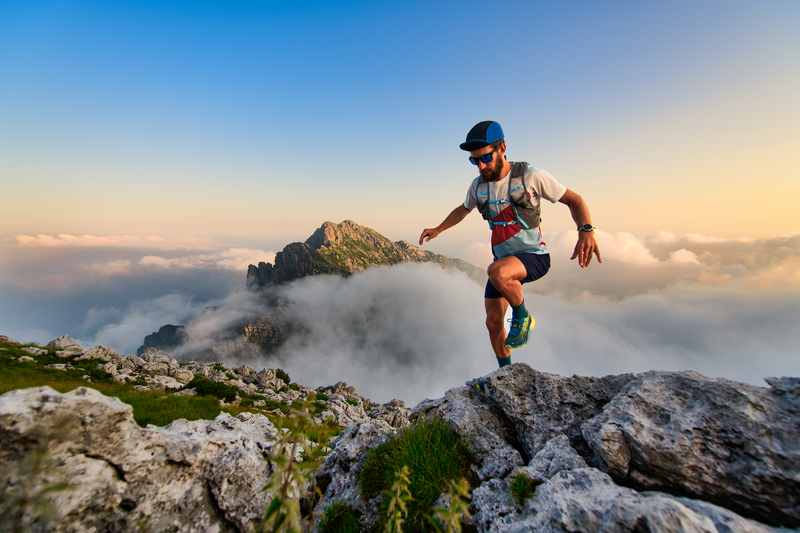
95% of researchers rate our articles as excellent or good
Learn more about the work of our research integrity team to safeguard the quality of each article we publish.
Find out more
ORIGINAL RESEARCH article
Front. For. Glob. Change , 18 September 2019
Sec. Forests and the Atmosphere
Volume 2 - 2019 | https://doi.org/10.3389/ffgc.2019.00055
This article is part of the Research Topic Climate Effects on Tree Volatile Phytochemistry: Patterns and Consequences View all 5 articles
Our knowledge on the biosynthesis of several biogenic volatile organic compounds (BVOCs) is still limited. In this regard, natural abundant stable carbon isotope ratios (δ13C) of BVOCs may provide a powerful tool to evaluate different metabolic pathways. In the present study, BVOC emissions, and their carbon isotope composition from two Mediterranean species, Quercus suber L. and Cistus ladanifer L. were investigated under field conditions in June and July 2018. Soil water content decreased between these months, which was reflected by an increase of the photosynthetic discrimination from −27.7 ± 0.2 to −26.2 ± 0.2‰ in Q. suber and from −27.0 ± 0.3 to −26.1 ± 0.3‰ in C. ladanifer. This change made an impact on the signatures of various BVOCs, which varied along a very broad range of −28.0 to −42.6‰ in June and −23.7 to −32.9‰ in July. Hence, the increasing photosynthetic discrimination had a cascading effect on the natural carbon isotope composition of the emitted BVOCs over time. Consistent differences in compound classes occurred among species and seasons: acyclic monoterpenoids were the most 13C enriched compound class (−23.7 to −31.3‰), followed by slightly more depleted cyclic monoterpenes (−27.6 to −32.9‰) and sesquiterpenes (−26.4 to −32.1‰). The detected oxygenated cyclic monoterpenoids (−31.5 to −37.0‰) and benzenoid aromatic compounds (−30.6 to −42.6‰) were strongly 13C depleted. Hierarchical clustering based on δ13C values confirmed the grouping of BVOCs with similar chemical structures to the same cluster. Hence, we suggest that isotopic fractionation occurs during the cyclization and oxygenation processes of monoterpenoids, as well as during the synthesis of volatile benzenoid aromatic compounds. The differences in δ13C values between BVOCs were consistent, although we collected BVOCs under highly varying light conditions, air temperatures, emission rates and from two different species. Here, we demonstrate that the natural carbon isotope composition may provide a robust framework to elucidate biosynthetic pathways of various BVOCs under field conditions.
Biogenic volatile organic compounds (BVOCs) play a large role in the secondary metabolism of plants, yet our knowledge about their precise biosynthesis and functions is still scarce (Dudareva et al., 2006, 2013). In general, four big groups of BVOCs have been identified in the past: (1) terpenoids, (2) benzenoid and phenylpropanoid compounds, (3) fatty acid derivatives and (4) amino acid derivatives (Dudareva et al., 2013). Each of these groups has distinct biosynthetic pathways, intermediate products and precursors, leading to various volatile products. However, the elucidation of these pathways and the biosynthetic history of single BVOCs is not always straightforward. Recently, Tan et al. (2018) suggested the investigation of the stable carbon isotope ratio of 13C/12C (δ13C) at natural abundance as a powerful tool to reveal individual biosynthetic pathways for BVOCs. It has been suggested that kinetic and thermodynamic isotope effects in metabolic processes naturally lead to an altered carbon isotope composition in the resulting products (Schmidt and Kexel, 1998; Schmidt et al., 2015). As plants are usually growing in an open system, where the substrate CO2 is infinite and the resulting products are constantly eliminated by emission, isotopic fractionation is likely to occur (Schmidt et al., 2015). This approach has been successfully applied in the past, elucidating differences in δ13C values of various primary and secondary plant metabolites, such as sucrose, starch, lipids, lignin or amino acids (Gleixner et al., 1993, 1998; Schmidt and Kexel, 1998; Lynch et al., 2016). An example is given by the pyruvate dehydrogenase involved in the cytosolic mevalonic acid (MVA) pathway, which has a natural preference for 12C, producing 13C-depleted acetyl CoA (Melzer and Schmidt, 1987) as a precursor for the resulting sesquiterpenoids (Jux et al., 2001; Tholl, 2015). Similarly, fatty acid derivatives produced in the lipoxygenase pathway are even more depleted in 13C, as they are completely synthesized from acetyl CoA (Jux et al., 2001; Dudareva et al., 2013). Moreover, the naturally 13C-enriched C1-position of pyruvate is decarboxylated leaving a 13C-depleted acetyl CoA moiety behind for anabolic processes (Priault et al., 2009; Werner and Gessler, 2011). However, such depletion in one product is usually part of a metabolic branching event, implying the production of at least one enriched counterpart (O'Leary, 1981; Schmidt and Kexel, 1998; Schmidt, 2003; Schmidt et al., 2015). Metabolic branching events can be involved in a series of transformations, including C-C bond formations and breakage (Tan et al., 2018). BVOCs are also transformed by oxidation, dehydrogenation, acylation or hydroxylation (Dudareva et al., 2004), which most likely involve more metabolic branching points and therefore, carbon isotope fractionation steps. These modifications are then reflected in the δ13C values of the respective products (Schmidt et al., 2015) as demonstrated for several sesquiterpenes (Tan et al., 2018) with varying amounts of formation steps under natural abundance.
However, kinetic and thermodynamic isotope effects can be altered or overlapped by external effects, such as changing environmental conditions (Schmidt et al., 2015), e.g., decreasing water availability. Many metabolic processes are fuelled by recently fixed carbon in photosynthesis, and thus, the carbon isotope composition of all de novo synthesized BVOCs should denote the effect of changing environmental conditions on the photosynthetic discrimination by RuBisCO of the fixed carbon, in C3 plants (e.g., Ehleringer and Cerling, 2002). RuBisCO has a natural preference for 12C (e.g., O'Leary, 1988) and photosynthetic 13C-discrimination depends, as a first approximation, on the demand of CO2 during assimilation and the supply of CO2 via stomata. When environmental stresses limit CO2 supply via stomatal closure, carbon isotope discrimination decreases (Farquhar et al., 1982). Hence, the primary photosynthetic products become 13C-enriched and, thus, all subsequently synthesized metabolites should also reflect this trend (Schmidt et al., 2015) if no alternative carbon sources or stored BVOCs are utilized. In case of emissions of storage BVOCs, the δ13C signature of BVOCs should be decoupled from the δ13C signature of the recently fixed photosynthetic products, as biosynthesis and emission are processes separated in time (Kesselmeier and Staudt, 1999). Hence, we hypothesize that (1) changing environmental conditions such as decreasing water resources have a cascading effect on the natural carbon isotope composition of recently fixed carbon and de novo emitted BVOCs. Furthermore, (2) we expect to reveal different metabolic pathways for various BVOC compound classes according to their δ13C signature.
We tested these hypotheses in two different species, Quercus suber L. and Cistus ladanifer L., known to emit a blend of different BVOCs (Haberstroh et al., 2018). Q. suber is an evergreen Mediterranean oak species well adapted to the naturally occurring summer drought by accessing deep-water resources, hydraulic lift and stomatal control to avoid water losses (David et al., 2007; Grant et al., 2010). C. ladanifer is a semi-deciduous shrub expressing high growth rates and water-use-efficiency (Correia et al., 1987; Werner et al., 1999; Correia and Ascensao, 2016), competing for water resources with Q. suber, especially during times of severe drought (Caldeira et al., 2015). Regarding BVOCs, Q. suber is a non-storing species (Loreto et al., 1996) and emits mainly monoterpenoids (Staudt et al., 2004; Pio et al., 2005; Haberstroh et al., 2018). C. ladanifer, on the other hand, stores substantial amounts of BVOCs in secretory trichomes (Gülz et al., 1996). Emissions include monoterpenoids, sesquiterpenoids and even diterpenoids in measurable amounts (Pio et al., 1993; Haberstroh et al., 2018; Yáñez-Serrano et al., 2018).
