- 1Department of Natural Resource Ecology and Management, Iowa State University, Ames, IA, United States
- 2Department of Integrative Biology, Oklahoma State University, Stillwater, OK, United States
Introduction: Multifarious selective pressures can interact to affect species’ life history evolution, with predation and thermal exposure as selective pressures for nesting birds. Gray Vireos (Vireo vicinior) seemingly nest on the periphery of their nesting substrate because of lower predation rates, thereby increasing exposure to weather. We explored how nest placement and vegetation structure can be used to account for the increased weather exposure that Gray Vireos experience when nesting on the periphery of the nesting substrate to avoid predation.
Methods: For each Gray Vireo nest, we placed temperature and light data loggers at three locations: at the nest site, at the opposite orientation of the nest within the nesting tree, and at the same orientation of the nest but in an adjacent tree. To measure nest orientation, we recorded the inverse compass azimuth (+/−1°) from the nest toward the trunk of the nesting tree, while accounting for declination. Nest temperatures and light exposure were compared across various dimensions of nest placement.
Results: The orientation of nests was cooler than the opposite orientation in the mornings and in the late afternoons. When nests were placed in hotter orientations (e.g., south- or west-facing), nests surrounded by more foliage or placed closer to the interior of trees could compensate for the increased exposure.
Discussion: Our findings suggest Gray Vireos accounted for the increased thermal exposure that comes from predator avoidance by using secondary dimensions of nest placement. Specifically, nests could be placed in orientations with cooler temperatures or in hotter orientations with greater shade potential. These results highlight how the interactive selection pressures of predation risk and microclimate can be tiered and shape life-history characteristics of birds.
1 Introduction
For birds, breeding life-histories and nesting ecology demonstrate unique ecological interactions and important insights needed for conservation and habitat management (Martin, 1995). The importance of natural selection as a dynamic force that controls nest-site selection and structure is well documented (Martin, 1993, Martin, 1995; Mainwaring et al., 2014). Selective forces, such as nest predation (Martin, 1993), brood parasitism (Moreras et al., 2021), and microclimate (Amat-Valero et al., 2014) continually shape nest-site selection at multiple scales. However, studies that consider the interactions of selective forces and their relative contributions are rare. Additionally, nest placement within a substrate and nest morphology may provide a unique framework for understanding multifarious selection because they manifest in spatial dimensions. Nest placement operates at three spatial dimensions within the nesting substrate (i.e., nesting tree or shrub), being nest height from the ground (Wilson and Cooper, 1998), distance from the foliar edge (Harris et al., 2021a), and orientation (Burton, 2006), being the cardinal direction of nests relative to the center of the nesting substrate. Nest morphology can vary in at least two additional spatial dimensions within a nesting substrate, being nest height and circumference. These five spatial dimensions are likely continually being optimized by dynamic, multifarious selective forces to increase nest survival, thereby shaping fine-scale nest placement and structure.
Nest predation is generally the primary selective pressure on nest placement and structure (Martin, 1988, Martin, 1992, Martin, 1993), resulting in species accounting for all other ecological pressures secondarily. Microclimate at nests may be an important secondary selective pressure, particularly for birds in arid environments (Marzluff, 1988). The influence of microclimate at nest sites has been shown to affect nest-site selection, nest placement within a substrate, and nest morphology (Amat-Valero et al., 2014; Mainwaring et al., 2014; Perez et al., 2020). Nest sites may be selected for cooler microclimates around nests (Hartman and Oring, 2003; Tieleman et al., 2008; Carroll et al., 2015). Cooler microclimates in arid environments have also been linked to increased survival probabilities (Carroll et al., 2015; Grisham et al., 2016) and normal rates of embryo development (Webb, 1987). Consequently, species-specific nesting strategies tend to minimize thermal variances and lower maximum temperatures in arid environments (Hartman and Oring, 2003; Tieleman et al., 2008; Carroll et al., 2015) via increased visual obstruction for shade (Carroll et al., 2015) or selecting an orientation of cavity openings to increase shade (Hartman and Oring, 2003; Ardia et al., 2006). The thermal environment is also thought to be one of the most important selective forces to nest morphology and composition (Perez et al., 2020). The construction of domed nests, for example, likely plays an important role in microclimate stability by minimizing direct exposure to sunlight (Griffith et al., 2016). However, the significance of the thermal environment as a driver of nest placement and morphology may be more important in environments with thermal extremes.
Species in the Vireonidae family tend to nest on the periphery of nesting substrates (Bent, 1965). This is common in species such as Bell’s Vireo (Vireo belli) (Kus et al., 2020), Plumbeous Vireo (Vireo plumbeus) (Goguen and Curson, 2020), Black-capped Vireo (Vireo atricapilla) (Grzybowski, 2020), and Gray Vireo (Vireo vicinior) (Harris et al., 2020). This strategy may be a mechanism to avoid high rates of predation by snakes and mammals. For example, Gray Vireos in New Mexico have higher predation and brood parasitism rates in the interior of nesting trees compared to nests on the exterior, particularly when trees have high foliage density (Harris et al., 2021a). Although Harris et al. (2021a) did not identify predators at nests, they hypothesized that interior predation pressure from mammals and snakes is greater than the exterior predation and parasitism pressure from corvids and Brown-headed Cowbirds (Molothrus ater), respectively. Consequently, this primary selection pressure likely forces Gray Vireo nests towards the exterior of trees (Harris et al., 2021a), which may increase thermal exposure.
Gray Vireos are short-distance migrants that predominantly breed in the southwestern United States (Barlow et al., 2020) from May–August. Breeding habitat is generally in pinyon pine (Pinus edulis) and juniper (Juniperus spp.) woodlands or in juniper savannas (Schlossberg, 2006; Harris et al., 2020; Harris et al., 2021b). In pinyon-juniper woodlands, Gray Vireos will almost exclusively nest in junipers (Harris et al., 2020) and nests are commonly located on the periphery of junipers (Barlow et al., 2020; Harris et al., 2020). Orientation of nests relative to the center of the nesting tree varies. Hargrove and Unitt (2017) found that Gray Vireo nests in California tended to be located on the south side of nesting shrubs, however nests in other regions can be north or west facing (Barlow et al., 2020).
