- 1Michael Sars Centre, University of Bergen, Bergen, Norway
- 2Department of Molecular Medicine, Institute of Basic Medical Sciences, University of Oslo, Oslo, Norway
A comparative overview is provided of Ca2+ signaling and its potential mechanistic roles during development in tunicates. As background, the review presents an introduction to tunicate taxonomy, and then a general overview of Ca2+ signaling and methods for recording and measuring Ca2+ signals. It then covers the dynamics and implicated mechanisms of Ca2+ signals during different phases of development from oocyte to larva. These include signals arising in the unfertilized oocyte, signals associated with fertilization and meiosis, intercellular signals occurring from early cleavage stages through gastrulation, intercellular signals during organogenesis, and signals associated with early behavior. Comparisons are made among different tunicate species and where relevant to other chordate species. In many tunicate species, Ca2+ currents across the oocyte membrane are present prior to fertilization, and in the appendicularian Oikopleura dioica regular Ca2+ transients have been recorded optically prior to fertilization. Ca2+ signals at this stage have been implicated in pre-fertilization oocyte maturation events. The fertilization transient is the most well-studied Ca2+ signal and is triggered by factors from the sperm, including pivotally a phospholipase C (PLC) isoform that catalyzes the generation of IP3, which elicits release of Ca2+ from the endoplasmic reticulum. Post-fertilization signals are similarly dependent on IP3 signaling and are regulated by cyclin-dependent kinase 1 (Cdk1), and thereby linked to the meiotic divisions required for zygote formation. Ca2+ signals associated with early cleavages through gastrulation arise in blastomeres of the muscle lineage and spread from these in a coordinated fashion to other blastomeres through gap junctions. Post-gastrulation Ca2+ signals begin to show tissue-specificity in their temporal pattern as organogenesis proceeds, likely associated with loss of general gap junction transmission. Once neurulation has occurred, Ca2+ signals arise first in the nervous system and are transmitted synaptically to muscle, while Ca2+ signals arising spontaneously in the epidermis follow a separate temporal pattern. Species differences in the spatiotemporal characteristics of pre- and postgastrulation Ca+2 signals are discussed.
Introduction
Calcium signaling is a universal feature of animal development and influences many developmental processes starting from oocyte maturation and fertilization and extending through organogenesis and cell differentiation. It has been investigated extensively in several chordate species at various development stages (reviewed in Webb and Miller, 2003; Whitaker, 2008).
As the invertebrate chordate clade most closely related phylogenetically to vertebrates (Delsuc et al., 2006, 2008), and with a relatively small number of constituent cells, tunicates provide a favorable model for studies of calcium signaling during development (McDougall et al., 2012). Indeed, some of the early studies of calcium signaling during development were carried out in tunicates (Block and Moody, 1987; Bosma and Moody, 1990; Speksnijder et al., 1990; Dale et al., 1991; Coombs et al., 1992; Arnoult and Villaz, 1994). This review provides an overview of current knowledge about the developmental dynamics and function of calcium signaling in tunicates.
Tunicate diversity
Tunicates are highly diverse in morphology, ecology and behavior, and encompass both sessile and pelagic forms (reviewed in Holland, 2016). They are commonly categorized into three classes, the sessile Ascidiacea and the pelagic Thaliacea and Larvacea (the latter also termed Appendicularia), although more robust cladistic groupings based on large-scale genomic analyses are now recognized (Appendicularia, Stolidobranchia, and Thaliacea + Phlebobranchia + Aplousobranchia, with the Ascidiacea being paraphyletic; Delsuc et al., 2018). Pending a more thorough resolution of evolutionary relationships, we use here the traditional (but admittedly outdated) taxonomic designations to illustrate general variations in developmental features. In ascidian, appendicularian and some thaliacean species, early development generates a free-swimming larval stage that exhibits the typical chordate body plan with a motile tail (some thaliaceans also employ sexual reproduction or an asexual budding reproductive mode that lacks a free-swimming larval stage). In appendicularians, the larval body plan is maintained in the adult (hence the name Larvacea), which remains individually mobile throughout life. Thaliaceans generally form pelagic colonies of tail-less adults, whereas ascidian larvae metamorphose into sessile bottom-dwelling adults with a sac-like body plan. Of relevance to the Ca2+ signaling events to be presented below, model tunicate species across taxa exhibit invariant cell lineages, most have a well-developed notochord, and a muscular tail is the main mode of locomotion.
Sources of Ca2+ signals
Intracellular Ca2+ signals (reviewed in Clapham, 2007) derive from two sources: 1) influx through the cell membrane mediated by Ca2+ channels, driven by the large electrochemical gradient for Ca2+, and 2) release from intracellular Ca2+ stores, principally the endoplasmic/sarcoplasmic reticulum but extending to other compartments such as mitochondria and lysosomes (Figure 1). Cell membrane influx can be mediated by voltage-gated Ca2+ channels (VGCs), mechanosensitive cation channels (MCCs, which pass both Ca2+ and other cations), ligand-gated Ca2+ channels (LGCs), and second messenger-activated Ca2+ channels, of which G-protein coupled receptors (GPCR) and calcium-activated Ca2+ channels are subcategories. Voltage-gated, mechanosensitive and ligand-gated Ca2+ channels have fast opening kinetics and generate inward Ca2+ currents of abrupt onset, variable duration (depending on the channel closing mechanisms) and localized action (since the Ca2+ concentration falls markedly within a few tens of nanometers from the inner surface of the cell membrane). Second messenger-activated Ca2+ channels are slower to open and close and typically generate more protracted Ca2+ signals.
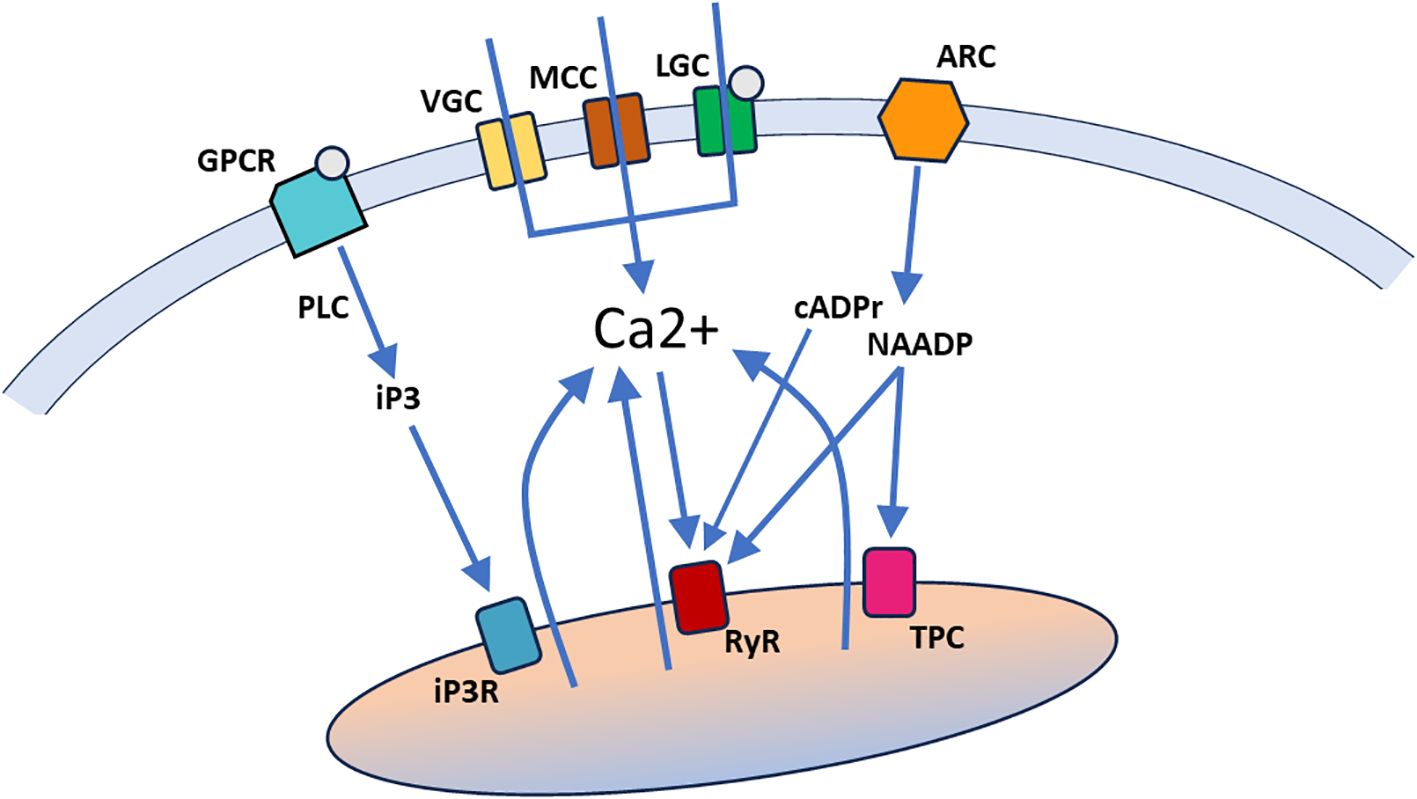
Figure 1. A general overview of Ca2+ signaling pathways. Ca2+ can enter the cell through ion channels in the cell membrane, including voltage-gated Ca2+ channels (VGC), mechanosensitive cation channels (MCC), and ligand-gated Ca2+ channels (LGC). Ca2+ can also be released from intracellular stores (indicated by a single organelle here that encompasses the various Ca2+ storing organelles), through binding of second messengers (iP3, cADPr, NAADP and Ca2+ itself) to specific receptors in the organelle membrane. Ligand binding by G-protein coupled receptors in the cell membrane (GPCR) activate phospholipase C (PLC) to generate iP3, which can bind to iP3 receptors (iP3R) in the endoplasmic/sarcoplasmic reticulum. Activation of ADP-ribosyl cyclases (ARC) in the cell membrane generates cADPr and NAADP, both of which bind to ryanodine receptors (RyR) in the endoplasmic/sarcoplasmic reticulum. NAADP also binds to two-pore channels (TPC) in acidic lysosomes.