This study aims at assessing the emissions and carbon isotope signatures of various BVOCs in order to: (1) reveal different metabolic pathways of various BVOCs under changing environmental conditions, (2) demonstrate cascading effects of 13C discrimination by RuBisCO in C3 plants on BVOCs, and (3) compare two Mediterranean species with different drought adaptation strategies and BVOC emission patterns.
In summer 2018, we conducted two measurement campaigns in a Q. suber ecosystem in Eastern Portugal (Vila Viçosa, Alentejo, 38° 47′ N, 7° 22′ W, 430 m a.s.l.). The mean annual air temperature is 16.5 °C with a mean annual precipitation of 585 mm (1981–2010, www.ipma.pt). The ecosystem is dominated by Q. suber trees, which have been progressively invaded by C. ladanifer, an invasive shrub species naturally occurring in Portugal. The experimental site consists of three blocks, which all include Q. suber and C. ladanifer individuals. All blocks were fenced in winter 2017/2018 to avoid disturbance by fauna. We retrieved meteorological data such as precipitation, air temperature, relative humidity and photosynthetically active photon flux density (PPFD) from small meteorological stations installed on each block. Vapor pressure deficit (VPD) was calculated from air temperature and relative humidity values. Volumetric soil water content was measured continuously with 5TM probes (METER Group, Inc. USA, Pullmann, WA, United States) in 0.20 m depth. The soil on site is poorly developed and classified as a haplic Leptosol (FAO, 2006), derived from schist with a high proportion of soil skeleton (see Caldeira et al., 2015; Haberstroh et al., 2018 for further details).
BVOC sampling took place from 19 to 26 June and 16–18 July 2018. On each of the three blocks, we chose four trees (n = 12) and two shrub individuals (n = 6) for measurements. The sampling started between 8 and 9 am and proceeded to 5 or 6 pm to cover a daily course of emissions. On each day, BVOCs were sampled with a dynamic enclosure system (Haberstroh et al., 2018) along the diurnal course with five measurements (n = 5) for 1–2 h on the same leaf or branch. This study set-up allowed us to collect 60 BVOC samples for Q. suber (n = 60) and 30 samples for C. ladanifer (n = 30) during each of the sampling campaigns. For Q. suber, custom-made enclosures with a volume of ~30 ml were used to fit a single, south exposed, current-year leaf at ~3 m height. In rare cases, two leaves were enclosed, which did not shade each other. Enclosures (~460 ml) for C. ladanifer were made to fit a south exposed branch with 8 to 19 current year leaves. Leaves and branches were enclosed at least 30 min before the first sampling period and remained inside the enclosure until the last measurement in the late afternoon. Reinstalling the enclosures for each measurement point raised concerns about the mechanical disturbance of the leaves and the production of artifacts from induced emissions. Air sampling pumps (210-1003MTX, SKC, Germany) were connected to the enclosures, which were permanently flushed with an ambient airflow rate of 200 ml min−1. Although the enclosures were flushed permanently, an enclosure effect on the measurement temperature cannot be excluded. All materials in contact with the gas stream were made of chemically inert PET foil (Bratschlauch, Toppits®, Minden, Germany) or perfluoroalkoxy tubing (Swagelok, Karlsruhe, Germany). During sampling, an air sampling glass tube (Gerstel, Mülheim a.d.R., Germany) filled with Tenax TA (Sigma Aldrich, Munich, Germany) was placed between enclosure and air sampling pump to accumulate the emitted BVOCs. Enclosed leaf samples were occasionally shaded with neutral density meshes in case of condensation effects on the enclosure walls. Natural shading effects occurred throughout the day depending on neighboring trees and solar altitude. PPFD was measured with a Ceptometer (ACCUPAR LP-80, METER Group, Inc., Washington, USA) parallel to the leaf or branch once during the sampling period. Ambient BVOC concentrations were determined 2 m above ground and sampled simultaneously with plant BVOCs. All adsorbent tubes were shaded during sampling with aluminum foil. After sampling, adsorbent tubes were stored at +4°C in Labco Exetainers (Labco Limited, Lampeter Ceredigion, United Kingdom) until further analysis. We harvested leaves of Q. suber and C. ladanifer after the last sampling period for determination of dry weight after oven drying (65°C, 48 h). Further description of the sampling procedure and sampling material is given in Haberstroh et al. (2018).
During each sampling campaign, net carbon assimilation, stomatal conductance and transpiration were measured with a portable LI-6400XT photosynthesis system (LICOR Inc., Nebraska, USA) equipped with a CO2 mixer and light source. Gas exchange measurements were always conducted on leaves close to the leaf enclosures at a PPFD of 300 μmol m−2 s−1 to match the conditions under natural or artificial shading and to allow for a general comparison of net carbon assimilation values. Due to the inaccessibility of Q. suber leaves with the heavy and sensitive gas exchange equipment in the tall canopies, gas exchange was measured on freshly detached branches (Haberstroh et al., 2018). For C. ladanifer, attached leaves were chosen, as shrubs were easily accessible. Photosynthetic discrimination (δ13Cplant) of both species was calculated in‰ according to Farquhar et al. (1982) for C3-plants as in equation 1 for values obtained from measurements at a PPFD of 300 μmol m−2 s−1:
where δ13Catm is the carbon signature of the ambient air, a is the fractionation factor due to diffusion of CO2 in air, b is the discrimination factor of RuBisCO, ci the internal CO2 partial pressure in the intercellular space and ca the atmospheric CO2 partial pressure. δ13Catm was taken as −8‰, a as 4.4‰ (Farquhar et al., 1982) and b as 27‰ (Cernusak et al., 2013). ci and ca were obtained from gas exchange measurements. Values for δ13Catm were taken from the literature, as no field measurements were available. We are aware, that δ13Catm is not always stable; however, the difference should have a minor effect in an open evergreen Mediterranean ecosystem. In addition, both measurement campaigns were conducted in close temporal proximity. The calculated daily mean values of photosynthetic discrimination were considered to be equal to the δ13C signature of the recently fixed carbon in photosynthesis. In addition, pre-dawn (ΨPD) and midday leaf water potential (ΨMD) were determined with a Scholander-type pressure chamber (PMS 1,000, PMS Instruments, Corvalis, Orgeon, OR, USA). The meteorological conditions during all sampling days were stable with clear skies, seldom intercepted by minor clouds. No precipitation occurred during the measurements.
Emitted BVOCs were analyzed on a gas chromatograph (GC, 6890A, Agilent Technologies Böblingen, Germany) with a mass-selective detector (MSD, 5975C, Agilent Technologies Böblingen, Germany) containing a thermodesorption/cold injection system (TDU-CIS, Gerstel, Germany). Briefly, adsorbent tubes were heated to 220°C to thermodesorb the volatiles, which were then cryotrapped at −50°C in the CIS. Heating to 240°C released the BVOCs onto the separation column (DB-5ms UI, 30 m × 0.25 mm ID, 0.25 μm film thickness, Agilent Technologies Böblingen, Germany). Helium was used as carrier gas at a flow rate of 1 ml min−1. The MSD operated at 70 eV with an ion source temperature of 230°C and a quadrupole temperature of 150°C. Further GC oven and MSD settings are given by Kleiber et al. (2017) and Haberstroh et al. (2018). Obtained mass spectra were processed with the MassHunter Software (Agilent Technologies, Böblingen, Germany). For compound identification mass spectra were compared to the NIST library together with available authentic standards, which were further used for quantification. Emission rates (Em) in μg g−1 DW h−1 of chosen BVOCs were calculated as in formula 2:
where co and cc (μg) are the amounts of BVOCs trapped on adsorbent tubes from plant enclosures and ambient air, respectively, dw (g) the dry weight of plant leaves in enclosures and t (h) the sampling time. We selected the acyclic monoterpenoids myrcene (MC), linalool (LN), and trans-β-ocimene (TBO), the cyclic monoterpenes α-pinene (AP), camphene (CP), limonene (LM), sabinene (SB), the monoterpene related alkyl benzene p-cymene (PC), the monoterpenoids verbenone (VB) and bornyl acetate (BAC), the sesquiterpenes δ-cadinene (DC) and ledene (LD) and two benzenoid aromatic compounds benzaldehyde (BZA) and durene (1,2,4,5-tetramethyl-benzene) (DU) for further investigation. All compounds were identified with a match factor of at least 90%. These compounds were chosen, as they were mostly present in considerable amounts in both species and represent chemically different BVOC classes. In addition, a certain amount of BVOCs is necessary to obtain a reliable and reproducible carbon isotopic ratio measurement (section carbon isotope ratio measurements). This was not the case for many BVOCs emitted at low rates.