A subset of these data were previously used to demonstrate that Gray Vireo nest survival is higher at the periphery of the nesting substrate, with predation as the primary cause of nest failure (Harris et al., 2021a). Therefore, we explored how variation in nest placement and morphology could account for the increased thermal exposure that is caused by nesting in these areas. We tested multiple hypotheses: i) Gray Vireos would choose nest orientations that are cooler (possibly due to light exposure or foliage density) relative to the opposite orientation of the nesting tree, ii) nests would have lower variance in temperature throughout the day than the opposite orientation, iii) when nests occurred on the south- and west-facing side of trees, they would utilize vegetation structure and nest placement to compensate for higher temperatures, and iv) smaller nests would be cooler given that they are more easily concealed and shaded. Additionally, we measured how light exposure varied by nest orientation and vegetation structure as a method to understand the mechanism and timing of nest warming.
2 Methods
2.1 Study site
We monitored Gray Vireo nests on Kirtland Air Force Base (KAFB) in 2016–2020. KAFB is located south of Albuquerque, New Mexico, and consists of approximately 108 ha of pinyon-juniper woodlands and juniper savannah (U.S. Air Force 2012). The area is immediately west of the Manzanita mountains, with elevation ranging from 1600–2400 m (US Air Force, 2012). During June 1–August 1 of 2016–2020, the mean daily precipitation total was 0.64 mm (range: 0–26.9 mm) (New Mexico Climate Center, 2019). The mean high and low daily temperatures from June 1–August 1 in 2016–2020 were 31.4°C (± 4.5°C SD) and 15.8°C (± 4.5°C SD), respectively (New Mexico Climate Center, 2019). There was a minimum ambient temperature of 2.8°C and a maximum ambient temperature of 40.9°C (New Mexico Climate Center, 2019) (https://weather.nmsu.edu).
2.2 Nests placement and morphology
We located Gray Vireo territories using call-back surveys at 50 random points in pinyon-juniper woodlands and juniper savannahs (US Air Force, 2012). For detailed methods on Gray Vireo nest searching, see Harris et al. (2020). Briefly, we surveyed Gray Vireos using methods from Kubel and Yahner (2007). When Gray Vireos were observed at a point, we recorded a distance and direction to determine the approximate location of the territory. Once a breeding territory had been identified, we searched for nests using behavioral cues, such as carrying nesting material, males singing from nests, or following females. Nests were monitored once a week until completion (i.e., fledged, depredated, parasitized, or abandoned).
At each nest, we measured the nest orientation relative to the center of the nesting tree, the distance of the nest from the edge of the nesting substrate (hereafter: distance to edge), nest height, and foliage density. To measure nest orientation, we recorded the inverse compass azimuth (+/− 1°) from the nest toward the trunk of the nesting tree, while accounting for declination. Foliage density was measured using a modified Braun-Blanquet method (Wikum and Shanholtzer, 1978; Harris et al., 2021a), where each nesting tree was categorized by the percentage of woody limbs obstructed by foliage: 1 (0–25%), 2 (26–50%), 3 (51–75%), or 4 (76–100%). For each nesting tree, we estimated a foliage density score at the four primary cardinal directions, which was then averaged as a single foliage density score.
We collected a subset of nests to measure nest morphological characteristics. Nests were clipped from the terminal fork of their branch to preserve nest dimensions during removal from nesting trees. We used digital calipers (+/− 0.2 mm accuracy, Fisher Scientific) and flexible rulers (+/− 1 mm accuracy, Avinet) to measure outer and inner diameter, depth, height, and wall thickness of nests. We recorded two orthogonal measurements of outer and inner diameter for each nest, along with four, equally distanced measurements of wall thickness. These measurements were then averaged for each nest prior to analysis.
2.3 Microclimate monitoring
Within one week after a nest had completed, we recorded air temperature (°C) (TN) and light exposure (lm1) (LN) at the nest site, at the opposite orientation of the nest (TO, LO) within the nesting tree, and at the same orientation of the nest but in an adjacent tree to account for variation in vegetation structure (TS, LS) (Figure 1). TN and LN were measured with a temperature/light data logger (HOBO pendant, Onset Computer Corporation, Bourne, MA) within the nest, yet situated so that the light sensor was above the rim of the nest. For destroyed nests, data loggers were hung in the tree at the location of the nest prior to destruction. TO and LO were measured by placing a data logger at the opposite orientation of the nest within the nesting tree, yet at the same relative location as the nest (i.e., same nest height and distance from the edge of the tree). Similarly, data loggers used to measure TS and LS were placed in the nearest adjacent tree of the same species, with the same orientation, nest height, and distance from the edge of the tree (Figure 1). For example, if a nest was located on the east side of a tree (90°), we placed data loggers at the nest site, at the west side of the nesting tree (270°), and at the east side of an adjacent tree (90°). This sampling method allowed us to test the relative importance of nest orientation and vegetation structure on nest microclimate. Data were recorded every 30 minutes from 0700–1900 hr. (approximate hours of sunlight) for five consecutive days. Five days were chosen arbitrarily but allowed us to avoid single-day weather extremes in analysis. Data were averaged for each time interval across the five days, as to have a single temperature and light exposure value for each time interval at each nest.
