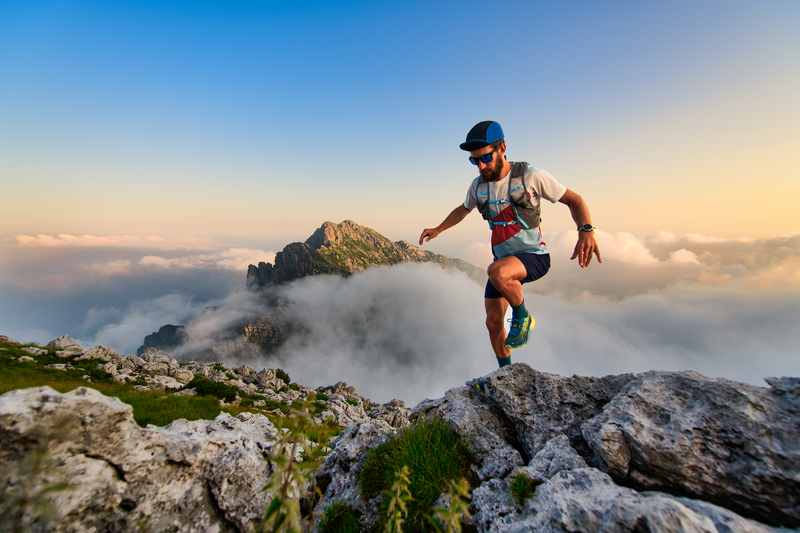
94% of researchers rate our articles as excellent or good
Learn more about the work of our research integrity team to safeguard the quality of each article we publish.
Find out more
MINI REVIEW article
Front. For. Glob. Change , 26 October 2018
Sec. Forest Ecophysiology
Volume 1 - 2018 | https://doi.org/10.3389/ffgc.2018.00004
This article is part of the Research Topic Fire, Flood, and Fury – Forest Ecophysiological Response to Extreme Events View all 3 articles
Tree mortality events driven by drought and warmer temperature, often amplified by pests and pathogens, are emerging as one of the predominant climate change impacts on plants. Understanding and predicting widespread tree mortality events in the future is vital as they affect ecosystem goods and services provided by forests and woodlands, including carbon storage needed to help offset warming. Additionally, if extensive enough, tree die-off events can influence not only local climate but also climate and vegetation elsewhere via ecoclimate teleconnections. Consequently, recent efforts have focused on improving predictions of tree mortality. One of the most commercially important genera of trees is Pinus, and the most studied species globally for drought-induced tree mortality is piñon pine, Pinus edulis. Numerous metrics have been developed in association with predicting mortality thresholds or variations in mortality for this species. In this article, we compiled metrics associated with drought and warming related mortality that were developed for P. edulis or for which P. edulis was a key example species used in a calculation or prediction. We grouped these metrics into three categories: (i) those related to simple climate variables, (ii) those related to physiological responses, and (iii) those that require multi-step calculations or modeling using climate, ecohydrological, and/or ecophysiological data; and we identified the spatial-temporal scale of each of these metrics. We also compiled factors shown to modify rates or sensitivities of mortality. The metrics to predict mortality include empirical ones which often have implicit linkages to expected mechanisms, and more mechanistic ones related to physiological drivers. The metrics for P. edulis have similarities with those available for other species of Pinus. Expected future mortality events will provide an opportunity to observationally and experimentally test and compare these metrics related to tree mortality for P. edulis via near-term ecological forecasting. The metrics for P. edulis may also be useful as potential analogs for other genera. Improving predictions of tree mortality for this species and others will be increasingly important as an aid to move toward anticipatory management.
One of the major impacts of climate change on terrestrial ecosystems is tree mortality events caused by drought and warmer temperature, often exacerbated by pests and pathogens (Allen et al., 2010, 2015; IPCC et al., 2014). Tree mortality impacts ecosystem services provided by forests, including carbon storage that helps offset warming impacts of emissions (Bonan, 2008; Kurz et al., 2008; Breshears et al., 2011; Anderegg et al., 2013; Ma et al., 2013). Tree mortality events can cause ecosystem state changes (Cobb et al., 2017) and, if extensive enough, can influence not only local climate but also climate and associated vegetation elsewhere—termed ecoclimate teleconnections (Garcia et al., 2016; Stark et al., 2016; Swann et al., 2018).
Recent reviews of rapidly expanding literature on tree mortality related to drought and warming include: compilation of observational case studies globally (Allen et al., 2010, updated sequentially in IPCC et al., 2014; Allen et al., 2015; Hartmann et al., 2018); physiological responses of plants (McDowell et al., 2008, 2011; Choat et al., 2012, 2018) including a synthesis specific to experimental results (Adams et al., 2017b) and one specific to tree functional traits (O'Brien et al., 2017); drought-insect interactions (Anderegg et al., 2015); ecological (Anderegg et al., 2013) and hydrological (Adams et al., 2012) consequences of tree die-off events; and dynamics and management options post die-off (Cobb et al., 2017).
One of the most important genera in terms of extent (Richardson and Rundel, 1998) and commercial value in forestry (LeMaitre, 1998) is Pinus, and the most studied species globally of Pinus relative to drought-related mortality is the piñon pine Pinus edulis (Allen et al., 2015; Meddens et al., 2015; Adams et al., 2017b). This species has been studied with respect to most key categories related to mortality (Allen et al., 2015). In this article, we summarize metrics and modifiers associated with mortality driven by drought and warming for P. edulis, explicitly describe the relevant spatial-temporal scales for each, relate these to other results for Pinus, and highlight their potential utility for other genera and for near-term ecological forecasting—where predictions are made iteratively and publicly shared and then tested by subsequent observations, updating the predictions as new information becomes available and factoring lessons learned back into predictions (Clark et al., 2001; Dietze, 2017; Dietze et al., 2018). This review focuses on compiling the broad set of relevant metrics related to mortality for P. edulis, complementing that of Meddens et al. (2015), which focused on meta-analysis of different physiographic and biotic drivers of mortality.
