- 1Forest Ecology, Department of Forest and Soil Sciences, University of Natural Resources and Life Sciences Vienna (BOKU), Vienna, Austria
- 2Institute of Atmospheric and Cryospheric Sciences, University of Innsbruck, Innsbruck, Austria
- 3Research Centre for Forestry and Wood, Council for Agricultural Research and Economics (CREA), Rome, Italy
- 4National Research Council of Italy (CNR), Institute of Research on Terrestrial Ecosystems (IRET), Rome, Italy
- 5Institute of Meteorology and Climate Research, Atmospheric Environmental Research Division (IMK-IFU), Karlsruhe Institute of Technology, Garmisch-Partenkirchen, Germany
- 6Department of Environmental and Biological Sciences, University of Eastern Finland, Kuopio, Finland
Tropospheric ozone (O3) is one of the most prominent air pollution problems in Europe and other countries worldwide. Human health is affected by O3 via the respiratory as well the cardiovascular systems. Even though trees are present in relatively low numbers in urban areas, they can be a dominant factor in the regulation of urban O3 concentrations. Trees affect the O3 concentration via emission of biogenic volatile organic compounds (BVOC), which can act as a precursor of O3, and by O3 deposition on leaves. The role of urban trees with regard to O3 will gain further importance as NOx concentrations continue declining and climate warming is progressing—rendering especially the urban ozone chemistry more sensitive to BVOC emissions. However, the role of urban vegetation on the local regulation of tropospheric O3 concentrations is complex and largely influenced by species-specific emission rates of BVOCs and O3 deposition rates, both highly modified by tree physiological status. In this review, we shed light on processes related to trees that affect tropospheric ozone concentrations in metropolitan areas from rural settings to urban centers, and discuss their importance under present and future conditions. After a brief overview on the mechanisms regulating O3 concentrations in urban settings, we focus on effects of tree identity and tree physiological status, as affected by multiple stressors, influencing both BVOC emission and O3 deposition rates. In addition, we highlight differences along the rural-urban gradient affecting tropospheric O3 concentrations and current knowledge gaps with the potential to improve future models on tropospheric O3 formation in metropolitan areas.
Introduction
Ground-level ozone (O3, tropospheric ozone) is one of the most prominent air pollution problems in Europe and other countries worldwide. When taken-up by plants, O3 irreversibly damages plant tissue-leading to reduced crop yields and forest growth (Mills et al., 2011). Human health is temporarily or chronically affected by O3 via the respiratory as well the cardiovascular systems (WHO, 2006). The World Health Organization (WHO) has repeatedly released updated recommendations regarding ozone exposure limits. In order to avoid negative impacts on human health, the alert threshold for 8-h average O3 exposure was lowered in 2005 from 120 μg m−3 (60 ppb) to 100 μg m−3 (50 ppb), and short-time concentrations should not exceed 160 μg m−3 (80 ppb, WHO, 2006). However, many (supra-) national legislations incl. the European Unions' (EU) Air Quality Directive (EU, 2008) are allowing higher O3 exposures (e.g., EU target value 120 μg m−3 not to be exceeded on >25 days year−1, averaged over 3 years). The set thresholds are frequently reached or exceeded in urban areas, especially during heat waves (Solberg et al., 2008; Holman et al., 2015). Recent data indicates that 95–99 % of the EU urban population were exposed to O3 concentrations above WHO air quality guidelines in the period 2006–2016 (EEA, 2018a,b). However, within metropolitan areas large temporal and spatial heterogeneities of tropospheric O3 levels exist (Paoletti et al., 2011; Calfapietra et al., 2016), which are influenced by a distinct rural-urban gradient and a broad range of abiotic and biotic factors (Sicard et al., 2013; Lahr et al., 2015; Hagenbjörk et al., 2017).
The recent literature on urban forests and greening (“green urban infrastructure”) largely advocates the environmental services of trees such as air pollution mitigation (e.g., CO2 sequestration, dry deposition), storm water interception, and heat mitigation (Escobedo et al., 2011; Connop et al., 2016; Livesley et al., 2016). In general, the substantial scientific evidence on many positive effects has led to a normative assertion by stakeholders that any increase in urban forests is desirable and will mitigate virtually all-kinds of environmental problems (Escobedo et al., 2011). However, the role of urban vegetation on the local regulation of tropospheric O3 concentrations is complex. Among the different urban vegetation forms, trees are key as they can both remove (Hardin and Jensen, 2007) but also contribute significantly to O3 formation (Jenkin et al., 2015). In addition, air chemistry reactions and thus O3 formation are indirectly affected by the cooling and shading properties of vegetation (Yli-Pelkonen et al., 2018). The net effect of a tree on the O3 concentration depends on tree species, its physiological status, environmental drivers of emission, as well as climate and air chemistry such as concentrations of volatile organic compounds (VOCs; see Box 1 for glossary) and nitrogen oxides (NOx). The emission of biogenic VOC (BVOC) by trees as source of tropospheric O3, will gain further importance as climate change progresses (Peñuelas and Staudt, 2010; Trenberth et al., 2014). It is also expected that future urban NOx concentrations will continue to decline by substituting combustion-powered vehicles, especially Diesel-powered models, with cleaner ones (O'Driscoll et al., 2018). Consequently, urban ozone chemistry might become more sensitive to BVOC emissions. While not all BVOCs will be equally effective and in some cases may actually contribute to a decomposition of O3 (Bonn et al., 2018; Neirynck and Verstraeten, 2018), the combination of higher urban temperatures and lower levels of NOx is generally thought to increase the importance of BVOCs as precursors of ozone in future urban environments (Chameides et al., 1988).
In this review, we summarize processes related to trees that affect tropospheric ozone concentrations in metropolitan areas from rural settings to urban centers, and discuss their importance under present and future conditions. Urban trees differ in their potential BVOC emission rates and are often exposed to a multiple and high stress environment, that is also particularly prone to climate change impacts; thus, we especially focus on tree species-specific effects and the physiological status as (potential) major factors influencing ground-level O3 formation. In addition, the review will highlight knowledge gaps, e.g., concerning the spatial distribution of species and environmental factors along rural-urban gradients, with the potential to improve future models on tropospheric O3 formation in metropolitan areas.
Tropospheric Ozone Formation and Sinks
The O3 forming process in the troposphere is generally catalyzed by nitrogen oxides in the presence of radiation, and fueled by methane, carbon monoxide and non-methane volatile organic compounds (NMVOC). The general pathways to tropospheric O3 formation are well understood (Brasseur et al., 1999), but the complexity of organic chemistry is still subject to intensive research (Fittschen et al., 2017). Ozone formation is always initiated by the photolysis of NO2. The recycling of NO to NO2 occurs after CO, methane or NMVOC react with OH and generate hydroxyl and peroxy radicals. These readily react with NO, regenerating NO2. Thus, NOx is recycled in what is referred to as the ROx-NOx cycle (Figure 1).
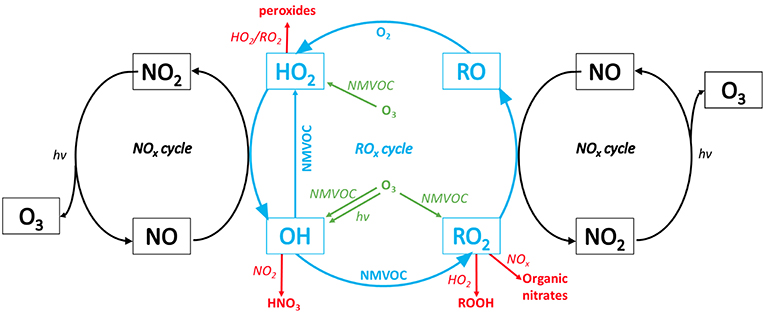
Figure 1. Ozone formation via the interactions between the ROx- (blue) and NOx-cycles (black). Interfering with the ROx-NOx cycle are ozone degrading reactions (green) via NMVOCs (non-methane volatile organic compounds) as well as terminating reactions (red); hν, light. Methane and carbon monoxide are not displayed (modified after Wang et al., 2017 with permission).
At low NOx concentrations, O3 formation becomes NOx limited. At medium NOx concentrations, OH recycling via the ROx-NOx cycle becomes efficient to sustain the oxidation of high levels of NMVOCs, which finally leads to a regime, where O3 formation becomes VOC-limited (Carter, 1994). The O3 forming potential also depends on the VOCs' reactivity, which differs among compounds. Isoprene, for example, is the most common BVOC and has a reactivity which is 22 times higher than that of the important anthropogenic VOC (AVOC) benzene (Carter, 1994; Wagner and Kuttler, 2014). The reaction rate constants of CO and methane with respect to OH are about 410 and 14,000 times lower than that of isoprene, which can therefore compete with their reactivity in many environments. For a more detailed description of the chemical reactions involved in tropospheric chemistry we refer to the specialized literature (Atkinson, 2000; Kirchner et al., 2001; Jenkin et al., 2015; Wang et al., 2017).
Recent measurements of OH reactivity (Kim et al., 2011; Kaiser et al., 2016; Nölscher et al., 2016; Williams et al., 2016) have suggested missing emission sources of reactive NMVOCs and missing secondary atmospheric reactions leading to reactive NMVOCs. This uncertainty is largely attributed to the complexity of organic chemistry and precursor emissions. On global and regional scales, BVOCs play a central role in tropospheric chemistry because of their reactivity with respect to OH (Guenther et al., 2012). While plants can release more than 30,000 different BVOCs (Trowbridge and Stoy, 2013), only a few are emitted in relatively large quantities and are highly reactive with atmospheric radicals (Atkinson and Arey, 2003). The most reactive classes of BVOC emitted by plants are isoprene (30%), monoterpenes and higher terpenoids (25 %; e.g., sesquiterpenes) whereas oxygenated VOCs (OVOC) are present in lower amounts and less reactive (Atkinson and Arey, 2003; Atkinson, 2007). Based on the maximum incremental reactivity scale (MIR), e.g., isoprene (MIR 10.6) reacts faster with ozone than monoterpenes (MIR 4.0) (Carter, 2000, 2010). Once in the atmosphere, isoprene is undergoing complex chemical cycling initiated by photochemistry, a fate all NMVOC have in common and are leading to a vast network of chemical reactions. Similar, higher molecular weight terpenes (>C15) can also play a significant role in atmospheric chemistry due to their reactivity (Atkinson and Arey, 2003).
Once being produced, O3 has several routes of removal. The most important ones include dry deposition and chemical loss (Monks et al., 2009; IPCC, 2014). On a global scale, the most important chemical loss term is the photolysis of O3 in the atmosphere and subsequent reaction with water vapor (H2O) leading to the production of OH radicals (Brasseur et al., 1999). Globally a gross chemical O3 loss of 4,260 ± 264 Tg year−1 and a net chemical production of 633 ± 275 Tg year−1 was estimated (Lamarque et al., 2013). Under high NOx emissions (e.g., in an urban context) another chemical conversion of O3 is triggered by the reaction with NO, leading to a temporary production of excess NO2. The reactions between NO, NO2, and O3 are often termed as the triad and represent a null cycle, where no net O3 is produced or removed (Figure 1, NOx cycle). Yet locally, O3 is chemically converted to NO2 and it can be speculated that O3 deposition can also be facilitated via NO2 uptake by plants. The second largest sink for O3 is deposition on surfaces. Estimates of O3 dry deposition are in the order of 1,094 ± 309 Tg year−1 (Young et al., 2013). Further, another sink of O3 is the ozonolysis of VOCs forming secondary organic aerosols (SOAs), initiated by O3 degrading C-C double bonds in alkenes and leading to the formation of energy-rich Criegee biradicals (Criegee, 1975; Pinto et al., 2010).
Whether the O3 level increases or decreases by VOCs is depending on the chemical species and the relative concentrations of the involved compounds (Sillman and He, 2002). To implement effective mitigation strategies, the relationship between the different reaction partners as well as their current absolute concentrations has to be considered.
Impact of Tree Species and Their Physiological Condition on the Ozone Dynamics of Urban Environments
Quantity and quality of BVOC emissions and O3 removal by urban trees depends on community composition, and short- and long-term environmental conditions—affecting tree size and physiological status. In the following, an overview is given on key traits and stressors influencing O3 removal and BVOC emission potential of urban tree vegetation—referring mainly to European examples to keep a manageable length and scope.
Species-Specific Effects
Community Composition
The sensitivity of plants to specific environmental conditions determines urban species composition, either by affecting mortality of established trees or by suggesting selection preferences to urban planners (Holmes et al., 2013). Three main locations of urban vegetation can be distinguished: street trees (“road-side” trees), parks and private gardens, and urban woodlands. Street trees are exposed to relatively high stress levels and the average lifespan of the trees is short, while trees in parks and private gardens are commonly exposed to less stress. Trees in urban woodland are subjected to relatively low stress levels and may reach a lifespan and size comparable to forests (Sæbø et al., 2005). The environmental conditions and the frequency of these different vegetation types, and thus species community composition, differs largely along rural-urban gradients as discussed below (section The Rural-Urban Gradient in Context of Tree-Ozone Interactions). However, despite an overall high number of species available for planning, only relatively few tree genera and species are highly abundant in streets and parks of northern and central European cities (Grote et al., 2016; Samson et al., 2017b). Common urban species in central to northern Europe are in particular: Tilia sp., Platanus sp., Aesculus sp., Fraxinus sp., Quercus sp., Acer sp., Picea abies, and Pinus sp. Additionally, Fagus sylvatica occurs frequently in parks, private gardens and urban woodlands. In southern Europe, a broader species pool is utilized but likewise dominated by a few tree genera and species: Platanus × acerifolia, Quercus ilex, Robinia pseudoacacia, Celtis australis, Celtis occidentalis, Sophora japonica, Morus sp., Populus sp., Ulmus sp., Pinus sp., and Prunus sp. (Pauleit et al., 2002; Grote et al., 2016). There is a general trend to introduce more exotic tree species that are supposed to withstand progressing “global warming” and diseases in urbanized areas better than their autochthonous counterparts (Youngsteadt et al., 2015). However, whether or not replacing native species with exotic species is still under debate as neophytes may disturb the ecological relationships between co-evolved species (Cornelis and Hermy, 2004; Kühn et al., 2004).
