipitation patterns in the study area are characterized by generally low rainfall, with occasional heavy rain events (Figure 3A) caused by thunderstorms. In the year 2018, there were notable snow or rain events on April 11th, May 31st, and June 10th. These were interrupting longer periods without substantial precipitation. Compared to the 30-years climate average, the year 2018 was characterized by a cold spell with minimum temperatures of −20°C in early April, followed by a steep increase of temperatures in the middle of April (Figure 3B). This was followed by above-average temperatures during the summer. In the fall, temperatures dropped sharply around mid September, with the first frost occurring shortly after. All four species mostly avoided the cold conditions at the beginning and end of the growing season in terms of xylem and phloem formation activity (comparing Figures 3B,C). In case of Siberian larch and aspen, phloem formation began on the upward slope of a cold spell in early April. However, bud break occurred after minimum temperatures had increased beyond 0°C. In contrast, leaf senescence appears to have been triggered by cool temperatures in the fall, as full senescence was reached only after the first frost.
Scanning electron microscopy was used to assess callose formation on the sieve plates of sieve elements of five balsam poplar trees (Figure 4). The first phloem samples were collected in April, prior to the beginning of wood formation. We only found open sieve pores without callose accumulations blocking or narrowing the sieve pores in the 2017 phloem (Figures 4A,B). The second samples were taken in July, in the 2018 phloem, and showed open sieve pores as well (Figures 4C,D). In October, after balsam poplar had dropped its leaves, callose was still mainly absent from sieve pores. Only a subset of samples in the 2018 phloem showed callose deposition (Figures 4E,F). For October, we found that about 20% of the investigated sieve plates had some callose formation. Callose formation was more apparent in the late phloem (26%) than in the early phloem (10%).
Figure 4. Scanning electron microscopy images showing the state of sieve plates in balsam poplar throughout the year. Samples were collected prior to the start of the growing season in April (A,B), at the peak of the growing season in July (C,D) and after the end of the growing season in October (E,F). In April and July, there was no or little callose deposition and pores were open. Samples collected in October had experienced several freeze-thaw cycles (minimum temperature: −5°C). Nonetheless, only two out of 21 observed plates in early phloem and 6 out of 23 observed plates in late phloem showed appreciable callose deposition; an example for plates with partially blocked pores is shown in F. Callose deposition will decrease translocation rates because the size and shape of the pores in sieve plates is highly influential for phloem transport capacity.
Our first objective was to evaluate if species differed in the seasonal dynamics of vascular tissue production. In all of the species considered in this study, the timing of bud burst and the start of wood formation coincided, and species differed by days, rather than weeks. This is likely caused by the boreal climate with high temperature differences between winter and summer and a short growing season, which minimizes the time difference between the start of wood formation and bud burst. Nonetheless, we found striking differences between the two co-occurring species in the genus Populus. Balsam poplar stood out as it was the last species to start with phloem and xylem formation, yet it was the first species to end wood formation.
As a result, balsam poplar had a shorter growing season compared to aspen when defined by the time period between budburst and leaf senescence as well as the duration of vascular tissue production. This suggests that balsam poplar should be less likely to experience frost damage in late summer or early autumn compared to aspen in the same location. However, this remains speculative since there are only a few studies that tested the vulnerability to frost damage directly between co-occurring aspen and balsam poplar populations (Man et al., 2014). Most studies focused on a growth rate vs. cold hardiness trade-off within species or very closely related species (Hawkins and Stoehr, 2009; Keller et al., 2011; Koehler et al., 2012; Schreiber et al., 2013a,b; Montwé et al., 2018; Sebastian-Azcona et al., 2019). Nonetheless, the frost tolerance ratings for aspen and balsam poplar are high and very high, respectively (Krajina et al., 1982). This is consistent with our findings that balsam poplar started wood and phloem formation later and ended earlier, which, in turn, reduces risk of exposure to frost in the shoulder seasons. Surprisingly, there are not many studies available that directly compare aspen and balsam poplar growth rates in order to better understand the growth characteristics of those two boreal species (Peterson and Peterson, 1992). This highlights an opportunity for further research to compare and link growth rates of these species to climatic and other abiotic factors. All four species avoided the late and early frost events in the 2018 growing season, indicating a general adaptation to the growing conditions at the sampled sites. However, Siberian larch had the longest period of wood formation and the longest leaf presence. As a non-native species, it utilized the growing season to a greater extent than the other deciduous species. This was especially apparent through the comparatively late date of leaf senescence. While budburst is more related to temperature, leaf senescence is mainly related to photoperiod (Lüttge and Hertel, 2009). While the origin of the planting stock is unknown, one could speculate about a mismatch in latitude between seed origin and planting site in Edmonton. This is because of the long period of wood and phloem formation, which would indicate an adaptation to a longer growing season. Hence, the climate of Edmonton in conjunction with this species' phenology involves greater exposure to frost damage in the spring and late summer/early fall. However, since the growing season length in boreal environments overall is projected to increase (Morin et al., 2009), this may be a beneficial strategy.