We compiled metrics associated with drought- and warming-related mortality that were developed for P. edulis or for which P. edulis was a key species used in a calculation or prediction (Table 1). These metrics were grouped into: (i) those driven solely by climate variables, (ii) physiological responses, and (iii) those that require multi-step calculations and modeling. The spatial-temporal scale of each of these metrics was explicitly identified (Figure 1). We also compiled factors shown to modify P. edulis mortality (Table 1). Many of these metrics and modifiers are related to one another directly or indirectly, but we group them together only if they use the same predictor variables or are complex ecosystem models requiring multiple inputs. A key figure illustrating each is provided in Supplementary Table S1. Many of these metrics are associated with the early 2000s drought in the Southwestern US (Breshears et al., 2005) and may be overly tied to those specific drought conditions. Important characteristics of the 2000s drought were that it was almost as severe as the 1950s drought in terms of low precipitation (with the 1950s drought being the worst drought in Southwest USA since the 1500s), but it was also warmer (Breshears et al., 2005)—the consequences of which become more evident when Vapor Pressure Deficit (VPD) is considered (Weiss et al., 2009). Importantly, the warmer conditions associated with the 2000s drought are expected to be somewhat indicative of future drought (Breshears et al., 2005; Allen et al., 2015). Time series of plant water potential and soil moisture pre-drought and through mortality provide additional details about this event (Breshears et al., 2005, 2009a,b). Also included are diverse experimental results (Adams et al., 2009, 2017a; Plaut et al., 2012; Krofcheck et al., 2014; Pangle et al., 2015).
Table 1. Compilation of key published metrics to predict Pinus edulis mortality (upper portion), which can be used in near-term ecological forecasting, followed by modifiers (lower portion) which need to be used in conjunction with additional information.
Figure 1. Spatial and temporal scale of each key metric (see section Metrics Associated With Mortality Driven by Drought and Warming for Pinus edulis) to predict tree mortality. Spatial scale of each metric is based on the spatial scale at which that metric was developed. The temporal scale of each metric is based on the frequency at which the metric can be updated and used for near-term ecological forecasting. Pattern denotes the metric category as climate (section Metrics Driven Primarily by Climatic Variables), ecophysiology (ecohydrological and physiological thresholds; section Metrics Based on Direct Ecohydrological or Physiological Thresholds) or integrative models (section Metrics Based on Multi-step Modeling of Climate, Ecohydrology, and/or Ecophysiology). “*” indicates long-term (15+ years) temporal data is needed for the predictions.
SPEI includes both a precipitation input component and an evaporative demand component (Vicente-Serrano et al., 2010), a common theme among some of the metrics. After considering all possible months of the year to begin in and SPEI durations of 1–24 mo, SPEI with a duration of 11 months starting in July was found to be most strongly positively correlated with P. edulis and P. ponderosa growth, with SPEI below −1.64 identified as a threshold to trigger mortality (Huang et al., 2015).
FDSI is an annual index that includes winter-spring precipitation and vapor pressure deficit during the early summer of the current year and the late summer of the year prior, and is standardized by applying a ratio of the current conditions to the long-term mean (Williams et al., 2013). FDSI strongly correlates to regional trends of tree growth and regional patterns of drought-related mortality agents, including bark beetle outbreaks and area burned by tree-killing wildfire. A FDSI ≤ −1.41 is thought to have resulted in widespread mortality (Williams et al., 2013), based on FDSI during the driest half of years during Southwest USA “megadrought” events (Swetnam and Betancourt, 1998).
Field observations of P. edulis mortality across central NM, USA in response to the 2000s drought were highly variable with two thresholds for mortality identified: sites with 2-year precipitation > 600 mm or for warm season (May-August) mean VPD over 2 years of < 1.7 kPa, had little to no mortality (< 10%), whereas at sites with < 600 mm or > 1.7 kPa, plant mortality was highly variable (0% to ~100%; Clifford et al., 2013).
A new type of bioclimatic envelope that focuses exclusively on mortality events for P. edulis was developed for saplings and small reproductively mature sized trees, based largely on climate manipulation experiments that varied precipitation and temperature (Law et al., in press). This bioclimatic envelope estimates a boundary between survival and mortality as a function of length of a dry period and growing season temperature. It indicates that at warmer temperatures (or greater VPD), the duration that P. edulis can survive is reduced.
Plant water stress as reflected in more negative plant water potential, usually measured at the twig scale for P. edulis, has been used to identify threshold values at which tree mortality occurs (Sperry et al., 1988; McDowell et al., 2008) and can be measured in the field. Although the plant water potential for stomatal closure varies somewhat (Breshears et al., 2009b), values associated with greater stress may be more variable among sites (Linton et al., 1998; West et al., 2007; Koepke and Kolb, 2013). A review of experimental studies of drought found that this metric occurred in association with mortality of every tree species studied (Adams et al., 2017b).
Increased stress associated with more negative plant water potential is also associated with loss in conductivity due to embolism intrusion, usually measured in stems in the lab, leading to disruptions of the hydraulic water column that can lead to mortality (Sperry et al., 1988; McDowell et al., 2008). Empirical and theoretical models suggest that this tightly coupled relationship accurately estimates loss of conductivity across species spanning an isohydry-anisohydry gradient (Cochard, 1992; Linton et al., 1998). A recent meta-analysis determined a key threshold of 60% PLC as a mortality tipping point (Adams et al., 2017b), for which site-specific relationships to P. edulis predawn water potential can be developed prior to drought (Linton et al., 1998; West et al., 2007; Koepke and Kolb, 2013).
Long-term soil moisture data obtained by neutron probe measurements that extended below the topsoil and into tuff bedrock (Breshears et al., 2005, 2009a) were adjusted for soil texture to estimate thresholds at which soil moisture above bedrock tuff becomes relatively unavailable to plants. During the 2000s drought, soil moisture above the bedrock tuff was below an availability threshold for 14 consecutive months, during which time tree mortality occurred (Breshears et al., 2009a).
For relatively more isohydric species, such as P. edulis, trees close stomata at a given level of water stress and then attempt to survive the duration of the drought, paying respiration costs during that period. Predawn plant water potential for P. edulis at the same site as Metric 7 was < -2.2 MPa, the point of stomatal closure (Lajtha and Barnes, 1991), for 10 consecutive months preceding tree mortality (Breshears et al., 2009b). Similarly, a field experiment removing 50% of ambient precipitation resulted in P. edulis mortality after 7 consecutive months of near zero conductance (Plaut et al., 2012).