Release from intracellular Ca2+ stores depends on second messenger systems and thus generates Ca2+ signals with a more gradual onset and relatively long durations. In addition, these signals can spread through a larger cytoplasmic volume due to the extent of the storage organelle(s) and the amplifying nature of the second messenger systems. The principal second messengers are inositol trisphosphate (IP3), generated by different isoforms of phospholipase C (PLC), cyclic ADP-ribose (cADPr) and nicotinic acid adenine dinucleotide phosphate (NAADP), generated by ADP-ribosyl cyclases (ARCs), and Ca2+ itself (Galione, 2019; Shah et al., 2022; Kim, 2022; Guse, 2023). To effect Ca2+ release, IP3 binds to IP3 receptors (IP3Rs) on the endoplasmic/sarcoplasmic reticulum, cADPr, NAADP and Ca2+ bind to ryanodine receptors (RYRs) on the endoplasmic/sarcoplasmic reticulum, and NAADP also binds to two-pore channels on acidic lysosomes (Figure 1). The full picture of regulated release from intracellular Ca2+ stores is more complex, but the general overview in Figure 1 provides sufficient context for the discussion of Ca2+ signal mechanisms in tunicate embryos below.
Methods for studying calcium signaling
Calcium signals involve the movement of Ca2+ ions across organelle and cell membranes by dint of calcium channels, pumps and antiporters, the diffusion of Ca2+ ions through gap junctions and over limited distances within the cytoplasm, and the binding of Ca2+ ions to specific calcium buffer and signaling proteins. Because Ca2+ is a universal intra- and intercellular signaling molecule, involved in a myriad of cell functions including cytoskeletal dynamics, cell adhesion, synaptic transmission and muscle contraction, its movement and distribution is highly regulated.
Methods for registering and measuring calcium signals take advantage of either the ionic charge of Ca2+ or the binding of Ca2+ to specific calcium-binding fluorophores or fluorescent proteins. In the former case, electrodes can be used to measure Ca2+ currents and/or resultant membrane potential changes with very high temporal resolution (limited by the electronics used, typically at sub-microsecond levels). A commonly used technique is patch clamp recording, which can measure Ca2+ currents through large populations of Ca2+ channels (as in whole cell recordings) and down to single channels (if only a single or a very few channels are opened or closed within the patch of membrane recorded from) (Cuomo et al., 2006; Gallo et al., 2013; Carvacho et al., 2018). Other types of electrode-based recording can also be used. For example, a vibrating probe system was developed to measure Ca2+ current densities across cell membranes (Jaffe and Nuccitelli, 1974), including associated with the activation current following fertilization of fish oocytes (Nuccitelli, 1987).
Calcium binding to calcium-sensitive fluorophores and fluorescent proteins has been utilized to transform Ca2+ signals into fluorescent signals that can be captured optically (Paredes et al., 2008; de Melo Reis et al., 2020). Calcium-sensitive fluorophores such as Fura-2, Fluo-4 and Calcium Green are organic molecules which, when photo-excited, emit fluorescence of specific wavelengths in a manner that is calcium-dependent. The kinetics of their response to changes in Ca2+ levels vary over a domain spanning milliseconds, limiting the temporal resolution obtainable relative to electrophysiological measurements, but still capable of discriminating signals such as broad calcium action potentials or protracted synaptic potentials. Most calcium-sensitive fluorophores (and fluorescent proteins, see below) only report relative changes in Ca2+ concentration. Fura-2 is an example of a calcium-sensitive fluorophore with a bimodal excitation spectrum, which permits ratiometric measurements that can be used to quantitate the concentration of Ca2+ ions at a given time point (Paredes et al., 2008).
Calcium-sensitive fluorescent proteins exist in nature and are intrinsically fluorescent or bioluminescent with an intensity that is dependent on Ca2+ concentration. Aequorin was the first such protein to be used for imaging Ca2+ signals, particularly in connection with the fertilization of oocytes (Ridgway et al., 1977; Steinhardt et al., 1977). More recently, genetic engineering has been used to create specially designed calcium-sensitive fluorescent proteins through combination of calcium-binding protein domains with an intrinsically fluorescent protein (Zhang et al., 2002). The advantage of engineered calcium-sensitive fluorescent proteins is that their expression can be genetically targeted to specific cell types. The GCaMP family of calcium-sensitive fluorescent proteins has become a particularly popular tool for imaging Ca2+ signals in a variety of organisms and cells (Kettunen, 2020; Shemesh et al., 2020) and encompasses variants of different sensitivities and kinetics. In particular, GCaMP has been used to assess Ca2+ signals during early embryogenesis in both vertebrates (Chen et al., 2017) and tunicates (Akahoshi et al., 2017; Mikhaleva et al., 2019; Tolstenkov et al., 2022). As is the case for calcium-sensitive fluorophores, calcium-sensitive fluorescent proteins have much slower kinetics than electrophysiological measurements but nevertheless can in many cases discriminate individual Ca2+ signals, especially when combined with temporal deconvolution methods (de Melo Reis et al., 2020).
Calcium signaling from oocyte to first cleavage
Pre-fertilization
Calcium is known to play an important role in oocyte maturation and the progression through meiotic arrest (Whitaker, 2008; Gallo et al., 2013). In line with this, Ca2+ currents and signals have been detected in ascidian and appendicularian oocytes prior to fertilization (Block and Moody, 1987; Moody and Bosma, 1989; Bosma and Moody, 1990; Dale et al., 1991; Coombs et al., 1992; Arnoult and Villaz, 1994; Silvestre et al., 2009; Tosti et al., 2011; Gallo et al., 2013; Mikhaleva et al., 2019). All but the most recent of these studies employed electrophysiological measurements to demonstrate the presence in the oocyte membrane of either mechanosensitive non-selective cation channels (which pass Ca2+ in addition to Na+ and K+) or voltage-sensitive Ca2+ channels, as well as other voltage-sensitive monovalent cation channels. These studies characterized channel types and kinetics and showed that Ca2+ currents depended on extracellular Ca2+ but did not describe the developmental dynamics of pre-fertilization calcium signals. Mikhaleva et al. (2019) employed Fluo-4 or GCaMP6 to visualize these signals in unfertilized oocytes of the appendicularian Oikopleura dioica and showed that regularly repetitive Ca2+ transients occur at a frequency of about one every 2-3 minutes. The transients have a stereotypical double-peaked waveform consisting of a minor peak lasting about 3 sec followed after a delay of 10-15 sec by a 20-fold higher amplitude major peak lasting 8-10 sec. They are always initiated at a focal point near the inner surface of the oocyte and spread from there throughout the oocyte as a wave.
It is unclear what initiates these pre-fertilization signals in Oikopleura, in particular whether the trigger is intrinsic or extrinsic, such as mechanical or chemical stimulation associated with spawning. In this context it is of interest to compare how appendicularian and ascidian oocytes are obtained experimentally prior to calcium imaging. In Oikopleura, natural spawning in free-swimming adults involves wholescale rupture of the gonads, a traumatic and life-terminating event. In the lab this can be induced by mechanical agitation. In both cases, oocytes are violently released en masse, likely resulting in substantial mechanical stimulation, although some protection of the oocyte membrane is afforded by the chorion. By contrast, in ascidians, natural and laboratory-based spawning involves a gradual, enzyme-mediated release of individual follicles from the ovary of sessile adults (Matsubara et al., 2019), which likely has a much milder mechanical impact on the oocytes. Mikhaleva et al. (2019) discuss the possibility that mechanical stimulation of oocytes arising from induced spawning, impalement with the electrodes used to inject GCaMP6 mRNA or the dechorionation necessary to allow loading with Fluo-4 might be stimuli that initiate pre-fertilization signals in Oikopleura. Irrespective of how these signals are initiated in the experimental context, it is clear that they do not depend on fertilization and exhibit regular, clock-like dynamics and a stereotypical waveform that are not disrupted by the fertilization transient (although there may be a resetting of the clock; Figure 2; Mikhaleva et al., 2019).
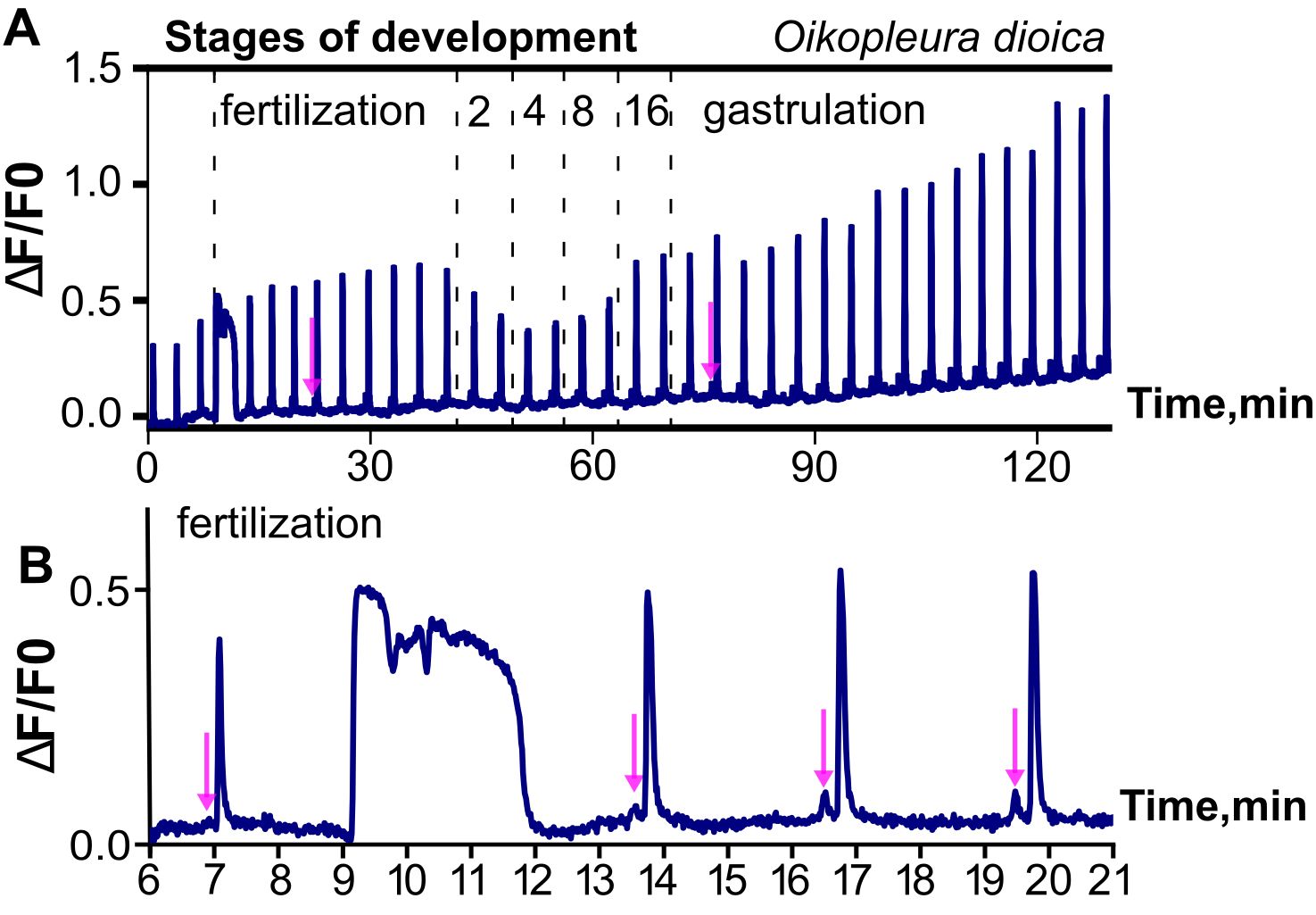
Figure 2. Illustration of ongoing, regular Ca2+ signals from pre-fertilization to gastrula stages of Oikopleura dioica. Ca2+ signals are represented as fractional changes of fluorescence (F) over a baseline (deltaF/F0). (A) Note the highly regular, repetitive nature of the signals, which begin in the unfertilized oocyte and continue as coordinated intercellular signals as the embryo develops, interrupted only by the fertilization transient. (B) Higher magnification highlights the much longer time course of the fertilization transient, and the double-peak waveform of the regular calcium signals, which begin with a small “mini-peak” (indicated by arrows in both A, B) followed by a main peak. Modified from Mikhaleva et al. (2019).