Our second objective was to determine if, and to what extent phloem production preceded xylem production. Previous studies in temperate environments found that phloem formation started up to 3–5 weeks before xylem formation in conifers (Alfieri and Evert, 1973; Gričar et al., 2014; Swidrak et al., 2014) and some diffuse-porous trees (Tucker and Evert, 1969; Davis and Evert, 1970; Prislan et al., 2013). In ring-porous species, by contrast, phloem and xylem differentiation began more or less simultaneously (Derr and Evert, 1967; Davis and Evert, 1970; Čufar et al., 2011). In three of the four species studied here, phloem formation began before the start of xylogenesis, but as hypothesized, the lag was shorter than what was reported for temperate environments with longer growing seasons. Again, balsam poplar stood out; it was the only species in which phloem and xylem production began nearly simultaneously.
We observed mature xylem after a shorter period than reported by Rossi et al. (2013), but our findings agree with Deslauriers et al. (2009) who recorded 10–14 days between the onset of xylem differentiation and the occurrence of the first mature vessels in poplar species. In contrast to these studies, we used samples from twigs as opposed to samples from the main stem. Differences in phenology among different parts of the tree are possible. However, insight into the phloem and xylem formation dynamics of twigs is valuable because of the proximity to the photosynthesizing leaves, and for the comparison with the tree physiological literature that is also often based on twigs (e.g., Hacke, 2015).
Expedited phloem development in spring serves the tree during the important period of bud burst when there may only be a small population of functional phloem cells near the cambium to support the unfolding leaves and other meristematic zones with food (Zamski and Zimmermann, 1979; Lavrič et al., 2017; Prislan et al., 2018). Radial translocation of sugars in rays will also be important at that time (Zamski and Zimmermann, 1979). The formation of new xylem may not be as urgent in diffuse-porous and conifer species, because water transport occurs in older xylem rings. The situation is different in ring-porous species, because their leaves tend to unfold later, and because xylem transport is severely impaired in older growth rings as a result of freezing-induced embolism (Zimmermann, 1983). We generally found a relatively high variability in the end of phloem and xylem formation within species. This agrees with findings of Heinrichs et al. (2007) who reported that the end of xylem cell production in six boreal tree species was “somewhat scattered among … the species,” varying by as much as 2 months. Prislan et al. (2013) suggested that this variability may be due to the physiological condition of a tree as well as external conditions.
We studied phloem development in balsam poplar in more detail to learn whether sieve plates would be blocked by callose prior to the start, during, and after the end of the growing season; this was our third objective. Davis and Evert (1970) studied the seasonal cycle of phloem development in P. tremuloides trees growing in Wisconsin, USA. They reported that all of a season's phloem increment functioned for that season only. All sieve elements were collapsed between late November and late March. Zamski and Zimmermann, 1979, p. 654) also state that “During the dormant period most sieve tubes are sealed with callose; the exception is those which are very close to the cambial zone and have not yet fully developed.”