δ13C values of plant volatiles were determined by gas chromatography—combustion interfaced—isotope ratio mass spectrometry (GC-C-IRMS). This system allows a high precision and accuracy of carbon isotopic ratios at natural abundance (Meier-Augenstein, 1999). For compound-specific δ13C analysis, the separation column ended at a split channeling 10% of the eluting compounds to the MSD for compound identification and quantification (see above), and 90% to a combustion furnace (GC5 interface, Elementar, Hanau, Germany) and ultimately into the IRMS (Isoprime precisION, Elementar, Hanau, Germany) where the carbon isotopic ratios of individual BVOCs were analyzed. The eluting compounds were combusted continuously at 850°C by CuO producing CO2 and H2O. After passing a Nafion H2O trap, CO2 entered the continuous flow IRMS to determine δ13C signatures for all compounds separated by GC. As reference compounds, we used octane (δ13C: −31.75 ± 0.01‰) and octadecane (δ13C: −32.70 ± 0.01‰) (available from Schimmelmann lab, Indiana University, https://arndt.schimmelmann.us/compounds.html). Data were analyzed with the Software IonIOS (Elementar Analysesysteme GmbH, Langenselbold, Germany). Due to small emission rates, it was not possible to obtain reliable δ13C values in some cases. Peaks with a height <0.5 nA and > 50 nA were excluded from the analysis.
We applied a two-way repeated measure analysis of variance (RMANOVA) to identify significant differences in assimilation, stomatal conductance, photosynthetic discrimination, Ψ and total BVOC emission rates between species and sampling dates. In case of statistical significance, we applied the post hoc Tukey's test. Beforehand the data was tested for normal distribution and equality of variances. If assumptions for an ANOVA were not met, data was log or square root transformed. Due to normality violations, differences in the emission of single BVOCs between months were tested for each species separately with the Wilcoxon signed-rank test to account for the repeated measures design. The δ13C values of single BVOCs were tested for significant differences between months with a one-way RMANOVA for each species separately. In rare cases, the assumptions for a RMANOVA were violated and results crosschecked and confirmed with the Wilcoxon signed-rank test. The δ13C values of BVOC were tested with Grubbs' test for outliers in a data sample (package “outliers” in R). In 37 out of 54 datasets, no outlier could be identified. The number of omitted data points (1–5) in the remaining datasets is given in Table 2. Afterwards, missing data was imputed with the package “mice” (multivariate imputation by chained equations) in R. The complete datasets for June and July were used to perform Ward hierarchical clustering (method: “Euclidean”) with the package “stats” in the statistical software R. All statistical analysis was conducted in R (version 3.4.3).
The two sampling dates were characterized by very similar meteorological conditions (Table 1). Air temperatures reached maximum values of 33.5 and 33.0°C in June and July, respectively. VPD and air temperatures were moderate in the morning and highest in the afternoon between 2 and 5 p.m. The light conditions were highly variable during the measurements ranging from 30 to 1,860 μmol m−2 s−1 in June and from 40 to 1,940 μmol m−2 s−1 in July, as sampled leaves were partially shaded in their natural position at some point during the day.
Table 1. Average air temperature (°C), vapor pressure deficit (kPa) during the sampling campaigns with maximum and minimum values in brackets and range of photosynthetically active photon flux density (μmol m−2 s−1) during the BVOC sampling.
Precipitation was twice as high 30 days prior to the first campaign compared to the second campaign in July (Table 1). Hence, the soil water content in 0.20 m depth decreased on average by 0.03 m3 m−3 from June to July. This trend was reflected onto the net carbon assimilation rates of both species, which significantly (p < 0.001) declined (Figure 1A). While assimilation of C. ladanifer was clearly higher compared to Q. suber in June (p < 0.01), differences vanished in July. The same pattern was observed for stomatal conductance, though values were not significantly different between species neither in June nor in July (Figure 1B). Likewise, photosynthetic discrimination increased significantly (p < 0.05) from −27.7 ± 1.7 to −26.2 ± 1.9‰ in Q. suber and non-significantly from −27.0 ± 1.8 to −26.1 ± 1.5‰ in C. ladanifer (Figure 1C). A similar trend is indicated by the leaf water potential measurements (Figure 1D), where ΨPD declined significantly in both species (p < 0.001), although more profoundly for C. ladanifer from −1.00 ± 0.05 MPa to −1.45 ± 0.03 MPa. ΨMD was significantly lower for C. ladanifer (p < 0.01) compared to Q. suber in both months. Between months, significant changes of ΨMD did not occur for either species.
Figure 1. Net assimilation (AN) (A), stomatal conductance (gs) with standard error (B), and photosynthetic discrimination (C) for Q. suber (n = 60), and C. ladanifer (n = 30) with 5 and 95% percentile in June and July. Pre-dawn (ΨPD) and midday (ΨMD) leaf water potential for Q. suber (n = 12) and C. ladanifer (n = 6) with standard error in June and July (D). Statistical differences (RMANOVA) between species and months are indicated by letters over bars at a significance level of p < 0.05.
Total BVOC emissions of Q. suber and C. ladanifer were highly variable and clearly differed (p < 0.05) between the two species (Figure 2). Total emissions of Q. suber slightly increased (p > 0.05) on average from 5.6 ± 1.0 μg g−1 h−1 in June to 7.0 ± 1.3 μg g−1 h−1 in July. Emissions were dominated by monoterpenoids, which made up over 99% of emissions in both months (Figures 2A,C). Other BVOCs such as bornyl acetate, ledene, benzaldehyde or durene were only detected in few plant samples and in low quantities (Figure 2E). Emissions of plant volatiles only increased significantly between June and July for sabinene (p < 0.001) and durene (p < 0.05) and decreased significantly for ledene (p < 0.001), benzaldehyde and bornyl acetate (both p < 0.05). The sesquiterpene δ-cadinene could not be detected in the emissions of Q. suber, and hence was only emitted by C. ladanifer. In June, total emissions of C. ladanifer were low with 1.2 ± 0.1 μg g−1 h−1 and almost doubled to 2.3 ± 0.3 μg g−1 h−1 in July (p < 0.01). This increase was mostly attributed to a significant increase in emissions of α-pinene, sabinene, camphene, p-cymene, limonene, myrcene, and bornyl acetate (p < 0.05). The dominant BVOCs were p-cymene and limonene with a share of at least 50% of emissions (Figures 2B,D). In contrast to Q. suber, emissions of myrcene, trans-β-ocimene and linalool were low and not always detected in C. ladanifer. On the other hand, the monoterpenoids, verbenone, and bornyl acetate had a considerable share of total emissions with 13% in June and 19% in July (Figure 2F). Sesquiterpene emissions were low, but constant from June to July. The two benzenoid aromatic compounds benzaldehyde and durene were emitted at similar rates by both species. It must be noted that we focused on BVOCs mostly present in both species for comparative reasons. However, the total emissions of C. ladanifer were more diverse in terms of total emitted compounds.
Figure 2. Emissions of selected BVOCs with standard error for acyclic and aromatic monoterpenoids (A,B), cyclic monoterpenes (C,D), and oxygenated monoterpenoids, sesquiterpenes and benzenoids (E,F) for Q. suber (green, n = 59 in June, n = 60 in July, A,C,E) and C. ladanifer (blue, n = 24 in June, n = 30 in July, B,D,F) for June and July. Due to a high variability of emission rates between BVOCs, data breaks (//) were introduced for a better overview. Significant changes (Wilcoxon signed rank test) in emission rates between months are indicated by asterisk over bars at a significance level of *p < 0.05, **p < 0.01, ***p < 0.001. MC, myrcene; TBO, trans-β-ocimene; LN, linalool; PC, p-cymene; LM, limonene; AP, α-pinene; SB, sabinene; CP, camphene; VB, verbenone; BAC, bornyl acetate; LD, ledene; DC, δ-cadinene; BZA, benzaldehyde; DU, durene. DC was not detected in emissions of Q. suber.
Between sampling dates and species, the obtained δ13C values of BVOCs varied along a very broad range from −23.7 to −42.6‰ (Figure 3, Table 2). Since no clear diurnal pattern of δ13C values could be detected for BVOCs or for the potential carbon source (Figure S1), we focused on differences between sampling campaigns, species and BVOC compound classes. In both species, δ13C values of most BVOCs became more enriched from June to July (Figure 3). This pattern reflected the calculated decrease in photosynthetic discrimination. Yet, the change in δ13C values was stronger for BVOCs, compared to the change in the potential carbon source. On average, δ13C values of BVOCs emitted by Q. suber increased by 3.4 ± 0.5‰ and by C. ladanifer by 1.8 ± 0.5‰ from June to July. For all BVOCs with sufficient number of replicates emitted by Q. suber, the carbon isotope composition changed significantly from June to July (p < 0.05). For verbenone, bornyl acetate, ledene, benzaldehyde, and durene (Figure 3E) a statistical test was not applied due to the small sample size (Table 2), since not all plant individuals emitted these compounds at rates above detection limits (Figure 2E, Table 2). In C. ladanifer, only 8 out of 14 compounds became significantly (p < 0.05) more enriched from June to July, namely myrcene, α-pinene, limonene, ledene, δ-cadinene, verbenone, bornyl acetate, and durene.