Under standard conditions, the contribution of an individual tree to either O3 removal or BVOC emissions depends primarily on species-specific traits [e.g., high or low BVOC emission rates, (Samson et al., 2017a)], and gaseous exchange is proportional to the size of its canopy, as measured by its leaf mass or surface area (Harrison et al., 2013; Vos et al., 2013). Similar “size-symmetric”-effects of larger tree individuals have been found with regard to air pollution mitigation in particularly when the main removal process is surface deposition rather than stomatal adsorption (Grote et al., 2016). However, large and dense canopies, for example, may allow emitted BVOCs to react on leaf surfaces within the canopy before reacting with gases (Forkel et al., 1999); vice versa ozone deposition on leaf surfaces within dense canopies may be reduced due to a non-uniform distribution of ozone sources (Zhu et al., 2009)—attenuating “size-symmetric”-effects.
It should be furthermore considered that canopy size, shape, and density of street trees, despite being highly species/cultivar-specific, can largely be modified by management measures (Janhäll, 2015). Therefore, allometric relationships typically used in the forestry sector (e.g., to determine biomass and crown size from stem diameter and tree height) generally fail to predict tree attributes in the urban context. Surprisingly, closed canopies of trees may contribute to higher pollution in urban canyons by decreasing dispersion (Gromke and Ruck, 2009; Vos et al., 2013). However, this effect is beneficial for pedestrians and bikers when the lanes are located just on the external side of the road (outside the canyon).
Ozone Deposition Capacity
Vegetation plays a major role for the dry depositional sink of O3. Dry deposition over lush vegetation is 40 times more efficient than over urban land according to common parameterizations in atmospheric models (Wesely, 1989). Deposition of O3 to vegetation is modulated by a stomatal and a non-stomatal component. In either case, the removal of O3 is ultimately driven by a chemical loss. Stomatal O3 uptake triggers antioxidant reactions in the intercellular space and is largely dependent on stomatal conductance, which is driven by environmental conditions and varies among tree species (as discussed below). If the stomata are open, O3 diffuses into the intercellular space from the atmosphere and is almost immediately deposited by reactions in the apoplast with membrane lipids, moisture and cell organelles; uptake increases with increasing outside concentrations (Wesely and Hicks, 2000). Non-stomatal O3 deposition occurs on leaf surfaces by reactions with waxes, moisture and salts (Barnes et al., 1988; Altimir et al., 2006) and in the boundary layer with emitted BVOCs (see sections Tropospheric Ozone Formation, and Sinks and BVOC Emission Rates). Tree species featuring traits such as a large leaf area index, hairy leaves, leaf waxes and moist surfaces, and keeping their stomata longer open under stressed conditions (see below, section Plant Water Status), are depositing O3 better than species without these traits (Barnes et al., 1988; Altimir et al., 2006; Cape et al., 2009). However, Calfapietra et al. (2016) showed that under standardized conditions, most common tree species in Rome, Italy had rather similar O3 deposition rates per m2 leaf area; however, photosynthetically very active trees like Populus × euramericana had higher and the gymnosperm Pinus pinea had lower O3 deposition rates than the average. As similar plant traits are likely affecting fine particulate matter (PM2.5) and O3 deposition rates, available rankings addressing the effectiveness of (deciduous) tree species for PM2.5 deposition (e.g., Yang et al., 2015) can probably be used as a guideline for ozone deposition capacity.
BVOC Emission Rates
The production and emission of BVOCs can serve as a protective mechanism of plants against O3-induced tissue damage, for increasing thermotolerance, communicating with neighboring and interfering individuals and sometimes possibly just to get rid of surplus energy by releasing byproducts of physiological processes (Niinemets et al., 2013). The profiles of emitted BVOCs vary considerably among tree species (Courtois et al., 2009; Niinemets and Monson, 2013) and even genotypes (Blanch et al., 2012). Figure 2 illustrates the available data on standard emission potentials of isoprene, monoterpene, sesquiterpenes and OVOCs of frequent tree species in European cities under non-stress conditions. Isoprene is known as the major BVOC emitted by trees globally (Figure 2) and also the one that has the highest potential contributing to tropospheric O3 formation (Kamens et al., 1982; Loreto and Velikova, 2001; Xie et al., 2008). Among the most common city trees, Populus nigra, P. tremula, Quercus robur and Q. pubescens were identified as high isoprene emitters. Monoterpenes are emitted by conifers and several broad-leaved trees including F. sylvatica, which is dominant in forests of central Europe (Kramer et al., 2010), and thus may be important pre-cursors for particles and O3 especially in rural-suburban transition zones. Common urban trees such as Acer platanoides and Ulmus minor are considered low emitters (Singh et al., 2007; Karl et al., 2009) while some as for example Fraxinus excelsior, and Tilia platyphyllos are generally assumed to be non-monoterpene and -isoprene emitters. Sesquiterpenes are mainly emitted in low quantities and have a similar O3 formation potential than monoterpenes, although less than isoprene, and have thus a minor influence on ozone formation (Holopainen and Blande, 2013). Oxygenated VOCs (OVOCs) have different but generally minor ozone forming potentials. They are typically emitted as signaling compounds or as response to any kind of stressful conditions (Seco et al., 2007). As plant ontogeny can influence i.a. foliar defense metabolites (Goodger et al., 2013), it is likely that BVOC emission rates of tree species are changing along ontogenetic trajectories.
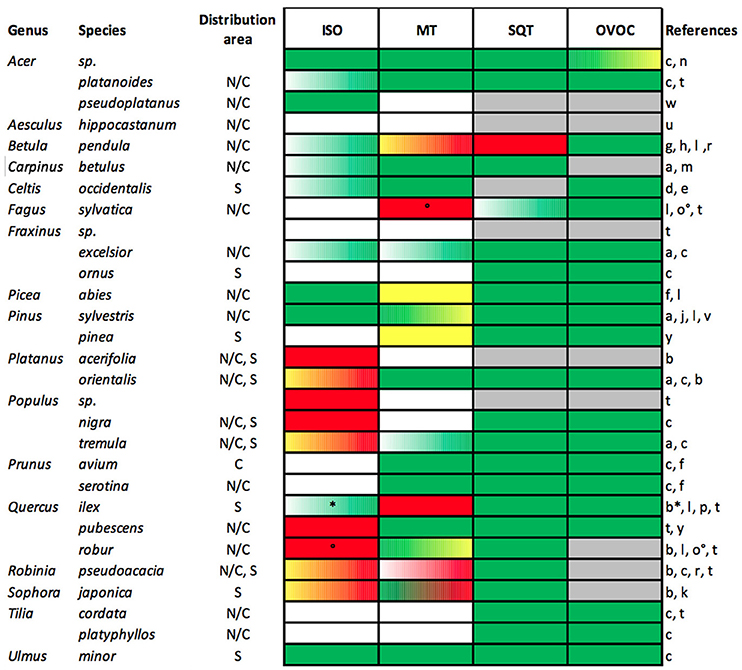
Figure 2. Standard emission potentials (μg g DW−1 h−1; at 30°C leaf temperature and 1,000 μmol m−2 s−1 PPFD) of isoprene (ISO), monoterpene (MT), sesquiterpenes (SQT), and oxygenated VOC (OVOC) of frequent tree species in urban areas of northern/central (N/C) and southern (S) Europe. Potential emission rates are grouped in no (white), low (green), medium (yellow), and high (red) emission classes. ISO: low < 10, high > 30.1; MT and OVOC: low < 2, high > 5.1; SQT: low < 0.5, high > 1.1; with medium categories with values in-between. A color gradient indicates emission rates crossing the defined classes; gray indicates: “no data available.” References: a (Aydin et al., 2014), b (Benjamin and Winer, 1998) *high ISO, c (EMEP/CORINAIR, 1999), d (Curtis et al., 2014), e (Geron et al., 1994), f (Grote et al., 2016), g (Hakola et al., 1998), h (Hakola et al., 2001), j (Hakola et al., 2006), k (Heinrich, 2007), l (Karl et al., 2009), m (König et al., 1995), n (Lamb et al., 1987), o (Meeningen et al., 2016) °low ISO, p (Owen et al., 1997), q (Paoletti et al., 2011), r (Préndez et al., 2013), s (Singh et al., 2007), t (Steinbrecher et al., 2009), u (Streiling and Matzarakis, 2003), v (Tarvainen et al., 2005), w (Tiwary et al., 2013), y (Veldt, 1989).
Other compounds are also occasionally reported as constitutive emissions from plants. Regarding trees in urban area, alkanes, aldehydes, alkenes, aromatic compounds, esters, and ketones have been determined to originate from Ginkgo biloba (Li et al., 2011). Their impact on air chemistry is generally assumed to be small since anthropogenic emissions are dominating the concentration of these compounds in urban areas by far (e.g., Costa and Baldasano, 1996; Cheng et al., 2010). However, alkanes and alkenes have been found as major emissions over many forests (Kourtchev et al., 2008; Halliday et al., 2015; Rhew et al., 2017); thus, at least the more reactive alkenes are assumed to play a significant role at larger to global levels (Goldstein et al., 1996; Rhew et al., 2017).
Looking at the community level, some general patterns in BVOC emissions can be identified among tree functional types (Dani et al., 2014). For example, trees featuring a high photosynthetic capacity are often also high monoterpene emitters. Deciduous trees are in general higher isoprene emitters and low monoterpene emitters whereas evergreen trees are low isoprene emitters and can be both low and high emitters of monoterpene (Dani et al., 2014). European forest biomes are generally thought to emit higher levels of monoterpenes relative to isoprene, compared to the continental US. For instance, Quercus sp. in the Mediterranean area are often evergreen and primarily monoterpene emitters in contrast to North-American Quercus sp., with relevant changes concerning the interaction of emitted isoprenoids with the atmosphere and air quality (Fares et al., 2013). As under normalized conditions the BVOC contribution of an individual plant is proportional to its BVOC emission rate (Kesselmeier and Staudt, 1999; Niinemets and Monson, 2013) and the size of its canopy (as discussed above), abundant and/or large species with high emission rates tend to dominate the BVOC emission inventory in a given landscape. Furthermore, plant phenology and ontogeny influence emission rates (Goodger et al., 2013; Jardine et al., 2016). However, as “standard conditions” rarely occur in urban settings, ozone removal capacities as well as BVOC emission rates are largely modified by stress as outlined below.
Stressors Modifying Species-Specific Effects via Tree Physiological Status
As urban plants are increasingly exposed to numerous stressors (Calfapietra et al., 2015), the vitality of street trees declined drastically over the last 3–4 decades (Bradshaw et al., 1995). While stress levels can vary considerable between urban centers and rural areas (see section The Rural-Urban Gradient in Context of Tree-Ozone Interactions), knowledge of the environmental physiology of trees in urban settings is key in order to understand the responses of trees to different stresses including feedback mechanisms on specific ecosystem services and/or disservices (Calfapietra et al., 2015).
Meteorological Factors and Air Composition
Higher temperatures and light intensities are often accompanied by increased formation of secondary air pollutants in the atmosphere including ozone (Chameides et al., 1988). Also, BVOC emissions are strongly positively correlated to temperature (Guenther et al., 1991; Niinemets and Monson, 2013; Guidolotti et al., 2019) as plants can use, for example, isoprene to stabilize the cell membrane during high temperatures (Singsaas et al., 1997). Behnke et al. (2013) showed that Populus × canescens leaves in which isoprene production was genetically removed were less heat resistant. However, the temperature sensitivity of BVOC emissions are highly species-specific, related to the emission traits that distinguish trees in isoprene or monoterpene emitters and, for the latter, in trees without and with isoprenoids pools (Niinemets et al., 2010; Grote et al., 2013). The direct effect of temperature on O3 deposition is believed to be small; however, as high temperature increases evapotranspiration, and thus potentially drought stress levels, the indirect decrease in O3 deposition by stomatal closure may be large (Morani et al., 2014). Another relevant but highly variable meteorological factor is wind. Ventilation of canopies increases evapotranspiration and cools down leaves (Drake et al., 1970). Cooler leaf temperatures lead to lower isoprene emissions in Populus sp. and Quercus sp. (Potosnak et al., 2014a). Further, strong wind gusts can damage trees—leading to the release of BVOCs (Loreto et al., 2006). For example, a burst of monoterpene emission has been measured at high wind speeds in Eucalypts, whose emissions are otherwise extremely low (Guidolotti et al., 2019). In contrast, higher wind speeds may render leaves within closed canopies more available for ozone deposition.
Urban areas are often characterized by higher concentrations of CO2 and air pollutants (O'Driscoll et al., 2018). Estimating how these conditions will affect BVOC emissions from urban trees is, however, not easy as a number of factors are interrelated, including the chemical composition of the compound(s), the exposure, the concentration and the plant species. However, there is for example a consensus that an increase in CO2 concentration will induce a reduction of isoprenoid emissions at least at leaf level (Rosenstiel et al., 2003; Calfapietra et al., 2008). On the other hand, an increase of O3 concentration can have opposite effects depending on the length and level of exposure—with a general stimulation in the cases of acute exposures and a general inhibition under chronic exposures (Calfapietra et al., 2013). For example, Carriero et al. (2016) states an increase of monoterpene emissions under increased ozone concentrations by B. pendula whereas Vuorinen et al. (2005) observed no emission changes. Similar, OVOC emissions by B. pendula under elevated ozone exposure were found to decrease (Hartikainen et al., 2012) or increase (Carriero et al., 2016), and isoprene emissions under O3 stress decreased in P. tremuloides (Calfapietra et al., 2008) but did not change for P. tremula (Hartikainen et al., 2009) and P. tremula × tremuloides (Blande et al., 2007). Despite the uncertainties, however, there are numerous publications that indicate a considerable induction of monoterpenes and various OVOCs by O3 while the effect on isoprene is mostly negative—either because the biosynthesis pathway is very sensitive to ozone damages or more likely because precursors are directed toward protecting agents (Grote, 2019). Ozone deposition is normally negatively influenced by increased CO2 and O3 levels as both decrease stomatal conductivity and thereby stomatal O3 deposition (Medlyn et al., 2001; Wittig et al., 2007). Globally, the increase of O3 since the industrialization is estimated to have decreased stomatal conductance by 13% (Wittig et al., 2007)—with likely higher values in urban areas.
Plant Water Status
Urban vegetation can experience exceptional stresses from water scarcity to flooding, caused by extreme heat, drought, and rainfall events (Wissmar et al., 2004; Collier, 2006; Livesley et al., 2016).