Callose is a polysaccharide that stains blue with resorcin blue and aniline blue. It plays a critical role during the formation of plasmodesmata and sieve plate pores (Esau and Thorsch, 1985; Xie and Hong, 2011). Callose is also deposited in sieve plates in response to wounding or other kinds of injury (Evert, 1990; Kalmbach and Helariutta, 2019). Evert (1990) distinguishes between “definitive” and “dormancy” callose. Definitive callose is found on senescing sieve elements until their death. Dormancy callose may be found in sieve elements that function for two or more years. This has been described for a few woody species including Tilia americana and Vitis riparia. In these species, dormancy callose is deposited in the fall and then removed in early spring as overwintering sieve elements are reactivated (Evert, 1990).
Based on these reports, we expected to find blocked sieve plates in mid-April, three weeks prior to the beginning of cambial activity, and in mid-October, 8 weeks after wood formation ended. Trees had experienced below-freezing temperatures (−5°C) and snow earlier by the end of September. In October, some callose formation was observed, which may indicate the beginning of blocking of the sieve elements over the winter. Nonetheless, callose formation was only found in some sieve plates, indicating that freezing temperatures per se did not trigger the consistent formation of callose in the current year's phloem. Our findings agree with a recent study on Fagus sylvatica (Prislan et al., 2018). These authors found open sieve pores in phloem formed in the previous year, prior to the beginning of cambial activity in March.
We chose balsam poplar for studying callose deposition patterns, but in retrospect we caution that this species may not be representative for other boreal species. This is because phloem production began relatively late in balsam poplar (which is consistent with open sieve pores in the older phloem) and because this species had the shortest growing season of all species considered in our study. Clearly, more data using high resolution SEM imaging in a variety of species is needed to establish sieve plate over-wintering patterns.
The raw data supporting the conclusions of this manuscript will be made available by the authors, without undue reservation, to any qualified researcher.
This idea to study vascular cambium formation was conceived by SS, UH, and DM. RS contributed the callose formation component. Field and lab work was conducted by DM, RS, and SS. The analysis was done by DM and UH. The manuscript was written by DM, UH, SS, and RS and reviewed by all authors.
Funding for this study was provided by an NSERC Discovery grant to UH.
SS was employed by company EnviroStats Solutions Inc.
The remaining authors declare that the research was conducted in the absence of any commercial or financial relationships that could be construed as a potential conflict of interest.
The Supplementary Material for this article can be found online at: https://www.frontiersin.org/articles/10.3389/ffgc.2019.00058/full#supplementary-material
Alfieri, F. J., and Evert, R. F. (1968). Seasonal development of the secondary phloem in Pinus. Am. J. Bot. 55, 518–528. doi: 10.1002/j.1537-2197.1968.tb07407.x
Alfieri, F. J., and Evert, R. F. (1973). Structure and seasonal development of the secondary phloem in the Pinaceae. Bot. Gaz. 134, 17–25. doi: 10.1086/336674
Benjamini, Y., and Hochberg, Y. (1995). Controlling the false discovery rate—a practical and powerful approach to multiple testing. J. R. Stat. Soc. Series B Stat. Methodol. 57, 289–300. doi: 10.1111/j.2517-6161.1995.tb02031.x