Figure 3. BVOC carbon isotope composition for acyclic and aromatic monoterpenoids (A, B), cyclic monoterpenes (C, D), and oxygenated monoterpenoids, sesquiterpenes, and benzenoids (E,F) for Q. suber (green, A,C,E) and C. ladanifer (blue, B,D,F) with 5 and 95 % percentile. Please see Table 2 for sample size for each compound and species. Short dashed lines represent the δ13C values of the carbon source in June; long dashed lines represent the δ13C values of the carbon source in July. For dotted boxplots, only few δ13C values were obtained. MC, myrcene; TBO, trans-β-ocimene; LN, linalool; PC, p-cymene; LM, limonene; AP, α-pinene; SB, sabinene; CP, camphene; VB, verbenone; BAC, bornyl acetate; LD, ledene; DC, δ-cadinene; BZA, benzaldehyde; DU, durene.
Table 2. Average δ13C values for chosen BVOCs with standard error for Q. suber and C. ladanifer for June and July.
In Q. suber, the most enriched compounds were linalool, myrcene and trans-β-ocimene with values of −28.3 to −28.8‰ in June and −23.7 to −25.9‰ in July. These BVOCs were either similar or even slightly 13C-enriched compared to the potential carbon source in Q. suber (Figure 3A). In C. ladanifer, δ13C values of myrcene were comparable (June: −30.5‰; July: −25.8‰), while trans-β-ocimene and linalool were more 13C-depleted (Figure 3B, June: −28.5 to −31.3‰; July: −29.3 to −30.5‰), yet emissions were low and not detected in all samples (Figure 2B). While δ13C values differed between species for p-cymene in June (Q. suber: −32.5‰; C. ladanifer: −28.0‰), they were in the same range of −27.0 to −27.3‰ in July (Figures 3A,B). The cyclic monoterpenes limonene, α-pinene, sabinene, and camphene were depleted in 13C compared to the potential carbon source with δ13C values between −29.4 and −32.9‰ in June and −27.6 and −29.8‰ in July, independent of the species (Figures 3C,D, Table 2). Of these four cyclic monoterpenes, α-pinene was the most enriched compound (−28.4 to −29.5‰), while camphene was the most depleted (−28.9 to −32.9‰). The oxygenated monoterpenoids verbenone and bornyl acetate were clearly depleted compared to all other terpenoids with values between −31.5 and −37.0‰ (Figures 3E,F). The two sesquiterpenes ledene and δ-cadinene, however, expressed δ13C values more similar to the cyclic monoterpenes (Figures 3C,D). The aromatics benzaldehyde and durene were also 13C-depleted, especially compared to the monoterpene related alkyl benzene p-cymene (Figures 3A,B). Although emissions of Q. suber were low and not present in all samples, δ13C values for ledene, verbenone, bornyl acetate, benzaldehyde, and durene were similar to the values measured for C. ladanifer (Figures 3E,F).
Given the overall similarities of δ13C values between the two species, we decided to conduct hierarchical clustering analyses independent of the species for June and July (Figures 4A,B). In June, two main clusters could be identified, of which the first (I) included the monoterpenoids verbenone, bornyl acetate and the benzenoid compound durene (Figure 4A). The remaining compounds were allocated to the second cluster (II), which split into two sub-clusters. All acyclic monoterpenoids clustered into the second of these two sub-clusters (ii). Moreover, the clustering approach for July yielded an even more consistent picture (Figure 4B), as the variability of δ13C values of most BVOCs was reduced from June to July (Figure 3). Both oxygenated monoterpenoids and benzenoid aromatic compounds were allocated to the first cluster (I). In the second cluster (II), all cyclic monoterpenes except camphene were found in the same sub-cluster (ii) with the acyclic monoterpenoids myrcene, linalool and trans-β-ocimene. The second sub-cluster (ii) included both sesquiterpenes and the cyclic monoterpene camphene. Hence, BVOCs with a similar chemical structure were allocated to the same group, showing similar δ13C values. It must be denoted that the dendrograms were created using imputed datasets and require careful interpretation. Hence, they only give a first indication of similarities and dissimilarities of the investigated BVOCs.
Figure 4. Hierarchical clustering of selected BVOCs according to their δ13C value independent of the species for June (A) and July (B). BVOCs were sorted according to their chemical structure into acyclic monoterpenoids (square, lightblue), cyclic monoterpenes (square, blue), aromatic monoterpenes (square, darkblue), oxygenated monoterpenoids (square, blue edge), sesquiterpenes (triangle, orange), and benzenoids (diamond, black).
The investigated BVOCs in this study reflect a wide array of δ13C values. In this regard, not only differences between single BVOCs were evident, but also changes over time. These differences are probably the result of different discrimination and fractionation processes in the primary and secondary metabolism of plants, which will be discussed hereafter. We also discuss why the study of the carbon isotope composition of BVOCs at natural abundance can be a powerful tool to support the elucidation of different biosynthetic pathways in the secondary metabolism of plants.
BVOCs are either emitted de novo or from storage pools (i.e., Fineschi et al., 2013). Independently of the emission source, the very first isotopic imprint on the carbon source for the production of plant volatiles derives from isotopic discrimination during photosynthetic carbon fixation by RuBisCO in the primary metabolism of C3-plants. This process itself strongly reflects changes in environmental conditions, as both the stomatal conductance to CO2 as well as assimilation rate determine the effective 13C-discrimination by RuBisCO (Farquhar et al., 1982). Hence, under favorable environmental conditions, relatively 13C-depleted carbon molecules are produced, as seen for both species in this study in June. From June to July, environmental conditions became slightly drier, which resulted in decreased net assimilation, stomatal conductance and photosynthetic discrimination of Q. suber and C. ladanifer. This 13C-enrichment of freshly produced sugars was clearly reflected in the carbon isotope composition of BVOCs in July, pointing mainly toward de novo emission from both species. Q. suber does not possess specialized storage structures for BVOCs (Loreto et al., 1996) and can, per se, only emit de novo produced BVOCs. Several studies also indicate that BVOC storing plants, such as C. ladanifer, are also able to emit substantial amounts of BVOCs from de novo production (Lluisà et al., 2010; Yáñez-Serrano et al., 2018). Hence, the changes of the δ13C-values of BVOCs over time support our first hypothesis that changing environmental conditions evoke changes in the carbon isotope composition of recently fixed carbon and de novo emitted BVOCs. Yet, the carbon isotope composition was not only different between months, but also between the investigated BVOC compound classes. These differences point toward various metabolic steps and processes in the formation of these BVOCs. Thus, we suggest a scheme for different metabolic pathways independently of the species for BVOCs investigated in this study based on the obtained δ13C values and available literature (Figure 5). As the variability of δ13C values for BVOCs was lower in July and yielded a clearer picture in the clustering approach (Figure 4B), this scheme is based on the δ13C-values obtained from samples with sufficient replicates in July.
Figure 5. Suggested scheme for different metabolic pathways for selected terpenoids and aromatics. The compound groups are given in italic letters. Processes, supposed to be involved in the formation of the respective volatile are given next to arrows. The information about these processes are taken from various studies mentioned in the discussion. We do not imply to have knowledge of the δ13C values of the respective precursors for monoterpenoids, sesquiterpenes, and benzenoids, hence they are displayed in dashed boxes. The formation of sesquiterpenes and cyclic monoterpenes must be seen independently of each other. The values for δ13C correspond to the July measurements. FPP, farnesyl pyrophosphate; GPP, geranyl pyrophosphate; Phe, phenylalanine.
The uniform precursor for most monoterpenoids is geranyl pyrophosphate (GPP) which is formed by head-to-tail condensation of dimethylallyl pyrophosphate (DMAPP) and isopentenyl pyrophosphate (IPP) in the plastidic 2-C-methyl-Derythritol 4-phosphate MEP-pathway (Davis and Croteau, 2000; Tholl, 2015). Subsequently, the activity of different terpene synthases leads to a wide array of different products (Dudareva et al., 2013), such as the acyclic monoterpenoids myrcene, trans-β-ocimene, or linalool (Figure 5). As these BVOCs did not undergo a cyclation process, the result is a linear structure compared to mono- or bicyclic monoterpenoids (Figure 5). Hence, their synthesis is simpler compared to cyclic monoterpenes (Shimada et al., 2005). Thus, they can be regarded as monoterpenoid products directly resulting from GPP (Figure 5). There is experimental evidence, that the formation of trans-β-ocimene and myrcene is achieved by reversible deprotonation and protonation from intermediates like GPP (Gleizes et al., 1982) and not necessarily under enzymatic control (Loreto et al., 1998). This similarity in their metabolic pathway is apparently reflected onto their carbon isotope composition, which is enriched compared to other investigated monoterpenoids, and lies in the range of the δ13C signature of the respective putative carbon source. Hence, we suppose that there might be a metabolic branching point involved in the cyclization of monoterpenes, giving rise to slightly 13C-enriched acyclic and 13C-depleted cyclic products. Croteau et al. (1988) stated that the isomerization-cyclization of GPP and linalyl pyrophosphate (LPP) is stereospecific. This assumption combined with the fact that many monoterpene cyclases produce multiple cyclic and acyclic products (Croteau et al., 1988; Wagschal et al., 1994; McGarvey and Croteau, 1995) raises the question if these cyclases are inducing differences in the carbon isotope composition of acyclic and cyclic monoterpenoids. This assumption is supported by the quite similar δ13C values of the investigated cyclic monoterpenes limonene, α-pinene, sabinene and camphene, independently of their mono- or bicyclic structure. Tan et al. (2018) attributed the difference of 2 to 3‰ in the carbon isotope composition of α-humulene and β-caryophyllene, two sesquiterpenes with a very similar structure, to the formation of a new carbon-carbon bond, a process also involved in the formation of cyclic monoterpenes. As δ13C values within the group of investigated cyclic monoterpenes also varied slightly, further isotope fractionation processes in their biosynthesis cannot fully be excluded.