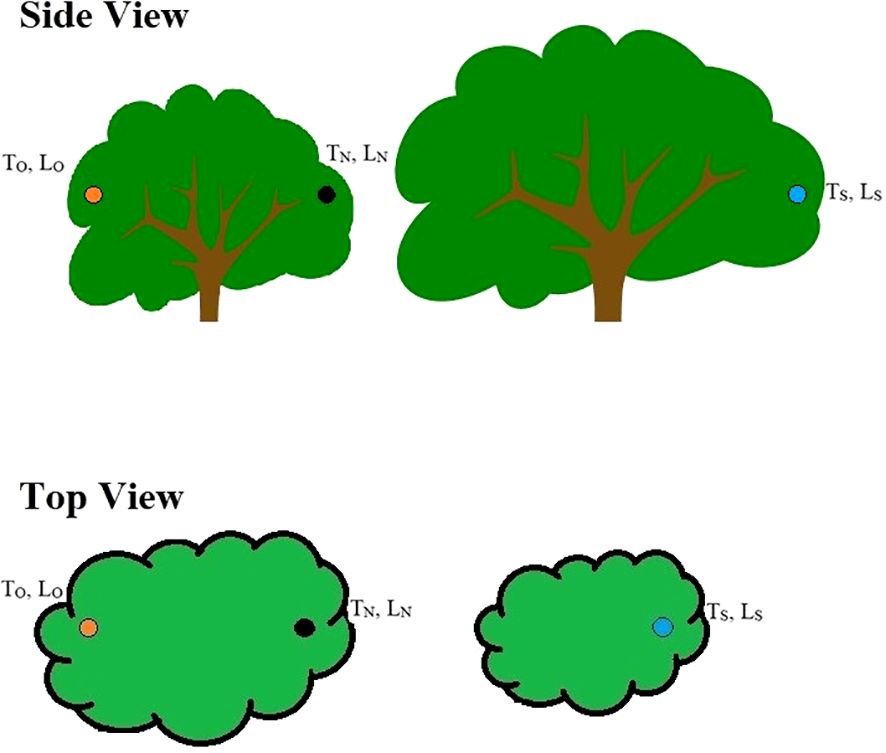
Figure 1. An illustration of the experimental design with the three sample locations. The black dot represents the location of a Gray Vireo nest (location of TN and LN samples). The orange dot represents the opposite orientation of the Gray Vireo nest but at the same relative position (TO, LO). And the blue dot represents the same orientation of the Gray Vireo nest in an adjacent tree to compare differences in vegetation structure (TS, LS).
2.4 Statistical analyses
We used multiple models to test our various hypotheses (Appendix A); however, most models had the general model structure of temperature and light exposure as dependent variables and “Nest ID” as a random effect using linear mixed effect models (LME). Models were created via the “nlme” package in Rstudio (2019, v. 1.2.1; Pinheiro et al., 2020; R Core Team, 2016). We used the “lme” function to assign an autocorrelation structure to our model, to account for temporal autocorrelation among 30-minute temperature/light readings. We used a first-order autoregressive process as an autocorrelation structure, with “Time of Day ‘‘ as time variable.
To test our hypothesis that Gray Vireos use nest orientation to minimize thermal exposure, we used “Sample Location” (Nest, Opposite Orientation, or Adjacent Tree), “Time of Day”, and “Year” as independent variables, which included an interaction between “Sample Placement’’ and “Time of Day” to account for changing effects of sample locations during hotter or cooler times of the day. Similarly, we used the same model structure but included distance to edge, nest height, and foliage density as additive independent variables to test the hypothesis that Gray Vireos would use vegetation structure and plasticity in nest placement to compensate for orientations with higher temperatures. As a post-hoc analysis, we used the “emmeans” package (Lenth et al., 2020) to calculate estimated marginal means (or least squares means) with a Bonferroni correction and a Tukey-adjusted pairwise comparison.
To test the hypothesis that nest orientation may be selected to decrease the thermal variance throughout the day, we compared mean hourly change in temperature between TN, TO and TS using a LME with “Nest ID” as a random effect and “Sample” as a fixed effect. We used an alpha value of 0.05 as a threshold for statistically significant differences for all analyses, and 0.05 < P < 0.10 indicate weak evidence of an effect (Muff et al., 2022).
Lastly, we constructed a global model with all nest morphology parameters as independent variables to test the hypothesis that smaller nests would be cooler than larger nests. We dredged the global model to identify nest morphology parameters correlated with maximum nest temperatures. If a parameter was included in a model with a delta Akaike Information Criterion (AIC) < 2.0, we determined if the 95% confidence interval of the estimate overlapped with zero. Models with a parameter that overlapped with zero were not considered competitive. If nest morphology parameters were correlated with cooler nest temperatures, then we included them in a two-way ANOVA with nest orientation to determine if morphology of nests could be used to compensate orientations with higher temperatures.
3 Results
3.1 Nest temperatures and rate of change
Gray Vireos initiated nesting on KAFB as early as 24 April (2019) and as late as 03 August (2017). We monitored the temperature of 94 nests, with subsets of 59 and 48 nests for light exposure and nest morphology, respectively. The subset for light exposure monitoring was due to a limited number of data loggers with light detection capabilities, while the subset for nest morphology was based on the number of nests that were undamaged and safely retrievable. The distribution of nest orientations was similar among the eight cardinal directions, with the most common orientations being east–northeast and south–southeast (n=15) and the least being west-southwest (n=4) (Table 1). Orientation data were missing for six nests (Table 1). We recorded nest temperatures ranging from 4.6°C–46.3°C. Nests began cooling at approximately 1600 hr., compared to TO, which began cooling at approximately 1730 hr. (Figure 2). Additionally, the rate of cooling for TN was faster than for TO in the afternoon (Figure 2). From 1600–1800 hr., TN decreased at a rate of 3.1°C/hr, while TO decreased at a rate of 1.5°C/hr. We also found weak evidence that the hourly change in temperature at nests were lower than the opposite orientation (P = 0.08).