Burke, M. J., Gusta, L. V., Quamme, H. A., Weiser, C. J., and Li, P. H. (1976). Freezing and injury in plants. Annu. Rev. Plant Physiol. Plant Mol. Biol. 27, 507–528. doi: 10.1146/annurev.pp.27.060176.002451
Chuine, I. (2010). Why does phenology drive species distribution? Phil. Trans. R. Soc. B Biol. Sci. 365, 3149–3160. doi: 10.1098/rstb.2010.0142
Čufar, K., Cherubini, M., Gričar, J., Prislan, P., Spina, S., and Romagnoli, M. (2011). Xylem and phloem formation in chestnut (Castanea sativa Mill.) during the 2008 growing season. Dendrochronologia. 29, 127–134. doi: 10.1016/j.dendro.2011.01.006
Čufar, K., Prislan, P., de Luis, M., and Gričar, J. (2008). Tree-ring variation, wood formation and phenology of beech (Fagus sylvatica) from a representative site in Slovenia, SE Central Europe. Trees-Struct. Funct. 22, 749–758. doi: 10.1007/s00468-008-0235-6
Davis, J. D., and Evert, R. F. (1970). Seasonal cycle of phloem development in woody vines. Bot. Gaz. 131, 128–138. doi: 10.1086/336523
Derr, W. F., and Evert, R. F. (1967). The cambium and seasonal development of the phloem in Robinia pseudoacacia. Am. J. Bot. 54, 147–153. doi: 10.1002/j.1537-2197.1967.tb06903.x
Deslauriers, A., Giovannelli, A., Rossi, S., Castro, G., Fragnelli, G., and Traversi, L. (2009). Intra-annual cambial activity and carbon availability in stem of poplar. Tree Physiol. 29, 1223–1235. doi: 10.1093/treephys/tpp061
Esau, K., and Thorsch, J. (1985). Sieve plate pores and plasmodesmata, the communication channels of the symplast: ultrastructural aspects and developmental relations. Am. J. Bot. 72, 1641–1653. doi: 10.1002/j.1537-2197.1985.tb08429.x
Evert, R. F. (1990). “Dicotyledons,” in Sieve Elements, eds H. D. Behnke and R. D. Sjolund (Berlin; Heidelberg: Springer-Verlag), 305. doi: 10.1007/978-3-642-74445-7_6
Evert, R. F. (2006). Esau's Plant Anatomy : Meristems, Cells, and Tissues of the Plant Body: Their Structure, Function, and Development, 3rd Edn. Hoboken, NY: John Wiley and Sons, Inc. doi: 10.1002/0470047380
Gärtner, H., Sandro, L., and Hans, S. F. (2014). New perspectives for wood anatomical analysis in dendrosciences: the GSL1-microtome. Dendrochronologia. 32, 47–51. doi: 10.1016/j.dendro.2013.07.002
Gärtner, H., and Schweingruber, F. (2013). Microscopic Preparation Techniques for Plant Stem Analysis. Remagen: Verlag Dr. Kessel.
Gričar, J., Prislan, P., Gryc, V., Vavrčík, H., de Luis, M., and Čufar, K. (2014). Plastic and locally adapted phenology in cambial seasonality and production of xylem and phloem cells in Picea abies from temperate environments. Tree Physiol. 34, 869–881. doi: 10.1093/treephys/tpu026
Hacke, U. (2015). Functional and Ecological Xylem Anatomy. Cham: Springer International Publishing. doi: 10.1007/978-3-319-15783-2
Hacke, U., and Sauter, J. J. (1996). Xylem dysfunction during winter and recovery of hydraulic conductivity in diffuse-porous and ring-porous trees. Oecologia. 105, 435–439. doi: 10.1007/BF00330005
Hacke, U. G., Spicer, R., Schreiber, S. G., and Plavcová, L. (2017). An ecophysiological and developmental perspective on variation in vessel diameter. Plant Cell Environ. 40, 831–845. doi: 10.1111/pce.12777
Hawkins, B. J., and Stoehr, M. (2009). Growth, phenology, and cold hardiness of 32 Douglas-fir full-sib families. Can. J. For. Res. Rev. Can. Recher. For. 39, 1821–1834. doi: 10.1139/X09-092
Heinrichs, D. K., Tardif, J. C., and Bergeron, Y. (2007). Xylem production in six tree species growing on an island in the boreal forest region of western Quebec, Canada. Can. J. Bot. Rev. Can. Bot. 85, 518–525. doi: 10.1139/B07-041