Multispectral assessments of whole-ecosystem responses of post- die-off can detect P. edulis die-off (e.g., Breshears et al., 2005; Rich et al., 2008; Huang et al., 2010; Krofcheck et al., 2014). Further, multispectral data for P. edulis needles alone revealed strong correlations between either plant water content or plant water potential with each of 5 multispectral indices for needles spanning healthy through dead (Stimson et al., 2005).
Using climate data, extended species distribution modeling was applied to assess whether P. edulis mortality during drought was greater in areas with lower historical climatic suitability (HCS; i.e., species distribution modeling using long-term average climate conditions) or with lower climatic suitability during a multi-year drought [episodic climatic suitability, ECS) (Lloret and Kitzberger, 2018). Highest mortality was found in areas with both high HCS and low ECS, suggesting trees have acclimated to the conditions historically experienced and are thus most sensitive to abrupt changes in climate.
Models based on plant ecophysiology have been developed to predict mortality based on known detailed physiological relationships (i.e., stomatal responses to limited water availability) of P. edulis (McDowell et al., 2008) coupled with information on temperature, drought intensity/duration and the role of biotic agents (hydraulic aspects of mortality, drawing on Sperry et al., 1988 are reviewed in Choat et al., 2018; see Adams et al., 2013 for carbohydrate results for P. edulis). The interactions among these specific drivers can push plants to mortality via combinations of carbon starvation, hydraulic failure, and/or pests and pathogens (McDowell et al., 2011; Anderegg et al., 2015).
Regional P. edulis mortality projections among three ecosystem models all predicted widespread die-off, after verifying the ability of each to reproduce predawn water potential accurately (McDowell et al., 2016 and references therein): (1) TREES, a dynamic ecosystem model of water and carbon flows, plant water balance and cavitation was coupled with stomatal conductance, photosynthesis, and evaporation (Mackay et al., 2003, 2010; Samanta et al., 2007; Loranty et al., 2010); (2) MuSICA, a multilayer, multi-leaf process-based biosphere-atmosphere exchange model, included detailed root water uptake, plant water storage dynamics, soil water hydraulic redistribution, root cavitation, and plant NSC storage dynamics (Ogée et al., 2003); and (3) ED(X), which tracks cohorts of trees based on their sizes, simulated tree mortality of cohorts based on carbon starvation and hydraulic failure, accounting for plant water storage and hydraulic conductivity (Moorcroft et al., 2001 with modifications described by Fisher et al., 2010; McDowell et al., 2013; and Xu et al., 2013). All three models used a critical mortality threshold of growing season predawn plant water potential associated with stomatal closure (−2.4 MPa) derived from a field experiment (Pangle et al., 2012; SI 5 in McDowell et al., 2016).
We also identified four categories of “modifiers” that influence the likelihood of P. edulis mortality (drought properties, soil properties, tree phentoype and genotype, and biotic interactions) that differ from “metrics” in that they cannot be used to independently estimate mortality without additional factors, nor can they be iteratively updated for near-term ecological forecasting.
The first modifier focuses on how temperature explicitly drives mortality. Pinus edulis was the first species for which warmer conditions during drought were shown to hasten tree mortality for a reproductively mature-sized tree (Adams et al., 2009). Further, P. edulis seedlings exhibited a similar slope in hastening of time-to-mortality of ~5% per °C increase in temperature across a wide range of temperatures (Adams et al., 2017a).
Spatial patterns of P. edulis mortality in response to the 1950s drought were a function of a topographic moisture index and elevation, with increased mortality at drier and lower sites (Allen, 1989; additional details in Allen and Breshears, 1998). Slope aspect and position influenced mortality in piñon-juniper woodlands in general, but the effects of elevation were mixed (Meddens et al., 2015).
Using publicly available soil data (SSURGO; scale of 1:20,000), P. edulis stands had greater mortality during the 2002–2003 drought where soil AWC (calculated based on soil texture and depth) was < 100 mm (Peterman et al., 2013). However, a smaller-scale study conducted in NM found no relationship between soil AWC and P. edulis mortality (Clifford et al., 2013), suggesting this metric may be more applicable across large geographic areas that vary greatly in soil AWC.
P. edulis mortality from the 2002–2003 drought was greater on soil parent material derived from volcanic cinder than from flow basalt or sedimentary substrate (Koepke et al., 2010).
Physical characteristics like size are expected to be modifiers of mortality (Bennett et al., 2015; McDowell and Allen, 2015). Larger diameter P. edulis trees have experienced greater levels of drought-related mortality (Floyd et al., 2009; Meddens et al., 2015), likely due to combinations of bark beetle selectivity for large diameter trees (Santos and Whitham, 2010; Gaylord et al., 2013), a greater vulnerability of taller trees to hydraulic failure (McDowell and Allen, 2015), and carbon starvation from increased metabolic demands (Mueller et al., 2005).
Phenotypic plasticity, including climate-induced variability in leaf area, sapwood area (Limousin et al., 2015), and tree-ring growth (Williams et al., 2013) can result in structural overshoot risks during rapid transitions from wet to dry periods (Jump et al., 2017), while the duration and sequencing of good and bad growth years affect both short- and long-term tree mortality risk (Ogle et al., 2000; Macalady and Bugmann, 2014).
Evaluating the 1950s, 1996, and the 2000s droughts, the best predictors for growth-mortality models of P. edulis included long-term (10–30 year) average growth rate combined with a metric of growth variability from the past 15 years and the number of abrupt growth increases over the past 10 years (Ogle et al., 2000; Macalady and Bugmann, 2014).
Investment in resin ducts represent a proxy for defense against insects and may vary in response to tree size and age dynamics. P. edulis trees that produce smaller and/or fewer resin ducts are more likely to die during drought (Kläy, 2011; Gaylord et al., 2013, 2015).
A study of P. edulis mortality during drought for trees that exhibited genetically-based resistance or susceptibility to the moth Dioryctria albovittella found that drought-related mortality of trees resistant to the moth was three times higher than for moth-susceptible trees (Sthultz et al., 2009).
The effects of stand tree density (i.e., both intra- and inter-specific competition) on P. edulis mortality are mixed, with more studies not detecting density effects (Meddens et al., 2015). A study on juvenile P. edulis survival during drought found that mortality of juveniles located in the canopy interspace of overstory trees and shrubs was greater in areas with higher grass cover (Redmond et al., 2015).