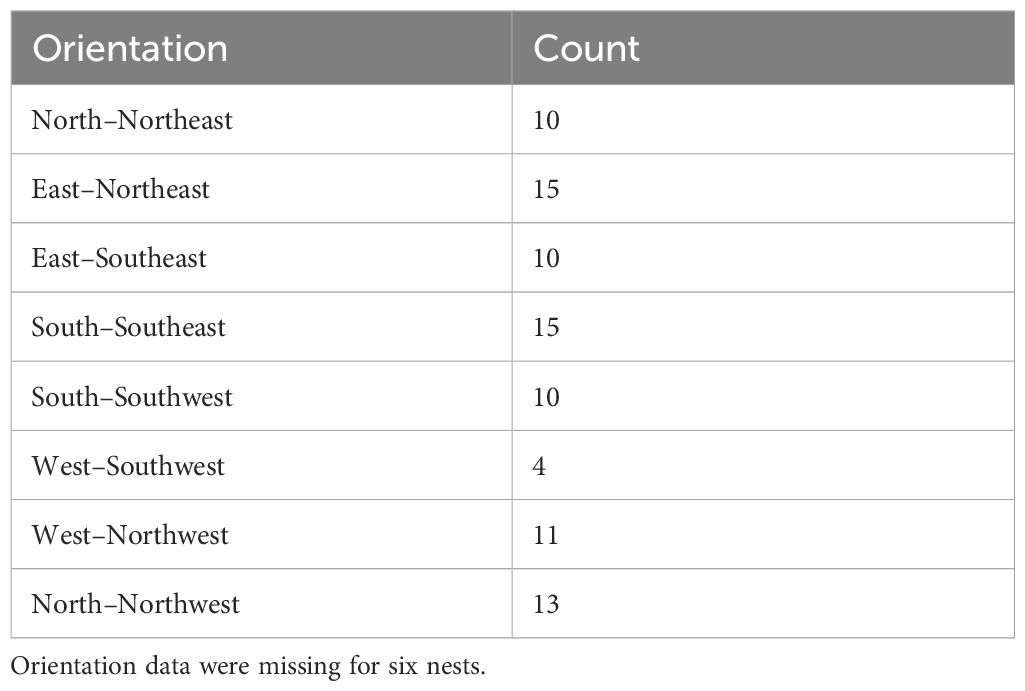
Table 1. Distribution of 88 Gray Vireo nest orientations found on Kirtland Air Force Base in Albuquerque, NM in 2016–2020.
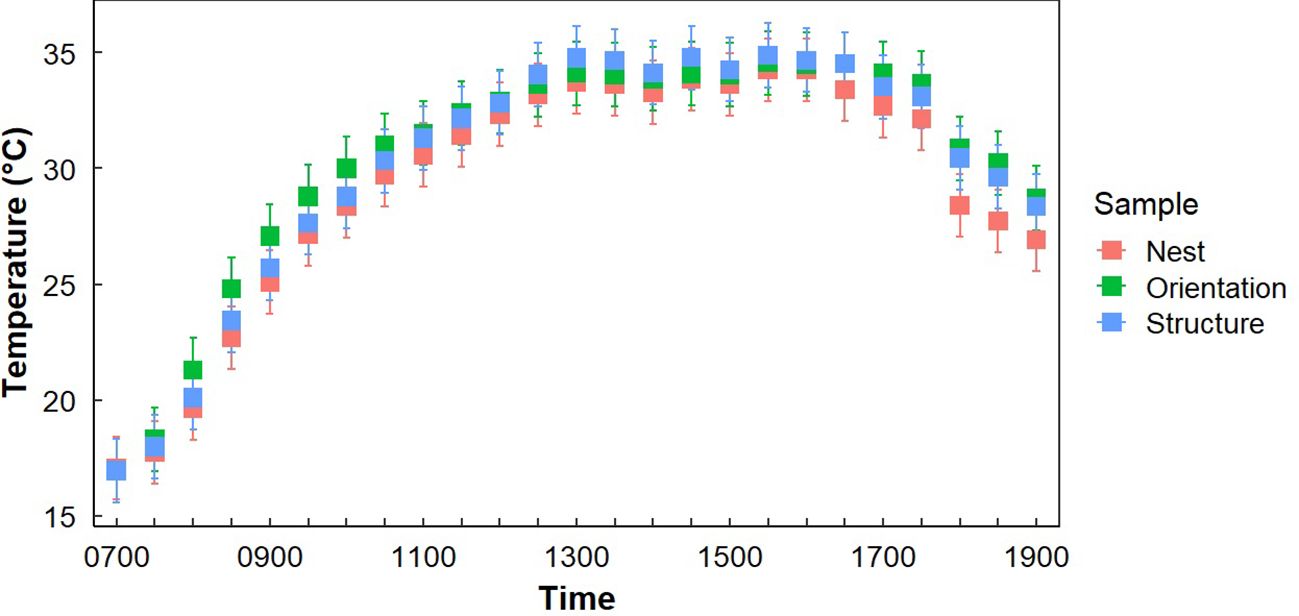
Figure 2. Average temperatures at Gray Vireo nest sites (TN), the opposite orientation (TO), and in different vegetation structures (TS) with 95% confidence intervals. Significant differences between TN and TO occurred between 1700–1830 hr.
3.2 Nest orientation
Both “Sample Placement” (nest, opposite orientation, or adjacent tree) and “Time of Day” significantly influenced differences in average temperature (P <0.001), but there was not a significant interaction of “Sample Placement” and “Time of Day” (P = 0.977) (Table 2). While average TN was less than average TO and TS at all times of the day (except at 0700 hr.), significant differences only occurred in the morning (0800–1030 hr.) and in the late afternoon (1700–1900 hr.) (Appendix B; Figure 2), with most of these differences occurring between TN and TO. During these times TN was on average 2.0°C (± 0.3°C) cooler than TO (Appendix B; Figure 2). TN was only significantly cooler than TS at 1800–1830 hr. (Appendix B). Differences in TN at different orientations were minimal. West-facing nests were significantly cooler than other orientations from 0730–1000 hr. South-facing nests were significantly warmer than north-facing nests from 0900–0930 hr. (Figure 3). Generally, north and east-facing nests had the most variability in temperature throughout the day, while south-facing nests tended to have lower variation (Figure 3). However, we found little evidence that any one orientation created an optimal microclimate (i.e., less thermal variation and/or fewer temperature extremes).