Howe, G. T., Aitken, S. N., Neale, D. B., Jermstad, K. D., Wheeler, N. C., and Chen, T. H. H. (2003). From genotype to phenotype: unraveling the complexities of cold adaptation in forest trees. Can. J. Bot. Rev. Can. Bot. 81, 1247–1266. doi: 10.1139/b03-141
Isaac-Renton, M., Montwé, D., Hamann, A., Spiecker, H., Cherubini, P., and Treydte, K. (2018). Northern forest tree populations are physiologically maladapted to drought. Nat. Commun. 9:9. doi: 10.1038/s41467-018-07701-0
Johnson, D., Eckart, P., Alsamadisi, N., Noble, H., and Martin, C. (2018). Polar auxin transport is implicated in vessel differentiation and spatial patterning during secondary growth in Populus (vol 105, pg 186, 2018). Am. J. Bot. 105, 1609–1609. doi: 10.1002/ajb2.1035
Kalmbach, L., and Helariutta, Y. (2019). Sieve plate pores in the phloem and the unknowns of their formation. Plants-Basel. 8:25. doi: 10.3390/plants8020025
Keller, S. R., Soolanayakanahally, R. Y., Guy, R. D., Silim, S. N., Olson, M. S., and Tiffin, P. (2011). Climate-driven local adaptation of ecophysiology and phenology in balsam poplar, Populus balsamifera L. (salicaceae). Am. J. Bot. 98, 99–108. doi: 10.3732/ajb.1000317
Koehler, K., Center, A., and Cavender-Bares, J. (2012). Evidence for a freezing tolerance-growth rate trade-off in the live oaks (Quercus ser. Virentes) across the tropical-temperate divide. New Phytol. 193, 730–744. doi: 10.1111/j.1469-8137.2011.03992.x
Krajina, V., Klinka, K., and Worrall, J. (1982). Distribution and Ecological Characteristics of Trees and Shrubs of British Columbia. Vancouver, BC: University of British Columbia, Faculty of Forestry.
Kuznetsova, A., Brockhoff, P. B., and Christensen, H. B. R. (2017). lmertest package: tests in linear mixed effects models. J. Stat. Softw. 82, 1–26. doi: 10.18637/jss.v082.i13
Lavrič, M., Eler, K., Ferlan, M., Vodnik, D., and Gričar, J. (2017). Chronological sequence of leaf phenology, xylem and phloem formation and sap flow of Quercus pubescens from abandoned karst grasslands. Front. Plant Sci. 8:314. doi: 10.3389/fpls.2017.00314
Little, E. L. (1971). Atlas of United States Trees, Vol. 1: Conifers and Important Hard-Woods. Washington, DC: U.S. Department of Agriculture, Forest Service, 1–877.
Lucas, W. J., Groover, A., Lichtenberger, R., Furuta, K., Yadav, S. R., Helariutta, Y., et al. (2013). The plant vascular system: evolution, development and functions. J. Integr. Plant Biol. 55, 294–388. doi: 10.1111/jipb.12041
Lüttge, U., and Hertel, B. (2009). Diurnal and annual rhythms in trees. Trees. 23, 683–700. doi: 10.1007/s00468-009-0324-1
Man, R., Colombo, S., Lu, P., Li, J., and Dang, L.-Q. (2014). Trembling aspen, balsam poplar, and white birch respond differently to experimental warming in winter months. Can. J. For. Res. 44, 1469–1476. doi: 10.1139/cjfr-2014-0302
Montwé, D., Isaac-Renton, M., Hamann, A., and Spiecker, H. (2018). Cold adaptation recorded in tree rings highlights risks associated with climate change and assisted migration. Nat. Commun. 9:7. doi: 10.1038/s41467-018-04039-5
Morin, X., Lechowicz, M. J., Augspurger, C., O' Keefe, J., Viner, D., and Chuine, I. (2009). Leaf phenology in 22 North American tree species during the 21st century. Glob. Chang. Biol. 15, 961–975. doi: 10.1111/j.1365-2486.2008.01735.x
Mullendore, D. L., Windt, C. W., Van As, H., and Knoblauch, M. (2010). Sieve tube geometry in relation to phloem flow. Plant Cell. 22, 579–593. doi: 10.1105/tpc.109.070094
Peterson, E. B., and Peterson, N. M. (1992). Ecology, management, and use of aspen and balsam poplar in the prairie provinces. Special Rep. 1, 1–252.
