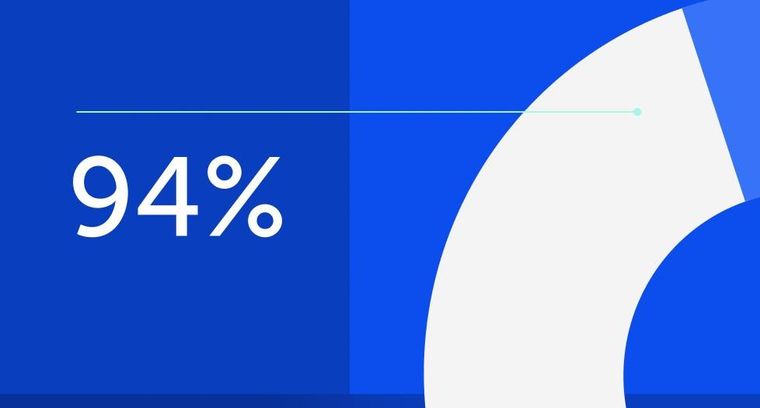
94% of researchers rate our articles as excellent or good
Learn more about the work of our research integrity team to safeguard the quality of each article we publish.
Find out more
ORIGINAL RESEARCH article
Front. Ecol. Evol., 03 April 2025
Sec. Behavioral and Evolutionary Ecology
Volume 13 - 2025 | https://doi.org/10.3389/fevo.2025.1543635
Small mammals inhabiting cold climates face high heat losses and thus, high energy demands for body temperature regulation. However, behavioral adaptations, such as seeking refuge from extreme cold in the subnivean space, can reduce the energetic cost of thermoregulation. Using automated cameras, we monitored collared lemmings (Dicrostonyx groenlandicus) at the northern limit of their range, as they surfaced from their snow burrows in spring, to assess the effect of weather variables on the occurrence of this behavior. We hypothesized that lemmings reduce the energetic cost of thermoregulation when they come to the surface of the snow in spring. As expected, the frequency of surface activity increased with air temperature, but decreased with cloud cover and wind speed. In addition, the operative temperature was higher above the snow than below, while snow profiles showed the absence of liquid water in the snowpack. These findings support that lemming surface activity in spring is a behavioral thermoregulation strategy. However, observations of several predators in the study area, combined with vigilance behavior observed in lemmings at the snow surface, stress the risks associated with such exposure on the snow. We therefore suggest that lemmings may face a trade-off between thermoregulation and predation risk. Given that data on lemming winter ecology are scarce, we also exploited this behavior to gather valuable knowledge on molting phenology and reproduction. Overall, our results provide insight into the complex trade-off between thermoregulation and other needs in small mammals inhabiting cold climates, and highlight some potential implications for arctic ecosystem dynamics based on predator-prey interactions.
Regulating body temperature is essential to all endothermic animals. Such regulation requires energy for heat production or active dissipation (McNab, 2002), but the energetic cost of thermoregulation can be reduced through behavioral adaptations (Terrien et al., 2011). For example, heat or cold-stressed animals can adjust their exposure to solar radiation by using thermal refuges or by sun-basking (Bicca-Marques and Calegaro-Marques, 1998; Long et al., 2005; Warnecke et al., 2007). Thermal refuges can indeed offer very different operative temperatures, that is the fine-scale environmental temperature combining the effects of wind, radiation and air temperature (Gagge, 1940; Bakken et al., 1985), compared to the surrounding environment. Sun-basking is widespread in most taxonomic groups, including birds (Kennedy, 1969; Mueller, 1972) and mammals (e.g. Kelley et al., 2016; Coppola et al., 2019; Flôres et al., 2021), with some species even using body orientation to modulate heat gain from sun radiation (Maloney et al., 2005; Brown and Downs, 2007). Given the limited energy resources available to wild animals (Kirkwood, 1983; Weiner, 1992), minimizing the energetic costs of thermoregulation enables them to allocate more energy towards other essential functions such as foraging, growth, and reproduction (Stearns, 1992; Mathot and Dingemanse, 2015).
However, sunbathing in open areas may increase predation risk. For example, wolverines (Gulo gulo) trade the protection provided by the subnivean space to rest on snow surfaces when weather facilitates thermoregulation, exposing themselves to potential killing attempts by wolves (Canis lupus), conspecifics, and humans (Glass et al., 2021). Conversely, during the snow season, pygmy rabbits (Brachylagus idahoensis) prioritize the protection offered by concealed resting sites over the thermoregulatory benefits offered by open areas (Milling et al., 2017). Whereas the costs of predator avoidance on reproduction or foraging have been extensively studied in endotherms (Brown and Kotler, 2004; Verdolin, 2006; Creel, 2018), the thermoregulatory costs have often been overlooked, especially in mammals (but see Veldhuis et al., 2019).
Thermoregulation is a major part of the daily energy expenditure of all endotherms (McNab, 2002), but due to their high surface-area-to-volume ratio, small mammals living in cold climates face unique challenges to maintain heat balance (Chappell, 1980a; Norris and Kunz, 2012). Collared lemmings (Dicrostonyx groenlandicus) exemplify this heat balance challenge, as they have the northernmost geographic distribution of all small mammals (Jarrel and Fredga, 1993; Gotthardt and McClory, 2006) and can be found in High Arctic polar deserts, where air temperature regularly reaches –40°C in winter. They remain active year-round, and move, forage, build nests, and even breed during the harsh Arctic winters (MacLean et al., 1974; Stenseth and Ims, 1993a). Whereas snow cover provides protection against snow-surface predators like Arctic foxes (Vulpes lagopus) (Duchesne et al., 2011; Bilodeau et al., 2013), lemmings also use subnivean burrows as thermal refuges (Pauli et al., 2013; Berteaux et al., 2017). Snow insulation generally protects them against the coldest air temperatures (Pomeroy and Brun, 2001; Reid et al., 2012), but, as spring progresses, the upper snow layers become warmer than the base layers, reversing the thermal gradient within the snowpack (Domine et al., 2016).
Snow properties, such as depth and density, play an important role in lemming survival and winter breeding success (Korslund and Steen, 2006; Duchesne et al., 2011; Reid et al., 2012), and thus in lemming abundance cycles (Millar, 2001; Bilodeau et al., 2013; Fauteux et al., 2015). The Arctic snowpack typically consists of two main layers: a hard, wind-shaped layer overlaying a soft, friable layer at the base known as the depth hoar (Domine et al., 2002). Collared lemmings dig tunnels in the upper part of the depth hoar (Poirier et al., 2019), benefiting from remarkable adaptations to the subnivean life. This includes seasonal morphological changes such as a pre-winter molt into white fur which improves insulation and camouflage (Hansen, 1959; Reynolds, 1993), and the development of oversized fore claws which facilitate digging into the snow (Fuller et al., 1975; Kays and Wilson, 2009).
As the snow offers a protective cover against predators, it would be expected that lemmings stay under the snow as long as they can, especially when they have turned brown after their spring molt. Yet, observations throughout the circumpolar North, including Barrow in Alaska (Batzli et al., 1980), Wrangel island in Russia (Ehrich et al., 2020; I. E. Menyushina, pers. comm.), and Alert in Canada (D. Berteaux, unpublished data) show that collared lemmings do surface while the snow cover is still intact. This surface activity is surprising given that Dicrostonyx lemmings are exposed to many surface predators, such as the arctic fox and the long-tailed jaeger (Stercorarius longicaudus) (Batzli et al., 1980; Gilg, 2002), and have developed sophisticated adaptations to decrease predation risk, such as the use of underground latrines (Boonstra et al., 1996).
Given the above, our general objective was to explain why Dicrostonyx lemmings surface from snow in May-June, when snow cover is still present but continuous light considerably increases chances of being detected by predators. We hypothesized that lemmings incur thermoregulatory benefits when coming to the snow surface (H1). We first predicted that lemming activity at the surface is positively related to air temperature (P1a), but negatively related to cloud cover (P1b) and wind speed (P1c). A related prediction was that lemming surface activity occurs when operative temperature is higher at the snow surface than at ground level (P1d). In addition, we predicted that once above the snow, lemmings adopt behaviors promoting positive heat transfers from the environment. Specifically, the proportion of time spent stationary should decrease with the increase of cloud cover (P1e), and lemmings should prioritize a body orientation perpendicular to sunrays (P1f). A partially overlapping hypothesis to H1 is that lemmings select snow surface to avoid wet conditions in the subnivean environment during snowmelt (H2), as these conditions increase both risks of drowning (Rausch, 1950; Brooks, 1970; Jacob, 2003) and negative energy transfers to the environment (Webb and King, 1984). Accordingly, we predicted that lemming surface activity would coincide with the accumulation of meltwater in the snowpack and at ground level (P2).