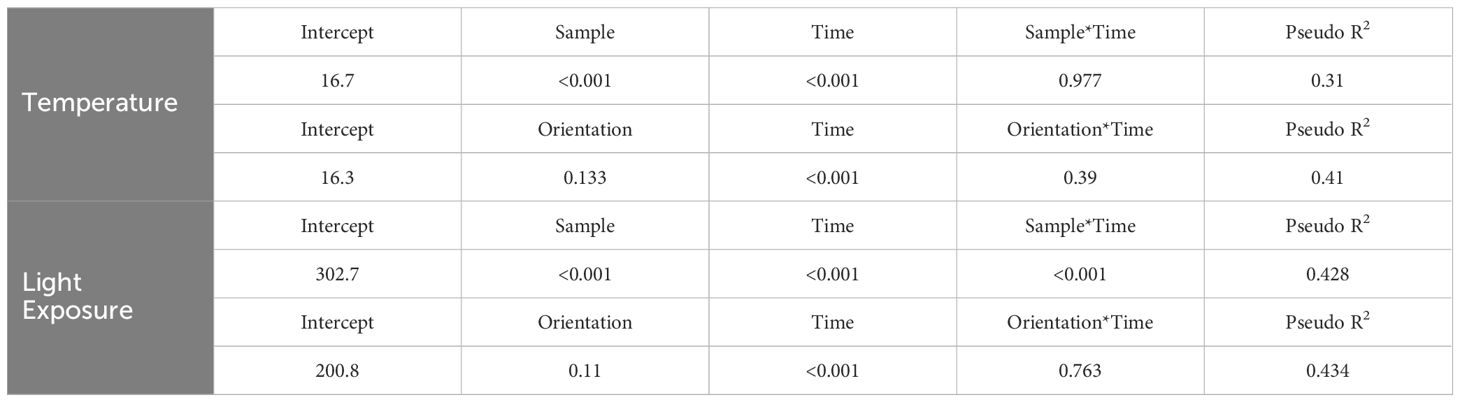
Table 2. Results from linear mixed-effect models testing differences in temperature and light exposure as functions of sample (i.e., nest, opposite orientation, different vegetation structure), nest orientation, and time.
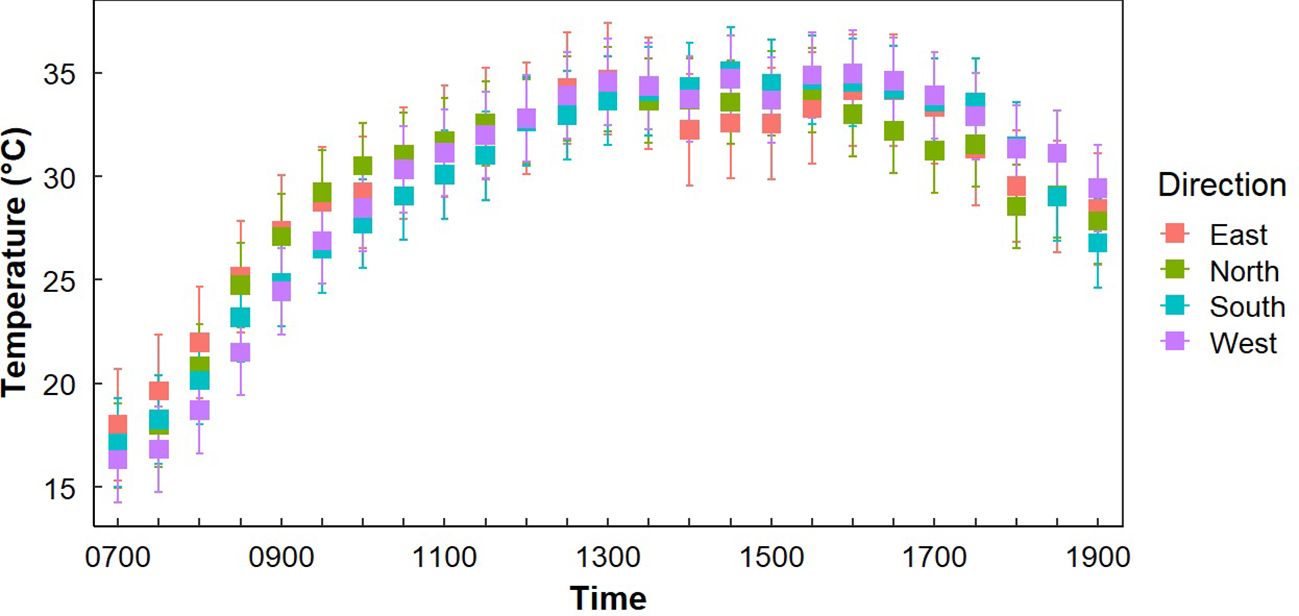
Figure 3. Average temperatures at Gray Vireo nest sites (n=71) in each cardinal direction with 95% confidence intervals. South-facing nests were significantly warmer than north-facing nests from 1700–1800 hr. West-facing nests were significantly cooler than other orientations from 1730–1000 hr.
Light exposure was different between “Sample Placement” at various times (Table 2; Appendix C). On average, nests received less light throughout the day than LO and LS, where the average LN was 6,569 lm (± 4,153 lm) less than the average LO and 9,780 lm (± 9,624 lm) less than LS (Appendix C; Figure 4). The degree of difference changed by time (P = <0.001). Differences between LN and LS occurred between 1000–1200 hr. and 1300–1400 hr. (Appendix C). Similarly, differences between LN and LO occurred between 0930–1030 hr. and then sporadically in the afternoon (Appendix C; Figure 4). Variation in LN was less throughout the day (σ = 35,439 lm) than LO (σ = 39,413 lm) and LS (σ = 40,702 lm). Additionally, variance of light exposure within time periods was also less for LN than LO and LS (Figure 4). We did not find a link between nest orientation and light exposure with time of day (Figure 5).