Plavcová, L., and Jansen, S. (2015). “The role of xylem parenchyma in the storage and utilization of non-structural carbohydrates,” in Functional and Ecological Xylem Anatomy, ed U. Hacke (Cham: Springer), 209–234. doi: 10.1007/978-3-319-15783-2_8
Prislan, P., Gričar, J., de Luis, M., Smith, K. T., and Čufar, K. (2013). Phenological variation in xylem and phloem formation in Fagus sylvatica from two contrasting sites. Agricult. For. Meteorol. 180, 142–151. doi: 10.1016/j.agrformet.2013.06.001
Prislan, P., Mrak, P., Žnidaršič, N., Štrus, J., Humar, M., Thaler, N., et al. (2018). Intra-annual dynamics of phloem formation and ultrastructural changes in sieve tubes in Fagus sylvatica. Tree Physiol. 39, 262–274. doi: 10.1093/treephys/tpy102
Rathgeber, C. B. K., Santenoise, P., and Cuny, H. E. (2018). CAVIAR: an R package for checking, displaying and processing wood-formation-monitoring data. Tree Physiol. 38, 1246–1260. doi: 10.1093/treephys/tpy054
Rossi, S., Anfodillo, T., Čufar, K., Cuny, H. E., Deslauriers, A., Fonti, P., et al. (2013). A meta-analysis of cambium phenology and growth: linear and non-linear patterns in conifers of the northern hemisphere. Ann. Bot. 112, 1911–1920. doi: 10.1093/aob/mct243
Rossi, S., Deslauriers, A., and Morin, H. (2003). Application of the Gompertz equation for the study of xylem cell development. Dendrochronologia. 21, 33–39. doi: 10.1078/1125-7865-00034
Rossi, S., Morin, H., Deslauriers, A., and Plourde, P. Y. (2011). Predicting xylem phenology in black spruce under climate warming. Glob. Chang. Biol. 17, 614–625. doi: 10.1111/j.1365-2486.2010.02191.x
Sakai, A., and Weiser, C. J. (1973). Freezing resistance of trees in North-America with reference to tree regions. Ecology. 54, 118–126. doi: 10.2307/1934380
Sanz-Perez, V., Castro-Diez, P., and Valladares, F. (2009). Differential and interactive effects of temperature and photoperiod on budburst and carbon reserves in two co-occurring Mediterranean oaks. Plant Biol. 11, 142–151. doi: 10.1111/j.1438-8677.2008.00119.x
Sauter, J. (2000). “Photosynthate allocation to the vascular cambium: facts and problems,” in Cell and Molecular Biology of Wood Formation, eds R. A. Savidge, J. R. Barnett, and R. Napier (Oxford: BIOS Scientific Publishers), 71–83.
Sauter, J. J., and van Cleve, B. (1994). Storage, mobilization and interrelations of starch, sugars, protein and fat in the ray storage tissue of poplar trees. Struct. Funct. 8, 297–304. doi: 10.1007/BF00202674
Savidge, R. A., Barnett, J. R., and Napier, R. (2000). Cell and Molecular Biology of Wood Formation. London: BIOS, 536.