Fertilization transient
Calcium plays a major role in polyspermy block at fertilization, by dint of the generation of a large, global Ca2+ transient that arises shortly after sperm contact, which initiates a chain of events that creates a barrier to penetration by later-arriving sperm. The fertilization transient is arguably the most studied Ca2+ signal in development and has been characterized in many invertebrate and vertebrate species (reviewed in Stricker, 1999; Nixon et al., 2000; Runft et al., 2002; Whitaker, 2006; Carvacho et al., 2018). In tunicates, the fertilization transient has been studied extensively in ascidians, including Ciona robusta (Ciona intestinalis type A), Ciona intestinalis (Ciona intestinalis type B), Ciona savignyi, Ascidiella aspersa and Phallusia mamillata (Hirano and Takahashi, 1984; Dale and DeFelice, 1984; Speksnijder et al., 1989; Brownlee and Dale, 1990; Speksnijder, 1992; Goudeau and Goudeau, 1993; Goudeau et al., 1994; Lambert et al., 1994; McDougall et al., 1995; Cuomo et al., 2006; Tosti et al., 2011; reviewed in Dumollard et al., 2004). It has the following general features conserved across tunicate species: 1) it is initiated at the site of sperm entry; 2) it involves multiple sources of Ca2+, including influx of extracellular Ca2+ through voltage-gated and potentially other types of Ca2+ channels in the oocyte membrane and a primary IP3-mediated release of Ca2+ from the endoplasmic reticulum triggered by sperm-derived factors introduced into the egg cytoplasm; 3) it lasts for about 3-5 min (reviewed in Dumollard et al., 2004; Whitaker, 2008). The rise of Ca2+ concentration during the fertilization transient is necessary for subsequent events that prevent polyspermy, such as the release of N-acetylglycosiminidase, which inactivates sperm receptors in the vitelline coat (Lambert et al., 1994; McDougall et al., 1995). In addition, ion depletion experiments show that an inward Na+ current contributes to the fertilization transient in Ciona, and that when Na+ is removed extracellularly the transient is diminished and development is defective (Tosti et al., 2011).
As in other species (both vertebrate and invertebrate), the fertilization transient in ascidians is triggered by the PLC-mediated generation of IP3 and binding of IP3 to IP3Rs on the endoplasmic reticulum (reviewed in Runft et al., 2002; Dumollard et al., 2004). In mouse and human, injection of the PLC zeta 1 isoform (PLCζ1), triggers the fertilization transient, and genetic knockout of PLCζ1 in mouse sperm prevents it (reviewed in Carvacho et al., 2018). It has been speculated that PLCζ1 is also the relevant sperm factor in tunicates (Dale et al., 2010), but this has not yet been confirmed. In ascidian oocytes, injection of IP3 is sufficient to initiate a fertilization transient, and specific blockade of PLC gamma (PLCγ) or Src protein tyrosine kinases prevents the fertilization transient, implicating these enzymes in the IP3 transduction signal (Runft and Jaffe, 2000). By contrast, inhibition of RyR does not prevent the fertilization transient (reviewed in Runft et al., 2002). Thus, the primacy of the IP3 pathway evidently is conserved in tunicates and non-tunicate species, although the PLC isoforms involved differ.
Post-fertilization signals prior to first cleavage
Most studies that have characterized the fertilization transient in tunicates note that it is followed by a series of highly regular Ca2+ signals during ensuing meiosis and cleavage (Figure 3, Speksnijder et al., 1989; Speksnijder, 1992; Mikhaleva et al., 2019: Matsuo et al., 2020). In the appendicularian Oikopleura, the regular post-fertilization signals are indistinguishable in frequency and waveform from the pre-fertilization signals (Figure 1; Mikhaleva et al., 2019). Moreover, in ascidians, the post-fertilization signals are similar to the pre- and post-fertilization signals in Oikopleura in that they are regular and have a much shorter time-course than the fertilization transient (reviewed in Dumollard et al., 2004). However, they appear to lack the doublet waveform seen in Oikopleura (Mikhaleva et al., 2019; an absence that may be artefactual depending on the temporal resolution of the Ca2+ recording used). The similarity of pre- and post-fertilization transients across tunicate classes suggests that they represent the same phenomenon and may occur independently of the fertilization transient rather than being necessarily triggered by it. Indeed, it is clear in Oikopleura that the fertilization transient arises on a background of regular signals that spans from pre- to post-fertilization, and neither initiates these nor alters their character once it has occurred (Mikhaleva et al., 2019).
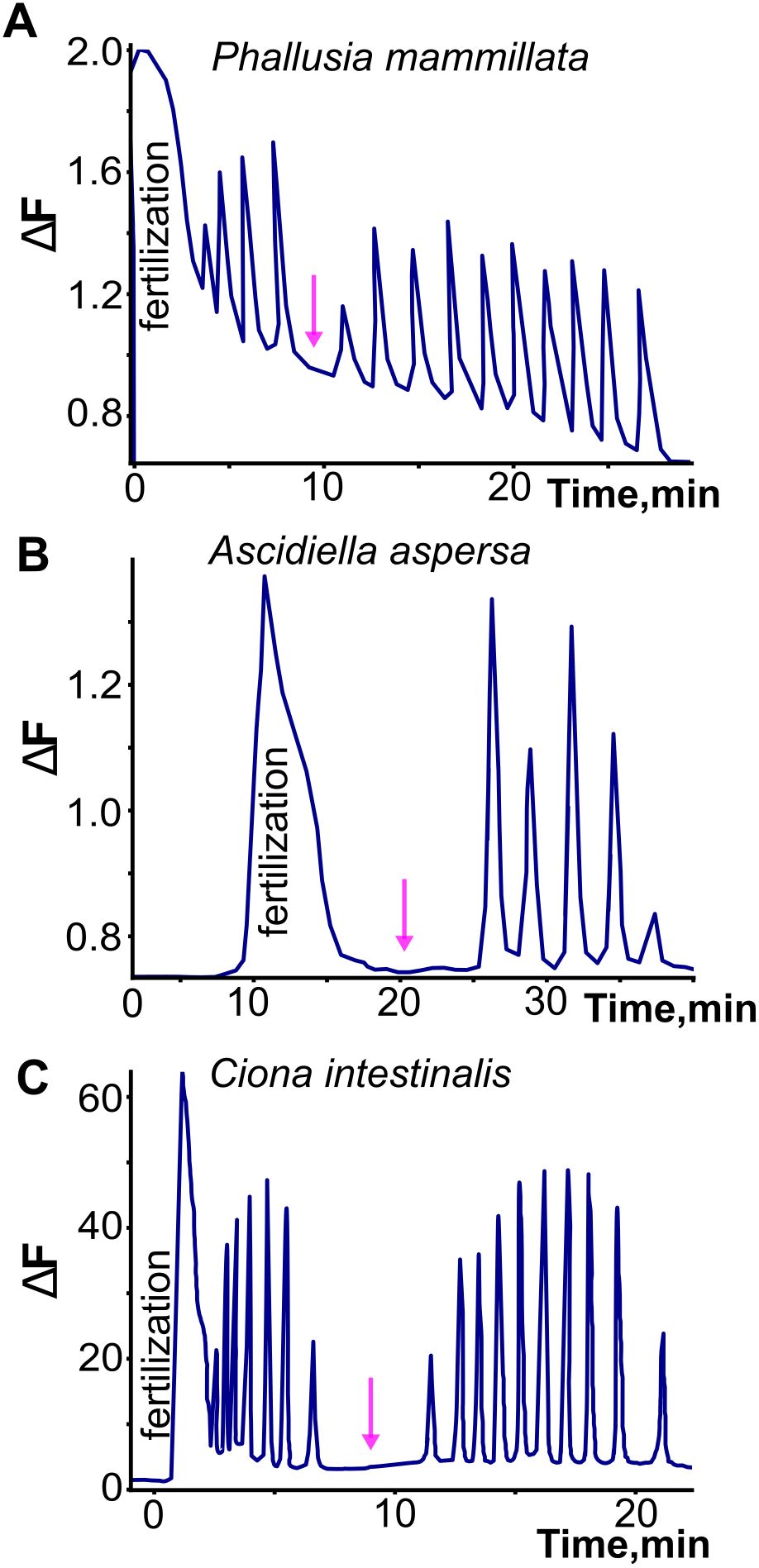
Figure 3. Illustration of the fertilization transient and subsequent post-fertilization signals in three ascidian species. Note the longer time course of the fertilization transient, and the regularity of the post-fertilization signals. In contrast to what has been observed in the appendicularian Oikopleura dioica, the post-fertilization signals are interrupted by a pause (indicated by arrows) to form two separate trains, related respectively to the two meiotic divisions that occur after the fertilization transient. Adapted and redrawn from Dumollard et al. (2004).