With increasing drought stress the stomata close and the stomatal depletion of ozone decreases (Wissmar et al., 2004; Livesley et al., 2016), increasing tropospheric ozone levels by up to 10% (Anav et al., 2018). However, trees have evolved different strategies to deal with low water availability. Isohydric species maintain a constant midday leaf water potential by closing their stomata earlier, while anisohydric species keep their stomata open longer and allow the leaf water potential to drop (Sade et al., 2012). By keeping the stomata open during moderate drought, anisohydric tree species such as Quercus sp. and Fagus sp. can maintain stomatal ozone deposition on hot summer days when ozone levels may become high (Grote et al., 2016). In contrast, isohydric tree species such as Acer sp. and Betula sp. are more likely to survive severe droughts and remain thus available for O3 deposition under post-stress conditions (Sade et al., 2012; Klein, 2014). Thus, in selecting tree species for maximizing urban ozone deposition there is a tradeoff between high stomatal deposition during moderate drought and survival during severe drought events.
BVOC emission rates under drought have been found increasing (Funk et al., 2004; Potosnak et al., 2014b), decreasing (Fortunati et al., 2008; Tiiva et al., 2009) or unaffected (Tingey et al., 1981; Pegoraro et al., 2006). Closing the stomata results in increasing leaf temperature as transpirational cooling decreases; this induces isoprene production in the short-term to increase the thermo-tolerance of tissue (Singsaas et al., 1997). Furthermore, when stomata close the supply of CO2 decreases, whereas the photosynthetic electron transport rate (ETR) remains high. The emission of at least isoprene seems to be positively correlated to the ratio of ETR to net carbon assimilation rate [NAR; (Dani et al., 2015)]. The carbon used for isoprene production is believed to come from stored carbon and isoprene production can thus be maintained even though CO2 assimilation decreases (de Souza et al., 2018). In contrast, closed stomata can lead also to decreased emissions of at least some BVOCs, which can less easily diffuse into the atmosphere (Saunier et al., 2017). The variable responses reported previously emphasize that BVOC emissions under drought are highly dependent on tree species (sensitivity) as well as the intensity and temporal extend of the stress events (Dani et al., 2015). Different effects are thus explained by species sensitivity as well as drought intensity (Niinemets, 2010; Klein, 2014; Saunier et al., 2018). For example, the same species can possess an increase of isoprene emissions under mild drought while severe drought decreases emissions (Brilli et al., 2007; Genard-Zielinski et al., 2014; Dani et al., 2015). Similar behavior has been observed for monoterpene emissions, with moderate stress suppressing photosynthesis but not BVOC emissions (Funk et al., 2004; Wu et al., 2015). Less is known on the effect of drought on highly volatile BVOC emissions such as methanol, formaldehyde or acetaldehyde (OVOC) or green leaf volatiles [GLV; (Vitale et al., 2007; Saunier et al., 2017)]. On the long term, drought stress is likely to decrease leaf biomass, which has a negative effect on both ozone deposition as well as BVOC emissions.
In snow-prone urban areas, de-icing salt (NaCl) ends up highly concentrated in street tree pits. De-icing salt has been found to have a negative effect on tree health (Czerniawska-Kusza et al., 2004; Munck et al., 2010; Rose and Webber, 2011; Ordóñez-Barona et al., 2018), by inducing water stress (“physiological drought”), with the effects describe above, but also malnutrition and accumulation of excess ions to potentially toxic levels (Ahmad et al., 2012). This is often decreasing leaf biomass & vitality (Shannon et al., 1999) and thereby the ozone deposition capacity. While emissions of isoprene and OVOC were found rather unaffected by mild salt stress (Loreto and Delfine, 2000; Teuber et al., 2008), it is currently unknown if the same holds true for severe salt stress.
Finally, flooding can also reduce stomatal conductance (Liao and Lin, 2001), and has consequently a negative effect on stomatal ozone deposition as described above (Chaves et al., 2003). Moreover, it has been observed that flooding can also increase methanol as well as GLV, but decrease isoprene emissions (Copolovici and Niinemets, 2010; Bourtsoukidis et al., 2013).
Nutrition Status
The urban ecosystem is highly heterogeneous in regard to nutrient availability; for example, some street trees have access only to the limited soil volume underneath the planting pit whereas others access nearby gardens and sewer pipes (Kopinga and Van den Burg, 1995; Day et al., 2010). Nutrients frequently limiting the growth of urban trees are potassium (K) and nitrogen (N), however, deficiencies of magnesium (Mg) and phosphorus (P) as well as micronutrients are common (Kopinga and Van den Burg, 1995; Sieghardt et al., 2005).
Deficient nutrition results in decreased growth and increased sensitivity to pests—leading to relatively reduced leaf area, affecting O3 deposition negatively (Carriero et al., 2016; Hu et al., 2018). On a single leaf scale, N deficiency has been shown to cause a decrease in BVOC production due to a lower photosynthetic efficiency and thus less available carbon for secondary metabolites (Lerdau et al., 1995; Ormeño et al., 2008). Although direct studies are missing, it can be hypothesized that K and Mg deficiency, key elements of the photosynthetic system, cause similar effects (Lambers et al., 1998). P deficiency could eventually also lead to a decrease in energy-demanding BVOC production due to a shortage of P-rich ATP and NADPH (Ormeño and Fernandez, 2012). In contrast, increased BVOC emissions under warm temperatures, destabilizing the cell membrane and releasing GLVs, may be spurred under P deficiency (Siwko et al., 2007; Ormeño and Fernandez, 2012). Only recently, nutrient deficiency has also found leading to a shift of the emitted BVOC composition; for example, from terpenoids toward GLV in nitrogen-limited Betula sp. trees (Carriero et al., 2016). While it thus becomes increasingly clear that a shift in BVOC emissions via nutrient deficiency affects the formation and degradation of O3 in the atmosphere, the contradictory results, i.e., either a positive or negative feedback of BVOC emissions due to malnutrition, may relate to reactions triggered on different timescales. Therefore, phenology and long-term allocation processes must be considered, combining the morphoanatomy of leaves with cell biochemistry and physiology (Lerdau et al., 1995, 1997; Ormeño and Fernandez, 2012).
Herbivores and Pathogens
Physical injuries by herbivores and pathogens frequently lead to increased GLV and monoterpene emissions (Gatehouse, 2002; Niinemets et al., 2013; Scala et al., 2013). These induced volatiles are emitted rapidly after the stress occurred (Ameye et al., 2018) and can account for 9 to 21-fold of the total emissions of damaged tissue (Ghimire et al., 2017). Fungal and pathogen infections of leaves also lead to increased emissions of GLV and monoterpene but often a decrease in isoprene emissions (Kesselmeier and Staudt, 1999; Steindel et al., 2005; Arimura et al., 2008; Jansen et al., 2009). Thus, even though isoprene decreases, the high monoterpene emission caused by herbivory and pathogens can, under high NOx levels, contribute to ozone formation (Holopainen and Blande, 2013). The decrease in leaf biomass due to herbivory and pests decreases the ozone deposition on the leaves causing increasing ozone concentrations in the air (Kesselmeier and Staudt, 1999).
The Rural-urban Gradient in Context of Tree-ozone Interactions
The commonly used terms “urban” and “rural” relate to a range of environmental conditions such as temperature, pollutant and CO2 levels, and land cover types including the extension of sealed surfaces and housing densities, as well as changes in species composition and abundance of vegetation. In general, the availability of growing spaces tends to decrease along the rural-urban gradient (Zipperer et al., 1997). The differences between rural and urban are furthermore fueled by a gradient of human activities, such as emissions from traffic and industries but also vegetation management, that all affect the ozone forming potential and depletion, and thereby the (local) O3 concentration.
Less abundant vegetation does not necessarily mean a low deposition capacity or BVOC emissions since both are strongly related to species, physiological state and stress severity as outlined above. It is therefore interesting that in many urban areas trees are predominantly deciduous (Grote et al., 2016) and in case of Quercus sp., Salix sp., and Populus sp. (and to some lesser degree Platanus sp. and Robinia pseudoacacia) belong to the topmost isoprene emitters. These high emitters contribute between 13% [Lancaster, UK; (Wolyniak and Elmendorf, 2011)] and 30% [Hamburg, Germany; (BUE, 2019)] of urban trees in Europe, and seem to have even higher shares in Asia [e.g., 30%, Beijing, China; (Ghirardo et al., 2016)] while US cities seem to have a bit less high emitters [11.4% in Chicago; (Nowak et al., 2010)]. In contrast, suburban and rural forests in northern and temperate regions are commonly formed by evergreen forests (Gallaun et al., 2010), which emit primarily monoterpenes that are far less effective regarding O3 formation. Similar holds true for Mediterranean rural areas that are dominated by Pinus sp. and evergreen Quercus sp. (Sheffer, 2012).
Besides differences in species composition, trees' physiological status differs in urban compared to rural conditions because of changes in environmental conditions. Most prominently, urban centers are characterized by higher air temperatures as compared to suburbs which is the so called urban heat island (UHI) effect (Ajaaj et al., 2018; Saaroni et al., 2018). Higher temperatures in urban centers trigger higher BVOC emissions leading to higher ozone concentrations, an effect that is expected to increase with global warming (IPCC, 2014; Li et al., 2015). In contrast, higher CO2 concentrations in urban areas do not only encourage growth but also tend to decrease BVOC emission capacity, particularly regarding isoprene (Gratani and Varone, 2014). The third effect on physiology that is not necessarily stress-related (if considering stress as damage that is not immediately reversible) is related to air humidity and soil moisture availability. Both are commonly lower in urban centers compared to urban woodlands and rural areas (Devakumar et al., 1999; Yang et al., 2017). Restricted water supply will decrease stomatal conductance and thereby stomatal ozone depletion and also modify BVOC emission rates as discussed above. Finally, urban trees, especially road-side trees, are more frequently stressed by drought (Clark and Kjelgren, 1990; Fahey et al., 2013; Bialecki et al., 2018), salt (Equiza et al., 2017), herbivores (Dale and Frank, 2014), and air pollution (Samson et al., 2017a). Stress-induced emissions are different from constitutively driven emissions in both intensity and composition. They are tightly linked to the intensity and duration of stress and thus depend for example on short-time ozone peaks. They also might eventually decrease if plants are substantially damaged although the necessary degree to reach this point is difficult to define. Indeed, considerable amounts of stress-related emission compounds have been found in metropolitan regions (Ghirardo et al., 2016).
In general, large uncertainty exists as to the effects of multiple factors, occurring in urban settings, on BVOC emissions (Holopainen and Gershenzon, 2010). They depend on species abundance and distribution, and furthermore interact with plant ecophysiological responses, such as stomatal regulation, and in particular stress responses, which are largely unknown for many urban species/ecotypes (Sjöman and Busse Nielsen, 2010). Studies show that the quota of ozone produced by BVOCs are up to 12% on average of the daily maximum 1-h O3 concentration in Berlin but up to 60% on very warm days (Churkina et al., 2017), 50–75% during summer in Italy and Spain (Duane et al., 2002), and by a factor of 50 in Las Vegas, NV (Papiez et al., 2009). With increasing temperatures in the future, the heating effect is likely to override the suggested suppressive effect of higher CO2 concentrations (Tingey et al., 1991; Lahr et al., 2015) and increase BVOC emissions in urban areas (Norton et al., 2015). It is therefore reasonable to speculate that combined effects will affect the emission by individual trees much more severely in the highly anthropogenic environment of city centers and suburbs than in rural areas. This assumption is supported by the fact that high temperatures and the occurrence of drought and ozone stress commonly occur in combination, which not only directly increases emissions but also decreases trees' resistance to pathogens and herbivores which then can lead to induction of further emissions (Holopainen, 2004; Loreto and Schnitzler, 2010). Indeed, several studies show that higher temperatures in urban areas in combination with drought stress increases insect herbivory (Meineke et al., 2013; Dale and Frank, 2014, 2017).
To calculate the effect of the diverging BVOC emission along the urban-rural gradient on the ozone concentration, it has to be set in relation to the NOx concentration. In city centers and suburbs, the emissions of NOx and AVOCs are high compared to rural areas. Toward the rural areas, NOx and AVOC emissions decrease while BVOC emissions might increase due to an increasing leaf area or decrease depending on conditions discussed above. The emissions of AVOCs in Europe have dropped dramatically in the last 20 years (Stemmler et al., 2005; Dollard et al., 2007). Thus, on warm summer days the emission of BVOCs may be dominant relative to the contribution of AVOCs to O3 formation (Wagner and Kuttler, 2014). In many other urban areas of the world (e.g., China), however, AVOCs are still the major driver of O3 formation (Ran et al., 2011; Wang et al., 2017). With high NOx concentrations within city boundaries, O3 formation is usually small due to low VOC:NOx ratios (Atkinson, 2000). With increasing distance from the urban center, however, NOx concentrations decrease relative to BVOCs—resulting in an increase of the VOC:NOx ratio and thereby a higher potential of O3 formation in the suburbs and rural areas. An O3 isopleth plot (Figure 3, dashed line) illustrates net O3 production P(O3) as a function of BVOC and NO2 reactivity giving data from Innsbruck, Austria as an example. A hypothetical trajectory from NOx-rich urban conditions to BVOC-rich rural conditions is depicted along the red arrows—as NOx is diluted, O3 production increases until a ridge after which it decreases due to becoming NOx-limited. This non-linear relationship of tropospheric O3 production has important ramifications for air quality management across urban gradients, because strategies of O3 mitigation will depend on the (local) interplay between NOx and NMVOC (Sillman and He, 2002).