We worked from 13 May – 12 June 2019 and 27 May – 14 June 2023 at Canadian Forces Station (CFS) Alert in the Canadian High Arctic, Nunavut (82°30’05” N, 62°20’20” W) (Figure 1). Alert is a polar desert (Callaghan, 2005) characterized by low annual precipitations averaging 156 mm, cold winter temperatures reaching – 40°C in winter, a short growing season (60-80 days), and 24-h direct sunlight from early April to early September (Desjardins et al., 2021). Collared lemmings exhibit strong fluctuations in abundance at Alert (Julien et al., 2014), and 2019 and 2023 were years of high abundance characterized by high trapping success (D. Berteaux, unpublished data).
Figure 1. Location of the study area in North America (insert) and locations of automated cameras (2019 – diamonds, 2023 – dots) at three sites near Canadian Forces Station (CFS) Alert in Nunavut, Canada. The red star locates CFS Alert and the red triangles indicate CFS Alert weather stations. The satellite picture (WorldView-2/3) was taken on 15 July 2020.
We assessed, each year, the presence of lemming predators in our study area by recording observations of Glaucous Gulls (Larus hyperboreus), Long-tailed Jaegers, Snowy Owls (Nyctea scandiaca), American Ermines (Mustela richardsonii), Arctic foxes and Arctic wolves (Canis lupus arctos) while performing other research tasks. Such incidental observations are a good indicator of wildlife abundance when observation effort is sufficient (e.g. ≥200 hr-observer; Fauteux et al., 2018) and wildlife visibility is high, as commonly experienced in the tundra (Bolduc et al., 2023). Seven observers contributed to the predator observations during both 2019 and 2023 monitoring periods (13 May – 12 June 2019 and 27 May – 14 June 2023). The average daily observation effort for each observer was 2.5 ± 1.2 hours in 2019 and 2.6 ± 1.0 hours in 2023, yielding a total of 407 and 250 hours of observations, respectively, across all observers.
The CFS Alert airfield weather station (82°31’ N, 62°17’ W) measured hourly air temperature at 1.5 m above ground, wind speed, and cloud cover. When values were missing, we used hourly air temperature, hourly wind speed and morning and evening cloud cover data from the Alert Environment and Climate Change Canada (ECCC) weather station (82°30’ N, 62°20’ W). Station personnel assessed cloud cover visually (10% increments) and we grouped this variable into four categories, namely Clear (0%), Mainly clear (10%-40%), Partially clear (50%-90%) and Overcast (100%) as indicators of solar radiation. We used binary day/night categories as additional indicators of solar radiation, since incoming radiation varies with the sun’s angle in the sky. This allowed us to account for some of the variations in solar radiation between cloudy days and clear nights. We considered 6:00 - 18:00 as day and 18:00 - 6:00 as night to reflect the daily variation in light intensity. The ECCC weather station estimated snow depth (cm) daily by averaging measurements from three snow depth gauges. Both weather stations are located within 3 km of the study sites described below.
To better capture the thermal microenvironment used by lemmings, we added operative temperature measurements to our monitoring protocol in 2023. From 27 May to 13 June 2023, we measured temperature at the snow surface and at the bottom of the snowpack. At the snow surface, we recorded the internal temperature of 10 lemming-shaped 3-D printed hollow plastic models covered with lemming fur collected at Alert for a previous project. Models precisely replicated the shape, size, color, and fur texture of a collared lemming (Figure 2A) to accurately estimate the operative temperature (Dzialowski, 2005; Bakken and Angilletta, 2014). We placed each model on the snow surface, 1.5 m away from a lemming snow burrow entrance (Figure 2B), to sample locations used by lemmings. We oriented each model randomly with respect to cardinal directions. We recorded their internal temperature at 6-minute intervals with iButton loggers (Model DS1922L, Alphamach Inc., precision ± 0.001°C) positioned in their center. At the bottom of the snowpack, we measured air temperature by installing 10 additional iButton loggers at ground level. IButtons were fixed at the base of a wooden stake (Figure 2C) and inserted in the snowpack. Because wind and sun radiation are absent beneath the snowpack, air temperature and operative temperature are equivalent in this context. For simplicity, we therefore used the term operative temperature to refer to temperatures recorded both above and under the snow.
Figure 2. Lemming-shaped 3-D plastic model (A) containing an iButton to monitor operative temperature at the snow surface near a lemming burrow entrance (B). A wooden stake supporting an iButton at its base (C) and an automated camera at its top (D) monitors temperature at the bottom of the snowpack and lemming activity at the snow surface, respectively. The wooden stake is driven into the snow approximately 1 m from the burrow entrance. Note feces and urine around the burrow entrance, which facilitate detection of burrows by predators. Photos (A, D) by Émilie Desjardins and (B, C) by Rachel Demers.
We conducted a camera-based sampling of lemming behavior in 2019 and 2023 before the snow-free season. On 13 May 2019, after opportunistically observing many lemmings at the surface of the snow, we placed an infrared triggered camera (Hyperfire 2, Reconyx, Holmen, Wisconsin, USA) (Figure 2D) near each of 10 snow burrow entrances. Each camera was mounted with a Universal Camera Mount (Reconyx, Holmen, Wisconsin, USA) on a wooden stake driven into the snow approximately 1 m from the burrow entrance. We placed cameras about 50 cm above the snow surface and centered their 37.7° field of view on the burrow entrance. We programmed cameras to take, after each triggering, a sequence of five pictures at 2 frames per second followed by a 10-second video (hereafter, a triggered sequence). There was no delay between triggered sequences. Cameras remained active until posts collapsed due to local snow melt from 9 to 12 June (median date = 10 June) (Supplementary Table S1 in Supplementary Material). All burrows were located on the plain adjacent to the CFS Alert airfield, referred to below as site 1 (Figure 1).
From 27 May – 3 June 2023, we placed 28 cameras (same methods as above) near 28 lemming burrow entrances at three sites where lemming snow burrows were abundant. Specifically, we monitored 12 burrows at site 1 (already used in 2019) and 8 burrows each at sites 2 and 3 (Figure 1). Cameras remained active until local snow melt, which occurred from 5 to 14 June (median date = 14 June) depending on burrow (Supplementary Table S1 in Supplementary Material).
We calculated a lemming surface activity index (SAI), defined independently for each burrow and each 1-hour period of camera observation as:
Where:
b is the considered burrow
h is the considered 1-hour period of camera observation at burrow b
t is a triggered sequence within the 1-hour period of camera observation
n is the total number of triggered sequences during the 1-h interval h
Lbht is the number of lemmings observed in the t-th triggered sequence.
For example, a burrow would be assigned an index of 7 for a given 1-h period if we recorded 6 triggered sequences with 3 different lemmings observed in the first sequence, 0 in the second, and 1 in each of the remaining 4 sequences. Several lemmings could appear on a given triggered sequence, and the same lemming could appear on many triggered sequences. Therefore, our lemming surface activity index reflects lemming activity at the snow surface around a given burrow during a given 1-hour period, rather than the number of individuals surfacing from the monitored burrow. We used Timelapse2 software (Greenberg et al., 2019) to facilitate data extraction from pictures and videos. Since some cameras sometimes became inactive (e.g. when their supporting stake fell), we only considered burrows with an active camera when calculating an average hourly lemming surface activity index across burrows.
For each lemming observed in a triggered sequence, we assessed four individual-level variables, namely size (small, intermediate, adult), fur color (white, intermediate, brown), distance from burrow (at burrow entrance, close to burrow, far from burrow), and vigilance (not vigilant, vigilant) (Table 1 details category criteria for each variable). We sometimes observed feces and urine within 10-20 cm of the burrow entrance and rated such latrines as absent (immaculate snow), small (snow surface covered by feces and urine < surface covered by an adult lemming) and large (surface covered > lemming size).
Table 1. Categories used to describe lemmings and their behavior when they surface from the snow at Alert, Nunavut, Canada.
We established activity budgets of lemmings observed above the snow using the 10-sec videos recorded in 2019. We extracted data from videos using the BORIS software (Friard and Gamba, 2016) and the behavioral classification of Table 2. We calculated activity budgets above the snow after excluding periods during the 10-sec videos when lemmings were in their burrow and thus not visible. During video recordings characterized by high solar radiation (assessed as a visible lemming shadow), we classified lemming orientation with respect to the sun according to Figure 3.
Table 2. Behavioral classification of collared lemmings observed above the snow at Alert, Nunavut, Canada.
Figure 3. Classification of lemming orientation with respect to the sun, as assessed from observation of lemming shadow.
Since snow conditions can influence lemming behavior and movements (Poirier et al., 2019, 2021), we added snow property measurements to our protocol in 2023 to assess sub-surface conditions. From 29 May to 20 June 2023, we visited each site approximately every three days and dug 2 or 3 snow pits per site per visit, for a total of 34 snow pits (site 1: 9 pits, site 2: 17 pits, site 3: 8 pits). We produced a snow stratigraphy at each snow pit. Based on the size and shape of snow grains and the relative hardness of each snow layer, we differentiated depth hoar crystals from other types of snow crystals, such as small rounded grains, faceting rounded crystals, melt-freeze polycrystals, or crystals from the wind slab or melt-freeze crusts (Pielmeier and Schneebeli, 2002; Fierz et al., 2009). We measured snow relative hardness every 10 cm from ground to snow surface, using the De Quervain hand hardness test (Fierz et al., 2009). This allowed us to identify the main snow layers, evaluate the onset of snow melt, and observe the potential accumulation of water at ground level and in the snowpack. We also measured the total thickness of the snowpack and the thickness of the main layers.