Adult trees in piñon-juniper woodlands provide substantial shading below tree canopies and as a function of the overall tree density (Royer et al., 2010, 2011). Facilitation by adults increases the mortality threshold to more extreme conditions relative to non-facilitated plants (Sthultz et al., 2007; Redmond et al., 2015).
Bark beetle (Ips confusus) outbreaks are usually associated with field observations and experiments of P. edulis mortality (Meddens et al., 2015). Note, however, that P. edulis mortality during drought has occurred in the absence of bark beetles (Mueller et al., 2005) and controlled experiments quantify rates of mortality caused by drought in the absence of bark beetles (Adams et al., 2009, 2017a; Anderegg and Anderegg, 2013).
The metrics and modifiers for P. edulis mortality share consistencies with other species in the Pinus genus. Several of the above metrics and modifiers (e.g., Metrics 1–2 and Modifier 1) were also predictive of P. ponderosa mortality. For instance, the increase of hastening in time-to-mortality for P. edulis of ~5% per °C also applies to P. ponderosa, as does the linear relationship of this response to a wide range of warming during drought (Adams et al., 2017b). Mortality of five European species of Pinus depended on warming and water limitation (Matias et al., 2017), consistent with the climate metrics for P. edulis, which include both a warming or evaporative demand component and a precipitation component; these species also differed in sensitivity between montane and lowland sites. Other Pinus species have similar ecophysiological characteristics as P. edulis (Olson et al., 2018) and are also vulnerable to bark beetles, particularly when under water stress (Anderegg et al., 2015). The effect of elevated CO2 on mortality has not been studied for P. edulis, but results for P. radiata found it did not extend time-to-mortality (Duan et al., 2015).
There are more studies of drought-related mortality for P. edulis than for any other tree species, yet the many interrelated and often untested metrics and modifiers indicate a need to determine which are the most robust, accounting for tradeoffs between robustness and data or computational requirements. Expected future mortality events will provide an opportunity to observationally and experimentally test and compare these metrics related to tree mortality for P. edulis via near-term ecological forecasting (Clark et al., 2001; Dietze, 2017; Dietze et al., 2018). Given that many current projections of P. edulis mortality predict extensive mortality in coming decades (e.g., Adams et al., 2009, 2017a; Williams et al., 2013; McDowell et al., 2016), we need to test these metrics and modifiers with upcoming droughts. These metrics and modifiers reinforce that Pinus, a widely distributed and commercially important genus, is likely to be sensitive to future hotter drought. These metrics also serve as potential analogs for species in other genera or trait groups. Improving predictions of tree mortality will be increasingly important in moving toward anticipatory management under warming climate (Bradford et al., 2018).
DB provided a first rough draft of the synthesis table and the manuscript. All authors contributed to substantial revision and identifying and summarizing key metrics and modifiers, as well as editing and refinement of the text, tables and figure.
This work was supported primarily by NSF DEB-1833529 to Colorado State University, DEB-1833502 to University of Arizona, and DEB-1833505 to Northern Arizona University. Additional support was provided by EF-1340624, EF-1550756, EAR-1331408, and EAR-1659546, and OISE-1748275 and OISE-1748204 for Near-Term Ecological Forecasting workshop participation.
The authors declare that the research was conducted in the absence of any commercial or financial relationships that could be construed as a potential conflict of interest.
We thank R. E. Gallery and M. C. Dietze for including DB in a Near-Term Ecological Forecasting workshop that inspired that aspect of this project, and H. D. Adams for feedback on specific points in the manuscript.
The Supplementary Material for this article can be found online at: https://www.frontiersin.org/articles/10.3389/ffgc.2018.00004/full#supplementary-material
Adams, H. D., Barron-Gafford, G. A., Minor, R. L., Gardea, A. A., Bentley, L. P., Law, D. J., et al. (2017a). Temperature response surfaces for mortality risk of tree species with future drought. Environ. Res. Lett. 12:115014. doi: 10.1088/1748-9326/aa93be
Adams, H. D., Germino, M. J., Breshears, D. D., Barron-Gafford, G. A., Guardiola-Claramonte, M., Zou, C. B., et al. (2013). Nonstructural leaf carbohydrate dynamics of Pinus edulis during drought-induced tree mortality reveal role for carbon metabolism in mortality mechanism. New Phytol. 197, 1142–1151. doi: 10.1111/nph.12102
Adams, H. D., Guardiola-Claramonte, M., Barron-Gafford, G. A., Villegas, J. C., Breshears, D. D., Zou, C. B., et al. (2009). Temperature sensitivity of drought-induced tree mortality portends increased regional die-off under global-change-type drought. Proc. Natl. Acad. Sci. U.S.A. 106, 7063–7066. doi: 10.1073/pnas.0901438106
Adams, H. D., Luce, C. H., Breshears, D. D., Allen, C. D., Weiler, M., Hale, V. C., et al. (2012). Ecohydrological consequences of drought-and infestation-triggered tree die-off: insights and hypotheses. Ecohydrology 5, 145–159. doi: 10.1002/eco.233
Adams, H. D., Zeppel, M. J. B., Anderegg, W. R. L., Hartmann, H., Landhäusser, S. M., Tissue, D. T., et al. (2017b). A multi-species synthesis of physiological mechanisms in drought-induced tree mortality. Nat. Ecol. Evol. 1, 1285–1291. doi: 10.1038/s41559-017-0248-x
Allen, C. D. (1989). Changes in the Landscape of the Jemez Mountains, New Mexico. Dissertation, University of California, Berkeley (Berkeley, CA).