In ascidians, the post-fertilization signals have been discriminated into two different series separated by a pause, with different pacemakers, related respectively to the first and second meiotic divisions (reviewed extensively in Dumollard et al., 2004). They are reported to cease after the second meiotic division. These temporal features differ from the unabated continuation of the post-fertilization signals into subsequent cleavage stages in the appendicularian Oikopleura (Mikhaleva et al., 2019, see below). Whereas the first series of post-fertilization signals in ascidians originate like the fertilization transient from the sperm entry site, the second series shifts to a different origin, within the cortex of the vegetal contraction pole. This region is rich in mitochondria and contains the so-called myoplasm, where maternal determinants of muscle and endoderm are concentrated. Both origins contain endoplasmic reticulum that provide the source of Ca2+ released by IP3 (reviewed in Dumollard et al., 2004).
The molecular mechanisms underlying the post-fertilization signals that regulate meiosis have been studied extensively in ascidians (McDougall and Sardet, 1995; Albrieux et al., 1997, 1998; Albrieux and Villaz, 2000; Levasseur and McDougall, 2000; Wilding et al., 2000; Carroll et al., 2003; Levasseur et al., 2007, 2013; Lambert, 2011), less so in appendicularians (Matsuo et al., 2020). Pharmacological block of IP3Rs using the competitive inhibitor heparin prevents the post-fertilization signals, and UV-stimulated release of caged IP3 is sufficient to trigger similar series of signals and the associated meiotic divisions, with a single large UV pulse sufficient for the first series and additional pulses necessary for the second (McDougall and Sardet, 1995). Direct injection of IP3 through a patch pipette also elicits similar series of signals (Albrieux et al., 1997), whereas injection of NAADP can inhibit them, presumably through depletion of the NAADP-dependent Ca2+ store (Albrieux et al., 1998). Neither injection of ryanodine nor of cADPr elicits the series, corroborating the primacy of IP3 signaling underlying them (Albrieux et al., 1997). The durations of the series are correlated with and regulated by cyclin-dependent kinase 1 (Cdk1) activity, underscoring their tight relationship to the meiotic cell cycle, and possibly extending that relationship to mitotic divisions (McDougall and Levasseur, 1998; Levasseur and McDougall, 2000). Inhibiting Cdk1 eliminates the Ca2+ signals, but not the ability of IP3R agonists to elicit them (Levasseur et al., 2007). Evidently, Cdk1 promotes IP3 formation rather than acting directly on IP3R-mediated Ca2+ release.
The results of these mechanistic studies have in several instances informed similar studies in vertebrates. The study of Ca2+ signaling in tunicates have thus contributed significantly to the overall view of the role of calcium in the early embryo (reviewed in Whitaker, 2008).
Calcium signaling from first cleavage to gastrulation
Although post-fertilization Ca2+ signals have been reported to cease after the second meiosis in several studies of ascidians, Ca2+ is known to play a pivotal role in mitosis (reviewed in Nugues et al., 2022), and calcium signaling elements, including voltage-sensitive Ca2+ channels and ryanodine receptor-sensitive and IP3-sensitive internal release, have been demonstrated in 2-cell embryos of the ascidians Boltenia villosa and Phallusia mammillata (Coombs et al., 1992; Albrieux and Villaz, 2000). Oscillating Ca2+ currents carried by T-type Ca2+ channels in the cell membranes have been recorded during early cleavage stages of Styela plicata, and pharmacological blockade of these channels reduces the rate of cleavage and disrupts normal embryonic development (Gallo et al., 2013). As noted above, in the appendicularian Oikopleura dioica post-fertilization calcium signals continue throughout the mitotic divisions that generate the blastula and gastrula (Mikhaleva et al., 2019). These are not linked temporally to cleavages but follow rather the distinctly different clock already established prior to fertilization (once every 2-3 min, as opposed to mitotic cleavages occurring once every 13 min). They exhibit a stereotyped, sequential pattern related to stage and tissue lineage (Figure 4). At the 2-cell stage, a Ca2+ signal is first generated in one blastomere and then spreads to the other. The order is not obligatory, as the two blastomeres can switch in taking the lead. At the 4-cell stage, a Ca2+ signal is first generated in one blastomere before spreading into a neighboring blastomere (either a sister or a non-sister blastomere), before spreading from these into the other pair of blastomeres in sequence. At the 8-cell stage, Ca2+ signals arise in two of the posterior blastomeres and spread from these in a coordinated manner to the other blastomeres. By the 16-cell stage, this temporal pattern achieves a clear tissue type relationship, as the initiation is always in the blastomeres giving rise to the muscle cell lineage (Mikhaleva et al., 2019). This may be related to the shift in post-fertilization Ca2+ signal origin to the myoplasm of the uncleaved ascidian oocyte, suggesting a link between early Ca2+ signaling and body pattern that begins already when ooplasm is reorganized prior to the first cleavage. During subsequent divisions up to gastrulation, the muscle lineage-specific origin is maintained in Oikopleura (Mikhaleva et al., 2019). The muscle cell lineage is also where Ca2+ signals are initiated in gastrulae of Ciona (Akahoshi et al., 2017), is where a coupling of voltage-gated Ca2+ channels to ryanodine receptors has been characterized in Halocynthia roretzi (Nakajo et al., 1999) and is the specific site of voltage-gated Ca2+ currents recorded electrophysiologically after gastrulation in Boltenia villosa (Simoncini et al., 1988). Thus, the muscle cell lineage as the origin of whole-embryo Ca2+ signals through gastrulation appears to be a conserved feature in tunicates.
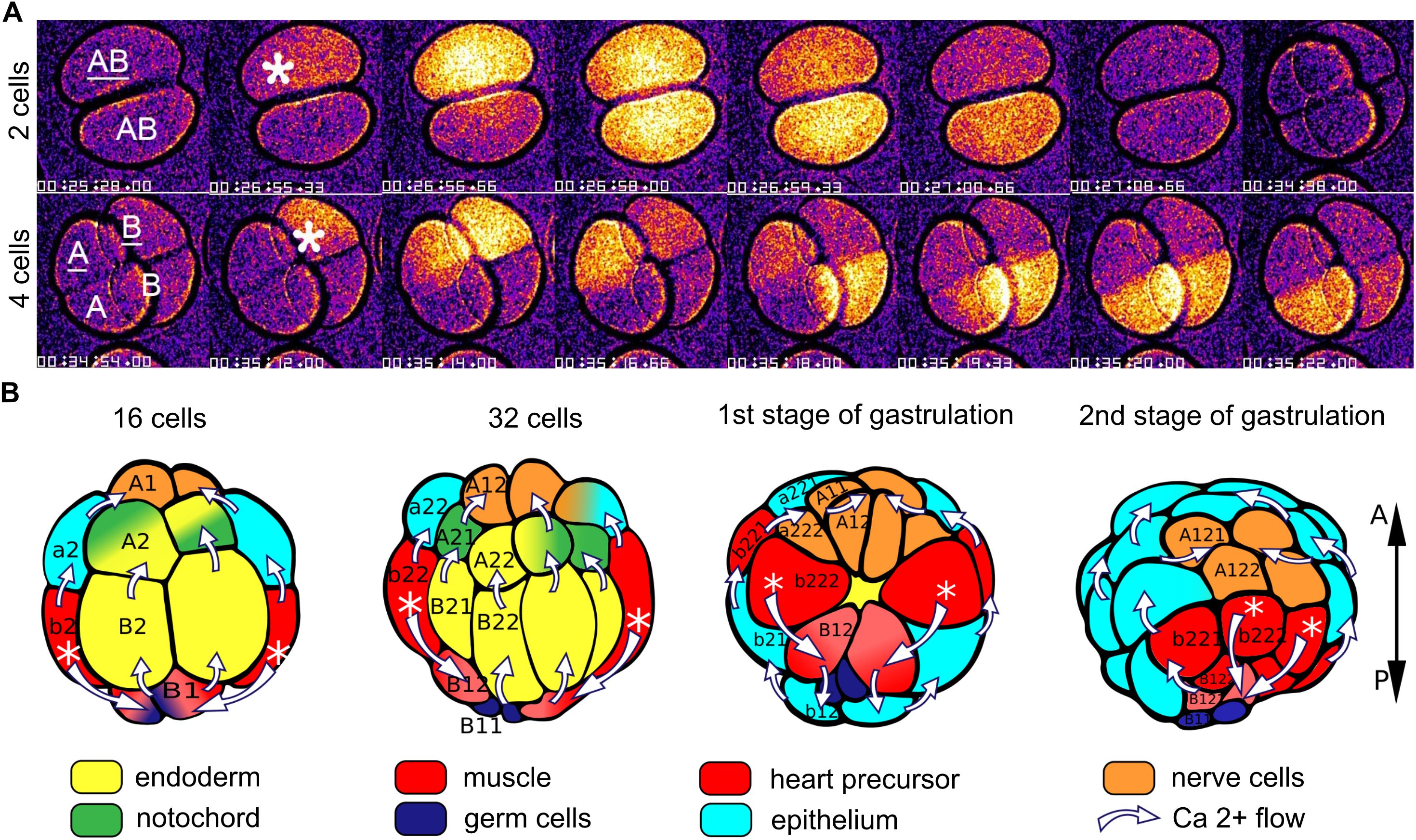
Figure 4. Illustration of intercellular Ca2+ signals in Oikopleura dioica. (A) Video recordings of Ca2+ signals in 2- and 4-cell embryos, showing the spread from the single blastomere of origin (indicated by asterisk) to the other blastromeres. (B) Diagrammatic representation of the intercellular spread of Ca2+ signals at later stages, oriented according to the anteroposterior (A-P) axis at the right. The white asterisks indicate the origin of the signals (in cells that give rise to the muscle cell lineage) and the white arrows indicate the trajectory of the signals among the cells. Tissue types are color coded as shown. Modified from Mikhaleva et al. (2019), Figures 2, 3.
Contrasting with the description in Oikopleura, Ca2+ signals in gastrulae of Ciona were not reported to follow a patterned sequence, but rather to arise in separate, independent cells (Akahoshi et al., 2017). This is likely due to a lower temporal resolution in the Ciona study (0.5-1 frames/second, as opposed to 4 frames/second used by Mikhaleva et al., 2019).
Throughout development to gastrulation in Oikopleura, the regular, spontaneous Ca2+ signals exhibit the same waveform as first seen pre-fertilization, with a small “mini-peak” followed by a larger, main peak (Mikhaleva et al., 2019). Pharmacological blockade of Ca2+ channels (using divalent cations or the more specific T-type Ca2+ channel blocker mibefradil) has various effects on the amplitude and shape of the signals, and in the case of the divalent cations Cd2+ and Ni2+ disrupts the coordinated progression of the Ca2+ signals among the blastomeres. Ca2+ channel blockade also leads to perturbed and arrested development, underscoring the importance of Ca2+ signaling in regulating early developmental processes. A similar effect of mibefradil has been reported in Styela plicata, in which it diminishes voltage gated Ca2+ currents recorded electrophysiologically, slows the cleavage rate and disrupts larval development (Gallo et al., 2013).