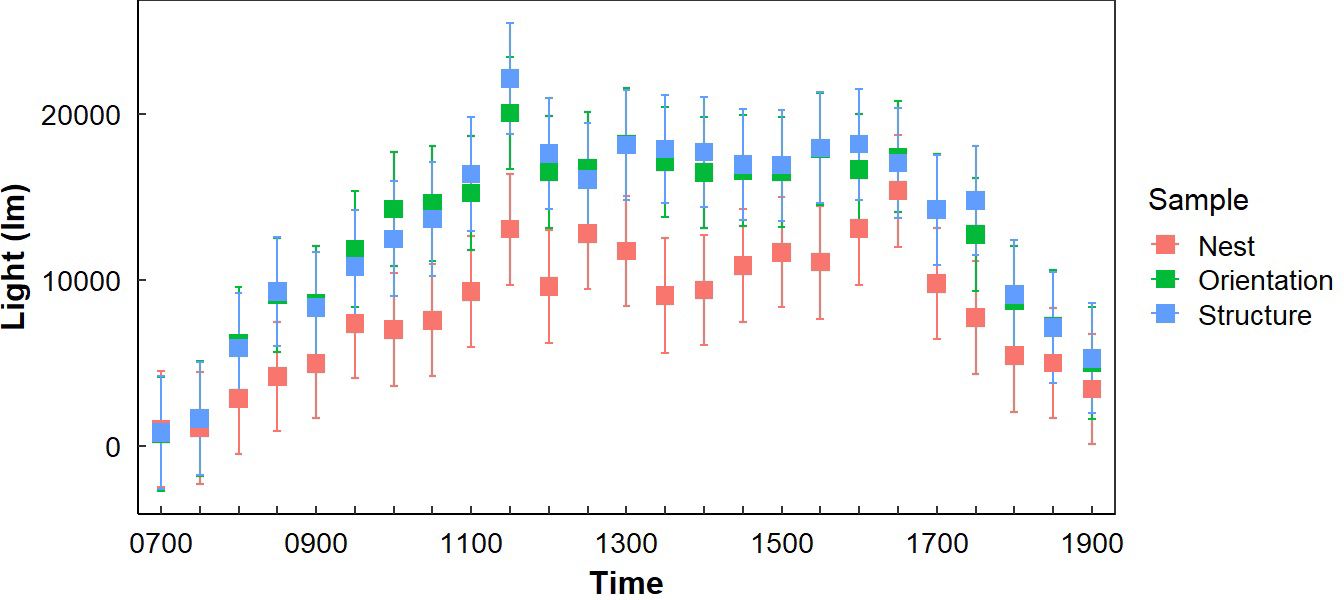
Figure 4. Average light exposure at Gray Vireo nest sites (LN), the opposite orientation (LO), and in different vegetation structures (LS) with 95% confidence intervals. Significant differences were generally greatest between LN and LS, with greatest differences occurring between 1000–1130 hr.
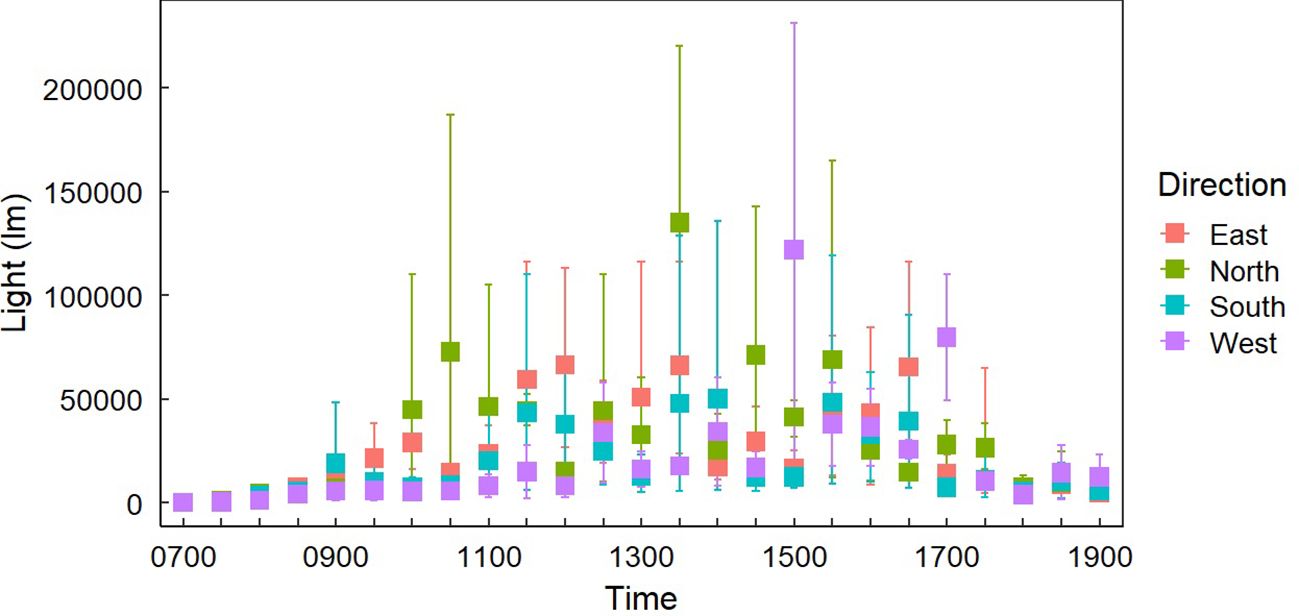
Figure 5. Average light exposure at Gray Vireo nest sites (n=37) in each cardinal direction with 95% confidence intervals. There were no clear differences in light exposure between orientations except for western orientations receiving more light at 1500 and 1700 hrs than other orientations.
3.3 Foliage density and distance to edge
We found that nest-tree foliage density and plasticity in nest placement can compensate for orientations with higher temperatures. Nests located on the south-facing side of nesting trees were hotter than other orientations if nesting trees had low foliage density (P = <0.001) (Figure 6) or if nests were located closer to the edge of the nesting tree (Figure 7). South-facing nests were statistically cooler when nesting on the interior of nesting trees at 1230 hr. (P = 0.05) and 1800 hr. (P = 0.02), with statistical trends occurring sporadically throughout the afternoon. Nests on the south-facing orientations were on average farther from the edge than other orientations (Figure 8); however, this relationship was not statistically significant (P = 0.212). Similarly, nests with the highest foliage density scores (i.e., 75–100%) were most common on the south and west-facing sides of trees (Figure 9), but there was no statistical difference in nest proportions across the four foliage density scores and orientations (P = 0.54).