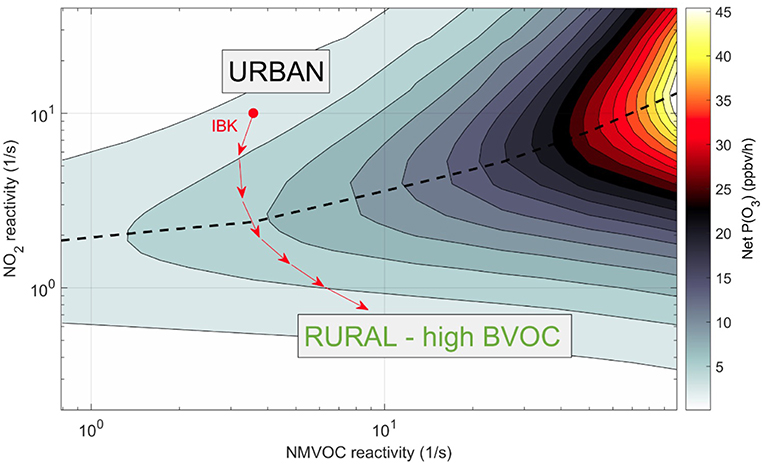
Figure 3. Ozone isopleth showing urban (black) and rural (green) conditions of the relationship of NMVOC, NOx, and O3. Calculations are based on a model-measurement analysis for Innsbruck (Karl et al., 2017) based on the full chemistry MCM from Leeds (http://mcm.leeds.ac.uk/MCM/). Rural area conditions characterized by high BVOC emissions represent a typical range observed in the Southeast USA (Kaser et al., 2015). The trajectory to the rural end point was estimated based on a typical scenario (Ehlers et al., 2016). The MCM was implemented in Matlab (C) based on Wolfe and Thornton (2011). The red inset denotes a hypothetical trajectory from NOx-rich urban conditions to BVOC-rich rural conditions in Innsbruck, Austria.
There is a strong societal demand on improving the air quality and comfort in cities. The focus is put on reducing gaseous and particulate pollution by reducing emissions from traffic, particularly of NOx. In addition, mitigation strategies include measures to increase deposition of pollutants, including NOx and ozone, which is preferably done by increasing leaf area (Taha et al., 2016; Tong et al., 2016). However, it should be noted that achieving reduction of NOx by increasing deposition on leaves but simultaneously increasing BVOC emissions by selecting high emitting species can potentially increase the ozone forming potential particularly in the highly populated urban centers (Figure 4).
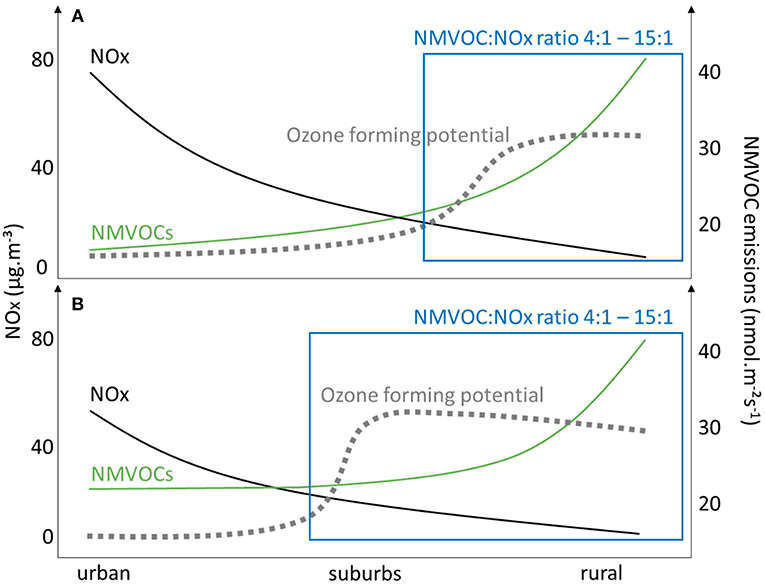
Figure 4. A schematic display of current NOx-VOC-O3 relations (approximated from literature values) (A), and a possible future scenario after decreasing NOx pollution (black solid line) and increased non-methane hydrocarbons (NMVOCs, green solid line) in cities due to greening (B). A left shift of the ozone forming potential curve (gray dotted line) indicates higher ozone pollution in suburbs, since the NMVOC:NOx ratio reaches optimal values to form ozone (blue box).
Considering future climate change scenarios with increasing temperatures, we hypothesize that ozone formation would expand toward the urban centers as with BVOC emissions increase, due to increasing temperatures, being the major driver. This effect would be enhanced if (exotic) species that are more tolerant against heat and drought but also have high BVOC emission potentials would be newly introduced in urban areas (Valkama et al., 2007; Himanen et al., 2009; Youngsteadt et al., 2015). Reduced emissions due to higher CO2 concentrations and more frequent drought stress are not likely to reverse this trend, particularly since trees are often irrigated in arid and semiarid regions or during heat wave events in order to sustain their cooling function. However, estimating the net effect of urban trees on ozone concentrations, deposition would need to be taken into account as well, which would be enhanced by more trees. Ozone concentrations furthermore depend on temporal and spatial variations of biogenic and anthropogenic emissions as well as wind speed, and thus require elaborated air chemistry transport models. Approaches have been made to couple physiological models to such models of air chemistry, but the multitude of direct and indirect effects described here have not been considered (Baumgardner et al., 2012; Cabaraban et al., 2013).
Outlook
Policies are strongly directed toward an improvement of air quality and an increase of resilience against climate change impacts in cities. Decisions need to be based on how these goals can be reached most efficiently while maintaining transport and other energy consuming functions. Obvious solutions are a reduction of emissions by introducing clean energy and transport concepts and providing green infrastructure for cooling and shading. Since a technological reduction of anthropogenic emissions will not immediately take place, an increase of green infrastructure is also supposed to enhance deposition and thus reduce air pollution. This is principally a valid and reasonable approach. However, the efficiency of vegetated urban area to fulfill these ecosystem services depends on various boundary conditions, cannot easily be evaluated, and potential ecosystem disservices, among which is the facilitation of ozone production via BVOC emissions, have to be considered. A general vote for a higher share of green infrastructure is thus difficult to implement and can eventually deliver results that might even be contradictory to expectations. It is thus of utmost importance to consider species properties, stress sensitivity, and physiological responses that can be expected under future environmental conditions. Achieving the goal to abate or at least not to increase ozone concentrations in cities, newly planted tree species would need the following requirements: (i) emit low or no BVOCs under current and future environmental conditions, (ii) have a large resistance to drought and biotic stresses, (iii) maximize biomass in terms of leaf area, and (iv) have a high photosynthetic capacity and therefore high stomatal conductance (and thus being high ozone sinks) (Nowak et al., 2018). This task is, however, complicated by the fact that the new plants will have a distinct effect on future urban environmental conditions.
The necessary efforts to enable such analyses will be substantial since our species-specific knowledge about stomatal responses as well as BVOC emission under various stress conditions is limited. The first requirement is therefore to refine our understanding of urban vegetation responses to local environmental conditions. A key knowledge gap is the response of BVOCs emissions to stressful conditions, which often lead to large increases that can potentially affect air quality (Grote et al., 2019). However, general responses to extreme heat and dry conditions that may trigger the death of the plant should also be evaluated in particular for urban tree species that might get more important in the future. Secondly, urban air quality needs to be investigated with integrated analyses considering climate, anthropogenic emissions, city structure as well as distribution and responses of vegetation, i.e., street and park trees. Current approaches are still suffering on coarse resolutions and missing climate-vegetation feedbacks but new developments are encouraging (Maronga et al., 2019). Finally, it should be considered that inventories of existing tree distributions are essential to evaluate any spatially explicit modeling approach. This also includes soil conditions and management that affects canopy dimension and physiology (e.g., pruning and irrigation). Only if this information is available, scenario analyses with varying climate, pollutant emissions, as well as vegetation abundance, composition and distribution can successfully be carried out. Thus, integrating ecosystem services and disservices into environmental quality strategies will therefore enable the identification of green infrastructure types, suitable species (combinations), as well as distribution and density of individuals in order to increase people's overall quality of life in a particular city at reasonable costs.
Author Contributions
AF, HS, and BR contributed conception and design of the review. AF and TK composed the figures. AF, HS, TK, AS, CC, SF, and BR wrote the first draft of the manuscript. AF, HS, TK, SF, RG, and BR wrote sections of the manuscript and added to the tables. All authors contributed to manuscript revision, read, and approved the submitted version.
Funding
AF was funded by the project Urban trees and air pollution: Effect of drought and salt stress on the production of VOC and absorption of ozone by different city trees (UOzone) by the Vienna Science and Technology Fund (WWTF, project number: ESR17-027).
Conflict of Interest Statement
The authors declare that the research was conducted in the absence of any commercial or financial relationships that could be construed as a potential conflict of interest.
Acknowledgments
The authors wish to thank the participants and organizers of the International Conference on Ozone and Plant Ecosystems, Florence, Italy (May 21–25, 2018), for impulses and intensive discussions. Two reviewers and the editor gave important inputs to revise an earlier version.
References
Ahmad, P., Azooz, M. M., and Prasad, M. N. V. (2012). Ecophysiology and Responses of Plants Under Salt Stress. New York, NY: Springer.
Ajaaj, A. A., Mishra, A. K., and Khan, A. A. (2018). Urban and peri-urban precipitation and air temperature trends in mega cities of the world using multiple trend analysis methods. Theor. Appl. Climatol. 132, 403–418. doi: 10.1007/s00704-017-2096-7
Altimir, N., Kolari, P., Tuovinen, J.-P., Vesala, T., Bäck, J., Suni, T., et al. (2006). Foliage surface ozone deposition: a role for surface moisture? Biogeosciences 2, 1739–1793. doi: 10.5194/bgd-2-1739-2005
Ameye, M., Allmann, S., Verwaeren, J., Smagghe, G., Haesaert, G., Schuurink, R. C., et al. (2018). Green leaf volatile production by plants: a meta-analysis. New Phytol. 220, 666–683. doi: 10.1111/nph.14671
Anav, A., Proietti, C., Menut, L., Carnicelli, S., Marco, A. D., and Paoletti, E. (2018). Sensitivity of stomatal conductance to soil moisture: implications for tropospheric ozone. Atmos. Chem. Phys. 18, 5747–5763. doi: 10.5194/acp-18-5747-2018
Arimura, G., Köpke, S., Kunert, M., Volpe, V., David, A., Brand, P., et al. (2008). Effects of feeding Spodoptera littoralis on lima bean leaves: IV. Diurnal and nocturnal damage differentially initiate plant volatile emission. Plant Physiol. 146, 965–973. doi: 10.1104/pp.107.111088
Atkinson, R. (2000). Atmospheric chemistry of VOCs and NOx. Atmos. Environ. 34, 2063–2101. doi: 10.1016/S1352-2310(99)00460-4
Atkinson, R. (2007). Gas-phase tropospheric chemistry of organic compounds: a review. Atmos. Environ. 41, 200–240. doi: 10.1016/j.atmosenv.2007.10.068
Atkinson, R., and Arey, J. (2003). Gas-phase tropospheric chemistry of biogenic volatile organic compounds: a review. Atmos. Environ. 37, 197–219. doi: 10.1016/S1352-2310(03)00391-1
Aydin, Y. M., Yaman, B., Koca, H., Dasdemir, O., Kara, M., Altiok, H., et al. (2014). Biogenic volatile organic compound (BVOC) emissions from forested areas in Turkey: Determination of specific emission rates for thirty-one tree species. Sci. Total Environ. 490, 239–253. doi: 10.1016/j.scitotenv.2014.04.132
Barnes, J., Davison, A., and Booth, T. (1988). Ozone accelerates structural degradation of epicuticular wax on Norway spruce needles. New Phytol. 110, 309–318. doi: 10.1111/j.1469-8137.1988.tb00267.x
Baumgardner, D., Varela, S., Escobedo, F. J., Chacalo, A., and Ochoa, C. (2012). The role of a peri-urban forest on air quality improvement in the Mexico City megalopolis. Environ. Pollut. 163, 174–183. doi: 10.1016/j.envpol.2011.12.016
Behnke, K., Ghirardo, A., Janz, D., Kanawati, B., Esperschütz, J., Zimmer, I., et al. (2013). Isoprene function in two contrasting poplars under salt and sunflecks. Tree Physiol. 33, 562–578. doi: 10.1093/treephys/tpt018
Benjamin, M. T., and Winer, A. M. (1998). Estimating the ozone-forming potential of urban trees and shrubs. Atmos. Environ. 32, 53–68. doi: 10.1016/S1352-2310(97)00176-3
Bialecki, M. B., Fahey, R. T., and Scharenbroch, B. (2018). Variation in urban forest productivity and response to extreme drought across a large metropolitan region. Urban Ecosyst. 21, 157–169. doi: 10.1007/s11252-017-0692-z
Blanch, J. S., Sampedro, L., Llusià, J., Moreira, X., Zas, R., and Peñuelas, J. (2012). Effects of phosphorus availability and genetic variation of leaf terpene content and emission rate in Pinus pinaster seedlings susceptible and resistant to the pine weevil, Hylobius abietis. Plant Biol. 14, 66–72. doi: 10.1111/j.1438-8677.2011.00492.x
Blande, J. D., Tiiva, P., Oksanen, E., and Holopainen, J. K. (2007). Emission of herbivore-induced volatile terpenoids from two hybrid aspen (Populus tremula × tremuloides) clones under ambient and elevated ozone concentrations in the field. Glob. Change Biol. 13, 2538–2550. doi: 10.1111/j.1365-2486.2007.01453.x
Bonn, B., von Schneidemesser, E., Butler, T., Churkina, G., Ehlers, C., Grote, R., et al. (2018). Impact of vegetative emissions on urban ozone and biogenic secondary organic aerosol: Box model study for Berlin, Germany. J. Clean. Prod. 176, 827–841. doi: 10.1016/j.jclepro.2017.12.164
Bourtsoukidis, E., Kawaletz, H., Radacki, D., Schütz, S., Hakola, H., Hellén, H., et al. (2013). Impact of Flooding and Drought Conditions on the Emission of Volatile Organic Compounds of Quercus robur and Prunus serotina. Berlin: Springer.
Bradshaw, A., Hunt, B., and Walmsley, T. (1995). Trees in the Urban Landscape: Principles and Practice. London; New York, NY: E & FN Spon.
Brasseur, G., Orlando, J. J., and Tyndall, G. S. (1999). Atmospheric Chemistry and Global Change. Oxford: Oxford University Press.