With p-cymene, verbenone and bornyl acetate, we investigated three additional monoterpenoids, which require further transformation processes after the cyclation process (Figure 5). The proposed metabolic pathway of biosynthesis of the aromatic monoterpene p-cymene is via aromatization of γ-terpinene (Poulose and Croteau, 1978) or α-terpinene (Albino et al., 2017). Unfortunately, we were not able to detect neither γ-terpinene nor α-terpinene. However, those terpenes are structurally closely related to limonene, as they are also members of the p-menthanes subfamily, and thus, should express similar δ13C values. Compared to limonene, p-cymene was only slightly enriched in both species and allocated to the same cluster in both months. This similarity supports the proposed metabolic pathway of p-cymene, yet a small fractionation process might be involved in the aromatization process (Figure 5).
Compared to all other monoterpenoids, verbenone and bornyl acetate were strongly depleted in 13C. Verbenone is a monoterpene ketone enzymatically derived from α-pinene (Vanek et al., 2005; Pereira Limberger et al., 2007). In this context, isotope fractionation is surprising, as no carbon bondage or breakage is involved in the formation of verbenone (Figure 5). α-pinene is simply transformed by autoxidation at the allylic position to trans-verbenol, which slowly transforms to verbenone by oxidation (Lindmark-Henriksson et al., 2003; Pereira Limberger et al., 2007). As verbenone was mostly detected in C. ladanifer, the possibility of storage emissions cannot be fully excluded. However, from some Q. suber trees, δ13C values of verbenone could be obtained, which were also depleted compared to α-pinene. Vitzthum von Eckstaedt et al. (2012) measured δ13C values depleted by 1.2‰ for 1,8-cineole in Eucalyptus diversicolor, another oxygenated monoterpenoid, compared to the cyclic monoterpene limonene. This possibly indicates that oxidation processes or therein-involved terpene synthases prefer 13C-depleted molecules. An alternative explanation may be isotopic fractionation during the volatilization of BVOCs, as oxygenated monoterpenoids, compared to non-oxygenated, have a higher water-solubility and can temporarily be stored at the aqueous phase inside the leaves (Niinemets et al., 2002). Their emission rates are related to Henry's law constant and are more sensitive to changes in stomatal conductance (Harley, 2013). For this reason, the volatilization could be the cause for the fractionation, as more depleted and hence lighter molecules might change faster from the liquid to the gaseous phase. Bouchard et al. (2008) observed this effect for petroleum hydrocarbons, where volatilized compounds were depleted by 1.4 to 4.8‰ compared to their respective source. However, we could not detect a similar effect for linalool, which is also an (acyclic) oxygenated monoterpene, in the emissions of Q. suber. In C. ladanifer, δ13C values for linalool were only detected in few samples, yet they were depleted compared to Q. suber by 3.0 to 4.6‰. This could possibly also indicate storage emissions, as linalool is commonly found in the essential oil of C. ladanifer (Teixeira et al., 2007). While isotopic fractionation during volatilization could also be an explanation for the depleted δ13C values of bornyl acetate, there are more steps involved in the respective biosynthesis. Bornyl acetate belongs to the camphane type monoterpenoids and can be formed by transformation of camphene (Croteau et al., 1990). In the acetylation process, two carbon molecules are added (Figure 5), probably from acetyl CoA, which is, depending on its origin, known to be depleted in 13C (Melzer and Schmidt, 1987). Hence, the carbon depletion might have a very different source than in the case of verbenone. It must also be noted that camphene was the most depleted cyclic monoterpene and, thus could also contribute slightly to the depletion of bornyl acetate.
The two sesquiterpenes δ-cadinene and ledene were mainly emitted by C. ladanifer, a species known to emit many sesquiterpenes (Haberstroh et al., 2018). It is generally assumed that farnesyl pyrophosphate (FPP), the precursor of sesquiterpenes, is formed in the MVA-pathway from acetyl CoA (Davis and Croteau, 2000; Dudareva et al., 2013) and should thus be depleted in 13C compared to the terpenoid precursors generated in the MEP-pathway (Jux et al., 2001). This is attributed to the reaction catalyzed by pyruvate dehydrogenase (PDH) leading to 13C-depleted acetyl CoA (Melzer and Schmidt, 1987). Although Jux et al. (2001) confirmed this assumption, we could not detect significant differences in the δ13C values of cyclic monoterpenes and sesquiterpenes. A possible explanation for this phenomenon could be given by the origin of the sesquiterpene precursor acetyl CoA, which should determine the δ13C-value of sesquiterpenes. Cytosolic acetyl CoA is assumed to have its origin in the mitochondria where it is produced by PDH and exported via a citrate shuttle (Fatland et al., 2002). However, four subcellular compartments are known to feature acetyl CoA production, not all of them involving PDH (Fatland et al., 2002). Hence, acetyl CoA, which is involved in the biosynthesis of sesquiterpenes, might be produced differently or have a mixed origin. One option is the production by pyruvate decarboxylase (PDC) in the cytosol. Pyruvate is decarboxylated by PDC to acetaldehyde, which is then oxidized to acetate. This acetate can be activated by acetyl CoA synthethase to yield acetyl CoA (Lin and Oliver, 2008). The decarboxylation process is not known to cause any fractionation (Gilbert et al., 2011). Hence, acetyl CoA produced via this metabolic pathway should not be 13C-depleted and, thus, could explain the δ13C values of the sesquiterpenes in this study. Indeed, a study of lipid biomolecules of Cryptomeria japonica likewise demonstrated that sesquiterpenes where even enriched in 13C compared to some terpenoids produced via the MEP-pathway. The δ13C values for δ-cadinene and its isomers are comparable to the values measured in our study (Chikaraishi et al., 2004). Hence, the assumption that terpenoids produced via the MVA-pathway are always more 13C-depleted than those formed in the MEP-pathway should be questioned. This is also indicated by the hierarchical clustering applied in this study, which did not yield a distinct differentiation between sesquiterpenes and monoterpenoids (Figure 4). Furthermore, it also has to be considered that Jux et al. (2001) used ocimene for their comparison, which is an acyclic monoterpene. In our study, acyclic monoterpenoids were also more 13C-enriched than sesquiterpenes (Figure 5). However, further modification processes can even lead to monoterpenoids, which are clearly more depleted than (simple) sesquiterpenes, as seen for the case of verbenone and bornyl acetate.
The common precursor for aromatic BVOCs is the aromatic amino acid phenylalanine, which is formed in the shikimate pathway (Dudareva et al., 2013). According to Schmidt et al. (2015) a metabolic branching event in the metabolism of plants leads to depleted fatty acids and enriched amino acids. This however, is a very broad statement and inconsistent with the measured δ13C values for benzaldehyde and durene in our study (Table 2). In a recent study, Lynch et al. (2016) investigated the carbon isotope composition of different amino acids in various plants, revealing that the aromatic amino acids phenylalanine and tyrosin were depleted by 1 to 4‰ in 13C compared to the average δ13C value of all investigated amino acids. Gleixner et al. (1998) even reported δ13C values of −37.2‰ in leaves and −38.1‰ in tubers of Solanum tuberosum for phenylalanine. This depletion of phenylalanine and further transformation steps involved in the synthesis of benzaldehyde and durene are most likely responsible for the overall depletion of these aromatic compounds.