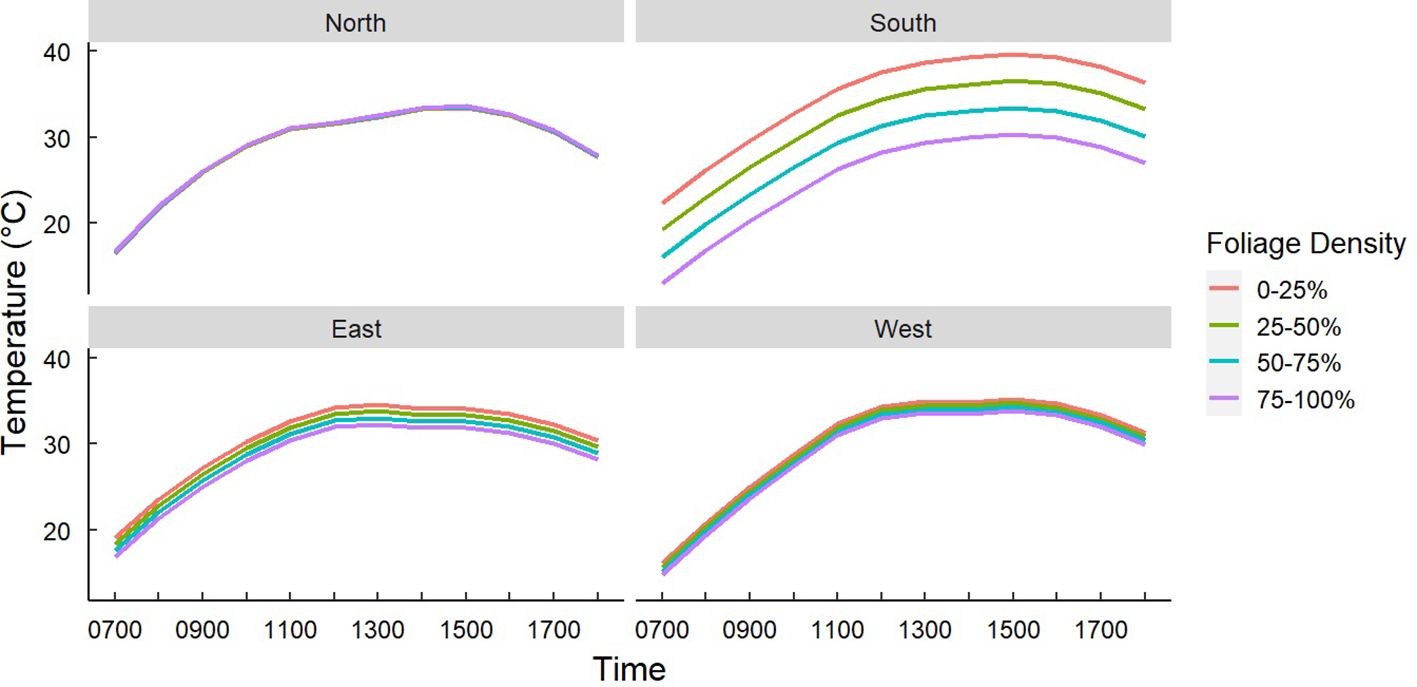
Figure 6. Predicted temperatures of Gray Vireo nests based on an interaction of nest orientation and nest-tree foliage density. Foliage density was categorized by the percentage of wood limbs obstructed by foliage. South-facing nests were significantly cooler if they were in nesting trees with higher foliage density. Foliage density did not affect the temperature of nests in other orientations.
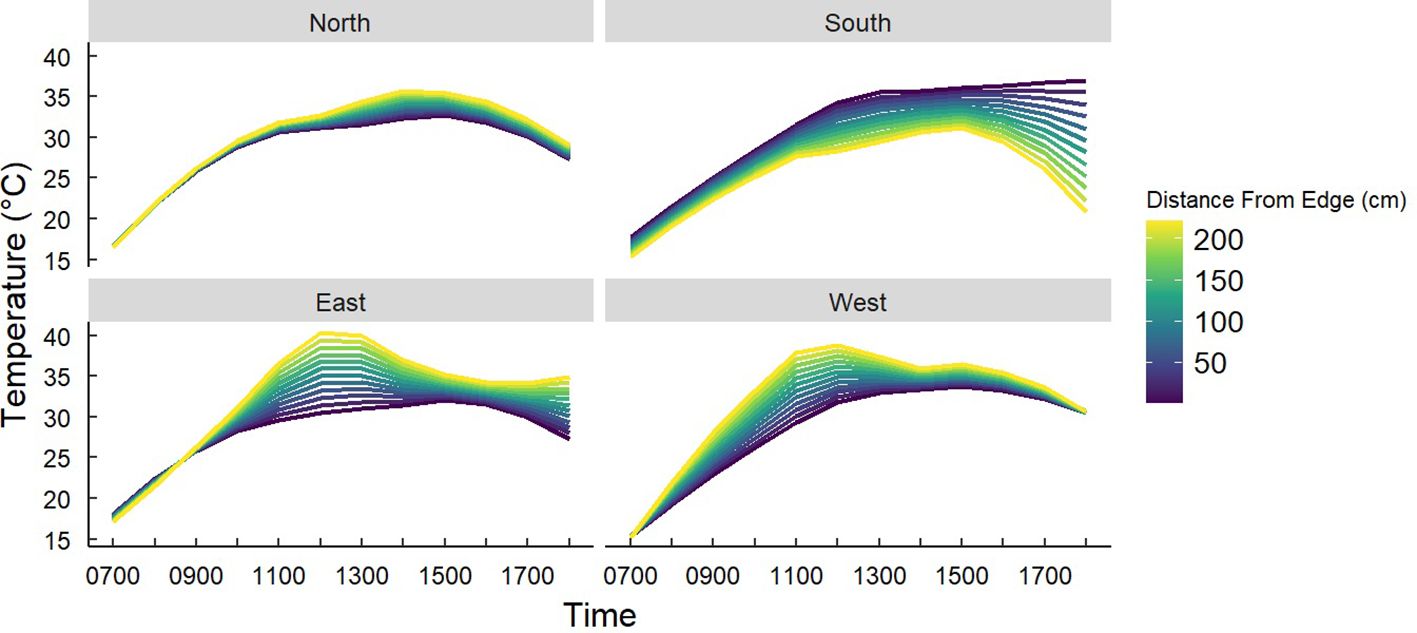
Figure 7. Predicted temperatures of Gray Vireo nests based on an interaction of nest orientation and the distance of nests from the edge of the nesting tree. South-facing nests were significantly cooler if they nested closer to the interior of the nesting tree at 1730–1800 hr.