Allen, C. D., and Breshears, D. D. (1998). Drought-induced shift of a forest-woodland ecotone: rapid landscape response to climate variation. Proc. Natl. Acad. Sci. U.S.A. 95, 14839–14842. doi: 10.1073/pnas.95.25.14839
Allen, C. D., Breshears, D. D., and McDowell, N. G. (2015). On underestimation of global vulnerability to tree mortality and forest die-off from hotter drought in the Anthropocene. Ecosphere 6, 1–55. doi: 10.1890/ES15-00203.1
Allen, C. D., Macalady, A. K., Chenchouni, H., Bachelet, D., McDowell, N., Vennetier, M., et al. (2010). A global overview of drought and heat-induced tree mortality reveals emerging climate change risks for forests. Forest Ecol. Manag. 259, 660–684. doi: 10.1016/j.foreco.2009.09.001
Anderegg, W. R. L., and Anderegg, L. D. L. (2013). Hydraulic and carbohydrate changes in experimental drought-induced mortality of saplings in two conifer species. Tree Physiol. 33, 252–260. doi: 10.1093/treephys/tpt016
Anderegg, W. R. L., Hicke, J. A., Fisher, R. A., Allen, C. D., Aukema, J., and Bentz, B. (2015). Tree mortality from drought, insects, and their interactions in a changing climate. New Phytol. 208, 674–683. doi: 10.1111/nph.13477
Anderegg, W. R. L., Kane, J. M., and Anderegg, L. D. L. (2013). Consequences of widespread tree mortality triggered by drought and temperature stress. Nat. Clim. Change 3, 30–36. doi: 10.1038/nclimate1635
Bennett, A. C., McDowell, N. G., Allen, C. D., and Anderson-Teixeira, K. J. (2015). Larger trees suffer most during drought in forests worldwide. Nat. Plants 1:15139. doi: 10.1038/nplants.2015.139
Bonan, G. B. (2008). Forests and climate change: Forcings, feedbacks, and the climate benefits of forests. Science 320, 1444–1449. doi: 10.1126/science.1155121
Bradford, J. B., Betancourt, J. L., Butterfield, B. J., Munson, S. M., and Wood, T. E. (2018). Anticipatory natural resource science and management for a changing future. Front. Ecol. Environ. 16, 295–303. doi: 10.1002/fee.1806
Breshears, D. D., Cobb, N. S., Rich, P. M., Price, K. P., Allen, C. D., Balice, R. G., et al. (2005). Regional vegetation die-off in response to global-change-type drought. Proc. Natl. Acad. Sci. U.S.A. 102, 15144–15148. doi: 10.1073/pnas.0505734102
Breshears, D. D., López-Hoffman, L., and Graumlich, L. J. (2011). When ecosystem services crash: preparing for big, fast, patchy climate change. Ambio 40, 256–263. doi: 10.1007/s13280-010-0106-4
Breshears, D. D., Myers, O. B., and Barnes, F. J. (2009a). Horizontal heterogeneity in the frequency of plant-available water with woodland intercanopy-canopy vegetation patch type rivals that occurring vertically by soil depth. Ecohydrology 2, 503–519. doi: 10.1002/eco.75
Breshears, D. D., Myers, O. B., Meyer, C. W., Barnes, F. J., Zou, C. B., Allen, C. D., et al. (2009b). Tree die-off in response to global change-type drought: mortality insights from a decade of plant water potential measurements. Front. Ecol. Environ. 7, 185–189. doi: 10.1890/080016
Choat, B., Brodribb, T. J., Brodersen, C. R., Duursma, R. A., López, R., and Medlyn, B. E. (2018). Triggers of tree mortality under drought. Nature 558, 531–539. doi: 10.1038/s41586-018-0240-x
Choat, B., Jansen, S., Brodribb, T. J., Cochard, H., Delzon, S., Bhaskar, R., et al. (2012). Global convergence in the vulnerability of forests to drought. Nature 491, 752. doi: 10.1038/nature11688
Clark, J. S., Carpenter, S. R., Barber, M., Collins, S., Dobson, A., Foley, J. A., et al. (2001). Ecological forecasts: an emerging imperative. Science 293, 657–660. doi: 10.1126/science.293.5530.657
Clifford, M. J., Royer, P. D., Cobb, N. S., Breshears, D. D., and Ford, P. L. (2013). Precipitation thresholds and drought-induced tree die-off: insights from patterns of Pinus edulis mortality along an environmental stress gradient. New Phytol. 200, 413–421. doi: 10.1111/nph.12362
Cobb, R. C., Ruthrof, K. X., Breshears, D. D., Lloret, F., Aakala, T., Adams, H. D., et al. (2017). Ecosystem dynamics and management after forest die-off: a global synthesis with conceptual state-and-transition models. Ecosphere 8:e02034. doi: 10.1002/ecs2.2034
Cochard, H. (1992). Vulnerability of several conifers to air embolism. Tree Physiol. 11, 73–83. doi: 10.1093/treephys/11.1.73
Dietze, M. C. (2017). Ecological Forecasting. Princeton, NJ: Princeton University Press. doi: 10.1515/9781400885459
Dietze, M. C., Fox, A., Beck-Johnson, L. M., Betancourt, J. L., Hooten, M. B., Jarnevich, C. S., et al. (2018). Iterative near-term ecological forecasting: needs, opportunities, and challenges. Proc. Natl. Acad. Sci. U.S.A. 115, 1424–1432. doi: 10.1073/pnas.1710231115
Duan, H., O'Grady, A. P., Duursma, R. A., Choat, B., Huang, G., Smith, R. A., et al. (2015). Drought responses of two gymnosperm species with contrasting stomatal regulation strategies under elevated [CO2] and temperature. Tree Physiol. 35, 756–770. doi: 10.1093/treephys/tpv047