We performed all statistical analyses in R version 4.1.1 (R Core Team, 2021).
We assessed the effects of environmental variables (air temperature, cloud cover, wind speed; P1a-c) on the lemming surface activity index after combining data from 2019 and 2023. We did so using a generalized additive mixed model (GAMM) from the mgcv package version 1.8-42 (Wood, 2017) with a log-link function and assumed Tweedie distribution. GAMMs are suitable when the relationship between explanatory and response variables is nonlinear, as observed here with temperature. We estimated the smoothing parameter for temperature using restricted maximum likelihood (REML). We chose a k basis dimension for temperature, following gam.check diagnostic information (Wood, 2017). We included burrow ID as a random factor (random intercept and slope) to control for unequal sample sizes between burrows. We also included the day/night binary variable alongside cloud cover as indicator of solar radiation intensity. Hours with no observed lemming activity (SAI = 0) were retained in the dataset to account for periods without surface activity. We did not include in our final analysis the year of observation and the interaction between air temperature and wind speed, as their effects on model fit were not significant or negligible. We checked correlations between explanatory variables prior to model selection using a Pearson correlation matrix from the GGally package version 2.1.2 (Schloerke et al., 2021), and we assessed model assumptions visually. We checked residual serial correlation using the autocorrelation function (ACF), which revealed weak correlation with values below 0.2 at all lags. We used the Akaike Information Criterion (AIC) for model selection (Zuur et al., 2007).
We compared mean operative temperature above and under the snowpack (P1d) using a paired t-test from the stats package version 4.4.1 (R Core Team, 2021). To ensure the independence of observations, we first assessed temporal autocorrelation using the autocorrelation function from the stats package (R Core Team, 2021). The results indicated a negligible correlation (coefficient < 0.2) over an 11-hour interval, which guided our choice to subsample the data every 12 hours. We collected measurements at noon and midnight to capture the midpoint of the “Day” and “Night” periods. Normality was confirmed using the Shapiro-Wilk test (stats package; R Core Team, 2021). As a complement to P1b and P1d testing, we also compared mean snow surface operative temperatures during daytime and nighttime using the same method.
We used a chi-squared test from stats package version 4.1.1 (R Core Team, 2021) to examine whether the total duration (s) of observed stationary behavior differed from random across cloud cover categories (P1e). We also used a chi-squared test to assess if lemmings, when stationary above the snow, selected a particular orientation with respect to the sun (P1f).
We present summary statistics as mean ± SD. Research techniques were approved by the Animal Care Committee of Université du Québec à Rimouski (permit # CPA-77-19-209) and the Government of Nunavut (Permit # WL 2018-020).
Incidental predator observations allowed us to confirm the presence of most snow-surface predators in the study area during our lemming monitoring periods. These predators included the Glaucous Gull (first observed on 14 May in 2019 and 23 May in 2023), Long-tailed Jaeger (28 May 2019, 1 June 2023), Snowy Owl (9 May 2019, observed only after the monitoring period in 2023), Arctic fox (26 May 2019, observed only after the monitoring period in 2023) and Arctic wolf (9 May 2019, 22 May 2023). Frequent observations after the first sightings occurred in both years for Glaucous Gull, Long-tailed Jaeger and Arctic wolf, whereas continued observations of Snowy owl and Arctic fox during the lemming monitoring period occurred only in 2019.
Snow depth during the monitoring periods varied from 7 to 18 cm (14.9 ± 2.4 cm, n = 31 days) in 2019 and from 30 to 50 cm (42.4 ± 6.0 cm, n = 19 days) in 2023 (Figures 4A, B). The start of snowmelt marked the end of our lemming monitoring periods on 12 June 2019 and 14 June 2023, but complete snowmelt took 5 days in 2019 and 14 days in 2023. Hourly air temperature during our study periods varied from – 14.9°C to 7.7°C (– 4.0 ± 4.6°C) in 2019 and from -13.3°C to 6.0°C (– 2.3 ± 3.3°C) in 2023, whereas hourly wind speed ranged from 0 km/h to 55 km/h (10.2 ± 10.5 km/h) in 2019 and 0 km/h to 72 km/h (16.7 ± 5.8 km/h) in 2023 (see Figures 4A, B for daily means).
Figure 4. Daily mean ambient temperature, wind speed, and snow depth at Alert (Nunavut, Canada) from 1 May to 1 July 2019 (A) and 2023 (B). Average daily lemming surface activity index, i.e. total number of lemmings detected per day, divided by number of hours of camera activity, during monitoring periods in 2019 (C) and 2023 (D). Dashed vertical lines indicate when the camera monitoring of lemming activity above the snow started (13 May 2019 and 27 May 2023) and ended (12 June 2019 and 14 June 2023).
Operative temperature, measured near 10 monitored burrows at site 1, was clearly higher at the snow surface (6.1 ± 3.9°C, Figure 5) than at ground level (– 5.4 ± 2.6°C) (n pairs for subsampled data = 34/34; t = 13.7, df = 33; p < 0.001), thus supporting P1d. It is noteworthy that 1) mean operative temperature gradient between the surface and the bottom of the snowpack was 11.5 ± 4.5°C (ranging from 1.35 to 28.2°C), 2) 95.3% of all mean operative temperatures measured at the snow surface were > 0°C, compared to 1.1% at ground level, 3) operative temperature above the snow was subject to greater variations (21.8°C difference from – 4.2 to 17.6°C) than temperature at the base of the snowpack (12.2°C difference from – 11.8 to 0.4°C), and 4) operative temperature above the snowpack was higher during the day (8.0 ± 3.8°C, range from – 0.9 to 17.6°C) than during the night (4.2 ± 3.0°C, range from – 4.2 to 13.0°C) (n pairs for subsampled data = 17; t = 5.5; df = 26; p < 0.001). Additionally, operative temperatures above the snow consistently exceeded air temperature (– 2.4 ± 2.8°C, ranged from – 9.9 to 6.0°C) measured by weather stations, with higher values recorded 99.6% of the time. The mean difference between above-snow operative temperature and air temperature was 8.5 ± 4.4°C (ranging from −0.9 to 24.5°C).
Figure 5. Mean operative temperature (± SD) recorded at the surface of the snowpack (dark red) and at ground level (grey) between 27 May and 13 June 2023 at site 1, Alert (Nunavut, Canada). Hourly air temperature from the weather stations appears in blue for reference, whereas yellow bands indicate daytime periods (from 6:00 to 18:00), when daily temperatures are highest although Alert receives 24-h direct sunlight in May and June.
Our monitoring effort represented 294 camera-days in 2019 (10 burrows monitored over 29.4 ± 1.6 days, range 31.0 – 27.9 days) and 332 camera-days in 2023 (28 burrows monitored over 11.9 ± 2.9 days, range 5.8 – 17.4 days). This yielded 1,995 lemming observations in 2019 and 4,615 lemming observations in 2023 (2,020 and 4,845 triggered sequences, respectively). We did not observe any predator attacks on lemmings, although long-tailed jaegers (n = 8), glaucous gulls (n = 3) and ermines (n = 2) were observed in triggered sequences in 2023. During the 2019 monitoring period, lemming surface activity peaked between 23 and 29 May when 77.7% of all lemming observations occurred (Figure 4C), while during the 2023 monitoring period, lemming surface activity peaked between 31 May and 5 June when 75.4% of all lemming observations occurred (Figure 4D). Most (86.8%) triggered sequences contained 1 lemming, but some contained 0 (9.1%), 2 (3.9%), 3 (0.2%) or even 4 (0.02%). Lemmings were usually of adult size (63.8%), but 27.6% had an intermediate size and 8.5% had a size clearly inferior to that of adults. A majority (67.9%) of observed lemmings wore their brown summer fur, but some (18.3%) showed white spots and 5.2% had over half of their body still covered by white winter fur. Fur color could not be assessed in 8.6% of observed lemmings. Latrines at the surface of the snow were observed at 33 of the 38 monitored burrows (26 burrows had a lot, and 7 a little urine and/or feces). Most observed lemmings (68.1%) stayed within 1 m of their burrow entrance.
Air temperature, cloud cover, daytime and wind speed all significantly influenced how many lemmings were observed at the snow surface, as shown by the best model explaining variations in lemming surface activity before snowmelt in 2019 and 2023 (deviance explained = 48.5%, R2 = 0.21, p < 0.001 for all weather variables; Supplementary Table S2). Specifically, more lemmings surfaced from the snow with increasing temperatures (P1asupported), although this effect disappeared at temperatures > – 4.4°C (± 0.7°C, representing the confidence interval around the estimated inflection point of the curve) (Figure 6A). Also as expected, lemming surface activity was positively affected by less cloudy skies (Figure 6B) and daytime (Figure 6D) (P1b supported). Regarding the effect of cloud cover, overcast conditions strongly restrained lemming surface activity. However, once some direct solar radiation became available through partial cloud cover, the percentage of cloud cover appeared to have little to no effect. As expected, wind speed negatively affected lemming surface activity (P1c supported) (Figure 6C).