The stereotyped pattern of Ca2+ signal progression, always starting in cells of the muscle lineage and spreading from these throughout the embryo in a choreographed sequence, suggests a selective intercellular communication, and prompted Mikhaleva et al. (2019) to test whether gap junctional signaling is involved. This they did by knocking down the expression of the two (of 29 total) connexin genes that are expressed at early developmental stages in Oikopleura, Od-CxA and Od-CxB, through injection of inhibitory dsRNA into oocytes prior to fertilization. No effects were seen through the 32-cell stage, as prior to this the embryo is still supplied by maternally derived transcripts. But by gastrulation, Od-CxA and Od-CxB transcripts were diminished to 20-50% of normal levels. The effect was a severe disruption of Ca2+ signal progression from cell to cell, which was slowed by a factor of 10, and perturbed development (failure of ingression and epiboly at gastrulation, and later tail structure defects in embryos that succeeded to gastrulate). Thus, the patterned intercellular spread of Ca2+ signals depends on the presence of gap junctions, and its disruption is paralleled by abnormal development.
Calcium signaling post-gastrulation
The characteristics and functions of Ca2+ signaling at post-gastrulation stages are less well studied and understood than at earlier stages, particularly in a comparative context (reviewed in Slusarski and Pelegri, 2007; Webb et al., 2005). In vertebrates, Ca2+ transients have been shown to be associated with, and in some cases mechanistically linked to, convergent extension of the body axis (Créton et al., 2000; Prudent et al., 2013; Wallingford et al., 2001; Whitaker, 2006), the establishment of left-right asymmetry (Chuang et al., 2007; Kreiling et al., 2008; Onuma et al., 2020), neural induction and neurulation (Abdul-Wajid et al., 2015; Christodoulou and Skourides, 2015; Créton et al., 1998; Gilland et al., 1999; Leclerc et al., 2000; Moreau et al., 1994; Smedley and Stanisstreet, 1985), somitogenesis (Cheung et al., 2011; Webb et al., 2012; Webb and Miller, 2011) and muscle cell differentiation (Ferrari and Spitzer, 1999; Ohtsuka and Okamura, 2007; Webb et al., 2012).
Calcium signaling during neurulation
The role of Ca2+ signaling during the process of neurulation has been studied in Ciona (Abdul-Wajid et al., 2015; Hackley et al., 2013). Abdul-Wajid et al. (2015) have demonstrated a requirement for Ca2+ currents mediated by a T-type calcium channel (coded by the CAV3 gene) for proper closure of the anterior neural tube. When expression of CAV3 is prevented by the bugeye mutation, the normal downregulation of the ephrinA-d gene at the end of neurulation is prevented. This disrupts EphrinA-EphA-mediated cell-cell interactions, which are pivotal for neural tube closure. Inhibition of EphrinA activity rescues the bugeye phenotype. Treatment of embryos with mibefradil phenocopies the bugeye mutation, supporting the importance of T-type Ca2+ channels in mediating ephrinA-d downregulation. Using morpholino-based knockdown of CAV3 homologues in Xenopus laevis embryos, Abdul-Wajid et al. (2015) showed that the dependence of anterior neural tube closure on T-type Ca2+ channel activity is conserved from tunicates to vertebrates.
Another mutation in Ciona intestinalis, frimousse, leads to abnormal specification of anterior neural plate cells, which adopt an epidermal rather than a neural fate. The frimousse mutation lies in a connexin gene (cx11) and abolishes Ca2+ signals in the anterior neural plate (Hackley et al., 2013). Pharmacological blockade of gap junctions and exposure to low-Ca2+ sea water phenocopy the mutation, underscoring the essential role of gap junction-mediated intercellular Ca2+ signals in core developmental processes.
Calcium signaling during general organogenesis
A complete assessment of the roles of Ca2+ during post-gastrulation development requires the recording of Ca2+ signals throughout the organism. Only a few studies have characterized whole-body Ca2+ signaling spanning extended periods of post-gastrulation development, and in only a few species (zebrafish: Créton et al., 1998; Gilland et al., 1999; Cheung et al., 2011; Webb et al., 2012; Tsuruwaka et al., 2017; Xenopus: Leclerc et al., 2000; Ciona: Abdul-Wajid et al., 2015; Akahoshi et al., 2017; Oikopleura: Tolstenkov et al., 2022).
Whole-embryo Ca2+ signals have been characterized in various tissues including the epidermis, palps, central nervous system, peripheral nervous system, muscle, heart, and notochord of Ciona, through expression of the genetically encoded Ca2+ indicator GCaMP (Abdul-Wajid et al., 2015; Akahoshi et al., 2017). At the early tailbud stage, oscillating Ca2+ signals have been observed at the midbrain-hindbrain boundary, in differentiating neuronal precursors in the neural plate and nerve cord, and in a rhythmic pattern in a small population of neurons in the visceral ganglion, an activity suggestive of the central pattern generator for swimming (Abdul-Wajid et al., 2015; Akahoshi et al., 2017). At the mid-tailbud stage, Ca2+ signals have been observed in epidermal cells and in posterior notochord cells. Starting at the late tailbud stage, frequent Ca2+ signals have been observed in the tail musculature during maturation of the muscle cells, corroborating an earlier study that showed that Ca2+ influx through voltage-gated Ca2+ channels was associated with muscle cell development (Ohtsuka and Okamura, 2007).
In Oikopleura, whole-embryo signals have been characterized using GCaMP6 expressed throughout the body (Tolstenkov et al., 2022). Initially, Ca2+ signals originate in the muscle cells and spread from these into other tissues (Figures 5, 6), continuing the situation through gastrulation in which Ca2+ signals originate from blastomeres of the muscle lineage and spread from these to other blastomeres. The similar spread of Ca2+ signals from differentiated muscle cells to other parts of the body is likely the result of gap junction-mediated coupling across tissue types, although this has not been tested directly by connexin gene knockdown as it has at pre-gastrulation and gastrulation stages (Tolstenkov et al., 2022; Mikhaleva et al., 2019). However, as organogenesis proceeds, Ca2+ signals begin to originate instead in the central nervous system and then spread from there to the muscle cells (Figures 5, 6). At the same time, Ca2+ signals in the epithelium are no longer temporally coupled with the signals in the CNS-muscle axis. Tolstenkov et al. (2022) have proposed that this transition from a muscle origin to separate origins in the CNS and epithelium involves the selective loss of general gap junction-mediated coupling across different tissue types, supplanted by more tissue-specific coupling, perhaps mediated by tissue-specific connexin expression. This hypothesis will require extensive investigation to test, since the number of connexin genes expressed increases as different tissues develop.
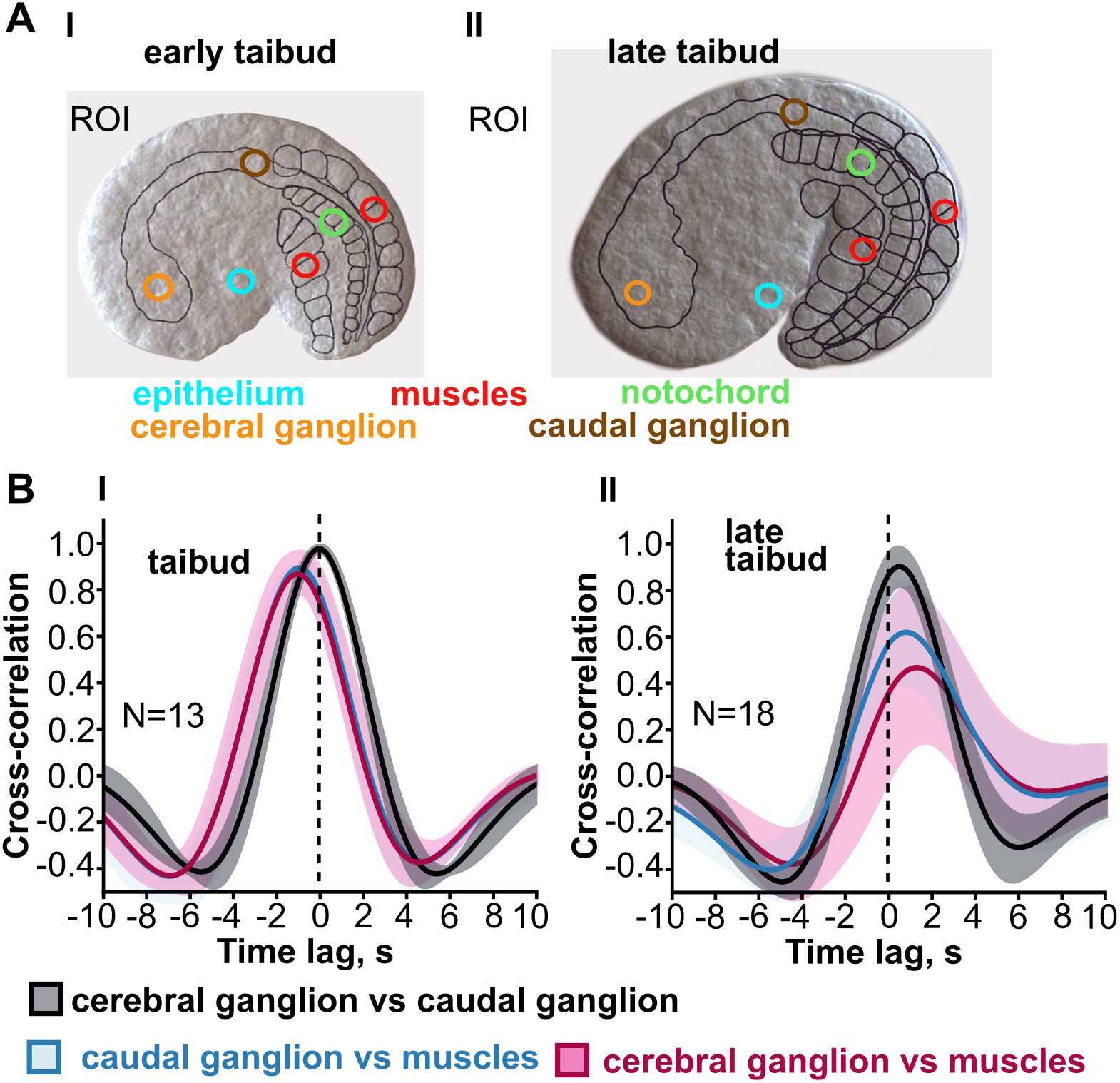
Figure 5. Transition from muscle lineage driven intercellular Ca2+ signals to neurally driven signals in Oikopleura dioica. (A) Signals were recorded from tissue-specific regions of interest (color coded in early and late tailbud embryos that express GCaMP6 throughout the body). (B) Cross-correlograms include (among others) the temporal relationship between signals in the cerebral ganglion and in muscle (maroon trace) and between signals in the caudal ganglion and muscle (blue trace). The time lag shift in these traces (in seconds, s) indicates that at the early tailbud stage Ca2+ signals in the tail muscle cells precedes that in either ganglion, whereas at the late tailbud stage the sequence is reversed. Modified from Tolstenkov et al. (2022), Figure 2.