Fisher, R., McDowell, N. G., Purves, D., Moorcroft, P., Sitch, S., Cox, P., et al. (2010). Assessing uncertainties in a second-generation dynamic vegetation model due to ecological scale limitations. New Phytol. 187, 666–681. doi: 10.1111/j.1469-8137.2010.03340.x
Floyd, M. L., Clifford, M., Cobb, N. S., Hanna, D., Delph, R., Ford, P., et al. (2009). Relationship of stand characteristics to drought-induced mortality in three Southwestern piñion–juniper woodlands. Ecol. Appl. 19, 1223–1230. doi: 10.1890/08-1265.1
Garcia, E. S., Swann, A. L. S., Villegas, J. C., Breshears, D. D., Law, D. J., Saleska, S. R., et al. (2016). Synergistic ecoclimate teleconnections from forest loss in different regions structure global ecological responses. PLoS ONE 11:e0165042. doi: 10.1371/journal.pone.0165042
Gaylord, M. L., Kolb, T. E., and McDowell, N. G. (2015). Mechanisms of piñon pine mortality after severe drought: a retrospective study of mature trees. Tree Physiol. 35, 806–816. doi: 10.1093/treephys/tpv038
Gaylord, M. L., Kolb, T. E., Pockman, W. T., Plaut, J. A., Yepez, E. A., Macalady, A. K., et al. (2013). Drought predisposes piñon-juniper woodlands to insect attacks and mortality. New Phytol. 198, 567–578. doi: 10.1111/nph.12174
Hartmann, H., Moura, C. F., Anderegg, W. R. L., Ruehr, N. K., Salmon, Y., Allen, C. D., et al. (2018). Research frontiers for improving our understanding of drought-induced tree and forest mortality. New Phytol. 218, 15–28. doi: 10.1111/nph.15048
Huang, C. Y., Asner, G. P., Barger, N. N., Neff, J. C., and Floyd, M. L. (2010). Regional aboveground live carbon losses due to drought-induced tree dieback in piñon-juniper ecosystems. Remote Sens. Environ. 114, 1471–1479. doi: 10.1016/j.rse.2010.02.003
Huang, K., Yi, C., Wu, D., Zhou, T., Zhao, X., Blanford, W. J., et al. (2015). Tipping point of a conifer forest ecosystem under severe drought. Environ. Res. Lett. 10:024011. doi: 10.1088/1748-9326/10/2/024011
IPCC 2014, Settele, J., Betts, R., Bunn, S., Leadley, P., Nepstad, D., et al. (2014). “Terrestrial and inland water systems.” in Climate Change 2014: Impacts,Adaptation, and Vulnerability. Part A: Global and Sectoral Aspects. Contribution of Working Group II to the Fifth Assessment Report of the Intergovernmental Panel on Climate Change, eds C. B. Field, V. R. Barros, D. J. Dokken, K. J. Mach, M. D. Mastrandrea, T. E. Bilir, M. Chatterjee, K. L. Ebi, Y. O. Estrada, R. C. Genova, B. Girma, E. S. Kissel, A. N. Levy, S. MacCracken, P. R. Mastrandrea, L. L. White (Cambridge, UK; New York, NY: Cambridge University Press), 271–359.
Jump, A. S., Ruiz-Benito, P., Greenwood, S., Allen, C. D., Kitzberger, T., Fensham, R., et al. (2017). Structural overshoot of tree growth with climate variability and the global spectrum of drought-induced forest dieback. Glob. Change Biol 23, 3742–3757. doi: 10.1111/gcb.13636
Kläy, M. (2011). Are Defensive Structures Good Predictors of Tree Mortality Under Drought and Insect Pressure? Master's Thesis, Zürich, Switzerland: Swiss Federal Institute of Technology in Zurich.
Koepke, D. F., and Kolb, T. E. (2013). Species variation in water relations and xylem vulnerability to cavitation at a forest-woodland ecotone. Forest Sci. 59, 524–535. doi: 10.5849/forsci.12-053
Koepke, D. F., Kolb, T. E., and Adams, H. D. (2010). Variation in woody plant mortality and dieback from severe drought among soils, plant groups, and species within a northern Arizona ecotone. Oecologia 163, 1079–1090. doi: 10.1007/s00442-010-1671-8
Krofcheck, D. J., Eitel, J. U., Vierling, L. A., Schulthess, U., Hilton, T. M., Dettweiler-Robinson, E., et al. (2014). Detecting mortality induced structural and functional changes in a pi-on-juniper woodland using Landsat and RapidEye time series. Remote Sens. Environ. 151, 102–113. doi: 10.1016/j.rse.2013.11.009
Kurz, W. A., Dymond, C. C., Stinson, G., Rampley, G. J., Neilson, E. T., Carroll, A. L., et al. (2008). Mountain pine beetle and forest carbon feedback to climate change. Nature 452, 987–990. doi: 10.1038/nature06777
Lajtha, K., and Barnes, F. J. (1991). Carbon gain and water-use in pinyon pine-juniper woodlands of northern New Mexico—field versus phytotron chamber measurements. Tree Phsiol. 9, 59–67. doi: 10.1093/treephys/9.1-2.59
Law, D. J., Adams, H. D., Breshears, D. D., Cobb, N. S., Bradford, J. B., Zou, C. B. (in press). Expanded use of environmental extremes versus trends in bioclimatic modeling to predict individual demographic events. Int. J. Plant Sci. 180., et al.
LeMaitre, D. C. (1998). “Pines in cultivation: a global view,” in Ecology and Biogeography of Pinus, ed D. M. Richardson (New York, NY: Cambridge University Press), 407–431.