Figure 6. Partial effects of environmental predictors ((A): temperature, (B): cloud cover, (C): wind speed, (D): binary day/night) on lemming activity above the snow in May – June 2019 and 2023. Effects were generated from our best generalized additive model (Supplementary Table S2). In panels A and B, where partial effects of continuous variables are represented, dashed lines around shadowed areas represent 95% confidence intervals, whereas marks on the x-axis represent the distribution of observations.
Using 1,722 videos collected in 2019 and containing at least one lemming (for a total of 4.78 hours of recording), we found that lemmings were stationary during 65% of the time spent above the snow (entirely visible: 48%, partially visible: 17%). Lemmings were moving 15% of the time while social interactions (2%) and other behaviors (1%) were rare. The remaining 17% of the time corresponds to lemmings being out of the camera field of view. In the 1,354 recordings where lemmings were visible for ≥ 5 seconds, signs of vigilance were detected in 94.5% of triggered sequences. An absence of lemming vigilance characterized only 1.4% of triggered sequences (vigilance level was undetermined in 4.1% of sequences).
There was weak evidence that the occurrence of lemming stationary behavior was associated with cloud cover when individuals were entirely visible (P = 0.07; X2 = 6.96; df = 3; mean difference between observed and expected total durations = 31.4 ± 38.1 sec), and no evidence that the occurrence of lemming stationary behavior was associated with cloud cover when individuals were partially visible (P = 0.64; X2 = 1.69; df = 3; mean difference between observed and expected total durations = 22.7 ± 8.9 sec), thus mostly contradicting P1e. Similarly, stationary lemmings did not select a specific body orientation with respect to the direction of sunrays (P = 0.13; X2 = 5.65; df = 3), contradicting P1f.
In the 35 snow pits dug in 2023, we found no liquid water or melt-freeze ice crust in the snowpack or at the ground surface. This does not support P2, suggesting that meltwater in the snowpack is not a prerequisite for lemmings surfacing behavior in Alert. Even if the thickness of the snowpack varied locally (range 19.0 – 85.0 cm, mean 43.0 ± 18.7 cm), we observed a general structure of the snowpack with two main layers. The bottom layer consisted of the depth hoar (soft, low-density snow composed of large snow grains) whereas the top layer consisted of a harder, denser snow often composed of smaller and more rounded grains (Figure 7). Mean depth hoar thickness was 7.3 ± 3.8 cm (range 0.0 – 15.0 cm). The first observation of melt-freeze polycrystals and ice in the snowpack occurred on 14 June (9 days after the end of the peak surface activity period), which marked the end of the lemming monitoring period.
Figure 7. General stratigraphy of the Alert snowpack in May and June 2023, before snowmelt. The structure shows two main layers, including a thick hard surface layer made of small compact rounded grains on top and a thinner, soft depth hoar made of large and loosely bounded grains of snow at the bottom.
Collared lemmings in the Canadian High Arctic were observed spending time above the snow during spring, when their fur had turned brown, daylight was continuous, and multiple surface predators were active. The likelihood of this behavior increased with air temperature and daytime, yet decreased with cloud cover and wind speed. Higher operative temperatures were recorded at the snow surface compared to ground level, with no liquid water present in or below the snowpack. These findings support our hypothesis that, before the onset of snowmelt in spring, lemmings surface primarily for thermoregulatory purposes, despite the associated predation risks. Conversely, the data do not support the hypothesis that lemmings surface either to disperse before the onset of snowmelt, as most of them stayed in close vicinity of their burrow entrance, or to escape wet conditions below the snow surface. We further discuss the intricate aspects of thermoregulation observed, with emphasis on the many potential trade-offs between thermoregulation and predation risk, with some consideration of foraging behavior.
We anticipated a positive effect of air temperature above the snow on lemming surface activity (P1a), as higher temperatures should reduce the thermal gradient between the environment and the lemming’s body, thereby decreasing heat loss (Birkebak, 1966; Gates, 1980; Tattersall et al., 2012). Similar correlations between air temperature and time spent outside the burrow, along with a positive impact on body temperature, have been observed in Arctic ground squirrels (Long et al., 2005). Conversely, in cooler conditions, lemmings spend more energy and allocate more time to foraging (Maier and Feist, 1991). However, lemming activity plateaued when temperatures reached approximately – 4°C (Figure 6A). This stabilization could be explained by two non-mutually exclusive hypotheses. First, under the surface activity constraint hypothesis, every lemming might have been surfacing at – 4°C, with daily time spent above the snow possibly maxing out due to competing demands such as foraging and sleep, leaving no room for the average surface activity index to increase further beyond – 4°C. Second, the predation risk hypothesis suggests that despite warmer temperatures, the arrival of new migratory predators, such as long-tailed jaegers (noted in 2019 and 2023, Supplementary Figure S1 in Supplementary Material), could have offset the benefits of thermoregulation, thus halting any further increase in surface activity beyond – 4°C due to an unfavorable balance between thermoregulatory benefits and predation risks.
Solar radiation significantly contributes to the thermal energy budget of lemmings by enhancing heat gains (Chappell, 1980b; Norris and Kunz, 2012). Maximizing daytime activity outside the snow is beneficial for lemming thermoregulation, as solar radiation peaks when the sun is highest. However, cloud cover can reduce the body temperature gains achieved during basking (Warnecke et al., 2007; Terrien et al., 2011), though lemmings still benefit from surfacing whenever some solar radiation penetrates the clouds (P1b). Both temperature and solar radiation are critical factors influencing the activity patterns of many rodent species across arctic and other habitats (Walsberg, 1988; Vispo and Bakken, 1993; Ellison et al., 1994; Eifler and Slade, 1998; Bozinovic et al., 2000; Long et al., 2005). Wind, on the other hand (P1c), increases convective heat loss and thus the energetic cost of thermoregulation (Chappell, 1980b). It may also impair lemmings’ ability to detect predators as windy conditions can affect prey’s olfactory, visual or auditive detection (Cherry and Barton, 2017). While solar radiation and wind speed interact, impacting radiative heat gain as wind speed increases (Chappell, 1980b), we were unable to include this interaction in our analysis due to our indirect assessment of radiation via cloud cover and day/night categories. Our measures of operative temperatures at the snow surface (P1d), however, served a similar function and further supported our general hypothesis that lemming surface activity promoted thermoregulatory benefits. Other explanatory variables, such as precipitations and visibility, could also enhance our understanding of lemming behavior, as snowfall likely hinders heat gains at the surface of the snow, and low visibility impairs predator detection. However, the temporal resolution of the precipitation and visibility data from the weather stations was insufficient for inclusion in our model and contained numerous missing values. Humidity inside and outside the burrows could also be a valuable variable for future research, as moisture decreases the insulating effectiveness of fur (Moen and Gilbert, 1973), potentially influencing lemming surface activity for thermoregulatory purposes.
Collared lemmings use well-drained elevated areas in summer and lower habitat with deeper snow accumulation and higher plant density in winter (Batzli et al., 1980; Stenseth and Ims, 1993b). Lemmings must proceed to the spring shift of habitat before low habitats are flooded by heavy snowmelt in early summer (Batzli et al., 1980). However, during our monitoring period, which ended with the onset of snowmelt, lemmings at the snow surface were mostly stationary near their burrow entrance (P1e). Although surfacing above the snow might allow them to gather valuable pre-dispersal information, our observations did not reveal clear sings of active dispersal. Our camera sampling method had limitations that may have prevented us from detecting dispersal events. Since we monitored only one entrance per burrow, lemmings could have exited elsewhere to disperse without being recorded. However, the rare occurrence of walking or running on the snow in our overall activity budget suggests that these behaviors, inherent to the active dispersal process, were uncommon. Additionally, in cases where burrows became inactive, we could not determine the fate of the individuals (i.e., whether they had died, relocated, or created new exits beyond the camera’s field of view). While further observations would be needed to fully assess the dispersal hypothesis, our data suggest that surface activity at this time serves primarily as an energy saving mechanism.
Lemmings on the snow were likely sun-basking to decrease the energetic demands of thermoregulation through passive heating via solar radiation (Terrien et al., 2011). Supporting this interpretation, Chappell (1980b) found using heated metal casts that lemmings concealed within their subnivean burrows faced higher thermoregulation costs compared to those exposed to sunlight. Surprisingly, however, lemmings did not orient their bodies perpendicular to the sunrays (P1f), as would be expected for maximizing energy absorption from solar radiation. Such orientation is known to maximize body surface exposure to sunlight, increasing solar heat gain, and has been observed in other species (Berry, 1984; Maloney et al., 2005; Brown and Downs, 2007; Hetem et al., 2011; Norris and Kunz, 2012).