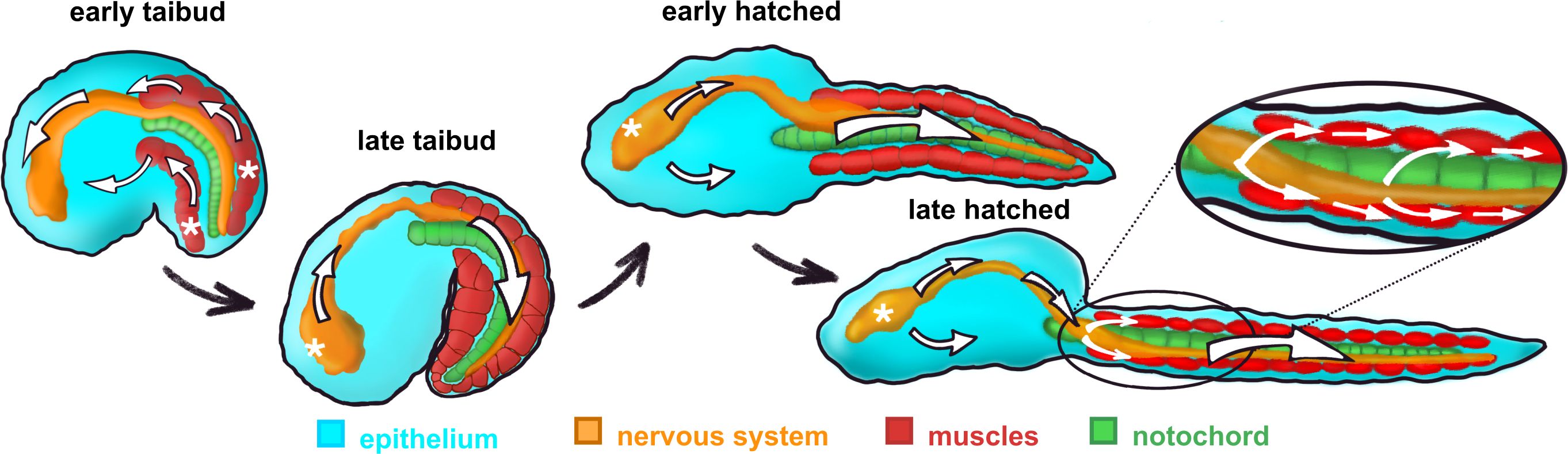
Figure 6. Diagrammatic illustration of the change in intercellular Ca2+ signal origin (white asterisks) and trajectory (white arrows) during post-gastrulation development in Oikopleura dioica. From Tolstenkov et al. (2022).
With respect to the CNS-muscle axis, the reversal of direction evidently relates to the development of neuromuscular synapse function. The transition is temporally linked to the developmental appearance of neuromuscular junctions (Søviknes et al., 2007), and the spread from CNS to muscle involves a much shorter temporal delay, consistent with neuromuscular synaptic transmission, and is prevented by pharmacological agents that block that transmission (Tolstenkov et al., 2022).
Calcium signals in the nervous system and relationship to early behavior patterns
In the neural plate of Ciona, Ca2+ signals appear in specific neural progenitor/neuronal precursor cells that evidently correspond to future motoneurons (MNs) and inhibitory interneurons (Akahoshi et al., 2017). Once the late tailbud stage is reached, repetitive Ca2+ signals appear in the visceral ganglion where MNs reside (Akahoshi et al., 2017), and Ca2+ signals also re-appear (after a period of quiescence at early tailbud stages) in the tail musculature. In Oikopleura dioica, the repetitive, neurally driven Ca2+ signals in the tail musculature are correlated with tail movements, and the signal in the muscle cells is protracted relative to the CNS signal, suggesting a link to excitation-contraction coupling, which lasts substantially longer than nerve impulse conduction and synaptic transmission. Tolstenkov et al. (2022) observed an interesting correlation between a given tail muscle contraction and the next initiating Ca2+ signal in the CNS: a new CNS signal arises shortly after and never before the cessation of the muscle signal and associated tail movement. A similar relationship has been observed in Ciona between Ca2+ signals recorded in a subpopulation of central neurons (GnRH+ neurons) and tail movements: a new signal in the neurons does not arise until the end of a tail movement (Okawa et al., 2020). This led Okawa et al. (2020) to postulate a sensory feedback loop that activates the central neurons once a tail movement is completed. An alternative explanation is that the cyclic nature of these phenomena is driven by an intrinsic clock that is independent of feedback signals. Of interest here is the observation by Tolstenkov et al. (2022) that the neurally driven Ca2+ signals have the same regularity as the earlier muscle-driven signals. One might expect that signals driven by sensory input would lose regularity and rather relate more to the vagaries of environmental conditions.
Calcium signals in the epidermis/epithelium
In both Ciona and Oikopleura, Ca2+ signals arise in the epidermis and epithelium, respectively, once these are established as definitive tissues (Akahoshi et al., 2017; Tolstenkov et al., 2022). Initially, signals in presumptive epidermis/epithelium are driven by the muscle lineage origin, but at about the time muscle signals begin to be driven by the CNS, the epidermal/epithelial signals begin to follow their own separate temporal pattern. At first haphazard, in separated individual cells, the signals eventually organize into a wave that typically starts in the head/trunk region and then travels caudally, often spreading into the notochord after reaching the tip of the tail. The function of these signals is unclear.
Conclusion
Calcium signaling in tunicates, as in other animal groups, plays a key role in a variety of developmental processes. From oocyte maturation to polyspermy block at fertilization, to the differentiation of specific cell types, in particular muscle cells, tunicates share calcium-dependent mechanisms that appear to be conserved throughout much of the chordate radiation. The evolutionary significance of other aspects of calcium signaling that have been characterized in tunicates deserves a more comprehensive assessment – both between different tunicate classes and between tunicates and vertebrates. Of note is the muscle lineage origin of whole embryo Ca2+ signals, clearly demonstrated in the appendicularian Oikopleura dioica, and its transition to neurally driven signals mediated by neuromuscular synaptic transmission. The role of gap junctions in coordinating the muscle lineage-driven whole embryo signals should be investigated in other tunicate species and compared to vertebrates. The same holds for the way this transitions to more tissue-specific signals, presumably through the regulation of tissue-specific connexin expression. The nature and underlying mechanisms of the pacemakers that drive Ca2+ signals, both in the single oocyte and in the multicellular embryo, need to be elucidated in more detail, again in a comparative context. As the embryo matures, the fate of the clock(s) that drive(s) intercellular Ca2+ signals needs to be determined, as the nervous system gains primacy in directing muscle activity, and other tissues and organs attain their specific functions. Of particular interest is the function of the primitive movements triggered by intrinsic waves of intercellular Ca2+ signals, as the forerunner of more complex, goal-directed movement repertoires that are engaged by sensory inputs. In all of these questions, tunicates – with their small larval size, low cell number, and rapid development - are well positioned to contribute insight into the developmental and evolutionary significance of calcium signaling in the chordate lineage.
Author contributions
JG: Writing – original draft, Writing – review & editing. OT: Writing – review & editing. YM: Writing – review & editing.
Funding
The author(s) declare financial support was received for the research, authorship, and/or publication of this article. Norwegian Research Council provided funding for salaries and research through grant number 234817.
Conflict of interest
The authors declare that the research was conducted in the absence of any commercial or financial relationships that could be construed as a potential conflict of interest.
Publisher’s note
All claims expressed in this article are solely those of the authors and do not necessarily represent those of their affiliated organizations, or those of the publisher, the editors and the reviewers. Any product that may be evaluated in this article, or claim that may be made by its manufacturer, is not guaranteed or endorsed by the publisher.