Limousin, J., Yepez, E. A., McDowell, N. G., and Pockman, W. T. (2015). Convergence in resource use efficiency across trees with differing hydraulic strategies in response to ecosystem precipitation manipulation. Funct. Ecol. 29, 1125–1136. doi: 10.1111/1365-2435.12426
Linton, M. J., Sperry, J. S., and Williams, D. G. (1998). Limits to water transport in Juniperus osteosperma and Pinus edulis: implications for drought tolerance and regulation of transpiration. Funct. Ecol. 12, 906–911. doi: 10.1046/j.1365-2435.1998.00275.x
Lloret, F., and Kitzberger, T. (2018). Historical and event-based bioclimatic suitability predicts regional forest vulnerability to compound effects of severe drought and bark beetle infestation. Glob. Change Biol. 24, 1952–1964. doi: 10.1111/gcb.14039
Loranty, M. M., Mackay, D. S., Ewers, B. E., Traver, E., and Kruger, E. L. (2010). Contribution of competition for light to within-species variability in stomatal conductance. Water Resour. Res. 46:W05516. doi: 10.1029/2009WR008125
Ma, D., Notaro, M., Liu, Z., Chen, G., and Liu, Y. (2013). Simulated impacts of afforestation in East China monsoon region as modulated by ocean variability. Clim. Dyn. 41, 2439–2450. doi: 10.1007/s00382-012-1592-9
Macalady, A. K., and Bugmann, H. (2014). Growth-mortality relationships in piñon pine (Pinus edulis) during severe droughts of the past century: shifting processes in space and time. PLoS ONE 9:e92770. doi: 10.1371/journal.pone.0092770
Mackay, D. S., Ahl, D. E., Ewers, B. E., Samanta, S., Gower, S. T., and Burrows, S. N. (2003). Physiological tradeoffs in the parameterization of a model of canopy transpiration. Adv. Water Resour. 26, 179–194. doi: 10.1016/S0309-1708(02)00090-8
Mackay, D. S., Ewers, B. E., Loranty, M. M., and Kruger, E. L. (2010). On the representativeness of plot size and location for scaling transpiration from trees to a stand. J. Geophys. Res. Biogeosci. 115:G02016. doi: 10.1029/2009JG001092
Matias, L., Castro, J., Villar-Salvador, P., Quero, J. L., and Jump, A. S. (2017). Differential impacts of hotter drought on seedling performance of five ecologically distinct pine species. Plant Ecol. 218, 201–212. doi: 10.1007/s11258-016-0677-7
McDowell, N., Pockman, W. T., Allen, C. D., Breshears, D. D., Cobb, N., Kolb, T., et al. (2008). Mechanisms of plant survival and mortality during drought: why do some plants survive while others succumb to drought? New Phytol. 178, 719–739. doi: 10.1111/j.1469-8137.2008.02436.x
McDowell, N. G., and Allen, C. D. (2015). Darcy's law predicts widespread forest mortality under climate warming. Nat. Clim. Change 5, 669–672. doi: 10.1038/nclimate2641
McDowell, N. G., Beerling, D. J., Breshears, D. D., Fisher, R. A., Raffa, K. F., and Stitt, M. (2011). The interdependence of mechanisms underlying climate-driven vegetation mortality. Trends in Ecol. and Evol. 26, 523–532. doi: 10.1016/j.tree.2011.06.003
McDowell, N. G., Fisher, R. A., Xu, C. G., Domec, J. C., Holtta, T., Mackay, D. S., et al. (2013). Evaluating theories of drought-induced vegetation mortality using a multimodel-experiment framework. New Phytol. 200, 304–321. doi: 10.1111/nph.12465
McDowell, N. G., Williams, A. P., Xu, C., Pockman, W. T., Dickman, L. T., Sevanto, S., et al. (2016). Multi-scale predictions of massive conifer mortality due to chronic temperature rise. Nat. Clim. Change 6, 295–300. doi: 10.1038/nclimate2873
Meddens, A. J. H., Hicke, J. A., Macalady, A. K., Buotte, P. C., Cowles, T. R., and Allen, C. D. (2015). Patterns and causes of observed piñon pine mortality in the southwestern United States. New Phytol. 206, 91–97. doi: 10.1111/nph.13193
Moorcroft, P. R., Hurtt, G. C., and Pacala, S. W. (2001). A method for scaling vegetation dynamics: the ecosystem demography model (ED). Ecol. Monogr. 71, 557–586. doi: 10.1890/0012-9615(2001)071[0557:AMFSVD]2.0.CO;2
Mueller, R. C., Scudder, C. M., Porter, M. E., Talbot Trotter, I. I. I., Gehring, C. A., and Whitham, T. G. (2005). Differential tree mortality in response to severe drought: evidence for long-term vegetation shifts. J. Ecol. 93, 1085–1093. doi: 10.1111/j.1365-2745.2005.01042.x
O'Brien, M. J., Engelbrecht, B. M., Joswig, J., Pereyra, G., Schuldt, B., Jansen, S. (2017), A synthesis of tree functional traits related to drought-induced mortality in forests across climatic zones. J Appl. Ecol. 54, 1669–1686. doi: 10.1111/1365-2664.12874, et al.
Ogée, J., Brunet, Y., Loustau, D., Berbigier, P., and Delzon, S. (2003). MuSICA, a CO2, water and energy multi-layer, multi-leaf pine forest model: evaluation from hourly to yearly time scales and sensitivity analysis. Glob. Change Biol. 9, 697–717. doi: 10.1046/j.1365-2486.2003.00628.x
Ogle, K., Whitham, T. G., and Cobb, N. S. (2000). Tree-ring variation in pinyon predicts likelihood of death following severe drought. Ecology 81, 3237–3243. doi: 10.1890/0012-9658(2000)081[3237:TRVIPP]2.0.CO;2
Olson, M. E., Soriano, D., Rosell, J., Anfodillo, T., Donoghue, M. J., Edwards, E. J., et al. (2018). Plant height and hydraulic vulnerability to drought and cold. Proc. Natl. Acad. Sci. U.S.A. 115, 7551–7556. doi: 10.1073/pnas.1721728115
Pangle, R. E., Hill, J. P., Plaut, J. A., Yepez, E. A., Elliot, J. R., Gehres, N., et al. (2012). Methodology and performance of a rainfall manipulation experiment in a piñon–juniper woodland. Ecosphere 3:28. doi: 10.1890/ES11-00369.1
Pangle, R. E., Limousin, J. M., Plaut, J. A., Yepez, E. A., Hudson, P. J., Boutz, A. L., et al. (2015). Prolonged experimental drought reduces plant hydraulic conductance and transpiration and increases mortality in a piñon-juniper woodland. Ecol. and Evol. 5, 1618–1638. doi: 10.1002/ece3.1422
Peterman, W., Waring, R. H., Seager, T., and Pollock, W. L. (2013). Soil properties affect pinyon pine–juniper response to drought. Ecohydrology 6, 455–463. doi: 10.1002/eco.1284
Plaut, J. A., Yepez, E. A., Hill, J., Pangle, R., Sperry, J. S., Pockman, W. T., et al. (2012). Hydraulic limits preceding mortality in a piñon-juniper woodland under experimental drought. Plant Cell Environ. 35, 1601–1617. doi: 10.1111/j.1365-3040.2012.02512.x
Redmond, M. D., Cobb, N. S., Clifford, M. J., and Barger, N. N. (2015). Woodland recovery following drought-induced tree mortality across an environmental stress gradient. Glob. Change Biol. 21, 3685–3695. doi: 10.1111/gcb.12976
Rich, P. M., Breshears, D. D., and White, A. B. (2008). Phenology Of mixed woody–herbaceous ecosystems following extreme events: net and differential responses. Ecology 89, 342–352. doi: 10.1890/06-2137.1
Richardson, D. M. (1998). “Ecology and biogeography of Pinus: an introduction,” in Ecology and Biogeography of Pinus, ed D. M. Richardson (New York, NY: Cambridge University Press) 3–46.