This paradox leads to four non-mutually exclusive hypotheses. First, the posture hypothesis suggests that lemmings might use body posture to reduce thermoregulation costs once out on the snow. For example, Wyoming ground squirrels (Urocitellus elegans) do not adjust their solar orientation but use different postures as a behavioral thermoregulation strategy (Byman, 1985). We observed various postures in stationary lemmings, such as standing on all fours or on their hind legs, which may have thermoregulatory functions. Second, the wind hypothesis posits that lemming orientation could be influenced by wind direction, as wind can increase convective heat loss, reducing the overall heat retained from radiation based on body orientation (Heller, 1972; Fortin et al., 2000). Third, the snow reflection hypothesis considers the high albedo of snow, which reflects 50-90% of solar radiation (Pomeroy and Brun, 2001; Gardner and Sharp, 2010). Lemmings might benefit from the high reflectivity of the surrounding snow, thus lessening the need to orient their bodies directly towards the sun. Finally, the predation hypothesis arises from our observation that lemmings frequently changed their body orientation while scanning their surroundings, a sign of vigilance. Vigilance, common in many mammal species, serves primarily to detect predators (Quenette, 1990). Thus, while the predation risk at the snow surface was low enough to allow lemmings to surface, it might still be high enough to prevent them from maintaining an optimal orientation for sun-basking. Further research on energy input from solar radiation and predation risk at Alert is essential to explore these hypotheses.
In early June 2023, snow stratigraphy at Alert featured a soft depth hoar layer covered by a harder surface layer, aligning with observations from late April 2000 in the same area (Domine et al., 2002) and mirroring patterns typical of other Arctic regions (Benson and Sturm, 1993; Sturm et al., 1995; Domine et al., 2012, 2018). Contrary to findings at other sites for lemmings (Rausch, 1950; I. E. Menyusnhina, Pers. Comm., 2021) and voles (Korslund and Steen, 2006), wet conditions in the subnivean environment were not a factor for lemming surface activity at Alert (P2). This demonstrates that, while snow melt may promote lemming’s activity at the snow surface in spring, it is not a prerequisite for such a behavior.
We confirmed the presence of several avian and terrestrial predators in Alert during the monitored period of lemming activity at the snow surface, including long-tailed jaegers and wolves which were observed capturing lemmings on the snow (R. Demers, E. Desjardins, D. Berteaux, unpublished data). Given that many predators rely on visual cues to locate their prey (Martin, 2017), it is clear that lemmings on the snow surface faced heightened predation risk. However, the automated cameras did not capture any attack from predators on lemmings. The small numbers of burrow entrances monitored (10 burrows in 2019, and 28 in 2023) compared to the abundance of burrows available might reduce the probability of capturing such predatory behaviors. This could also indicate a low risk of predation for lemmings staying close to their burrow entrance, which could favor the surfacing behavior of lemmings in Alert. One limitation of our qualitative predation risk assessment is that we observed ermines in Alert after the study period in both 2019 and 2023, suggesting they were present in spring as well. Since ermines primarily hunt under the snow, lemmings may reduce their exposure to ermines by remaining on the snow surface. Thus, increasing exposure to surface predators may decrease vulnerability to subnivean predators (Kotler et al., 1992), making trade-offs between thermoregulation and predation risk even more complex to assess. This is compounded by the fact that ermines can also hunt on the surface, and foxes and wolves are capable of catching lemmings below the snow.
In addition to the suggested conflict between thermoregulation and predation risk, several other trade-offs may arise. These include the balance between social interactions and thermoregulation, as lemmings might need to engage in parental or sibling-related social behaviors. Similarly, foraging time may be traded for thermoregulation, especially if increased food intake at cooler temperatures in lemmings (Maier and Feist, 1991; Nagy and Negus, 1993) allows to meet the high energetic demands of physiological heat production (McNab, 2002) or if the quality of available vegetation diminishes towards the end of winter. The analysis of these potential trade-offs is highly complex, particularly when considering that exposure to sunlight may confer additional benefits, such as hormonal regulation (Gower et al., 1993) or the synthesis of vitamins that are otherwise unavailable in their diet (Hickie et al., 1982), further promoting surfacing behavior.
The onset of spring molt in lemmings is initiated by the increase in light availability (Maier and Feist, 1991; Nagy et al., 1993), which inhibits the secretion of melatonin and stimulates the secretion of prolactin, responsible for the production of brown fur (Gower et al., 1993). Temperature, on the other hand, appears to influence the rate of molt in several northern species such as snowshoe hare and collared lemming (Zimova et al., 2018). As daylight returns in the last days of February and 24-h continuous sunlight starts in early April in Alert, most lemmings observed in May and June had already been through their spring molt. However, summer brown fur provides more visibility and less insulation than its winter white counterpart (Reynolds and Lavigne, 1988; Maier and Feist, 1991; Reynolds, 1993). Given that snowmelt in 2019 and 2023 did not occur later than the long-term average, and considering the typical minimum air temperatures of around – 15°C during our study, it is puzzling why the studied population did not evolve a later summer molt. Particularly, this adaptation could potentially reduce the observed color mismatch between lemming fur and the snowy background, which likely increases predation risk (Caro, 2005; Zimova et al., 2016; Atmeh et al., 2018; Otte et al., 2024). One hypothesis involves thermoregulatory benefits for lemmings with brown fur exposed to solar radiation. Specifically, small mammals, including lemmings, with brown pelage spend less energy maintaining body temperature while sun-basking than those with white pelage (Chappell, 1980b). Therefore, under spring conditions where the ground remains snow-covered and temperatures are low, yet solar radiation is strong, the energetic benefits of brown fur may outweigh the higher insulation and lower visibility associated with white fur. Staying in the close vicinity of their burrow entrance when on the snow surface might also be a behavioral adaptation to limit the predation risk heightened by this color mismatch. This could further explain the absence of predation events detected by the automated cameras.
Lemming burrow entrances, often marked by urine and feces, were easily detectable, even when we were riding our snowmobiles. This visibility suggests that predators such as jaegers, foxes, and wolves could also easily spot locations where lemmings exited from the snow. However, summer observations in the Northwest Territories of Canada reveal that collared lemmings conceal their feces (and presumably urine) in underground latrines, likely to evade detection by predators (Boonstra et al., 1996). It is intriguing that lemmings adopt this concealment strategy during the summer but not in the winter when tracks are more visible against the white snow. To explain this apparent discrepancy, it’s notable that while lemmings at Alert use underground latrines in summer, our experiments using white plastic cardboards placed near summer burrows showed that they still occasionally excrete small quantities of urine and feces above ground (D. Berteaux, pers. obs.). Therefore, the ‘indoor plumbing’ reported by Boonstra et al. (1996) may not ensure a completely clean floor, suggesting that our winter observations might not be as contradictory as they initially seem.
Monitoring lemming activity above the snow has provided new insights into their winter population dynamics. Observations of up to four lemmings surfacing from the same snow burrow, including individuals significantly smaller than adults, indicate that lemmings reproduced during the winters of 2019 and 2023. This reproductive activity aligns with the high lemming abundance observed at Alert during the subsequent summers of 2019 and 2023, as evidenced by incidental observations, trapping, and monitoring of predator reproduction (D. Berteaux, unpublished data). Collared lemmings are known to reproduce under favorable winter conditions (Stenseth and Ims, 1993a; Krebs et al., 1995; Millar, 2001), and such winter reproduction is crucial for the rapid population increases leading to peak density years (Millar, 2001; Ims et al., 2011; Fauteux et al., 2015). Given that data on winter reproduction are scarce, spring observations above the snow are a valuable source of new knowledge. A next step is to investigate whether spring surface activity also occurs in years of low lemming abundance, and what this activity might reveal about the age structure, molt phenology, or lemming behavior across the various phases of the lemming cycle.
With the now widespread availability of infrared-triggered cameras, we were able for the first time to closely study the spring activity of lemmings at the snow surface. Thermoregulatory costs significantly contribute to the energy expenditure of small arctic mammals (Chappell, 1980b; McNab, 2002) and, interestingly, collared lemmings choose to expose themselves to surface predators in exchange for thermoregulatory benefits. While similar trade-offs have been noted in other mammal species (Brown and Downs, 2005; Glass et al., 2021), their implications on predator-prey dynamics in the Arctic tundra, where collared lemmings are a central piece of the food web (Batzli et al., 1980), need to be explored.
The raw data supporting the conclusions of this article will be made available by the authors, without undue reservation.
The animal study was approved by Animal Care Committee of Université du Québec à Rimouski (permit # CPA-77-19-209). The study was conducted in accordance with the local legislation and institutional requirements.
RD: Conceptualization, Data curation, Formal analysis, Investigation, Methodology, Visualization, Writing – original draft, Writing – review & editing. ÉD: Investigation, Writing – review & editing. DF: Writing – review & editing. FV: Writing – review & editing. AT: Writing – review & editing. DB: Conceptualization, Funding acquisition, Investigation, Methodology, Supervision, Writing – review & editing.