References
Abdul-Wajid S., Morales-Diaz H., Khairallah S. M., Smith W. C. (2015). T-type calcium channel regulation of neural tube closure and EphrinA/EPHA expression. Cell Rep. 13, 829–839. doi: 10.1016/j.celrep.2015.09.035
Akahoshi T., Hotta K., Oka K. (2017). Characterization of calcium transients during early embryogenesis in ascidians Ciona robusta (Ciona intestinalis type A) and Ciona savignyi. Dev. Biol. 431, 205–214. doi: 10.1016/j.ydbio.2017.09.019
Albrieux M., Lee H. C., Villaz M. (1998). Calcium signaling by cyclic ADP-ribose, NAADP, and inositol trisphosphate are involved in distinct functions in ascidian oocytes. J. Biol. Chem. 273, 14566–14574. doi: 10.1074/jbc.273.23.14566
Albrieux M., Sardet C., Villaz M. (1997). The two intracellular Ca2+ release channels, ryanodine receptor and inositol 1,4,5-trisphosphate receptor, play different roles during fertilization in ascidians. Dev. Biol. 189, 174–185. doi: 10.1006/dbio.1997.8674
Albrieux M., Villaz M. (2000). Bilateral asymmetry of the inositol trisphosphate-mediated calcium signaling in two-cell ascidian embryos. Biol. Cell. 92, 277–284. doi: 10.1016/S0248-4900(00)01066-2
Arnoult C., Villaz M. (1994). Differential developmental fates of the two calcium currents in early embryos of the ascidian Ciona intestinalis. J. Membr. Biol. 137, 127–135. doi: 10.1007/BF00233482
Block M. L., Moody W. J. (1987). Changes in sodium, calcium and potassium currents during early embryonic development of the ascidian Boltenia villosa. J. Physiol. 393, 619–634. doi: 10.1113/jphysiol.1987.sp016844
Bosma M. M., Moody W. J. (1990). Macroscopic and single-channel studies of two Ca2+ channel types in oocytes of the ascidian Ciona intestinalis. J. Membr Biol. 114, 231–243. doi: 10.1007/BF01869217
Brownlee C., Dale B. (1990). Temporal and spatial correlation of fertilization current, Ca2+ waves and cytoplasmic contraction in eggs of Ciona intestinalis. Proc. R Soc. Lond B 239, 321–328. doi: 10.1098/rspb.1990.0019
Carroll M., Levasseur M., Wood C., Whitaker M., Jones K. T., McDougall A. (2003). Exploring the mechanism of action of the sperm-triggered calcium-wave pacemaker in ascidian zygotes. J. Cell Sci. 116, 4997–5004. doi: 10.1242/jcs.00846
Carvacho I., Piesche M., Maier T. J., Machaca K. (2018). Ion channel function during oocyte maturation and fertilization. Front Cell Dev. Biol. 6, 63. doi: 10.3389/fcell.2018.00063
Chen J., Xia L., Bruchas M. R., Solnica-Krezel L. (2017). Imaging early embryonic calcium activity with GCaMP6s transgenic zebrafish. Dev. Biol. 430, 385–396. doi: 10.1016/j.ydbio.2017.03.010
Cheung C. Y., Webb S. E., Love D. R., Miller A. L. (2011). Visualization, characterization and modulation of calcium signaling during the development of slow muscle cells in intact zebrafish embryos. Int. J. Dev. Biol. 55, 153–174. doi: 10.1387/ijdb.103160cc
Christodoulou N., Skourides P. A. A. (2015). Cell-autonomous Ca2+ flashes elicit pulsed contractions of an apical actin network to drive apical constriction during neural tube closure. Cell Rep. 13, 2189–2202. doi: 10.1016/j.celrep.2015.11.017
Chuang C. F., VanHoven M. K., Fetter R. D., Verselis V. K., Bargmann C. I. (2007). An innexin-dependent cell network establishes left-right neuronal asymmetry in C. elegans. Cell 129, 787–799. doi: 10.1016/j.cell.2007.02.052
Coombs J. L., Villaz M., Moody W. J. (1992). Changes in voltage-dependent ion currents during meiosis and first mitosis in eggs of an ascidian. Dev. Biol. 153, 272–282. doi: 10.1016/0012-1606(92)90112-T
Créton R., Kreiling J. A., Jaffe L. F. (2000). Presence and roles of calcium gradients along the dorsal-ventral axis in Drosophila embryos. Dev. Biol. 217, 375–385. doi: 10.1006/dbio.1999.9542
Créton R., Speksnijder J. E., Jaffe L. F. (1998). Patterns of free calcium in zebrafish embryos. J. Cell Sci. 111, 1613–1622. doi: 10.1242/jcs.111.12.1613
Cuomo A., Silvestre F., De Santis R., Tosti E. (2006). Ca2+ and Na+ current patterns during oocyte maturation, fertilization, and early developmental stages of Ciona intestinalis. Mol. Reprod. Dev. 73, 501–511. doi: 10.1002/mrd.20404
Dale B., DeFelice L. J. (1984). Sperm-activated channels in ascidian oocytes. Dev. Biol. 101, 235–239. doi: 10.1016/0012-1606(84)90135-0
Dale B., Talevi R., DeFelice L. J. (1991). L- type Ca 2+ currents in ascidian eggs. Exp. Cell Res. 192, 302–306. doi: 10.1016/0014-4827(91)90190-6
Dale B., Wilding M., Coppola G., Tosti E. (2010). How do spermatozoa activate oocytes? Reprod. BioMed. Online. 21, 1–3. doi: 10.1016/j.rbmo.2010.02.015
Delsuc F., Brinkmann H., Chourrout D., Philippe H. (2006). Tunicates and not cephalochordates are the closest living relatives of vertebrates. Nature 439, 965–968. doi: 10.1038/nature04336
Delsuc F., Philippe H., Tsagkogeorga G., Simion P., Tilak M. K., Turon X., et al. (2018). A phylogenomic framework and timescale for comparative studies of tunicates. BMC Biol. 16, 39. doi: 10.1186/s12915-018-0499-2
Delsuc F., Tsagkogeorga G., Lartillot N., Philippe H. (2008). Additional molecular support for the new chordate phylogeny. Genesis 46, 592–604. doi: 10.1002/dvg.v46:11
de Melo Reis R. A., Freitas H. R., de Mello F. G. (2020). Cell calcium imaging as a reliable method to study neuron–glial circuits. Front. Neurosci. 14, 569361. doi: 10.3389/fnins.2020.569361
Dumollard R., McDougall A., Rouvière C., Sardet C. (2004). Fertilisation calcium signals in the ascidian egg. Biol. Cell. 96, 29–36. doi: 10.1016/j.biolcel.2003.11.002
Ferrari M. B., Spitzer N. C. (1999). Calcium signaling in the developing Xenopus myotome. Dev. Biol. 213, 269–282. doi: 10.1006/dbio.1999.9387
Galione A. (2019). NAADP receptors. Cold Spring Harb. Perspect. Biol. 11, a035071. doi: 10.1101/cshperspect.a035071
Gallo A., Russo G. L., Tosti E. (2013). T-type Ca2+ current activity during oocyte growth and maturation in the ascidian Styela plicata. PLoS One 8, e54604. doi: 10.1371/journal.pone.0054604
Gilland E., Miller A. L., Karplus E., Baker R., Webb S. E. (1999). Imaging of multicellular large-scale rhythmic calcium waves during zebrafish gastrulation. Proc. Natl. Acad. Sci. U.S.A. 96, 157–161. doi: 10.1073/pnas.96.1.157
Goudeau H., Depresle Y., Rosa A., Goudeau M. (1994). Evidence by a voltage-clamp study of an electrically mediated block to polyspermy in the egg of the ascidian Phallusia mammillata. Dev. Biol. 166, 489–501. doi: 10.1006/dbio.1994.1332
Goudeau M., Goudeau H. (1993). In the egg of the ascidian Phallusia mammillata, removal of external Ca2+ modifies the fertilization potential, induces polyspermy, and blocks the resumption of meiosis. Dev. Biol. 160, 165–177. doi: 10.1006/dbio.1993.1295
Guse A. H. (2023). Enzymology of Ca2+-mobilizing second messengers derived from NAD: from NAD glycohydrolases to (Dual) NADPH oxidases. Cells 12, 675. doi: 10.3390/cells12040675
Hackley C., Mulholland E., Kim G. J., Newman-Smith E., Smith W. C. (2013). A transiently expressed connexin is essential for anterior neural plate development in Ciona intestinalis. Development 140, 147–155. doi: 10.1242/dev.084681
Hirano T., Takahashi K. (1984). Comparison of properties of calcium channels between the differentiated 1 cell embryo and the egg cell of ascidians. J. Physiol. (Lond) 347, 301–325. doi: 10.1113/jphysiol.1984.sp015067
Jaffe L. F., Nuccitelli R. (1974). An ultrasensitive vibrating probe for measuring steady extracellular currents. J. Cell. Biol. 63, 614–628. doi: 10.1083/jcb.63.2.614
Kettunen P. (2020). Calcium imaging in the Zebrafish. Adv. Exp. Med. Biol. 1131, 901–942. doi: 10.1007/978-3-030-12457-1_36
Kim U. H. (2022). Roles of cADPR and NAADP in pancreatic beta cell signaling. Cell Calcium 103, 102562. doi: 10.1016/j.ceca.2022.102562
Kreiling J. A., Balantac Z. L., Crawford A. R., Ren Y., Toure J., Zchut S., et al. (2008). Suppression of the endoplasmic reticulum calcium pump during zebrafish gastrulation affects left-right asymmetry of the heart and brain. Mech. Dev. 125, 396–410. doi: 10.1016/j.mod.2008.02.004
Lambert C. C. (2011). Signaling pathways in ascidian oocyte maturation: the roles of cAMP/Epac, intracellular calcium levels, and calmodulin kinase in regulating GVBD. Mol. Reprod. Dev. 78, 726–733. doi: 10.1002/mrd.v78.10/11
Lambert C. C., Gonzales G. P., Miller K. M. (1994). Independent initiation of calcium dependent glycosidase release and cortical contractions during the activation of ascidian eggs: (Ascidian, Ryanodine, Thimerosal, Thapsigargin, N-acetylglucosaminidase). Dev. Growth Differ 36, 133–139. doi: 10.1111/j.1440-169X.1994.00133.x
Leclerc C., Webb S. E., Daguzan C., Moreau M., Miller A. L. (2000). Imaging patterns of calcium transients during neural induction in Xenopus laevis embryos. J. Cell Sci. 113, 3519–3529. doi: 10.1242/jcs.113.19.3519
Levasseur M., Carroll M., Jones K. T., McDougall A. (2007). A novel mechanism controls the Ca2+ oscillations triggered by activation of ascidian eggs and has an absolute requirement for Cdk1 activity. J. Cell Sci. 120, 1763–1771. doi: 10.1242/jcs.003012
Levasseur M., Dumollard R., Chambon J. P., Hebras C., Sinclair M., Whitaker M., et al. (2013). Release from meiotic arrest in ascidian eggs requires the activity of two phosphatases but not CaMKII. Development 140, 4583–4593. doi: 10.1242/dev.096578
Levasseur M., McDougall A. (2000). Sperm-induced calcium oscillations at fertilisation in ascidians are controlled by cyclin B1-dependent kinase activity. Development 127, 631–641. doi: 10.1242/dev.127.3.631
Matsubara S., Shiraishi A., Osugi T., Kawada T., Satake H. (2019). The regulation of oocyte maturation and ovulation in the closest sister group of vertebrates. eLife 8, e49062. doi: 10.7554/eLife.49062.035