Schreiber, S. G., Ding, C., Hamann, A., Hacke, U. G., Thomas, B. R., and Brouard, J. S. (2013a). Frost hardiness vs. growth performance in trembling aspen: an experimental test of assisted migration. J. Appl. Ecol. 50, 939–949. doi: 10.1111/1365-2664.12102
Schreiber, S. G., Hamann, A., Hacke, U. G., and Thomas, B. R. (2013b). Sixteen years of winter stress: an assessment of cold hardiness, growth performance and survival of hybrid poplar clones at a boreal planting site. Plant Cell Environ. 36, 419–428. doi: 10.1111/j.1365-3040.2012.02583.x
Sebastian-Azcona, J., Hamann, A., Hacke, U. G., and Rweyongeza, D. (2019). Survival, growth and cold hardiness tradeoffs in white spruce populations: implications for assisted migration. For. Ecol. Manage. 433, 544–552. doi: 10.1016/j.foreco.2018.10.046
Shuman, J. K., Shugart, H. H., and O'Halloran, T. L. (2011). Sensitivity of Siberian larch forests to climate change. Glob. Chang. Biol. 17, 2370–2384. doi: 10.1111/j.1365-2486.2011.02417.x
Sperry, J. S., Donnelly, J. R., and Tyree, M. T. (1988). Seasonal occurrence of xylem embolism in sugar maple (Acer saccharum). Am. J. Bot. 75, 1212–1218. doi: 10.1002/j.1537-2197.1988.tb08834.x
Sperry, J. S., Nichols, K. L., Sullivan, J. E. M., and Eastlack, S. E. (1994). Xylem embolism in ring-porous, diffuse-porous, and coniferous trees of northern Utah and interior Alaska. Ecology. 75, 1736–1752. doi: 10.2307/1939633
Stanfield, R. C., Schulte, P. J., Randolph, K. E., and Hacke, U. G. (2018). Computational models evaluating the impact of sieve plates and radial water exchange on phloem pressure gradients. Plant Cell Environ. 42, 1–14. doi: 10.1111/pce.13414
Swidrak, I., Gruber, A., and Oberhuber, W. (2014). Xylem and phloem phenology in co-occurring conifers exposed to drought. Trees. 28, 1161–1171. doi: 10.1007/s00468-014-1026-x
Tucker, C. M., and Evert, R. F. (1969). Seasonal development of the secondary phloem in Acer negundo. Am. J. Bot. 56, 275–284. doi: 10.1002/j.1537-2197.1969.tb07534.x
Way, D. A. (2011). Tree phenology responses to warming: spring forward, fall back? Tree Physiol. 31, 469–471. doi: 10.1093/treephys/tpr044
Wesolowski, T., and Rowinski, P. (2006). Timing of bud burst and tree-leaf development in a multispecies temperate forest. For. Ecol. Manage. 237, 387–393. doi: 10.1016/j.foreco.2006.09.061
Xie, B., and Hong, Z. (2011). Unplugging the callose plug from sieve pores. Plant Signal. Behav. 6, 491–493. doi: 10.4161/psb.6.4.14653
Zalasky, H. (1976). Frost damage in poplar on prairies. For Chronicle. 52, 61–64. doi: 10.5558/tfc52061-2
Zamski, E., and Zimmermann, M. (1979). Sieve tube longevity in white ash (Fraxinus americana) studied with a new histochemical test for the identification of sugar. Can J Bot. 57, 650–656. doi: 10.1139/b79-083
Keywords: xylem, phloem, callose, phenology, xylogenesis, populus
Citation: Montwé D, Hacke U, Schreiber SG and Stanfield RC (2019) Seasonal Vascular Tissue Formation in Four Boreal Tree Species With a Focus on Callose Deposition in the Phloem. Front. For. Glob. Change 2:58. doi: 10.3389/ffgc.2019.00058
Received: 16 July 2019; Accepted: 09 September 2019;
Published: 24 September 2019.
Edited by:
Zuoqiang Yuan, Institute of Applied Ecology (CAS), ChinaReviewed by:
Peter Prislan, Slovenian Forestry Institute, SloveniaCopyright © 2019 Montwé, Hacke, Schreiber and Stanfield. This is an open-access article distributed under the terms of the Creative Commons Attribution License (CC BY). The use, distribution or reproduction in other forums is permitted, provided the original author(s) and the copyright owner(s) are credited and that the original publication in this journal is cited, in accordance with accepted academic practice. No use, distribution or reproduction is permitted which does not comply with these terms.
*Correspondence: David Montwé, ZGF2aWQubW9udHdlQHVhbGJlcnRhLmNh
Disclaimer: All claims expressed in this article are solely those of the authors and do not necessarily represent those of their affiliated organizations, or those of the publisher, the editors and the reviewers. Any product that may be evaluated in this article or claim that may be made by its manufacturer is not guaranteed or endorsed by the publisher.
Research integrity at Frontiers
Learn more about the work of our research integrity team to safeguard the quality of each article we publish.