Given the large amount of microbes on leaf surfaces (Lindow and Brandl, 2003) and their ability to produce, absorb and alter volatiles (e.g., Korpi et al., 2009; Junker and Tholl, 2013), a contribution to the observed emission rates and δ13C values cannot be excluded and even seems likely in some cases. All VOCs presented in this study, except durene, have been detected to be also emitted from either bacteria or fungi as microbial VOC (mVOC) and can be found in the mVOC 2.0 (http://bioinformatics.charite.de/mvoc/) database (Lemfack et al., 2017). Especially, for substances with relatively low emission rates, the contribution of mVOC could affect the measured δ13C values and could add to the high variability of δ13C values of some VOCs, even though very little is known to date. Hence, the δ13C values of myrcene, trans-β-ocimene and linalool emitted by C. ladanifer and verbenone, bornyl acetate and benzaldehyde emitted by Q. suber could point toward a mixed or microbiome dominated origin of emissions. It is intriguing that the variability of δ13C values for those substances decreased strongly from June to July. The microbiome is known to change in richness and diversity in response to changes in UV light (Jacobs and Sundin, 2001; Lindow and Brandl, 2003) and seasonality, such as progressing drought conditions (Peñuelas et al., 2012). Thus, potential changes in the microbial composition and their emission spectrum from June to July could explain the reduced variability in δ13C values, but also the re-allocation of benzaldehyde from the second to the first cluster (Figure 4), however further research is needed. Benzaldehyde is a compound which is produced by many microbes present in the phyllo- and rhizosphere, e.g., Pedobacter sp. (Garbeva et al., 2014) or Pseudomonas sp. (Hunziker et al., 2015). Yet, to fully evaluate the contribution of the microbiome, the calculation of flux weighted effects would be necessary.
A contribution of mVOC emissions to total measured VOC emissions and δ13C values for other VOCs can also not be fully excluded, but might be negligible in some cases due to high plant emission rates. This seems in general to be the case for most monoterpenes found in the emissions of Q. suber, as α-pinene, limonene, myrcene, and sabinene are commonly the main BVOC components detected in this species (Staudt et al., 2004; Loreto et al., 2009; Haberstroh et al., 2018). To elucidate the influence and contribution of the microbiome to VOCs emitted at high rates by plants, more studies that are specific targeted at the microbiome, its emissions, and metabolism are certainly necessary. However, as the metabolism for mVOC for terpenoids and aromatic benzenoid compounds is believed to be similar to the secondary plant metabolism (Korpi et al., 2009), the scheme in Figure 5 might also be valid for the microbial secondary metabolism.
The results of our study demonstrate that the investigation of the carbon isotope composition at natural abundance has a great potential to elucidate different metabolic pathways and fractionation processes during the biosynthesis of BVOCs under field conditions. Up to date, we are only aware of a few studies taking advantage of this carbon isotope composition natural abundance approach in BVOC studies, namely Affek and Yakir (2003), Jux et al. (2001) and Tan et al. (2018). The advantages of this approach lie in the simplicity of the application, where no costly carbon labeling is necessary (Tan et al., 2018). In vivo sampling in field studies is especially important as conditions reflect the real world more closely than laboratory approaches, where most environmental factors are generally kept constant. Although we observed some variability in our carbon isotope data (Figure 3), the hierarchical clustering confirmed the validity of our approach, allocating BVOCs with a similar chemical structure into the same cluster (Figure 4). This is especially intriguing, as we sampled BVOCs under highly varying environmental conditions, such as PPFD (Figure S2) and air temperatures (Table 1) as well as emission rates (Figure 2) over diurnal courses. In addition, we investigated two species, which are known to possess dissimilar emission patterns (Haberstroh et al., 2018) and different reactions to changing environmental conditions (Caldeira et al., 2015). Moreover, single plant individuals of the same species can have a very distinct fingerprint regarding the composition and amplitude of their emissions, even at controlled environmental conditions (Loreto et al., 2009; Yáñez-Serrano et al., 2018). Nevertheless, both species and all individuals reacted similar in their photosynthetic discrimination to decreasing water availability, which reflected synonymously the carbon isotope ratio of emitted BVOCs. In conclusion, we show that the investigation of the carbon isotope composition at natural abundance can be a robust measure to further advance our knowledge on similarities and dissimilarities in the biosynthetic pathway of BVOCs. Moreover, the δ13C values detected in this study and the proposed metabolic pathways demonstrate how much there is still to learn about the secondary metabolism of plants.
All datasets for this study are included in the manuscript/Supplementary Files.
SH conducted the field work, statistical and data analysis, and wrote the manuscript. RL, HB, and HG conducted the field work with SH. JK and ME performed the TD-GC-MS and GC-C-IRMS analysis and processed the raw data. SH, JK, MC, and CW planned the experiment, conceived the study, and interpreted the data. All authors critically discussed and reviewed the manuscript.
We would like to acknowledge funding from the ERC project VOCO2 (647008), DFG (WE 2681/10-1), and FCT, the Portuguese Fundação para a Ciência e a Tecnologia I.P. (Lisboa-01-0145-FEDER-030406–PTDC/ASP-SIL/3406/2017). SH would like to acknowledge funding from the Studienstiftung des deutschen Volkes (Promotionsförderung). RL was funded by a postdoctoral fellowship from the FCT (SFRH/BPD/86938/2012). Centro de Estudos Florestais (CEF) is a research unit funded by FCT, Portugal (UID/AGR/00239/2019). Furthermore, we would like to acknowledge funding from the German Academic Exchange Service (DAAD) in the bilateral exchange program (2100206701) for HG. The article processing charge was funded by the German Research Foundation (DFG) and the University of Freiburg in the funding program Open Access Publishing.
The authors declare that the research was conducted in the absence of any commercial or financial relationships that could be construed as a potential conflict of interest.
We sincerely thank Joaquim Mendes, Jasper Mohr, and Anna Höfer for field assistance. Many thanks go to Maren Dubbert for the organization and management of the bilateral exchange program of the DAAD. We thank the Fundação da Casa de Bragança for permission to undertake research at the field site. We also thank Filip Volders for his help to set up the GC-C-IRMS.
The Supplementary Material for this article can be found online at: https://www.frontiersin.org/articles/10.3389/ffgc.2019.00055/full#supplementary-material
Affek, H. P., and Yakir, D. (2003). Natural abundance carbon isotope composition of isoprene reflects incomplete coupling between isoprene synthesis and photosynthetic carbon flow. Plant Physiol. 131, 1727–1736. doi: 10.1104/pp.102.012294
Albino, R. C., Oliveira, P. C., Prosdocimi, F., da Silva, O. F., Rizzo, H. R., Gama, P. E., et al. (2017). Oxidation of monoterpenes in Protium heptaphyllum oleoresins. Phytochemistry 136, 141–146. doi: 10.1016/j.phytochem.2017.01.013
Bouchard, D., Höhener, P., and Hunkeler, D. (2008). Carbon isotope fractionation during volatilization of petroleum hydrocarbons and diffusion across a porous medium: a column experiment. Environ. Sci. Technol. 42, 7801–7806. doi: 10.1021/es800391s
Caldeira, M. C., Lecomte, X., David, T. S., Pinto, J. G., Bugalho, M. N., and Werner, C. (2015). Synergy of extreme drought and shrub invasion reduce ecosystem functioning and resilience in water-limited climates. Sci. Rep. 5:15110. doi: 10.1038/srep15110
Cernusak, L. A., Ubierna, N., Winter, K., Holtum, J. A. M., Marshall, J. D., and Farquhar, G. D. (2013). Environmental and physiological determinants of carbon isotope discrimination in terrestrial plants. New Phytol. 200, 950–965. doi: 10.1111/nph.12423
Chikaraishi, Y., Naraoka, H., and Poulson, S. R. (2004). Carbon and hydrogen isotopic fractionation during lipid biosynthesis in a higher plant (Cryptomeria japonica). Phytochemistry 65, 323–330. doi: 10.1016/j.phytochem.2003.12.003
Correia, O., and Ascensao, L. (2016). “Summer semi-deciduous species of the Mediterranean landscape: a winning strategy of cistus species to face the predicted changes of the Mediterranean climate,” in Plant Biodiversity. Monitoring, Assessment and Conservation, eds A. A. Ansari, S. S. Gill, Z. K. Abbas, and M. Naeem (Oxfordshire, UK: CAB International, 195–217.
Correia, O., Catarino, F. M., Tenhunen, J. D., and Lange, O. L. (1987). “Regulation of water use by four species of Cistus in the scrub vegetation of the Serra da Arrábida, Portugal,” in Plant responses to stress. Functional analysis in Mediterranean ecosystems, eds J. D. Tenhunen, F. M. Catarino, O. L. Lange, and W. C. Oechel (Berlin;Heidelberg: NATO ASI Series;Springer-Verlag, 247–258.
Croteau, R., Gershenzon, J., Wheeler, C. J., and Satterwhite, D. M. (1990). Biosynthesis of monoterpenes: steroechemistry of the coupled isomerization and cyclization of geranyl pyrophosphate to camphene and isocamphane monoterpenes. Arch. Biochem. Biophys. 227, 374–381. doi: 10.1016/0003-9861(90)90593-N
Croteau, R., Satterwhite, D. M., Cane, D. E., and Chang, C. C. (1988). Biosynthesis of monoterpenes. J. Biol. Chem. 263, 10063–10071.