The author(s) declare that financial support was received for the research and/or publication of this article. This project was financially supported by the Canada Foundation for Innovation (grant #38881), Canada Research Chairs Program, Department of National Defence of Canada, Natural Sciences and Engineering Research Council of Canada (grant # 509948-2018, RGPIN-2019-0592, RGPNS-2019-305531), Fonds de recherche du Québec – Nature et technologies, BIOS2 NSERC CREATE program (grant #509948-2018), and Network of Centers of Excellence of Canada ArcticNet.
This research was made possible by the logistical support provided by the Department of National Defence of Canada and the CFS Alert personnel. We thank Sandra Lai and Jacob Caron Carrier for preliminary data collection, the members of the SnowBird and HareForce 2023 teams for their aid in data collection, Ryan S. O’Connor, Lincoln Savi and Marc Szymansky for their help in the 3-D plastic models making process, Audrey Grolleau, Timothée Nozieres and Nabila Djahnine for their assistance in establishing lemming activity budgets, Marie-Jeanne Rioux and Audrey LePogam for help coordinating fieldwork campaigns, and Alain Caron for statistical advice. Finally, we thank the reviewers for their time and their insightful comments and suggestions, which helped us to improve the quality of the manuscript.
The authors declare that the research was conducted in the absence of any commercial or financial relationships that could be construed as a potential conflict of interest.
The author(s) declare that Generative AI was used in the creation of this manuscript. DB used ChatGPT 4o for assistance during language improvement of the discussion of this manuscript. This use has been declared in the acknowledgements section of the manuscript.
All claims expressed in this article are solely those of the authors and do not necessarily represent those of their affiliated organizations, or those of the publisher, the editors and the reviewers. Any product that may be evaluated in this article, or claim that may be made by its manufacturer, is not guaranteed or endorsed by the publisher.
The Supplementary Material for this article can be found online at: https://www.frontiersin.org/articles/10.3389/fevo.2025.1543635/full#supplementary-material
Supplementary Figure 1 | Average daily avian (purple) and terrestrial (green) predator index (A, C), i.e. total number of incidental observations of lemming predators detected per day, divided by mean daily observational effort (hours) for one observer, from 13 May to 12 June 2019 (A) and 27 May to 14 June 2023 (C). The temporal evolution of the predator index shows (particularly in A) the increased local activity of avian migratory predators throughout our study period as they arrive from migration. Daily mean ambient temperature (curve) and average daily lemming surface activity index (histogram) are presented in (B) (2019) and (D) (2023) for reference. The dashed red lines in (B, D) represent the – 4.4°C mark, where the effect of temperature on lemming surface activity plateaued.
Atmeh K., Andruszkiewicz A., Zub K. (2018). Climate change is affecting mortality of weasels due to camouflage mismatch. Sci. Rep. 8, 7648. doi: 10.1038/s41598-018-26057-5
Bakken G. S., Angilletta M. J. (2014). How to avoid errors when quantifying thermal environments. Funct. Ecol. 28, 96–107. doi: 10.1111/1365-2435.12149
Bakken G. S., Santee W. R., Erskine D. J. (1985). Operative and standard operative temperature: tools for thermal energetics studies. Am. Zool 25, 933–943. doi: 10.1093/icb/25.4.933
Batzli G. O., White R. G., MacLean S. F., Pitelka F. A., Collier B. D. (1980). “The herbivore-based trophic system,” in An Arctic ecosystem: the coastal tundra at Barrow, Alaska. Eds. Brown J., Miller P. C., Tieszen L. L., Bunnell F. L. (Dowden, Hutchinson & Ross, Stroudsburg), 335–410.
Benson C. S., Sturm M. (1993). Structure and wind transport of seasonal snow on the Arctic slope of Alaska. Ann. Glaciol 18, 261–267. doi: 10.3189/S0260305500011629
Berry H. H. (1984). Orientation of wildebeest in relation to sun angle and wind direction. Madoqua 13, 297–301. doi: 10.10520/AJA10115498_299
Berteaux D., Gauthier G., Domine F., Ims R. A., Lamoureux S. F., Lévesque E., et al. (2017). Effects of changing permafrost and snow conditions on tundra wildlife: critical places and times. Arct Sci. 3, 65–90. doi: 10.1139/as-2016-0023
Bicca-Marques J. C., Calegaro-Marques C. (1998). Behavioral thermoregulation in a sexually and developmentally dichromatic neotropical primate, the black-and-gold howling monkey (Alouatta caraya). Am. J. Phys. Anthropol 106, 533–546. doi: 10.1002/(SICI)1096-8644(199808)106:4<533::AID-AJPA8>3.0.CO;2-J
Bilodeau F., Gauthier G., Berteaux D. (2013). Effect of snow cover on the vulnerability of lemmings to mammalian predators in the Canadian Arctic. J. Mammal 94, 813–819. doi: 10.1644/12-MAMM-A-260.1
Birkebak R. C. (1966). “Heat transfer in biological systems,” in International review of general and experimental zoology. Eds. Felts W. J. L., Harrison R. J. (Academic Press, New York), 269–344.
Bolduc D., Fauteux D., Gagnon C. A., Gauthier G., Bêty J., Legagneux P. (2023). Testimonials to reconstruct past abundances of wildlife populations. Basic Appl. Ecol. 68, 23–34. doi: 10.1016/j.baae.2022.11.005
Boonstra R., Krebs C. J., Kenney A. (1996). Why lemmings have indoor plumbing in summer. Can. J. Zool 74, 1947–1949. doi: 10.1139/z96-220
Bozinovic F., Lagos J. A., Vásquez R. A., Kenagy G. J. (2000). Time and energy use under thermoregulatory constraints in a diurnal rodent. J. Therm Biol. 25, 251–256. doi: 10.1016/S0306-4565(99)00031-5
Brooks R. J. (1970). Ecology and acoustic behavior of the collared lemming, (Dicrostonyx groenlandicus (Traill) (Urbana: University of Illinois).
Brown J. S., Kotler B. P. (2004). Hazardous duty pay and the foraging cost of predation: Foraging cost of predation. Ecol. Lett. 7, 999–1014. doi: 10.1111/j.1461-0248.2004.00661.x
Brown K. J., Downs C. T. (2005). Seasonal behavioural patterns of free-living rock hyrax (Procavia capensis). J. Zool 265, 311–326. doi: 10.1017/S0952836905006412
Brown K. J., Downs C. T. (2007). Basking behaviour in the rock hyrax (Procavia capensis) during winter. Afr Zool 42, 70–79. doi: 10.1080/15627020.2007.11407379
Byman D. (1985). Thermoregulatory behavior of a diurnal small mammal, the wyoming ground squirrel (Spermophilus elegans). Physiol. Zool 58, 705–718. doi: 10.1086/physzool.58.6.30156074
Callaghan T. V. (2005). Arctic tundra and polar desert ecosystems. in Arctic climate impact assessment [Report]. (New York: Cambridge University Press), pp. 243–352.
Caro T. (2005). The adaptive significance of coloration in mammals. BioScience 55, 125–136. doi: 10.1641/0006-3568(2005)055[0125:TASOCI]2.0.CO;2
Chappell M. A. (1980a). Insulation, radiation, and convection in small arctic mammals. J. Mammal 61, 268–277. doi: 10.2307/1380048
Chappell M. A. (1980b). Thermal energetics and thermoregulatory costs of small arctic mammals. J. Mammal 61, 278–291. doi: 10.2307/1380049
Cherry M. J., Barton B. T. (2017). Effects of wind on predator-prey interactions. Food Webs 13, 92–97. doi: 10.1016/j.fooweb.2017.02.005
Coppola F., Vecchio G., Felicioli A. (2019). Diurnal motor activity and “sunbathing” behaviour in crested porcupine (Hystrix cristata L). Sci. Rep. 9, 14283. doi: 10.1038/s41598-019-50784-y
Creel S. (2018). The control of risk hypothesis: reactive vs. proactive antipredator responses and stress-mediated vs. food-mediated costs of response. Ecol. Lett. 21, 947–956. doi: 10.1111/ele.12975
Desjardins É., Lai S., Payette S., Dubé M., Sokoloff P. C., St-Louis A., et al. (2021). Survey of the vascular plants of Alert (Ellesmere Island, Canada), a polar desert at the northern tip of the Americas. CheckList 17, 181–225. doi: 10.15560/17.1.181
Domine F., Barrere M., Sarrazin D. (2016). Seasonal evolution of the effective thermal conductivity of the snow and the soil in high Arctic herb tundra at Bylot Island, Canada. Cryosphere 10, 2573–2588. doi: 10.5194/tc-10-2573-2016
Domine F., Cabanes A., Legagneux L. (2002). Structure, microphysics, and surface area of the Arctic snowpack near Alert during the ALERT 2000 campaign. Atmos Environ. 36, 2753–2765. doi: 10.1016/S1352-2310(02)00108-5
Domine F., Gallet J.-C., Bock J., Morin S. (2012). Structure, specific surface area and thermal conductivity of the snowpack around Barrow, Alaska. J. Geophys Res. 117, 1–12. doi: 10.1029/2011JD016647
Domine F., Gauthier G., Vionnet V., Fauteux D., Dumont M., Barrere M. (2018). Snow physical properties may be a significant determinant of lemming population dynamics in the high Arctic. Arct Sci. 4, 813–826. doi: 10.1139/as-2018-0008
Duchesne D., Gauthier G., Berteaux D. (2011). Habitat selection, reproduction and predation of wintering lemmings in the Arctic. Oecologia 167, 967–980. doi: 10.1007/s00442-011-2045-6
Dzialowski E. M. (2005). Use of operative temperature and standard operative temperature models in thermal biology. J. Therm Biol. 30, 317–334. doi: 10.1016/j.jtherbio.2005.01.005
Ehrich D., Schmidt N. M., Gauthier G., Alisauskas R., Angerbjörn A., Clark K., et al. (2020). Documenting lemming population change in the Arctic: Can we detect trends? Ambio 49, 786–800. doi: 10.1007/s13280-019-01198-7
Eifler M. A., Slade N. A. (1998). Activity patterns in relation to body mass and ambient temperature among overwintering cotton rats (Sigmodon hispidus). Can. J. Zool 76, 668–672. doi: 10.1139/z97-241
Ellison G. T. H., Skinner J. D., Ferguson J. W. H. (1994). Interactive effects of temperature and photoperiod on the daily activity and energy metabolism of pouched mice (Saccostomus campestris: Cricetidae) from southern Africa. J. Comp. Physiol. B 164, 62–68. doi: 10.1007/BF00714572
Fauteux D., Gauthier G., Berteaux D. (2015). Seasonal demography of a cyclic lemming population in the Canadian Arctic. J. Anim. Ecol. 84, 1412–1422. doi: 10.1111/1365-2656.12385
Fauteux D., Gauthier G., Mazerolle M. J., Coallier N., Bêty J., Berteaux D. (2018). Evaluation of invasive and non-invasive methods to monitor rodent abundance in the Arctic. Ecosphere 9, e02124. doi: 10.1002/ecs2.2124
Fierz C., Armstrong R. L., Durand Y., Etchevers P., Greene E., McClung D. M., et al. (2009). International classification for seasonal snow on the ground (Paris: UNESCO - International hydrological programme).