Brilli, F., Barta, C., Fortunati, A., Lerdau, M., Loreto, F., and Centritto, M. (2007). Response of isoprene emission and carbon metabolism to drought in white poplar (Populus alba) saplings. New Phytol. 175, 244–254. doi: 10.1111/j.1469-8137.2007.02094.x
Cabaraban, M. T., Kroll, C. N., Hirabayashi, S., and Nowak, D. J. (2013). Modeling of air pollutant removal by dry deposition to urban trees using a WRF/CMAQ/i-Tree Eco coupled system. Environ. Pollut. 176, 123–133. doi: 10.1016/j.envpol.2013.01.006
Calfapietra, C., Morani, A., Sgrigna, G., Di Giovanni, S., Muzzini, V., Pallozzi, E., et al. (2016). Removal of ozone by urban and peri-urban forests: evidence from laboratory, field, and modeling approaches. J. Environ. Qual. 45, 224–233. doi: 10.2134/jeq2015.01.0061
Calfapietra, C., Mugnozza, G. S., Karnosky, D. F., Loreto, F., and Sharkey, T. D. (2008). Isoprene emission rates under elevated CO2 and O3 in two field-grown aspen clones differing in their sensitivity to O3. New Phytol. 179, 55–61. doi: 10.1111/j.1469-8137.2008.02493.x
Calfapietra, C., Pallozzi, E., Lusini, I., and Velikova, V. (2013). “Modification of BVOC emissions by changes in atmospheric [CO2] and air pollution,” in Biology, Controls and Models of Tree Volatile Organic Compound Emissions. (Dotrecht: Springer, 253–284.
Calfapietra, C., Peñuelas, J., and Niinemets, U. (2015). Urban plant physiology: adaptation-mitigation strategies under permanent stress. Trends Plant Sci. 20, 72–75. doi: 10.1016/j.tplants.2014.11.001
Cape, J. N., Hamilton, R., and Heal, M. R. (2009). Reactive uptake of ozone at simulated leaf surfaces: Implications for ‘non-stomatal' ozone flux. Atmos. Environ. 43, 1116–1123. doi: 10.1016/j.atmosenv.2008.11.007
Carriero, G., Brunetti, C., Fares, S., Hayes, F., Hoshika, Y., Mills, G., et al. (2016). BVOC responses to realistic nitrogen fertilization and ozone exposure in silver birch. Environ. Pollut. 213, 988–995. doi: 10.1016/j.envpol.2015.12.047
Carter, W. P. L. (1994). Development of ozone reactivity scales for volatile organic compounds. J. Air Waste Manage. 44, 881–899. doi: 10.1080/1073161X.1994.10467290
Carter, W. P. L. (2000). Documentation of the SAPRC-99 Chemical Mechanism for VOC Reactivity Assessment. Sacramento, CA: California Air Resources Board.
Carter, W. P. L. (2010). Updated Maximum Incremental Reactivity Scale and Hydrocarbon Bin Reactivities for Regulatory Applications. Sacramento, CA: California Air Resources Board.
Chameides, W. L., Lindsay, R. W., Richardson, J., and Kiang, C. S. (1988). The role of biogenic hydrocarbons in urban photochemical smog: Atlanta as a case study. Science 241, 1473–1475. doi: 10.1126/science.3420404
Chaves, M. M., Maroco, J. P., and Pereira, J. S. (2003). Understanding plant responses to drought—from genes to the whole plant. Funct. Plant Biol. 30, 239–264. doi: 10.1071/FP02076
Cheng, H. R., Guo, H., Saunders, S. M., Lam, S. H. M., Jiang, F., Wang, X. M., et al. (2010). Assessing photochemical ozone formation in the Pearl River Delta with a photochemical trajectory model. Atmos. Environ. 44, 4199–4208. doi: 10.1016/j.atmosenv.2010.07.019
Churkina, G., Kuik, F., Bonn, B., Lauer, A., Grote, R., Tomiak, K., et al. (2017). Effect of VOC emissions from vegetation on air quality in Berlin during a heatwave. Environ. Sci. Technol. 51, 6120–6130. doi: 10.1021/acs.est.6b06514
Clark, J. R., and Kjelgren, R. (1990). Water as a limiting factor in the development of urban trees. J. Arboric. 16, 203–208.
Collier, C. G. (2006). The impact of urban areas on weather. Q. J. R. Meteor. Soc. 132, 1–25. doi: 10.1256/qj.05.199
Connop, S., Vandergert, P., Eisenberg, B., Collier, M. J., Nash, C., Clough, J., et al. (2016). Renaturing cities using a regionally-focused biodiversity-led multifunctional benefits approach to urban green infrastructure. Environ. Sci. Policy 62, 99–111. doi: 10.1016/j.envsci.2016.01.013
Copolovici, L., and Niinemets, U. (2010). Flooding induced emissions of volatile signalling compounds in three tree species with differing waterlogging tolerance. Plant Cell Environ. 33, 1582–1594. doi: 10.1111/j.1365-3040.2010.02166.x
Cornelis, J., and Hermy, M. (2004). Biodiversity relationships in urban and suburban parks in Flanders. Landsc. Urban Plan. 69, 385–401. doi: 10.1016/j.landurbplan.2003.10.038
Costa, M., and Baldasano, J. M. (1996). Development of a source emission model for atmospheric pollutants in the Barcelona area. Atmos. Environ. 30, 309–318. doi: 10.1016/1352-2310(95)00221-J
Courtois, E. A., Paine, C. E., Blandinieres, P. A., Stien, D., Bessiere, J. M., Houel, E., et al. (2009). Diversity of the volatile organic compounds emitted by 55 species of tropical trees: a survey in French Guiana. J. Chem. Ecol. 35:1349. doi: 10.1007/s10886-009-9718-1
Criegee, R. (1975). Mechanism of ozonolysis. Angew. Chem. Int. Edit. 14, 745–752. doi: 10.1002/anie.197507451
Curtis, A. J., Helmig, D., Baroch, C., Daly, R., and Davis, S. (2014). Biogenic volatile organic compound emissions from nine tree species used in an urban tree-planting program. Atmos. Environ. 95, 634–643. doi: 10.1016/j.atmosenv.2014.06.035
Czerniawska-Kusza, I., Kusza, G., and Duzynski, M. (2004). Effect of deicing salts on urban soils and health status of roadside trees in the Opole region. Environ. Toxicol. 19, 296–301. doi: 10.1002/tox.20037
Dale, A. G., and Frank, S. D. (2014). The effects of urban warming on herbivore abundance and street tree condition. PLoS ONE 9:e102996. doi: 10.1371/journal.pone.0102996
Dale, A. G., and Frank, S. D. (2017). Warming and drought combine to increase pest insect fitness on urban trees. PLoS ONE 12:e0173844. doi: 10.1371/journal.pone.0173844
Dani, K. G., Jamie, I. M., Prentice, I. C., and Atwell, B. J. (2014). Evolution of isoprene emission capacity in plants. Trends Plant Sci. 19, 439–446. doi: 10.1016/j.tplants.2014.01.009
Dani, K. S., Jamie, I. M., Prentice, I. C., and Atwell, B. J. (2015). Species-specific photorespiratory rate, drought tolerance and isoprene emission rate in plants. Plant Signal. Behav. 10:e990830. doi: 10.4161/15592324.2014.990830
Day, S. D., Wiseman, P. E., Dickinson, S. B., and Harris, J. R. (2010). Contemporary concepts of root system architecture of urban trees. Arboric Urban For 36, 149–159. doi: 10.1016/j.atmosenv.2010.02.045
de Souza, V. F., Niinemets, Ü., Rasulov, B., Vickers, C. E., Júnior, S. D., Araújo, W. L., et al. (2018). Alternative carbon sources for isoprene emission. Trends Plant Sci. 23, 1081–1101. doi: 10.1016/j.tplants.2018.09.012
Devakumar, A., Prakash, P. G., Sathik, M., and Jacob, J. (1999). Drought alters the canopy architecture and micro-climate of Hevea brasiliensis trees. Trees Struct. Funct. 13, 161–167. doi: 10.1007/PL00009747
Dollard, G., Dumitrean, P., Telling, S., Dixon, J., and Derwent, R. (2007). Observed trends in ambient concentrations of C2-C8 hydrocarbons in the United Kingdom over the period from 1993 to 2004. Atmos. Environ. 41, 2559–2569. doi: 10.1016/j.atmosenv.2006.11.020
Drake, B. G., Raschke, K., and Salisbury, F. B. (1970). Temperature and transpiration resistances of Xanthium leaves as affected by air temperature, humidity, and wind speed. Plant Physiol. 46, 324–330. doi: 10.1104/pp.46.2.324
Duane, M., Poma, B., Rembges, D., Astorga, C., and Larsen, B. (2002). Isoprene and its degradation products as strong ozone precursors in Insubria, Northern Italy. Atmos. Environ. 36, 3867–3879. doi: 10.1016/S1352-2310(02)00359-X
EEA (2018a). Air Quality in Europe−2018 Report, EEA Report No 12/2018, EEA Reports. European Environment Agency, Copenhagen.
EEA (2018b). Exceedance of Air Quality Standards in Urban Areas (CSI 004). 02 October 2018 ed. Copenhagen: European Environmental Agency.
Ehlers, C., Klemp, D., Rohrer, F., Mihelcic, D., Wegener, R., Kiendler-Scharr, A., et al. (2016). Twenty years of ambient observations of nitrogen oxides and specified hydrocarbons in air masses dominated by traffic emissions in Germany. Faraday Dis. 189, 407–437. doi: 10.1039/C5FD00180C
EMEP/CORINAIR (1999). Atmospheric Emission Inventory Guidebook, 2nd Edn. Copenhagen: European Environmental Agency.
Equiza, M., Calvo-Polanco, M., Cirelli, D., Senorans, J., Wartenbe, M., Saunders, C., et al. (2017). Long-term impact of road salt (NaCl) on soil and urban trees in Edmonton, Canada. Urban Forest. Urban Green. 21, 16–28. doi: 10.1016/j.ufug.2016.11.003
Escobedo, F. J., Kroeger, T., and Wagner, J. E. (2011). Urban forests and pollution mitigation: analyzing ecosystem services and disservices. Environ. Pollut. 159, 2078–2087. doi: 10.1016/j.envpol.2011.01.010
EU (2008). Directive 2008/50/EC of the European Parliament and of the Council of 21 May 2008 on Ambient Air Quality and Cleaner Air for Europe (OJ L 152, 11.6.2008). (Brussels: European Union, 1–44.
Fahey, R. T., Bialecki, M. B., and Carter, D. R. (2013). Tree growth and resilience to extreme drought across an urban land-use gradient. Arboric. Urban Forest. 39, 279–285. doi: 10.1007/s11104-012-1459-1
Fares, S., Schnitzhofer, R., Jiang, X., Guenther, A., Hansel, A., and Loreto, F. (2013). Observations of diurnal to weekly variations of monoterpene-dominated fluxes of volatile organic compounds from mediterranean forests: implications for regional modeling. Environ. Sci. Technol. 47, 11073–11082. doi: 10.1021/es4022156
Fittschen, C., Assaf, E., and Vereecken, L. (2017). Experimental and theoretical investigation of the reaction NO + OH + O2 → HO2 + NO2. J. Phys. Chem. A 121, 4652–4657. doi: 10.1021/acs.jpca.7b02499
Forkel, R., Stockwell, W. R., and Steinbrecher, R. (1999). Multilayer canopy/chemistry model to simulate the effect of in-canopy processes on the emission rates of biogenic VOCs. WIT Trans. Ecol. Environ. 28, 45–49.
Fortunati, A., Barta, C., Brilli, F., Centritto, M., Zimmer, I., Schnitzler, J. P., et al. (2008). Isoprene emission is not temperature-dependent during and after severe drought-stress: a physiological and biochemical analysis. Plant J. 55, 687–697. doi: 10.1111/j.1365-313X.2008.03538.x
Funk, J., Mak, J., and Lerdau, M. (2004). Stress-induced changes in carbon sources for isoprene production in Populus deltoides. Plant Cell Environ. 27, 747–755. doi: 10.1111/j.1365-3040.2004.01177.x
Gallaun, H., Zanchi, G., Nabuurs, G.-J., Hengeveld, G., Schardt, M., and Verkerk, P. J. (2010). EU-wide maps of growing stock and above-ground biomass in forests based on remote sensing and field measurements. Forest Ecol. Manag. 260, 252–261. doi: 10.1016/j.foreco.2009.10.011
Gatehouse, J. A. (2002). Plant resistance towards insect herbivores: a dynamic interaction. New Phytol. 156, 145–169. doi: 10.1046/j.1469-8137.2002.00519.x
Genard-Zielinski, A. C., Ormeno, E., Boissard, C., and Fernandez, C. (2014). Isoprene emissions from downy oak under water limitation during an entire growing season: what cost for growth? PLoS ONE 9:e112418. doi: 10.1371/journal.pone.0112418
Geron, C. D., Guenther, A. B., and Pierce, T. E. (1994). An improved model for estimating emissions of volatile organic compounds from forests in the eastern United States. J. Geophys. Res. Atmos. 99, 12773–12791. doi: 10.1029/94JD00246
Ghimire, R. P., Kivimäenpää, M., Kasurinen, A., Häikiö, E., Holopainen, T., and Holopainen, J. K. (2017). Herbivore-induced BVOC emissions of Scots pine under warming, elevated ozone and increased nitrogen availability in an open-field exposure. Agric. Forest Meteorol. 242, 21–32. doi: 10.1016/j.agrformet.2017.04.008
Ghirardo, A., Xie, J., Zheng, X., Wang, Y., Grote, R., Block, K., et al. (2016). Urban stress-induced biogenic VOC emissions and SOA-forming potentials in Beijing. Atmos. Chem. Phys. 16, 2901–2920. doi: 10.5194/acp-16-2901-2016
Goldstein, A. H., Fan, S. M., Goulden, M. L., Munger, J. W., and Wofsy, S. C. (1996). Emissions of ethene, propene and 1-butene by a midlatitude forest. J. Geophys. Res. 101, 9149–9157. doi: 10.1029/96JD00334
Goodger, J. Q., Heskes, A. M., and Woodrow, I. E. (2013). Contrasting ontogenetic trajectories for phenolic and terpenoid defences in Eucalyptus froggattii. Ann. Bot. 112, 651–659. doi: 10.1093/aob/mct010
Gratani, L., and Varone, L. (2014). Atmospheric carbon dioxide concentration variations in Rome: relationship with traffic level and urban park size. Urban Ecosyst. 17, 501–511. doi: 10.1007/s11252-013-0340-1
Gromke, C., and Ruck, B. (2009). On the impact of trees on dispersion processes of traffic emissions in street canyons. Bound Lay Meteorol. 131, 19–34. doi: 10.1007/s10546-008-9301-2
Grote, R. (2019). Environmental Impacts on Biogenic Emissions of Volatile Organic Compounds (VOCs), UBA-FB 002772/Eng. Dessau-Rosslau: Umweltbundesamt.