Matsuo M., Onuma T. A., Omotezako T., Nishida H. (2020). Protein phosphatase 2A is essential to maintain meiotic arrest, and to prevent Ca2+ burst at spawning and eventual parthenogenesis in the larvacean Oikopleura dioica. Dev. Biol. 460, 155–163. doi: 10.1016/j.ydbio.2019.12.005
McDougall A., Levasseur M. (1998). Sperm-triggered calcium oscillations during meiosis in ascidian oocytes first pause, restart, then stop: correlations with cell cycle kinase activity. Development 125, 4451–4459. doi: 10.1242/dev.125.22.4451
McDougall A., Sardet C. (1995). Function and characteristics of repetitive calcium waves associated with meiosis. Curr. Biol. 5, 318–328. doi: 10.1016/s0960-9822(95)00062-5
McDougall A., Chenevert J., Dumollard R. (2012). Cell-cycle control in oocytes and during early embryonic cleavage cycles in Ascidians. Int. Rev. Cell Mol. Biol. 297, 235–264. doi: 10.1016/B978-0-12-394308-8.00006-6
McDougall A., Sardet C., Lambert C. C. (1995). Different calcium-dependent pathways control fertilisation-triggered glycoside release and the cortical contraction in ascidian eggs is followed by regular bursts whose initiation relocates to a focal point within the ER. Zygote 3, 251–258. doi: 10.1017/S0967199400002641
Mikhaleva Y., Tolstenkov O., Glover J. C. (2019). Gap junction-dependent coordination of intercellular calcium signalling in the developing appendicularian tunicate Oikopleura dioica. Dev. Biol. 450, 9–22. doi: 10.1016/j.ydbio.2019.03.006
Moody W. J., Bosma M. M. (1989). A nonselective cation channel activated by membrane deformation in oocytes of the ascidian Boltenia villosa. J. Membr. Biol. 107, 179–188. doi: 10.1007/BF01871723
Moreau M., Leclerc C., Gualandris-Parisot L., Duprat A. M. (1994). Increased internal Ca2+ mediates neural induction in the amphibian embryo. PNAS 91, 12639–12643. doi: 10.1073/pnas.91.26.12639
Nakajo K., Chen L., Okamura Y. (1999). Cross-coupling between voltage-dependent Ca2+ channels and ryanodine receptors in developing ascidian muscle blastomeres. J. Physiol. 515, 695–710. doi: 10.1111/j.1469-7793.1999.695ab.x
Nixon V. L., McDougall A., Jones K. T. (2000). Ca 2+ oscillations and the cell cycle at fertilisation of mammalian and ascidian eggs. Biol. Cell 92, 187–196. doi: 10.1016/S0248-4900(00)01068-6
Nuccitelli R. (1987). The wave of activation current in the egg of the medaka fish. Dev. Biol. 122, 522–534. doi: 10.1016/0012-1606(87)90316-2
Nugues C., Helassa N., Haynes L. P. (2022). Mitosis, focus on calcium. Front. Physiol. 13, 951979. doi: 10.3389/fphys.2022.951979
Ohtsuka Y., Okamura Y. (2007). Voltage-dependent calcium influx mediates maturation of myofibril arrangement in ascidian larval muscle. Dev. Biol. 301, 361–373. doi: 10.1016/j.ydbio.2006.08.013
Okawa N., Shimai K., Ohnishi K., Ohkura M., Nakai J., Horie T., et al. (2020). Cellular identity and Ca(2+) signaling activity of the non-reproductive GnRH system in the Ciona intestinalis type A (Ciona robusta) larva. Sci Rep. 10, 18590. doi: 10.1038/s41598-020-75344-7
Onuma T. A., Hayashi M., Gyoja F., Kishi K., Wang K., Nishida H. (2020). A chordate species lacking Nodal utilizes calcium oscillation and Bmp for left–right patterning. PNAS 117, 4188–4198. doi: 10.1073/pnas.1916858117
Paredes R. M., Etzler J. C., Watts L. T., Zheng W., Lechleiter J. D. (2008). Chemical calcium indicators. Methods 46, 143–151. doi: 10.1016/j.ymeth.2008.09.025
Prudent J., Popgeorgiev N., Bonneau B., Thibaut J., Gadet R., Lopez J., et al. (2013). Bcl-wav and the mitochondrial calcium uniporter drive gastrula morphogenesis in zebrafish. Nat. Commun. 4, 2330. doi: 10.1038/ncomms3330
Ridgway E. B., Gilkey J. C., Jaffe L. F. (1977). Free calcium increases explosively in activating medaka eggs. PNAS 74, 623–627. doi: 10.1073/pnas.74.2.623
Runft L. L., Jaffe L. A. (2000). Sperm extract injection into ascidian eggs signals Ca(2+) release by the same pathway as fertilization. Development 127, 3227–3236. doi: 10.1242/dev.127.15.3227
Runft L. L., Jaffe L. A., Mehlmann L. M. (2002). Egg activation at fertilization: where it all begins. Dev. Biol. 245, 237–254. doi: 10.1006/dbio.2002.0600
Shah K. R., Guan X., Yan J. (2022). Diversity of two-pore channels and the accessory NAADP receptors in intracellular Ca(2+) signaling. Cell Calcium 104, 102594. doi: 10.1016/j.ceca.2022.102594
Shemesh O. A., Linghu C., Piatkevich K. D., Goodwin D., Celiker O. T., Gritton H. J., et al. (2020). Precision calcium imaging of dense neural populations via a cell-body-targeted calcium indicator. Neuron 107, 470–486.e11. doi: 10.1016/j.neuron.2020.05.029
Silvestre F., Cuomo A., Tosti E. (2009). Ion current activity and molecules modulating maturation and growth stages of ascidian (Ciona intestinalis) oocytes. Mol. Reprod. Dev. 76, 1084–1093. doi: 10.1002/mrd.v76:11
Simoncini L., Block M. L., Moody W. J. (1988). Lineage-specific development of calcium currents during embryogenesis. Science 242, 1572–1575. doi: 10.1126/science.2849207
Slusarski D. C., Pelegri F. (2007). Calcium signaling in vertebrate embryonic patterning and morphogenesis. Dev. Biol. 307, 1–13. doi: 10.1016/j.ydbio.2007.04.043
Smedley M. J., Stanisstreet M. (1985). Calcium and neurulation in mammalian embryos. J. Embryol. Exp. Morphol. 89, 1–14. doi: 10.1242/dev.89.1.1
Søviknes A. M., Chourrout D., Glover J. C. (2007). Development of the caudal nerve cord, motoneurons, and muscle innervation in the appendicularian urochordate Oikopleura dioica. J. Comp. Neurol. 503, 224–243. doi: 10.1002/cne.v503:2
Speksnijder J. E. (1992). The repetitive calcium waves in the fertilized ascidian egg are initiated near the vegetal pole by a cortical pacemaker. Dev. Biol. 153, 259–271. doi: 10.1016/0012-1606(92)90111-S
Speksnijder J. E., Corson D. W., Sardet C., Jaffe L. F. (1989). Free calcium pulses following fertilization in the ascidian egg. Dev. Biol. 135, 182–190. doi: 10.1016/0012-1606(89)90168-1
Speksnijder J. E., Sardet C., Jaffe L. F. (1990). Periodic calcium waves cross ascidian eggs after fertilization. Dev. Biol. 142, 246–249. doi: 10.1016/0012-1606(90)90168-I
Steinhardt R., Zucker R., Schatten G. (1977). Intracellular calcium release at fertilization in the sea urchin egg. Dev. Biol. 58, 185–196. doi: 10.1016/0012-1606(77)90084-7
Stricker S. A. (1999). Comparative biology of calcium signaling during fertilization and egg activation in animals. Dev. Biol. 211, 157–176. doi: 10.1006/dbio.1999.9340
Tolstenkov O., Mikhaleva Y., Glover J. C. (2022). Post-gastrulation transition from whole-body to tissue-specific intercellular calcium signaling in the appendicularian tunicate Oikopleura dioica. Dev. Biol. 492, 37–46. doi: 10.1016/j.ydbio.2022.09.004
Tosti E., Gallo A., Silvestre F. (2011). Ion currents involved in oocyte maturation, fertilization and early developmental stages of the ascidian Ciona intestinalis. Mol. Reprod. Dev. 78, 854–860. doi: 10.1002/mrd.v78.10/11
Tsuruwaka Y., Shimada E., Tsutsui K., Ogawa T. (2017). Ca2+ dynamics in zebrafish morphogenesis. PeerJ 5, e2894. doi: 10.7717/peerj.2894
Wallingford J. B., Ewald A. J., Harland R. M., Fraser S. E. (2001). Calcium signaling during convergent extension in Xenopus. Curr. Biol. 11, 652–661. doi: 10.1016/S0960-9822(01)00201-9
Webb S. E., Cheung C. C., Chan C. M., Love D. R., Miller A. L. (2012). Application of complementary luminescent and fluorescent imaging techniques to visualize nuclear and cytoplasmic Ca2+ signalling during the in vivo differentiation of slow muscle cells in zebrafish embryos under normal and dystrophic conditions. Clin. Exp. Pharmacol. Physiol. 39, 78–86. doi: 10.1111/j.1440-1681.2011.05582.x
Webb S. E., Miller A. L. (2011). Visualization of Ca²+ signaling during embryonic skeletal muscle formation in vertebrates. Cold Spring Harb. Perspect. Biol. 3, a004325. doi: 10.1101/cshperspect.a004325
Webb S. E., Miller A. L. (2003). Calcium signalling during embryonic development. Nat. Rev. Mol. Cell Biol. 4, 539–551. doi: 10.1038/nrm1149
Webb S. E., Moreau M., Leclerc C., Miller A. L. (2005). Calcium transients and neural induction in vertebrates. Cell Calcium 37, 375–385. doi: 10.1016/j.ceca.2005.01.005
Whitaker M. (2006). Calcium at fertilization and in early development. Physiol. Rev. 86, 25–88. doi: 10.1152/physrev.00023.2005
Whitaker M. (2008). Calcium signaling in early embryos. Phil. Trans. R. Soc B 363, 1401–1418. doi: 10.1098/rstb.2008.2259
Wilding M., Marino M., Monfrecola V., Dale B. (2000). Meiosis-associated calcium waves in ascidian oocytes are correlated with the position of the male centrosome. Zygote 8, 285–293. doi: 10.1017/S0967199400001088
Keywords: oocyte, blastula, gastrula, neurulation, Oikopleura, Ciona, ascidian, appendicularian
Citation: Glover JC, Tolstenkov O and Mikhaleva Y (2024) Calcium signaling in tunicate development. Front. Ecol. Evol. 12:1470671. doi: 10.3389/fevo.2024.1470671
Received: 25 July 2024; Accepted: 12 November 2024;
Published: 03 December 2024.
Edited by:
Lucia Manni, University of Padua, ItalyReviewed by:
Nathalie Oulhen, Brown University, United StatesUgo Coppola, Florida Gulf Coast University, United States
Copyright © 2024 Glover, Tolstenkov and Mikhaleva. This is an open-access article distributed under the terms of the Creative Commons Attribution License (CC BY). The use, distribution or reproduction in other forums is permitted, provided the original author(s) and the copyright owner(s) are credited and that the original publication in this journal is cited, in accordance with accepted academic practice. No use, distribution or reproduction is permitted which does not comply with these terms.
*Correspondence: Joel C. Glover, am9lbC5nbG92ZXJAbWVkaXNpbi51aW8ubm8=