Flôres D. E. F. L., Jannetti M. G., Improta G. C., TaChinardi P., Valentinuzzi V. S., Oda G. A. (2021). Telling the seasons underground: The circadian clock and ambient temperature shape light exposure and photoperiodism in a subterranean rodent. Front. Physiol. 12. doi: 10.3389/fphys.2021.738471
Fortin D., Larochelle J., Gauthier G. (2000). The effect of wind, radiation and body orientation on the thermal environment of Greater Snow goose goslings. J. Therm Biol. 25, 227–238. doi: 10.1016/S0306-4565(99)00028-5
Friard O., Gamba M. (2016). BORIS: a free, versatile open-source event-logging software for video/audio coding and live observations. Methods Ecol. Evol. 7, 1325–1330. doi: 10.1111/2041-210X.12584
Fuller W. A., Martell A. M., Smith R. F. C., Speller S. W. (1975). High-arctic lemmings, Dicrostonyx groenlandicus. II. Demography. Can. J. Zool 53, 867–878. doi: 10.1139/z75-100
Gagge A. P. (1940). Standard operative temperature: A generalized temperature scale, applicable to direct and partitional calorimetry. Am. J. Physiol. 131, 93–103. doi: 10.1152/ajplegacy.1940.131.1.93
Gardner A. S., Sharp M. J. (2010). A review of snow and ice albedo and the development of a new physically based broadband albedo parameterization. J. Geophys Res. 115, F01009. doi: 10.1029/2009JF001444
Gates D. M. (1980). Biophysical ecology., 1st edn (New York: Springer New York). doi: 10.1007/978-1-4612-6024-0
Gilg O. (2002). The summer decline of the collared lemming, Dicrostonyx groenlandicus, in high arctic Greenland. Oikos 99, 499–510. doi: 10.1034/j.1600-0706.2002.11989.x
Glass T. W., Breed G. A., Robards M. D., Williams C. T., Kielland K. (2021). Trade-off between predation risk and behavioural thermoregulation drives resting behaviour in a cold-adapted mesocarnivore. Anim. Behav. 175, 163–174. doi: 10.1016/j.anbehav.2021.02.017
Gotthardt T. A., McClory J. G. (2006). Northern collared lemming and Alaska subspecies. Anchorage, AK: Resources Institute, University of Alaska Anchorage.
Gower B. A., Nagy T. R., Stetson M. H. (1993). Role of prolactin and the gonads in seasonal physiological changes in the collared lemming (Dicrostonyx groenlandicus). J. Exp. Zool 266, 92–101. doi: 10.1002/jez.1402660203
Greenberg S., Godin T., Whittington J. (2019). Design patterns for wildlife-related camera trap image analysis. Ecol. Evol. 9, 13706–13730. doi: 10.1002/ece3.5767
Hansen R. M. (1959). Aspects of coat color in young varying lemmings. J. Mammal 40, 205–213. doi: 10.2307/1376435
Heller H. C. (1972). Measurements of convective and radiative heat transfer in small mammals. J. Mammal 53, 289–295. doi: 10.2307/1379164
Hetem R. S., Maartin Strauss W., Heusinkveld B. G., De Bie S., Prins H. H. T., Van Wieren S. E. (2011). Energy advantages of orientation to solar radiation in three African ruminants. J. Therm Biol. 36, 452–460. doi: 10.1016/j.jtherbio.2011.07.012
Hickie J. P., Lavigne D. M., Woodward W. D. (1982). Vitamin D and winter reproduction in the collared lemming, Dicrostonyx Groenlandicus. Oikos 39, 71. doi: 10.2307/3544533
Ims R. A., Yoccoz N. G., Killengreen S. T. (2011). Determinants of lemming outbreaks. Proc. Natl. Acad. Sci. U.S.A. 108, 1970–1974. doi: 10.1073/pnas.1012714108
Jacob J. (2003). The response of small mammal populations to flooding. Mamm Biol. 68, 102–111. doi: 10.1078/1616-5047-00068
Jarrel G. H., Fredga K. (1993). “How many kinds of lemmings? A taxonomic overview,” in The biology of lemmings. Eds. Stenseth N. C., Ims R. A. (Linnean Society of London, Academic Press, London, UK), 45–57.
Julien J.-R., Legagneux P., Gauthier G., Morrison R. I. G., Therrien J.-F., Bêty J. (2014). Contribution of allochthonous resources to breeding in a high-arctic avian predator. Polar Biol. 37, 193–203. doi: 10.1007/s00300-013-1423-4
Kays R. W., Wilson D. E. (2009). Mammals of north america. 2nd (New Jersey: Princeton University Press).
Kelley E. A., Jablonski N. G., Chaplin G., Sussman R. W., Kamilar J. M. (2016). Behavioral thermoregulation in Lemur catta: The significance of sunning and huddling behaviors. Am. J. Primatol 78, 745–754. doi: 10.1002/ajp.22538
Kirkwood J. K. (1983). A limit to metabolisable energy intake in mammals and birds. Comp. Biochem. Physiol. A 75, 1–3. doi: 10.1016/0300-9629(83)90033-6
Korslund L., Steen H. (2006). Small rodent winter survival: snow conditions limit access to food resources. J. Anim. Ecol. 75, 156–166. doi: 10.1111/j
Kotler B. P., Blaustein L., Brown J. S. (1992). Predator facilitation: the combined effect of snakes and owls on the foraging behavior of gerbils. Ann. Zool. Fennici 29, 199–206.
Krebs C. J., Boonstra R., Kenney A. J. (1995). Population dynamics of the collared lemming and the tundra vole at Pearce Point, Northwest Territories, Canada. Oecologia 103, 481–489. doi: 10.1007/BF00328687
Long R. A., Martin T. J., Barnes B. M. (2005). Body temperature and activity patterns in free-living Arctic ground squirrels. J. Mammal 86, 314–322. doi: 10.1644/BRG-224.1
MacLean S. F., Fitzgerald B. M., Pitelka F. A. (1974). Population cycles in arctic lemmings: winter reproduction and predation by weasels. Arct Alp Res. 6, 1–12. doi: 10.2307/1550365
Maier H. A., Feist D. D. (1991). Thermoregulation, growth, and reproduction in alaskan collared lemmings: role of short day and cold. Am. J. Physiol-Reg I 261, R522–R530. doi: 10.1152/ajpregu.1991.261.3.R522
Maloney S. K., Moss G., Mitchell D. (2005). Orientation to solar radiation in black wildebeest (Connochaetes gnou). J. Comp. Physiol. A 191, 1065–1077. doi: 10.1007/s00359-005-0031-3
Mathot K. J., Dingemanse N. J. (2015). Energetics and behavior: unrequited needs and new directions. Trends Ecol. Evol. 30, 199–206. doi: 10.1016/j.tree.2015.01.010
McNab B. K. (2002). The physiological ecology of vertebrates: A view from energetics (New York: Cornell University Press).