Grote, R., Monson, R. K., and Niinemets, Ü. (2013). “Leaf-level models of constitutive and stress-driven volatile organic compound emissions,” in Biology, Controls and Models of Tree Volatile Organic Compound Emissions, eds Ü. Niinemets and R. K. Monson (Dordrecht: Springer, 315–355.
Grote, R., Samson, R., Alonso, R., Amorim, J. H., Cariñanos, P., Churkina, G., et al. (2016). Functional traits of urban trees: air pollution mitigation potential. Front. Ecol. Environ. 14, 543–550. doi: 10.1002/fee.1426
Grote, R., Sharma, M., Ghirardo, A., and Schnitzler, J. P. (2019). A new modelling approach for estimating abiotic and biotic stress-induced de novo emissions of biogenic volatile organic compounds from plants. Front. Forest. Glob. Change. 2:26. doi: 10.3389/ffgc.2019.00026
Guenther, A. B., Jiang, X., Heald, C. L., Sakulyanontvittaya, T., Duhl, T., Emmons, L. K., et al. (2012). The model of emissions of gases and aerosols from nature version 2.1 (MEGAN2.1): an extended and updated framework for modeling biogenic emissions. Geosci. Model. Dev. 5, 1471–1492. doi: 10.5194/gmd-5-1471-2012
Guenther, A. B., Monson, R. K., and Fall, R. (1991). Isoprene and monoterpene emission rate variability: observations with eucalyptus and emission rate algorithm development. J. Geophys. Res. Atmos. 96, 10799–10808. doi: 10.1029/91JD00960
Guidolotti, G., Pallozzi, E., Gavrichkova, O., Scartazza, A., Mattioni, M., Loreto, F., et al. (2019). Emission of constitutive isoprene, induced monoterpenes and other volatiles under high temperatures in Eucalyptus camaldulensis: a 13C labelling study. Plant Cell Environ. 42, 1929–1938. doi: 10.1111/pce.13521
Hagenbjörk, A., Malmqvist, E., Mattisson, K., Sommar, N. J., and Modig, L. (2017). The spatial variation of O3, NO, NO2 and NOx and the relation between them in two Swedish cities. Environ. Monit. Assess. 189:161. doi: 10.1007/s10661-017-5872-z
Hakola, H., Laurila, T., Lindfors, V., Hellén, H., Gaman, A., and Rinne, J. (2001). Variation of the VOC emission rates of birch species during the growing season. Boreal. Environ. Res. 6, 237–249.
Hakola, H., Rinne, J., and Laurila, T. (1998). The hydrocarbon emission rates of tea-leafed willow (Salix phylicifolia), silver birch (Betula pendula) and European aspen (Populus tremula). Atmos. Environ. 32, 1825–1833. doi: 10.1016/S1352-2310(97)00482-2
Hakola, H., Tarvainen, V., Bäck, J., Ranta, H., Bonn, B., Rinne, J., et al. (2006). Seasonal variation of mono-and sesquiterpene emission rates of Scots pine. Biogeosciences 3, 93–101. doi: 10.5194/bg-3-93-2006
Halliday, H. S., Thompson, A. M., Kollonige, D. W., and Martins, D. K. (2015). Reactivity and temporal variability of volatile organic compounds in the Baltimore/DC region in July 2011. J. Atmos. Chem. 72, 197–213. doi: 10.1007/s10874-015-9306-4
Hardin, P. J., and Jensen, R. R. (2007). The effect of urban leaf area on summertime urban surface kinetic temperatures: a Terre Haute case study. Urban Forest. Urban Green. 6, 63–72. doi: 10.1016/j.ufug.2007.01.005
Harrison, S. P., Morfopoulos, C., Dani, K. S., Prentice, I. C., Arneth, A., Atwell, B. J., et al. (2013). Volatile isoprenoid emissions from plastid to planet. New Phytol. 197, 49–57. doi: 10.1111/nph.12021
Hartikainen, K., Nerg, A.-M., Kivimäenpää, M., Kontunen-Soppela, S., Mäenpää, M., Oksanen, E., et al. (2009). Emissions of volatile organic compounds and leaf structural characteristics of European aspen (Populus tremula) grown under elevated ozone and temperature. Tree Physiol. 29, 1163–1173. doi: 10.1093/treephys/tpp033
Hartikainen, K., Riikonen, J., Nerg, A.-M., Kivimäenpää, M., Ahonen, V., Tervahauta, A., et al. (2012). Impact of elevated temperature and ozone on the emission of volatile organic compounds and gas exchange of silver birch (Betula pendula Roth). Environ. Exp. Bot. 84, 33–43. doi: 10.1016/j.envexpbot.2012.04.014
Heinrich, A. (2007). An estimate of biogenic emissions of volatile organic compounds during summertime in China. Environ. Sci. Pollut. Res. Int. 14, 69–75. doi: 10.1065/espr2007.02.376
Himanen, S. J., Nerg, A. M., Nissinen, A., Pinto, D. M., Stewart, C. N., Poppy, G. M., et al. (2009). Effects of elevated carbon dioxide and ozone on volatile terpenoid emissions and multitrophic communication of transgenic insecticidal oilseed rape (Brassica napus). New Phytol. 181, 174–186. doi: 10.1111/j.1469-8137.2008.02646.x
Holman, C., Harrison, R., and Querol, X. (2015). Review of the efficacy of low emission zones to improve urban air quality in European cities. Atmos. Environ. 111, 161–169. doi: 10.1016/j.atmosenv.2015.04.009
Holmes, K. R., Nelson, T. A., Coops, N. C., and Wulder, M. A. (2013). Biodiversity indicators show climate change will alter vegetation in parks and protected areas. Diversity 5, 352–373. doi: 10.3390/d5020352
Holopainen, J. K. (2004). Multiple functions of inducible plant volatiles. Trends Plant Sci. 9, 529–533. doi: 10.1016/j.tplants.2004.09.006
Holopainen, J. K., and Blande, J. D. (2013). Where do herbivore-induced plant volatiles go? Front. Plant Sci. 4:185. doi: 10.3389/fpls.2013.00185
Holopainen, J. K., and Gershenzon, J. (2010). Multiple stress factors and the emission of plant VOCs. Trends Plant Sci. 15, 176–184. doi: 10.1016/j.tplants.2010.01.006
Hu, B., Jarosch, A.-M., Gauder, M., Graeff-Hönninger, S., Schnitzler, J.-P., Grote, R., et al. (2018). VOC emissions and carbon balance of two bioenergy plantations in response to nitrogen fertilization: a comparison of Miscanthus and Salix. Environ. Pollut. 237, 205–217. doi: 10.1016/j.envpol.2018.02.034
IPCC (2014). “Climate change 2014: Impacts, adaptation, and vulnerability. Part A: Global and sectoral aspects,” in Contribution of Working Group II to the Fifth Assessment Report of the Intergovernmental Panel on Climate Change, eds C. B. Field, V. R. Barros, D. J. Dokken, K. J. Mach, M. D. Mastrandrea, T. E. Bilir, et al. (Cambridge, UK; New York, NY: Cambridge University Press).
Janhäll, S. (2015). Review on urban vegetation and particle air pollution–deposition and dispersion. Atmos. Environ. 105, 130–137. doi: 10.1016/j.atmosenv.2015.01.052
Jansen, R. M., Miebach, M., Kleist, E., Van Henten, E. J., and Wildt, J. (2009). Release of lipoxygenase products and monoterpenes by tomato plants as an indicator of Botrytis cinerea-induced stress. Plant Biol. 11, 859–868. doi: 10.1111/j.1438-8677.2008.00183.x
Jardine, K. J., Jardine, A. B., Souza, V. F., Carneiro, V., Ceron, J. V., Gimenez, B. O., et al. (2016). Methanol and isoprene emissions from the fast growing tropical pioneer species Vismia guianensis (Aubl.) Pers. (Hypericaceae) in the central Amazon forest. Atmos. Chem. Phys. 16, 6441–6452. doi: 10.5194/acp-16-6441-2016
Jenkin, M., Young, J., and Rickard, A. (2015). The MCM v3. 3.1 degradation scheme for isoprene. Atmos. Chem. Phys. 15, 11433–11459. doi: 10.5194/acp-15-11433-2015
Kaiser, J., Skog, K. M., Baumann, K., Bertman, S. B., Brown, S. B., Brune, W. H., et al. (2016). Speciation of OH reactivity above the canopy of an isoprene-dominated forest. Atmos. Chem. Phys. 16, 9349–9359. doi: 10.5194/acp-16-9349-2016
Kamens, R. M., Gery, M. W., Jeffries, H. E., Jackson, M., and Cole, E. I. (1982). Ozone-isoprene reactions: Product formation and aerosol potential. Int. J. Chem. Kinet. 14, 955–975.
Karl, M., Guenther, A., Köble, R., Leip, A., and Seufert, G. (2009). A new European plant-specific emission inventory of biogenic volatile organic compounds for use in atmospheric transport models. Biogeosciences 6, 1059–1087. doi: 10.5194/bg-6-1059-2009
Karl, T., Graus, M., Striednig, M., Lamprecht, C., Hammerle, A., Wohlfahrt, G., et al. (2017). Urban eddy covariance measurements reveal significant missing NOx emissions in Central Europe. Sci. Rep. UK 7:2536. doi: 10.1038/s41598-017-02699-9
Kaser, L., Karl, T., Yuan, B., Mauldin, R. L. III., Cantrell, C., Guenther, A. B., et al. (2015). Chemistry-turbulence interactions and mesoscale variability influence the cleansing efficiency of the atmosphere. Geophys. Res. Lett. 42,894–810; 903. doi: 10.1002/2015GL066641
Kesselmeier, J., and Staudt, M. (1999). Biogenic volatile organic compounds (VOC): an overview on emission, physiology and ecology. J. Atmos. Chem. 33, 23–88. doi: 10.1023/A:1006127516791
Kim, S., Guenther, A., Karl, T., and Greenberg, J. (2011). Contributions of primary and secondary biogenic VOC tototal OH reactivity during the CABINEX (Community Atmosphere-Biosphere INteractions Experiments)-09 field campaign. Atmos. Chem. Phys. 11, 8613–8623. doi: 10.5194/acp-11-8613-2011
Kirchner, F., Jeanneret, F., Clappier, A., Krüger, B., van den Bergh, H., and Calpini, B. (2001). Total VOC reactivity in the planetary boundary layer: 2. A new indicator for determining the sensitivity of the ozone production to VOC and NOx. J. Geophys. Res. Atmos. 106, 3095–3110. doi: 10.1029/2000JD900603
Klein, T. (2014). The variability of stomatal sensitivity to leaf water potential across tree species indicates a continuum between isohydric and anisohydric behaviours. Funct. Ecol. 28, 1313–1320. doi: 10.1111/1365-2435.12289
König, G., Brunda, M., Puxbaum, H., Hewitt, C. N., Duckham, S. C., and Rudolph, J. (1995). Relative contribution of oxygenated hydrocarbons to the total biogenic VOC emissions of selected mid-European agricultural and natural plant species. Atmos. Environ. 29, 861–874. doi: 10.1016/1352-2310(95)00026-U
Kopinga, J., and Van den Burg, J. (1995). Using soil and foliar analysis to diagnose the nutritional status of urban trees. J. Arboric. 21:17.
Kourtchev, I., Ruuskanen, T. M., Keronen, P., Sogacheva, L., Dal Maso, M., Reissell, A., et al. (2008). Determination of isoprene and alpha-/beta-pinene oxidation products in boreal forest aerosols from Hyytiälä, Finland: diel variations and possible link with particle formation events. Plant Biol. 10, 138–149. doi: 10.1055/s-2007-964945
Kramer, K., Degen, B., Buschbom, J., Hickler, T., Thuiller, W., Sykes, M. T., et al. (2010). Modelling exploration of the future of European beech (Fagus sylvatica L.) under climate change—range, abundance, genetic diversity and adaptive response. Forest. Ecol. Manage. 259, 2213–2222. doi: 10.1016/j.foreco.2009.12.023
Kühn, I., Brandl, R., and Klotz, S. (2004). The flora of German cities is naturally species rich. Evol. Ecol. Res. 6, 749–764.
Lahr, E. C., Schade, G. W., Crossett, C. C., and Watson, M. R. (2015). Photosynthesis and isoprene emission from trees along an urban-rural gradient in Texas. Glob. Chang. Biol. 21, 4221–4236. doi: 10.1111/gcb.13010
Lamarque, J.-F., Shindell, D. T., Josse, B., Young, P., Cionni, I., Eyring, V., et al. (2013). The atmospheric chemistry and climate model intercomparison project (ACCMIP): overview and description of models, simulations and climate diagnostics. Geosci. Model. Dev. 6, 179–206. doi: 10.5194/gmd-6-179-2013
Lamb, B., Guenther, A., Gay, D., and Westberg, H. (1987). A national inventory of biogenic hydrocarbon emissions. Atmos. Environ. 21, 1695–1705. doi: 10.1016/0004-6981(87)90108-9
Lambers, H., Chapin, F. S. III, and Pons, T. L. (1998). Plant Physiological Ecology. New-York, NY: Springer.
Lerdau, M., Litvak, M., Palmer, P., and Monson, R. (1997). Controls over monoterpene emissions from boreal forest conifers. Tree Physiol. 17, 563–569. doi: 10.1093/treephys/17.8-9.563
Lerdau, M., Matson, P., Fall, R., and Monson, R. (1995). Ecological controls over monoterpene emissions from Douglas-fir (Pseudotsuga menziesii). Ecology 76, 2640–2647. doi: 10.2307/2265834
Li, D., Sun, T., Liu, M., Yang, L., Wang, L., and Gao, Z. (2015). Contrasting responses of urban and rural surface energy budgets to heat waves explain synergies between urban heat islands and heat waves. Environ. Res. Lett. 10:054009. doi: 10.1088/1748-9326/10/5/054009
Li, D. W., Shi, Y., He, X. Y., and Chi, G. Y. (2011). Seasonal variations of BVOCs emission from Ginkgo biloba linn in urban area. Appl. Mech. Mater. 71–78, 2891–2894. doi: 10.4028/www.scientific.net/AMM.71-78.2891
Liao, C.-T., and Lin, C.-H. (2001). Physiological adaptation of crop plants to flooding stress. Proc. Natl. Sci. Counc. Repub. China B 25, 148–157.