Royer, P. D., Breshears, D. D., Zou, C. B., Cobb, N. S., and Kurc, S. A. (2010). Ecohydrological energy inputs in semiarid coniferous gradients: responses to management- and drought-induced tree reductions. Forest Ecol. Manag. 260, 1646–1655. doi: 10.1016/j.foreco.2010.07.036
Royer, P. D., Cobb, N. S., Clifford, M. J., Huang, C. Y., Breshears, D. D., Adams, H. D., et al. (2011). Extreme climatic event-triggered overstorey vegetation loss increases understorey solar input regionally: primary and secondary ecological implications. J. Ecol. 99, 714–723. doi: 10.1111/j.1365-2745.2011.01804.x
Samanta, S., Mackay, D. S., Clayton, M. K., Kruger, E. L., and Ewers, B. E. (2007). Bayesian analysis for uncertainty estimation of a canopy transpiration model. Water Resour. Res. 43:W04424. doi: 10.1029/2006WR005028
Santos, M. J., and Whitham, T. G. (2010). Predictors of Ips confusus outbreaks during a record drought in southwestern USA: implications for monitoring and management. Environ. Manag. 45, 239–249. doi: 10.1007/s00267-009-9413-6
Sperry, J. S., Donnelly, J. R., and Tyree, M. T. (1988). A method for measuring hydraulic conductivity and embolism in xylem. Plant Cell Environ. 11, 35–40. doi: 10.1111/j.1365-3040.1988.tb01774.x
Stark, S. C., Breshears, D. D., Garcia, E. S., Law, D. J., Minor, D. M., Saleska, S. R., et al. (2016). Toward accounting for ecoclimate teleconnections: intra- and inter-continental consequences of altered energy balance after vegetation change. Landscape Ecol. 31, 181–194. doi: 10.1007/s10980-015-0282-5
Sthultz, C. M., Gehring, C. A., and Whitham, T. G. (2007). Shifts from competition to facilitation between a foundation tree and a pioneer shrub across spatial and temporal scales in a semi-arid woodland. New Phytol. 173, 135–145. doi: 10.1111/j.1469-8137.2006.01915.x
Sthultz, C. M., Gehring, C. A., and Whitham, T. G. (2009). Deadly combinations of genes and drought: increased mortality of herbivore-resistant trees in a foundation species. Glob. Change Biol. 15, 1949–1961. doi: 10.1111/j.1365-2486.2009.01901.x
Stimson, H. C., Breshears, D. D., Ustin, S. L., and Kefauver, S. C. (2005). Spectral sensing of foliar water conditions in two co-occurring conifer species: Pinus edulis and Juniperus monosperma. Remote Sens. Environ. 96, 108–145. doi: 10.1016/j.rse.2004.12.007
Swann, A. L. S., Lagu,ë, M. M., Garcia, E. S., Field, J. P., Breshears, D. D., Moore, D. J. P., et al. (2018). Continental-scale consequences of tree die-offs in North America: identifying where forest loss matters most. Environ. Res. Lett. 13:055014. doi: 10.1088/1748-9326/aaba0f
Swetnam, T. W., and Betancourt, J. L. (1998). Mesoscale disturbance and ecological response to decadal climatic variability in the American Southwest. J. Climate. 11, 3128–3147. doi: 10.1175/1520-0442(1998)011 < 3128:MDAERT>2.0.CO;2
Vicente-Serrano, S. M., Beguería, S., and López-Moreno, J. I. (2010). A multiscalar drought index sensitive to global warming: the standardized precipitation evapotranspiration index. J. Climate 23, 1696–1718. doi: 10.1175/2009JCLI2909.1
Weiss, J. L., Castro, C. L., and Overpeck, J. T. (2009). Distinguishing pronounced droughts in the Southwestern United States: seasonality and effects of warmer temperatures. J. Clim. 22, 5918–5932. doi: 10.1175/2009JCLI2905.1
West, A. G., Hultine, K. R., Jackson, T. L., and Ehleringer, J. R. (2007). Differential summer water use by Pinus edulis and Juniperus osteosperma reflects contrasting hydraulic characteristics. Tree Phys. 27, 1711–1720. doi: 10.1093/treephys/27.12.1711
Williams, A. P., Allen, C. D., Macalady, A. K., Griffin, D., Woodhouse, C. A., Meko, D. M., et al. (2013). Temperature as a potent driver of regional forest drought stress and tree mortality. Nat. Clim. Change 3, 292–297. doi: 10.1038/nclimate1693
Keywords: climate change, die-off, drought, mortality, Pinus edulis, tree
Citation: Breshears DD, Carroll CJW, Redmond MD, Wion AP, Allen CD, Cobb NS, Meneses N, Field JP, Wilson LA, Law DJ, McCabe LM and Newell-Bauer O (2018) A Dirty Dozen Ways to Die: Metrics and Modifiers of Mortality Driven by Drought and Warming for a Tree Species. Front. For. Glob. Change 1:4. doi: 10.3389/ffgc.2018.00004
Received: 20 July 2018; Accepted: 06 September 2018;
Published: 26 October 2018.
Edited by:
Jeffrey M. Warren, Oak Ridge National Laboratory (DOE), United StatesReviewed by:
Cate Macinnis-Ng, University of Auckland, New ZealandCopyright © 2018 Breshears, Carroll, Redmond, Wion, Allen, Cobb, Meneses, Field, Wilson, Law, McCabe and Newell-Bauer. This is an open-access article distributed under the terms of the Creative Commons Attribution License (CC BY). The use, distribution or reproduction in other forums is permitted, provided the original author(s) and the copyright owner(s) are credited and that the original publication in this journal is cited, in accordance with accepted academic practice. No use, distribution or reproduction is permitted which does not comply with these terms.
*Correspondence: David D. Breshears, ZGF2ZWJAZW1haWwuYXJpem9uYS5lZHU=
Disclaimer: All claims expressed in this article are solely those of the authors and do not necessarily represent those of their affiliated organizations, or those of the publisher, the editors and the reviewers. Any product that may be evaluated in this article or claim that may be made by its manufacturer is not guaranteed or endorsed by the publisher.
Research integrity at Frontiers
Learn more about the work of our research integrity team to safeguard the quality of each article we publish.