David, T. S., Henriques, M. O., Kurz-Besson, C., Nunes, J., Valente, F., Vaz, et al. (2007). Water-use strategies in two co-occurring Mediterranean evergreen oaks: surviving the summer drought. Tree Physiol. 27, 793–803. doi: 10.1093/treephys/27.6.793
Davis, E. M., and Croteau, R. (2000). Cyclization enzymes in the biosynthesis of monoterpenes, sesquiterpenes, and diterpenes. Top. Curr. Chem. 209, 54–95. doi: 10.1007/3-540-48146X_2
Dudareva, N., Klempien, A., Muhlemann, J. K., and Kaplan, I. (2013). Biosynthesis, function and metabolic engineering of plant volatile organic compounds. New Phytol. 198, 16–32. doi: 10.1111/nph.12145
Dudareva, N., Negre, F., Nagegowda, D. A., and Orlova, I. (2006). Plant volatiles: recent advances and future perspectives. Criti. Rev. Plant Sci. 25, 417–440. doi: 10.1080/07352680600899973
Dudareva, N., Pichersky, E., and Gershenzon, J. (2004). Biochemistry of plant volatiles. Plant Physiol. 135, 1893–1902. doi: 10.1104/pp.104.049981
Ehleringer, J. R., and Cerling, T. E. (2002). “C3 and C4 photosynthesis,” in The Earth System: Biological and Ecological Dimensions of Global Environmental Change, eds H. A. Mooney, J. G. Canadell (Chinchester: John Wiley & Sons Ltd., 186–190.
FAO (2006). World Reference Base for Soil Resources 2006. A Framework for International Classification, Correlation and Communication. Rome: Food and Agriculture Organization of the United Nations.
Farquhar, G. D., O'Leary, M. H., and Berry, J. A. (1982). On the relationship between carbon isotope discrimination and the intercellular carbon dioxide concentration in leaves. Aust. J. Plant Physiol. 9, 121–137. doi: 10.1071/PP9820121
Fatland, B. L., Ke, J., Anderson, M. D., Mentzen, W. I., Cui, L. W., Allred, C. C., et al. (2002). Molecular characterization of a heteromeric ATP-citrate lyase that generates cytosolic acetyl-coenzyme A in Arabidopsis. Plant Physiol. 130, 740–756. doi: 10.1104/pp.008110
Fineschi, S., Loreto, F., Staudt, M., and Peñuelas, J. (2013). “Diversification of volatile isoprenoid emissions from trees: evolutionary and ecological perspectives,” in Biology, Controls and Models of Tree Volatile Organic Compounds Emissions, eds Ü. Niinemets (Berlin: Springer, 209–235.
Garbeva, P., Hordijk, C., Gerards, S., and de Boer, W. (2014). Volatile-mediated interactions between phylogenetically different soil bacteria. Front. Microbiol. 5:289. doi: 10.3389/fmicb.2014.00289
Gilbert, A., Silvestre, V., Segebarth, N., Tcherkez, G., Guillou, C., Robins, R. J., et al. (2011). The intramolecular 13C-distribution in ethanol reveals the influence of the CO2-fixation pathway and environmental conditions on the site-specific 13C variation in glucose. Plant Cell Environ. 34, 1104–1112. doi: 10.1111/j.1365-3040.2011.02308.x
Gleixner, G., Danier, H. J., Werner, R. A., and Schmidt, H. L. (1993). Correlations between the 13C content of primary and secondary plant products in different cell compartments and that in decomposing basidiomycetes. Plant Physiol. 102, 1287–1290. doi: 10.1104/pp.102.4.1287
Gleixner, G., Scrimgeour, C., Schmidt, H. L., and Viola, R. (1998). Stable isotope distribution in the major metabolites of source and sink organs of Solanum tuberosum L.: a powerful tool in the study of metabolic partitioning in intact plants. Planta 207, 241–245. doi: 10.1007/s004250050479
Gleizes, M., Marpeau, A., Pauly, G., and Bernard-Dagan, C. (1982). Role of acyclic compounds in monoterpene biosynthesis in Pinus pinaster. Phytochemistry 21, 2641–2644. doi: 10.1016/0031-9422(82)83091-4
Grant, O. M., Tronina, Ł., Ramalho, J. C., Besson, C. K., Lobo-do-Vale, R., Pereira, J. S., et al. (2010). The impact of drought on leaf physiology of Quercus suber L. trees: comparison of an extreme drought event with chronic rainfall reduction. J. Exp. Bot. 61, 4361–4371. doi: 10.1093/jxb/erq239
Gülz, P. G., Herrmann, T., and Hangst, K. (1996). Leaf trichomes in the genus Cistus. Flora 191, 85–104. doi: 10.1016/S0367-2530(17)30692-8
Haberstroh, S., Kreuzwieser, J. K., Lobo-do-Vale, R., Caldeira, M. C., and Werner, C. (2018). Terpenoid emissions of two Mediterranean woody species in response to drought stress. Front. Plant Sci. 9:1071. doi: 10.3389/fpls.2018.01071
Harley, P. C. (2013). “The roles of stomatal conductance and compound volatility in controlling the emission of volatile organic compounds from leaves,” in Biology, Controls and Models of Tree Volatile Organic Compounds Emissions, eds Ü. Niinemets (Berlin: Springer), 181–208. doi: 10.1007/978-94-007-6606-8_8
Hunziker, L., Bönisch, D., Groenhagen, U., Bailly, A., Schulz, S., and Weisskopf, L. (2015). Pseudomonas strains naturally associated with potato plants produce volatiles with high potential for inhibition of Phytophthora infestans. Appl. Eviron. Biol. 81, 821–830. doi: 10.1128/AEM.02999-14
Jacobs, J. L., and Sundin, G. W. (2001). Effect of solar UV-B radiation on a phyllosphere bacterial community. Appl. Environ. Biol. 67, 5488–5496. doi: 10.1128/AEM.67.12.5488–5496.2001
Junker, R. R., and Tholl, D. (2013). Volatile organic compound mediated interactions at the plant-microbe interface. J. Chem. Ecol. 39, 810–825. doi: 10.1007/s10886-013-0325-9
Jux, A., Gleixner, G., and Boland, W. (2001). Classification of terpenoids according to the methylerythritolphosphate or the mevalonate pathway with natural 12C/13C isotope ratios: dynamic allocation of resources in induced plants. Angew. Chem. Int. Ed. Engl. 40, 2091–2093. doi: 10.1002/1521-3773(20010601)40:11<2091::AID-ANIE2091>3.0.CO;2-5
Kesselmeier, J., and Staudt, M. (1999). Biogenic volatile organic compounds (VOC): an overview on emission, physiology and ecology. J. Atmos. Chem. 33, 23–88. doi: 10.1023/A:100612751679
Kleiber, A., Duan, Q., Jansen, K., Junker, L. V., Kammerer, B., Rennenberg, H., et al. (2017). Drought effects on root and needle terpenoid content of a coastal and interior Douglas fir provenance. Tree Physiol. doi: 10.1093/treephys/tpx113
Korpi, A., Järnberg, J., and Pasanen, A. L. (2009). Microbial volatile organic compounds. Crit. Rev. Toxicol. 39, 139–193. doi: 10.1080/10408440802291497
Lemfack, M. C., Gohlke, B. O., Toguem, S. M. T., Preissner, S., Piechulla, B., and Preissner, R. (2017). mVOC 2.0: a database of microbial volatiles. Nucleic Acids Res. 46, D1261–D1265. doi: 10.1093/nar/gkx1016
Lin, M., and Oliver, D. J. (2008). The role of acetyl-coenzyme a synthetase in Arabidopsis. Plant Physiol. 147, 1822–1829. doi: 10.1104/pp.108.121269
Lindmark-Henriksson, M., Isaksson, D., Sjödin, K., Högberg, H. E., Vanek, T., and Valterová, I. (2003). Transformation of α-pinene using Picea abies suspension culture. J. Nat. Prod. 66, 337–343. doi: 10.1021/np020426m
Lindow, S. E., and Brandl, M. T. (2003). Microbiology of the phyllosphere. Appl. Environ. Microbiol. 69, 1875–1883. doi: 10.1128/AEM.69.4.1875–1883.2003
Lluisà, J., Peñuelas, J., Ogaya, R., and Alessio, G. (2010). Annual and seasonal changes in foliar terpene content and emission rates in Cistus albidus L. submitted to soil drought in Prades forest (Catalonia, NE Spain). Acta Physiol. Plant. 32, 387–394. doi: 10.1007/s11738-009-0416-y
Loreto, F., Bagnoli, F., and Fineschi, S. (2009). One species, many terpenes: matching chemical and biological activity. Trends Plant Sci. 14, 416–420. doi: 10.1016/j.tplants.2009.06.003
Loreto, F., Ciccioli, Cecinato, A., Brancaleoni, E., Frattoni, M., Fabozzi, C., et al. (1996). Evidence of the photosynthetic origin of monoterpenes emitted by Quercus ilex L. leaves by 13C labeling. Plant Physiol. 110, 1317–1322. doi: 10.1104/pp.110.4.1317
Loreto, F., Förster, A., Dürr, M., and Seufert, G. (1998). On the monoterpene emission under heat stress and on the increased thermotolerance of leaves of Quercus ilex L. fumigated with selected monoterpenes. Plant Cell Environ. 21, 101–107. doi: 10.1046/j.1365-3040.1998.00268.x
Lynch, A. H., Kruger, N. J., Hedges, R. E. M., and McCullagh, J. S. O. (2016). Variability in the carbon isotope composition of individual amino acids in plant proteins from different sources: 1 Leaves. Phytochemistry 125, 27–34. doi: 10.1016/j.phytochem.2016.01.011
McGarvey, D. J., and Croteau, R. (1995). Terpenoid metabolism. Plant Cell 7, 1015–1026. doi: 10.1105/tpc.7.7.1015
Meier-Augenstein, W. (1999). Applied gas chromatography coupled to isotope ratio mass spectrometry. J. Chromatogr. 842, 351–371. doi: 10.1016/S0021-9673(98)01057-7
Melzer, E., and Schmidt, H. L. (1987). Carbon isotope effects on the pyruvate dehydrongenase reaction and their importance for relative carbon-13-C depletion in lipids. J. Biol. Chem. 262, 8159–8164.