Livesley, S. J., McPherson, G. M., and Calfapietra, C. (2016). The urban forest and ecosystem services: impacts on urban water, heat, and pollution cycles at the tree, street, and city scale. J. Environ. Qual. 45, 119–124. doi: 10.2134/jeq2015.11.0567
Loreto, F., Barta, C., Brilli, F., and Nogues, I. (2006). On the induction of volatile organic compound emissions by plants as consequence of wounding or fluctuations of light and temperature. Plant Cell Environ. 29, 1820–1828. doi: 10.1111/j.1365-3040.2006.01561.x
Loreto, F., and Delfine, S. (2000). Emission of isoprene from salt-stressed Eucalyptus globulus leaves. Plant Physiol. 123, 1605–1610. doi: 10.1104/pp.123.4.1605
Loreto, F., and Schnitzler, J.-P. (2010). Abiotic stresses and induced BVOCs. Trends Plant Sci. 15, 154–166. doi: 10.1016/j.tplants.2009.12.006
Loreto, F., and Velikova, V. (2001). Isoprene produced by leaves protects the photosynthetic apparatus against ozone damage, quenches ozone products, and reduces lipid peroxidation of cellular membranes. Plant Physiol. 127, 1781–1787. doi: 10.1104/pp.010497
Maronga, B., Gross, G., Raasch, S., Banzhaf, S., Forkel, R., Heldens, W., et al. (2019). Development of a new urban climate model based on the model PALM–Project overview, planned work, and first achievements. Meteorol. Z. 28, 105–119. doi: 10.1127/metz/2019/0909
Medlyn, B. E., Barton, C. V. M., Broadmeadow, M. S. J., Ceulemans, R., De Angelis, P., Forstreuter, M., et al. (2001). Stomatal conductance of forest species after long-term exposure to elevated CO2 concentration: a synthesis. New Phytol. 149, 247–264. doi: 10.1046/j.1469-8137.2001.00028.x
Meeningen, Y. V., Schurgers, G., Rinnan, R., and Holst, T. (2016). BVOC emissions from English oak (Quercus robur) and European beech (Fagus sylvatica) along a latitudinal gradient. Biogeosciences 13, 6067–6080. doi: 10.5194/bg-13-6067-2016
Meineke, E. K., Dunn, R. R., Sexton, J. O., and Frank, S. D. (2013). Urban warming drives insect pest abundance on street trees. PLoS ONE 8:e59687. doi: 10.1371/journal.pone.0059687
Mills, G., Hayes, F., Simpson, D., Emberson, L., Norris, D., Harmens, H., et al. (2011). Evidence of widespread effects of ozone on crops and (semi-)natural vegetation in Europe (1990–2006) in relation to AOT40- and flux-based risk maps. Glob. Change Biol. 17, 592–613. doi: 10.1111/j.1365-2486.2010.02217.x
Monks, P., Granier, C., Fuzzi, S., Stohl, A., Williams, M., Akimoto, H., et al. (2009). Atmospheric composition change–global and regional air quality. Atmos. Environ. 43, 5268–5350. doi: 10.1016/j.atmosenv.2009.08.021
Morani, A., Nowak, D., Hirabayashi, S., Guidolotti, G., Medori, M., Muzzini, V., et al. (2014). Comparing i-Tree modeled ozone deposition with field measurements in a periurban Mediterranean forest. Environ. Pollut. 195, 202–209. doi: 10.1016/j.envpol.2014.08.031
Munck, I. A., Bennett, C. M., Camilli, K. S., and Nowak, R. S. (2010). Long-term impact of de-icing salts on tree health in the Lake Tahoe Basin: environmental influences and interactions with insects and diseases. Forest. Ecol. Manage. 260, 1218–1229. doi: 10.1016/j.foreco.2010.07.015
Neirynck, J., and Verstraeten, A. (2018). Variability of ozone deposition velocity over a mixed suburban temperate forest. Front. Environ. Sci. 6:82. doi: 10.3389/fenvs.2018.00082
Niinemets, Ü. (2010). Mild versus severe stress and BVOCs: thresholds, priming and consequences. Trends Plant Sci. 15, 145–153. doi: 10.1016/j.tplants.2009.11.008
Niinemets, Ü., Arneth, A., Kuhn, U., Monson, R., Peñuelas, J., and Staudt, M. (2010). The emission factor of volatile isoprenoids: stress, acclimation, and developmental responses. Biogeosciences 7, 2203–2223. doi: 10.5194/bg-7-2203-2010
Niinemets, Ü., Kännaste, A., and Copolovici, L. (2013). Quantitative patterns between plant volatile emissions induced by biotic stresses and the degree of damage. Front. Plant Sci. 4:262. doi: 10.3389/fpls.2013.00262
Niinemets, Ü., and Monson, R. K. (2013). Biology, Controls and Models of Tree Volatile Organic Compound Emissions. Dordrecht: Springer. doi: 10.1007/978-94-007-6606-8
Nölscher, A. C., Yanez-Serrano, A. M., Wolff, S., De Araujo, A. C., Lavrič, J. V., Kesselmeier, J., et al. (2016). Unexpected seasonality in quantity and composition of Amazon rainforest air reactivity. Nat. Commun. 7:10383. doi: 10.1038/ncomms10383
Norton, B. A., Coutts, A. M., Livesley, S. J., Harris, R. J., Hunter, A. M., and Williams, N. S. (2015). Planning for cooler cities: a framework to prioritise green infrastructure to mitigate high temperatures in urban landscapes. Landsc. Urban Plan. 134, 127–138. doi: 10.1016/j.landurbplan.2014.10.018
Nowak, D. J., Hirabayashi, S., Doyle, M., McGovern, M., and Pasher, J. (2018). Air pollution removal by urban forests in Canada and its effect on air quality and human health. Urban Forest. Urban Green. 29, 40–48. doi: 10.1016/j.ufug.2017.10.019
Nowak, D. J., Hoehn, R. E. III., Crane, D. E., Stevens, J. C., and Fisher, C. L. (2010). Assessing urban forest effects and values, Chicago's urban forest. Resour. Bull. 37, 1–27. doi: 10.2737/NRS-RB-37
O'Driscoll, R., Stettler, M. E., Molden, N., Oxley, T., and ApSimon, H. M. (2018). Real world CO2 and NOx emissions from 149 Euro 5 and 6 diesel, gasoline and hybrid passenger cars. Sci. Total Environ. 621, 282–290. doi: 10.1016/j.scitotenv.2017.11.271
Ordóñez-Barona, C., Sabetski, V., Millward, A. A., and Steenberg, J. (2018). De-icing salt contamination reduces urban tree performance in structural soil cells. Environ. Pollut. 234, 562–571. doi: 10.1016/j.envpol.2017.11.101
Ormeño, E., Baldy, V., Ballini, C., and Fernandez, C. (2008). Production and diversity of volatile terpenes from plants on calcareous and siliceous soils: effect of soil nutrients. J. Chem. Ecol. 34:1219. doi: 10.1007/s10886-008-9515-2
Ormeño, E., and Fernandez, C. (2012). Effect of soil nutrient on production and diversity of volatile terpenoids from plants. Curr. Bioact. Comp. 8, 71–79. doi: 10.2174/157340712799828188
Owen, S., Boissard, C., Street, R., Duckham, S., Csiky, O., and Hewitt, C. (1997). Screening of 18 Mediterranean plant species for volatile organic compound emissions. Atmos. Environ. 31, 101–117. doi: 10.1016/S1352-2310(97)00078-2
Paoletti, E., Bardelli, T., Giovannini, G., and Pecchioli, L. (2011). Air quality impact of an urban park over time. Procedia Environ. Sci. 4, 10–16. doi: 10.1016/j.proenv.2011.03.002
Papiez, M. R., Potosnak, M. J., Goliff, W. S., Guenther, A. B., Matsunaga, S. N., and Stockwell, W. R. (2009). The impacts of reactive terpene emissions from plants on air quality in Las Vegas, Nevada. Atmos. Environ. 43, 4109–4123. doi: 10.1016/j.atmosenv.2009.05.048
Pauleit, S., Jones, N., Garcia-Martin, G., Garcia-Valdecantos, J. L., Rivière, L. M., Vidal-Beaudet, L., et al. (2002). Tree establishment practice in towns and cities–Results from a European survey. Urban Forest. Urban Green. 1, 83–96. doi: 10.1078/1618-8667-00009
Pegoraro, E., Rey, A. N. A., Abrell, L., Haren, J., and Lin, G. (2006). Drought effect on isoprene production and consumption in Biosphere 2 tropical rainforest. Glob. Change Biol. 12, 456–469. doi: 10.1111/j.1365-2486.2006.01112.x
Peñuelas, J., and Staudt, M. (2010). BVOCs and global change. Trends Plant Sci. 15, 133–144. doi: 10.1016/j.tplants.2009.12.005
Pinto, D. M., Blande, J. D., Souza, S. R., Nerg, A. M., and Holopainen, J. K. (2010). Plant volatile organic compounds (VOCs) in ozone (O3) polluted atmospheres: the ecological effects. J. Chem. Ecol. 36, 22–34. doi: 10.1007/s10886-009-9732-3
Potosnak, M. J., LeStourgeon, L., and Nunez, O. (2014a). Increasing leaf temperature reduces the suppression of isoprene emission by elevated CO2 concentration. Sci. Total Environ. 481, 352–359. doi: 10.1016/j.scitotenv.2014.02.065
Potosnak, M. J., LeStourgeon, L., Pallardy, S. G., Hosman, K. P., Gu, L., Karl, T., et al. (2014b). Observed and modeled ecosystem isoprene fluxes from an oak-dominated temperate forest and the influence of drought stress. Atmos. Environ. 84, 314–322. doi: 10.1016/j.atmosenv.2013.11.055
Préndez, M., Carvajal, V., Corada, K., Morales, J., Alarcón, F., and Peralta, H. (2013). Biogenic volatile organic compounds from the urban forest of the Metropolitan Region, Chile. Environ. Pollut. 183, 143–150. doi: 10.1016/j.envpol.2013.04.003
Ran, L., Zhao, C. S., Xu, W. Y., Lu, X. Q., Han, M., Lin, W. L., et al. (2011). VOC reactivity and its effect on ozone production during the HaChi summer campaign. Atmos. Chem. Phys. 11, 4657–4667. doi: 10.5194/acp-11-4657-2011
Rhew, R. C., Deventer, M. J., Turnipseed, A. A., Warneke, C., Ortega, J., Shen, S., et al. (2017). Ethene, propene, butene and isoprene emissions from a ponderosa pine forest measured by Relaxed Eddy Accumulation. Atmos. Chem. Phys. 17, 13417–13438. doi: 10.5194/acp-17-13417-2017
Rose, D., and Webber, J. (2011). De-Icing Salt Damage to Trees. Pathology Advisory Note 11, Forest Research. Edinburgh: Forestry Commission.
Rosenstiel, T. N., Potosnak, M. J., Griffin, K. L., Fall, R., and Monson, R. K. (2003). Increased CO2 uncouples growth from isoprene emission in an agriforest ecosystem. Nature 421:256. doi: 10.1038/nature01312
Sæbø, A., Borzan, Ž., Ducatillion, C., Hatzistathis, A., Lagerström, T., Supuka, J., et al. (2005). “The selection of plant materials for street trees, park trees and urban woodland,” in Urban Forests and Trees, eds C. Konijnendijk, K. Nilsson, T. Randrup and J. Schipperijn. (Berlin; Heidelberg: Springer, 257–280. doi: 10.1007/3-540-27684-X_11
Saaroni, H., Amorim, J. H., Hiemstra, J., and Pearlmutter, D. (2018). Urban Green Infrastructure as a tool for urban heat mitigation: Survey of research methodologies and findings across different climatic regions. Urban Clim. 24, 94–110. doi: 10.1016/j.uclim.2018.02.001
Sade, N., Gebremedhin, A., and Moshelion, M. (2012). Risk-taking plants: anisohydric behavior as a stress-resistance trait. Plant Signal. Behav. 7, 767–770. doi: 10.4161/psb.20505
Samson, R., Grote, R., Calfapietra, C., Cariñanos, P., Fares, S., Paoletti, E., et al. (2017a). “Urban trees and their relation to air pollution,” in The Urban Forest: Cultivating Green Infrastructure for People and the Environment, eds D. Pearlmutter, C. Calfapietra, R. Samson, L. O'Brien, S. Krajter Ostoić, G. Sanesi et al. (Cham: Springer International Publishing, 21–30.
Samson, R., Ningal, T. F., Tiwary, A., Grote, R., Fares, S., Saaroni, H., et al. (2017b). “Species-specific information for enhancing ecosystem services,” in The Urban Forest, eds D. Pearlmutter, C. Calfapietra, R. Samson, L. O'Brien, S. Krajter Ostoić, G. Sanesi, and R. Alonso del Amo (Cham: Springer), 111–144.