Millar J. S. (2001). On reproduction in lemmings. Ecoscience 8, 145–150. doi: 10.1080/11956860.2001.11682639
Milling C. R., Rachlow J. L., Johnson T. R., Forbey J. S., Shipley L. A. (2017). Seasonal variation in behavioral thermoregulation and predator avoidance in a small mammal. Behav. Ecol. 28, 1236–1247. doi: 10.1093/beheco/arx084
Moen A. N., Gilbert D. L. (1973). “Thermal energy exchange between organism and environment,” in Wildlife ecology: an analytical approach (Freeman, San Francisco).
Mueller H. C. (1972). Sunbathing in birds. Z Tierpsychol 30, 253–258. doi: 10.1111/j.1439-0310.1972.tb00853.x
Nagy T. R., Gower B. A., Stetson M. H. (1993). Threshold photoperiods for the induction of short day traits in collared lemmings (Dicrostonyx groenlandicus). J. Exp. Zool 267, 57–66. doi: 10.1002/jez.1402670109
Nagy T. R., Negus N. C. (1993). Energy acquisition and allocation in male collared lemmings (Dicrostonyx groenlandicus): Effects of photoperiod, temperature, and diet quality. Physiol. Zool 66, 537–560. doi: 10.1086/physzool.66.4.30163807
Norris A. L., Kunz T. H. (2012). "Effects of solar radiation on animal thermoregulation" in Solar Radiation. Ed. E. B. Babatunde (InTech, Rijieka), 195–220.
Otte P. J., Cromsigt J. P. G. M., Smit C., Hofmeester T. R. (2024). Snow cover-related camouflage mismatch increases detection by predators. J. Exp. Zool Pt A. 341, 327-337. doi: 10.1002/jez.2784
Pauli J. N., Zuckerberg B., Whiteman J. P., Porter W. (2013). The subnivium: a deteriorating seasonal refugium. Front. Ecol. Environ. 11, 260–267. doi: 10.1890/120222
Pielmeier C., Schneebeli M. (2002). Snow stratigraphy measured by snow hardness and compared to surface section images (British Columbia: British Columbia Ministry of Transportation, Snow Avalanche Programs).
Poirier M., Fauteux D., Gauthier G., Domine F., Lamarre J. (2021). Snow hardness impacts intranivean locomotion of arctic small mammals. Ecosphere 12, 1–14. doi: 10.1002/ecs2.3835
Poirier M., Gauthier G., Domine F. (2019). What guides lemmings movements through the snowpack? J. Mammal 100, 1416–1426. doi: 10.1093/jmammal/gyz129
Pomeroy J. W., Brun E. (2001). “Physical properties of snow,” in Snow ecology: an interdisciplinary examination of snow-covered ecosystems. Eds. Jones G., Pomeroy J. W., Walker D. A., Hoham R. W. (Cambridge University Press, Cambridge, UK), 45–126.
Quenette P.-Y. (1990). Functions of vigilance behaviour in mammals: a review. Acta Oecol 11, 801–818.
Rausch R. (1950). Observations on a cyclic decline of lemmings (Lemmus) on the arctic coast of Alaska during the spring of 1949. Arctic 3, 166–177. doi: 10.14430/arctic3964
R Core Team (2021). R: a language and environment for statistical computing. Available online at: https://www.r-project.org/ (Accessed June 06, 2022).
Reid D. G., Bilodeau F., Krebs C. J., Gauthier G., Kenney A. J., Gilbert B. S., et al. (2012). Lemming winter habitat choice: a snow-fencing experiment. Oecologia 168, 935–946. doi: 10.1007/s00442-011-2167-x
Reynolds P. S. (1993). Effects of body size and fur on heat loss of collared lemmings, Dicrostonyx groenlandicus. J. Mammal 74, 291–303. doi: 10.2307/1382384
Reynolds P. S., Lavigne D. M. (1988). Photoperiodic effects on body size and energetics of the collared lemming, Dicrostonyx groenlandicus. Can. J. Zool 66, 835–841. doi: 10.1139/z88-123
Schloerke B., Cook D., Larmarange J., Briatte F., Marbach M., Thoen E., et al. (2021). GGally: extension to ggplot2. Available online at: https://CRAN.R-project.org/package=GGally (Accessed March 03, 2023).
Stenseth N. C., Ims R. A. (1993a). “Population dynamics of lemmings: temporal and spatial variation - an introduction,” in The biology of lemmings. Eds. Stenseth N. C., Ims R. A. (Academic Press, London), 61–96.
Stenseth N. C., Ims R. A. (1993b). “The evolutionary history and distribution of lemmings - an introduction,” in The biology of lemmings (Academic Press, London), 37–44.
Sturm M., Holmgren J., Liston G. E. (1995). A seasonal snow cover classification system for local to global applications. J. Climate 8, 1261–1283. doi: 10.1175/1520-0442(1995)008<1261:ASSCCS>2.0.CO;2
Tattersall G. J., Sinclair B. J., Withers P. C., Fields P. A., Seebacher F., Cooper C. E., et al. (2012). Coping with thermal challenges: physiological adaptations to environmental temperatures. Compr. Physiol. 2, 2151–2202. doi: 10.1002/cphy.c110055
Terrien J., Perret M., Aujard F. (2011). Behavioral thermoregulation in mammals: a review. Front. Biosci. 16, 1428. doi: 10.2741/3797
Veldhuis M. P., Kihwele E. S., Cromsigt J. P. G. M., Ogutu J. O., Hopcraft J. G. C., Owen-Smith N., et al. (2019). Large herbivore assemblages in a changing climate: incorporating water dependence and thermoregulation. Ecol. Lett. 22, 1536–1546. doi: 10.1111/ele.13350
Verdolin J. L. (2006). Meta-analysis of foraging and predation risk trade-offs in terrestrial systems. Behav. Ecol. Sociobiol 60, 457–464. doi: 10.1007/s00265-006-0172-6
Vispo C. R., Bakken G. S. (1993). The influence of thermal conditions on the surface activity of thirteen-lined ground squirrels. Ecology 74, 377–389. doi: 10.2307/1939300
Walsberg G. E. (1988). Heat flow through avian plumages: The relative importance of conduction, convection, and radiation. J. Therm Biol. 13, 89–92. doi: 10.1016/0306-4565(88)90018-6
Warnecke L., Turner J. M., Geiser F. (2007). Torpor and basking in a small arid zone marsupial. Naturwissenschaften 95, 73–78. doi: 10.1007/s00114-007-0293-4
Webb D. R., King J. R. (1984). Effects of wetting of insulation of bird and mammal coats. J. Therm Biol. 9, 189–191. doi: 10.1016/0306-4565(84)90020-2
Weiner J. (1992). Physiological limits to sustainable energy budgets in birds and mammals: Ecological implications. Trends Ecol. Evol. 7, 384–388. doi: 10.1016/0169-5347(92)90009-Z
Wood S. N. (2017). Generalized additive models: an introduction with R. 2nd (Boca Raton, Florida, US: CRC Press).
Zimova M., Hackländer K., Good J. M., Melo-Ferreira J., Alves P. C., Mills L. S. (2018). Function and underlying mechanisms of seasonal colour moulting in mammals and birds: what keeps them changing in a warming world? Biol. Rev. 93, 1478–1498. doi: 10.1111/brv.12405
Zimova M., Mills L. S., Nowak J. J. (2016). High fitness costs of climate change-induced camouflage mismatch. Ecol. Lett. 19, 299–307. doi: 10.1111/ele.12568
Keywords: behavioral thermoregulation, Dicrostonyx, small mammal, operative temperature, automated cameras, vigilance, trade-off, weather
Citation: Demers R, Desjardins É, Fauteux D, Vézina F, Tam A and Berteaux D (2025) Spring fever: collared lemmings warm up above the snow despite predation risk. Front. Ecol. Evol. 13:1543635. doi: 10.3389/fevo.2025.1543635
Received: 11 December 2024; Accepted: 18 March 2025;
Published: 03 April 2025.
Edited by:
Ann Valerie Hedrick, University of California, Davis, United StatesReviewed by:
Audun Stien, UiT The Arctic University of Norway, NorwayCopyright © 2025 Demers, Desjardins, Fauteux, Vézina, Tam and Berteaux. This is an open-access article distributed under the terms of the Creative Commons Attribution License (CC BY). The use, distribution or reproduction in other forums is permitted, provided the original author(s) and the copyright owner(s) are credited and that the original publication in this journal is cited, in accordance with accepted academic practice. No use, distribution or reproduction is permitted which does not comply with these terms.
*Correspondence: Rachel Demers, UmFjaGVsZGVtZXJzQG91dGxvb2suY29t; Dominique Berteaux, RG9taW5pcXVlX0JlcnRlYXV4QHVxYXIuY2E=
Disclaimer: All claims expressed in this article are solely those of the authors and do not necessarily represent those of their affiliated organizations, or those of the publisher, the editors and the reviewers. Any product that may be evaluated in this article or claim that may be made by its manufacturer is not guaranteed or endorsed by the publisher.
Research integrity at Frontiers
Learn more about the work of our research integrity team to safeguard the quality of each article we publish.