Niinemets, Ü., Seufert, G., Steinbrecher, R., and Tenhunen, J. D. (2002). A model coupling foliar monoterpene emissions to leaf photosynthetic characteristics in Mediterranean evergreen Quercus species. New Phytol. 153, 257–275. doi: 10.1046/j.0028-646X.2001.00324.x
O'Leary, M. H. (1981). Carbon isotope fractionation in plants. Phytochemistry 20, 553–567. doi: 10.1016/0031-9422(81)85134-5
O'Leary, M. H. (1988). Carbon isotopes in photosynthesis. BioScience 38, 328–336. doi: 10.2307/1310735
Peñuelas, J., Rico, L., Jump, A. S., and Terradas, J. (2012). Summer season and long-term drought increase the richness of bacteria and fungi in the foliar phyllosphere of Quercus ilex in a mixed Mediterranean forest. Plant Biol. 14, 565–575. doi: 10.1111/j.1438-8677.2011.00532.x
Pereira Limberger, R., Mendes Aleixo, A., Fett-Neto, A., and Henriques, A. T. (2007). Bioconversion of (+)- and (-)-alpha-pinene to (+)- and (-)-verbenone by plant cell cultures of Psychotria brachyceras and Rauvolfia sellowii. Electronic J. Biotechnol. 10, 501–507. doi: 10.2225/vol10-issue4-fulltext-8
Pio, C. A., Nunes, T. V., and Brito, S. (1993). “Volatile hydrocarbon emissions from common and native species of vegetation in Portugal,” in General Assessment of Biogenic Emissions and Deposition of Nitrogen Compounds, Sulphur Compounds and Oxidants in Europe, eds J. Slanina, G. Angeletti, and S. Beilke (Brussels: CEC Air Pollution Research Report 47, E. Guyot SA, 291–298.
Pio, C. A., Silva, P. A., Cerqueira, M. A., and Nunes, T. V. (2005). Diurnal and seasonal emissions of volatile organic compounds from cork oak (Quercus suber) trees. Atmos. Environ. 39, 1817–1827. doi: 10.1016/j.atmosenv.2004.11.018
Poulose, A. J., and Croteau, R. (1978). Biosynthesis of aromatic monoterpenes. Arch. Biochem. Biophys. 187, 307–314. doi: 10.1016/0003-9861(78)90039-5.
Priault, P., Wegener, F., and Werner, C. (2009). Pronounced differences in diurnal variation of carbon isotope composition of leaf respired CO2 among functional groups. New Phytol. 181, 400–412. doi: 10.1111/j.1469-8137.2008.02665.x
Schmidt, H. L. (2003). Fundamentals and systematics of the non-statistical distributions of isotopes in natural compounds. Naturwissenschaften 90, 537–552. doi: 10.1007/s00114-003-0485-5
Schmidt, H. L., and Kexel, H. (1998). Metabolite pools and metabolic branching as factors of in-vivo isotope discriminations by kinetic isotope effects. Isotopes Environ. Health Stud. 34, 19–30. doi: 10.1080/10256019708036328
Schmidt, H. L., Robins, R. J., and Werner, R. A. (2015). Multi-factorial in vivo stable isotopes fractionation: causes, correlations, consequences and applications. Isotopes Environ. Health Stud. 51, 155–199. doi: 10.1080/10256016.2015.1014355
Shimada, T., Endo, T., Fujii, H., Hara, M., and Omura, M. (2005). Isolation and characterization of (E)-beta-ocimene and 1,8 cineole synthases in Citrus unshiu Marc. Plant Sci. 168, 987–995. doi: 10.1016/j.plantsci.2004.11.012
Staudt, M., Mir, C., Joffre, R., Rambal, S., Bonin, A., Landais, D., et al. (2004). Isoprenoid emissions of Quercus spp. (Q. suber and Q. ilex) in mixed stands contrasting in interspecific genetic introgression. New Phytol. 163, 573–584. doi: 10.1111/j.1469-8137.2004.01140.x
Tan, W., Bartram, S., and Boland, W. (2018). Mechanistic studies of sesquiterpene cyclases based on their carbon isotope ratios at natural abundance. Plant Cell Environ. 41, 39–49. doi: 10.1111/pce.12901
Teixeira, S., Mendes, A., Alves, A., and Santos, L. (2007). Simultaneous distillation-extraction of high-value volatile compounds from Cistus ladanifer L. Anal. Chim. Acta 584, 439–446. doi: 10.1016/j.aca.2006.11.054
Tholl, D. (2015). Biosynthesis and biological functions of terpenoids in plants. Adv. Biochem. Eng. Biotechnol. 148, 63–106. doi: 10.1007/10_2014_295
Vanek, T., Halík, J., Vanková, R., and Valterová, I. (2005). Formation of trans-verbenol and verbenone from α-pinene catalysed by immobilised Picea abies cells. Biosci. Biotechnol. Biochem. 69, 321–325. doi: 10.1271/bbb.69.321
Vitzthum von Eckstaedt, C. D., Grice, K., Ioppolo-Armanios, M., Kelly, D., and Gibberd, M. (2012). Compound specific carbon and hydrogen stable isotope analyses of volatile organic compounds in various emissions of combustion processes. Chemosphere 89, 1407–1413. doi: 10.1016/j.chemosphere.2012.06.005
Wagschal, K. C., Pyun, H. J., Coates, R. M., and Croteau, R. (1994). Monoterpene biosynthesis: isotope effects associated with bicyclic olefin formation catalysed by pinene synthases from Sage (Salvia officinalis). Arch. Biochem. Biophys. 308, 477–487. doi: 10.1006/abbi.1994.1068
Werner, C., Correia, O., and Beyschlag, W. (1999). Two different strategies of Mediterranean macchia plants to avoid photoinhibitory damage by excessive radiation levels during summer drought. Acta Oecol. 20, 15–23. doi: 10.1016/S1146-609X(99)80011-3
Werner, C., and Gessler, A. (2011). Diel variations in the carbon isotope composition of respired CO2 and associated carbon sources: a review of dynamics and mechanisms. Biogeosciences 8, 2437–2459. doi: 10.5194/bg-8-2437-2011
Keywords: BVOC, stable isotopes, 13C, discrimination, Quercus suber, Cistus ladanifer, terpenoids, benzenoids
Citation: Haberstroh S, Kreuzwieser J, Boeddeker H, Eiblmeier M, Gutte H, Lobo-do-Vale R, Caldeira MC and Werner C (2019) Natural Carbon Isotope Composition Distinguishes Compound Groups of Biogenic Volatile Organic Compounds (BVOC) in Two Mediterranean Woody Species. Front. For. Glob. Change 2:55. doi: 10.3389/ffgc.2019.00055
Received: 01 April 2019; Accepted: 04 September 2019;
Published: 18 September 2019.
Edited by:
Jaana Bäck, University of Helsinki, FinlandReviewed by:
Pawel K. Misztal, University of California, Berkeley, United StatesCopyright © 2019 Haberstroh, Kreuzwieser, Boeddeker, Eiblmeier, Gutte, Lobo-do-Vale, Caldeira and Werner. This is an open-access article distributed under the terms of the Creative Commons Attribution License (CC BY). The use, distribution or reproduction in other forums is permitted, provided the original author(s) and the copyright owner(s) are credited and that the original publication in this journal is cited, in accordance with accepted academic practice. No use, distribution or reproduction is permitted which does not comply with these terms.
*Correspondence: Simon Haberstroh, c2ltb24uaGFiZXJzdHJvaEBjZXAudW5pLWZyZWlidXJnLmRl
Disclaimer: All claims expressed in this article are solely those of the authors and do not necessarily represent those of their affiliated organizations, or those of the publisher, the editors and the reviewers. Any product that may be evaluated in this article or claim that may be made by its manufacturer is not guaranteed or endorsed by the publisher.
Research integrity at Frontiers
Learn more about the work of our research integrity team to safeguard the quality of each article we publish.