Saunier, A., Ormeño, E., Boissard, C., Wortham, H., Temime-Roussel, B., Lecareux, C., et al. (2017). Effect of mid-term drought on Quercus pubescens; BVOCs' emission seasonality and their dependency on light and/or temperature. Atmos. Chem. Phys. 17, 7555–7566. doi: 10.5194/acp-17-7555-2017
Saunier, A., Ormeno, E., Havaux, M., Wortham, H., Ksas, B., Temime-Roussel, B., et al. (2018). Resistance of native oak to recurrent drought conditions simulating predicted climatic changes in the Mediterranean region. Plant Cell Environ. 41, 2299–2312. doi: 10.1111/pce.13331
Scala, A., Allmann, S., Mirabella, R., Haring, M., and Schuurink, R. (2013). Green leaf volatiles: a plant's multifunctional weapon against herbivores and pathogens. Int. J. Mol. Sci. 14, 17781–17811. doi: 10.3390/ijms140917781
Seco, R., Peñuelas, J., and Filella, I. (2007). Short-chain oxygenated VOCs: Emission and uptake by plants and atmospheric sources, sinks, and concentrations. Atmos. Environ. 41, 2477–2499. doi: 10.1016/j.atmosenv.2006.11.029
Shannon, M., Banuelos, G., Draper, J., Ajwa, H., Jordahl, J., and Licht, L. (1999). Tolerance of hybrid poplar (Populus) trees irrigated with varied levels of salt, selenium, and boron. Int. J. Phytoremediat. 1, 273–288. doi: 10.1080/15226519908500020
Sheffer, E. (2012). A review of the development of Mediterranean pine–oak ecosystems after land abandonment and afforestation: are they novel ecosystems? Ann. Forest. Sci. 69, 429–443. doi: 10.1007/s13595-011-0181-0
Sicard, P., De Marco, A., Troussier, F., Renou, C., Vas, N., and Paoletti, E. (2013). Decrease in surface ozone concentrations at Mediterranean remote sites and increase in the cities. Atmos. Environ. 79, 705–715. doi: 10.1016/j.atmosenv.2013.07.042
Sieghardt, M., Mursch-Radlgruber, E., Paoletti, E., Couenberg, E., Dimitrakopoulus, A., Rego, F., et al. (2005). “The abiotic urban environment: impact of urban growing conditions on urban vegetation,” in Urban Forests and Trees, eds C. Konijnendijk, K. Nilsson, T. Randrup, and J. Schipperijn (Cham: Springer, 281–323. doi: 10.1007/3-540-27684-X_12
Sillman, S., and He, D. (2002). Some theoretical results concerning O3-NOx-VOC chemistry and NOx-VOC indicators. J. Geophys. Res. D22:4659 doi: 10.1029/2001JD001123
Singh, A. P., Varshney, C. K., and Singh, U. K. (2007). Seasonal variations in isoprene emission from tropical deciduous tree species. Environ. Monit. Assess. 131, 231–235. doi: 10.1007/s10661-006-9471-7
Singsaas, E. L., Lerdau, M., Winter, K., and Sharkey, T. D. (1997). Isoprene increases thermotolerance of isoprene-emitting species. Plant Physiol. 115, 1413–1420. doi: 10.1104/pp.115.4.1413
Siwko, M. E., Marrink, S. J., de Vries, A. H., Kozubek, A., Uiterkamp, A. J. S., and Mark, A. E. (2007). Does isoprene protect plant membranes from thermal shock? A molecular dynamics study. Biochim. Biophys. Acta Biomembr. 1768, 198–206. doi: 10.1016/j.bbamem.2006.09.023
Sjöman, H., and Busse Nielsen, A. (2010). Selecting trees for urban paved sites in Scandinavia – a review of information on stress tolerance and its relation to the requirements of tree planners. Urban Forest. Urban Green. 9, 281–293. doi: 10.1016/j.ufug.2010.04.001
Solberg, S., Hov, Ø., Søvde, A., Isaksen, I. S. A., Coddeville, P., De Backer, H., et al. (2008). European surface ozone in the extreme summer 2003. J. Geophys. Res. Atmos. 113:D07307. doi: 10.1029/2007JD009098
Steinbrecher, R., Smiatek, G., Köble, R., Seufert, G., Theloke, J., Hauff, K., et al. (2009). Intra-and inter-annual variability of VOC emissions from natural and semi-natural vegetation in Europe and neighbouring countries. Atmos. Environ. 43, 1380–1391. doi: 10.1016/j.atmosenv.2008.09.072
Steindel, F., Beauchamp, J., Hansel, A., Kesselmeier, J., Kleist, E., Kuhn, U., et al. (2005). “Stress induced VOC emissions from mildew infested oak,” in Geophysical Research Abstract 7 EGU05-A-03010.
Stemmler, K., Bugmann, S., Buchmann, B., Reimann, S., and Staehelin, J. (2005). Large decrease of VOC emissions of Switzerland's car fleet during the past decade: results from a highway tunnel study. Atmos. Environ. 39, 1009–1018. doi: 10.1016/j.atmosenv.2004.10.010
Streiling, S., and Matzarakis, A. (2003). Influence of single and small clusters of trees on the bioclimate of a city: a case study. J. Arboric. 29, 309–316.
Taha, H., Wilkinson, J., Bornstein, R., Xiao, Q., McPherson, G., Simpson, J., et al. (2016). An urban-forest control measure for ozone in the Sacramento, CA Federal Non-Attainment Area (SFNA). Sustain. Cities Soc. 21, 51–65. doi: 10.1016/j.scs.2015.11.004
Tarvainen, V., Hakola, H., Hellén, H., Bäck, J., Hari, P., and Kulmala, M. (2005). Temperature and light dependence of the VOC emissions of Scots pine. Atmos. Chem. Phys. 5, 989–998. doi: 10.5194/acp-5-989-2005
Teuber, M., Zimmer, I., Kreuzwieser, J., Ache, P., Polle, A., Rennenberg, H., et al. (2008). VOC emissions of Grey poplar leaves as affected by salt stress and different N sources. Plant Biol. 10, 86–96. doi: 10.1111/j.1438-8677.2007.00015.x
Tiiva, P., Faubert, P., Räty, S., Holopainen, J. K., Holopainen, T., and Rinnan, R. (2009). Contribution of vegetation and water table on isoprene emission from boreal peatland microcosms. Atmos. Environ. 43, 5469–5475. doi: 10.1016/j.atmosenv.2009.07.026
Tingey, D., Turner, D., and Weber, J. (1991). “Factors controlling the emissions of monoterpenes and other volatile organic compounds,” in Trace Gas Emission by Plants, eds T. D. Sharkey, E. A. Holland, and H. A. Mooney (Academic Press), 93−119.
Tingey, D. T., Evans, R., and Gumpertz, M. (1981). Effects of environmental conditions on isoprene emission from live oak. Planta 152, 565–570. doi: 10.1007/BF00380829
Tiwary, A., Namdeo, A., Fuentes, J., Dore, A., Hu, X.-M., and Bell, M. (2013). Systems scale assessment of the sustainability implications of emerging green initiatives. Environ. Pollut. 183, 213–223. doi: 10.1016/j.envpol.2013.03.049
Tong, Z., Baldauf, R. W., Isakov, V., Deshmukh, P., and Max Zhang, K. (2016). Roadside vegetation barrier designs to mitigate near-road air pollution impacts. Sci. Total Environ. 541, 920–927. doi: 10.1016/j.scitotenv.2015.09.067
Trenberth, K. E., Dai, A., Van Der Schrier, G., Jones, P. D., Barichivich, J., Briffa, K. R., et al. (2014). Global warming and changes in drought. Nat. Clim. Change 4, 17–22. doi: 10.1038/nclimate2067
Trowbridge, A. M., and Stoy, P. C. (2013). “BVOC-mediated plant-herbivore interactions,” in Biology, Controls and Models of Tree Volatile Organic Compound Emissions, Ü. Niinemets and R. K. Monson (Dordrecht: Springer, 21–46.
Valkama, E., Koricheva, J., and Oksanen, E. (2007). Effects of elevated O3, alone and in combination with elevated CO2, on tree leaf chemistry and insect herbivore performance: a meta-analysis. Glob. Chang. Biol. 13, 184–201. doi: 10.1111/j.1365-2486.2006.01284.x
Veldt, C. (1989). Leaf Biomass Data for the Estimation of Biogenic VOC Emissions. Apeldoorn: MTO-TNO Report 99–306.
Vitale, M., Salvatori, E., Loreto, F., Fares, S., and Manes, F. (2007). Physiological responses of Quercus ilex leaves to water stress and acute ozone exposure under controlled conditions. Water Air Soil Pollut. 189, 113–125. doi: 10.1007/s11270-007-9560-4
Vos, P. E., Maiheu, B., Vankerkom, J., and Janssen, S. (2013). Improving local air quality in cities: to tree or not to tree? Environ. Pollut. 183, 113–122. doi: 10.1016/j.envpol.2012.10.021
Vuorinen, T., Nerg, A.-M., Vapaavuori, E., and Holopainen, J. K. (2005). Emission of volatile organic compounds from two silver birch (Betula pendula Roth) clones grown under ambient and elevated CO2 and different O3 concentrations. Atmos. Environ. 39, 1185–1197. doi: 10.1016/j.atmosenv.2004.09.077
Wagner, P., and Kuttler, W. (2014). Biogenic and anthropogenic isoprene in the near-surface urban atmosphere—a case study in Essen, Germany. Sci. Total Environ. 475, 104–115. doi: 10.1016/j.scitotenv.2013.12.026
Wang, T., Xue, L., Brimblecombe, P., Lam, Y. F., Li, L., and Zhang, L. (2017). Ozone pollution in China: a review of concentrations, meteorological influences, chemical precursors, and effects. Sci. Total Environ. 575, 1582–1596. doi: 10.1016/j.scitotenv.2016.10.081
Wesely, M. (1989). Parameterization of surface resistances to gaseous dry deposition in regional-scale numerical models. Atmos. Environ. 23, 1293–1304. doi: 10.1016/0004-6981(89)90153-4
Wesely, M., and Hicks, B. (2000). A review of the current status of knowledge on dry deposition. Atmos. Environ. 34, 2261–2282. doi: 10.1016/S1352-2310(99)00467-7
WHO (2006). WHO Air Quality Guidelines for Particulate Matter, Ozone, Nitrogen Dioxide and Sulfur Dioxide: Global Update 2005: Summary of Risk Assessment (No. WHO/SDE/PHE/OEH/06.02). Geneva: World Health Organization.
Williams, J., Kessel, S. U., Nölscher, A. C., Yang, Y. D., Lee, Y., Yanez-Serrano, A. M., et al. (2016). Opposite OH reactivity and ozone cycles in the Amazon rainforest and megacity Beijing: subversion of biospheric oxidant control by anthropogenic emissions. Atmos. Environ. 125, 112–118. doi: 10.1016/j.atmosenv.2015.11.007
Wissmar, R. C., Timm, R. K., and Logsdon, M. G. (2004). Effects of changing forest and impervious land covers on discharge characteristics of watersheds. Environ. Manage. 34, 91–98. doi: 10.1007/s00267-004-0224-5
Wittig, V. E., Ainsworth, E. A., and Long, S. P. (2007). To what extent do current and projected increases in surface ozone affect photosynthesis and stomatal conductance of trees? A meta-analytic review of the last 3 decades of experiments. Plant Cell Environ. 30, 1150–1162. doi: 10.1111/j.1365-3040.2007.01717.x
Wolfe, G., and Thornton, J. (2011). The chemistry of atmosphere-forest exchange (CAFÉ) model–Part 1: model description and characterization. Atmos. Chem. Phys. 11, 77–101. doi: 10.5194/acp-11-77-2011
Wolyniak, B., and Elmendorf, W. F. (2011). Lancaster City Tree Inventory: Summary Report. Penn State School of Forest Resources.
Wu, C., Pullinen, I., Andres, S., Carriero, G., Fares, S., Goldbach, H., et al. (2015). Impacts of soil moisture on de novo monoterpene emissions from European beech, Holm oak, Scots pine, and Norway spruce. Biogeosciences 12, 177–191. doi: 10.5194/bg-12-177-2015
Xie, X., Shao, M., Liu, Y., Lu, S., Chang, C.-C., and Chen, Z.-M. (2008). Estimate of initial isoprene contribution to ozone formation potential in Beijing, China. Atmos. Environ. 42, 6000–6010. doi: 10.1016/j.atmosenv.2008.03.035
Yang, J., Chang, Y., and Yan, P. (2015). Ranking the suitability of common urban tree species for controlling PM2.5 pollution. Atmos. Pollut. Res. 6, 267–277. doi: 10.5094/APR.2015.031
Yang, P., Ren, G., and Hou, W. (2017). Temporal–spatial patterns of relative humidity and the urban dryness Island effect in Beijing City. J. Appl. Meteorol. Climatol. 56, 2221–2237. doi: 10.1175/JAMC-D-16-0338.1
Yli-Pelkonen, V., Viippola, V., Rantalainen, A.-L., Zheng, J., and Setälä, H. (2018). The impact of urban trees on concentrations of PAHs and other gaseous air pollutants in Yanji, northeast China. Atmos. Environ. 192, 151–159. doi: 10.1016/j.atmosenv.2018.08.061
Young, P. J., Archibald, A. T., Bowman, K. W., Lamarque, J. F., Naik, V., Stevenson, D. S., et al. (2013). Pre-industrial to end 21st century projections of tropospheric ozone from the Atmospheric Chemistry and Climate Model Intercomparison Project (ACCMIP). Atmos. Chem. Phys. 13, 2063–2090. doi: 10.5194/acp-13-2063-2013
Youngsteadt, E., Dale, A. G., Terando, A. J., Dunn, R. R., and Frank, S. D. (2015). Do cities simulate climate change? A comparison of herbivore response to urban and global warming. Glob. Chang. Biol. 21, 97–105. doi: 10.1111/gcb.12692
Zhu, Z., Tsokankunku, A., Plake, D., Falge, E., Foken, T., and Meixner, F. (2009). “Multi-level eddy covariance measurements for ozone fluxes above, within and below spruce forest canopy,” in Proceedings of the International Conference of Atmospheric Transport and Chemistry in Forest Ecosystems (Thurnau, Bayreuth University; Abteilung Mikrometeorologie).
Keywords: biogenic volatile organic compounds, ozone formation and deposition, roadside trees, rural-urban gradient, stress, urban forest, urban trees
Citation: Fitzky AC, Sandén H, Karl T, Fares S, Calfapietra C, Grote R, Saunier A and Rewald B (2019) The Interplay Between Ozone and Urban Vegetation—BVOC Emissions, Ozone Deposition, and Tree Ecophysiology. Front. For. Glob. Change 2:50. doi: 10.3389/ffgc.2019.00050
Received: 03 June 2019; Accepted: 13 August 2019;
Published: 06 September 2019.
Edited by:
Mark Potosnak, DePaul University, United StatesReviewed by:
Xu Yue, Nanjing University of Information Science and Technology, ChinaDasa Gu, Hong Kong University of Science and Technology, Hong Kong
Copyright © 2019 Fitzky, Sandén, Karl, Fares, Calfapietra, Grote, Saunier and Rewald. This is an open-access article distributed under the terms of the Creative Commons Attribution License (CC BY). The use, distribution or reproduction in other forums is permitted, provided the original author(s) and the copyright owner(s) are credited and that the original publication in this journal is cited, in accordance with accepted academic practice. No use, distribution or reproduction is permitted which does not comply with these terms.
*Correspondence: Hans Sandén, aGFucy5zYW5kZW5AYm9rdS5hYy5hdA==