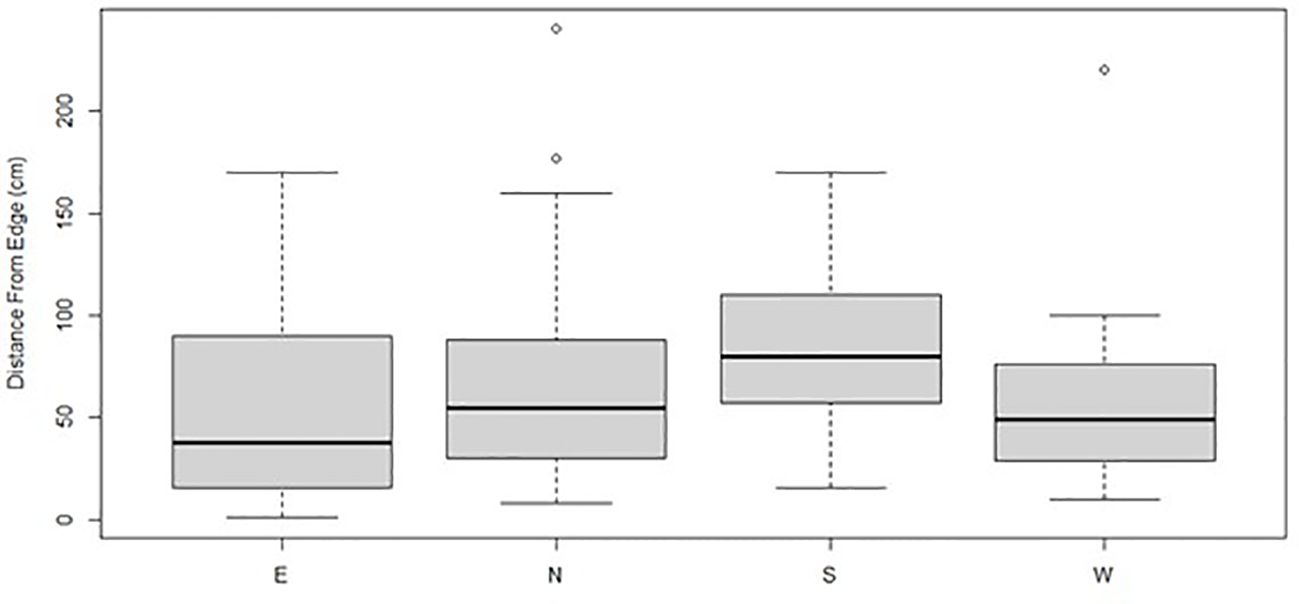
Figure 8. Boxplots represent the mean and quartile distances of Gray Vireo nests from the edge of the nesting tree for different nest orientations. On average, south-facing nests were closer to the interior of the nesting tree than other orientations; however, this relationship was not statistically significant.
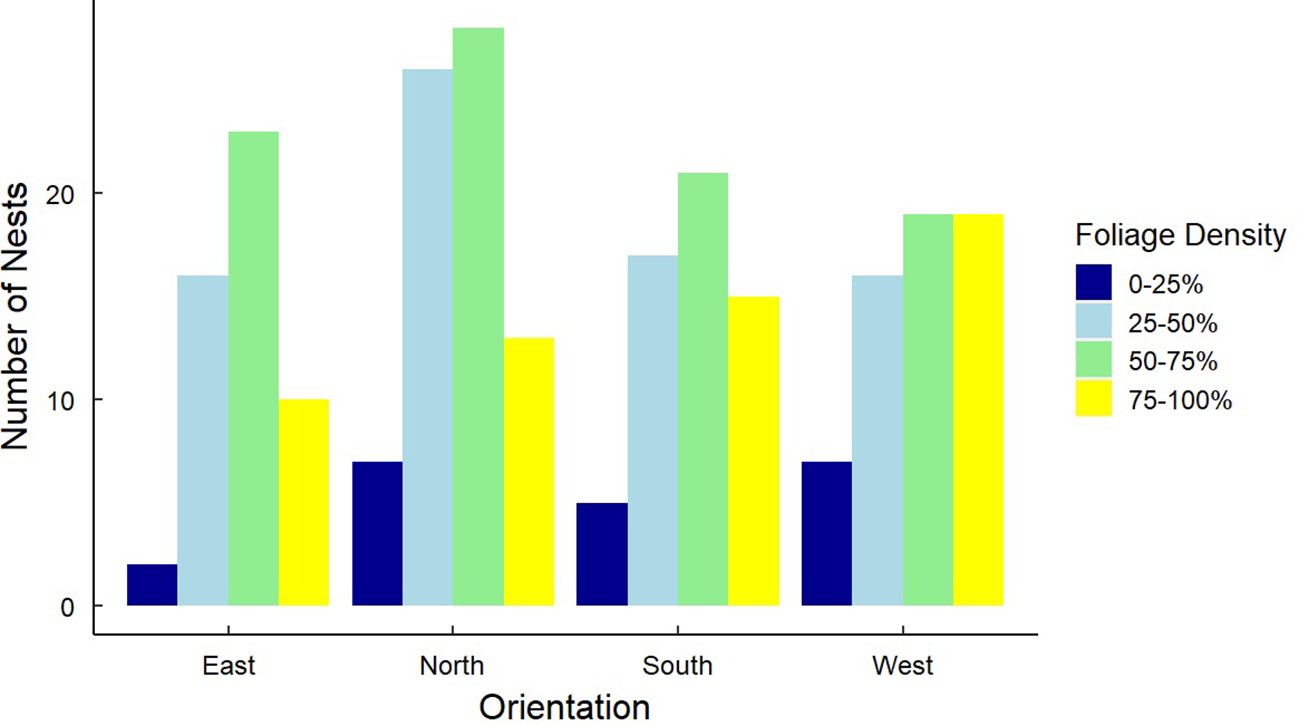
Figure 9. Frequency distributions for 246 Gray Vireo nest orientations and the foliage density of nesting trees. There was no relationship between nest orientation and nest-tree foliage density.
3.4 Nest morphology
The top model testing nest morphology characteristics on maximum nest temperature was the null model, suggesting that there was no relationship between nest morphology and microclimate.
4 Discussion
Evolutionarily, Gray Vireo nests have been pushed to the periphery of nesting trees (Bent, 1965; Barlow et al., 2020), seemingly to reduce nest predation (Harris et al., 2021a). In New Mexico, nests on the periphery of the nesting tree had high survival probabilities when nest-tree foliage density was high (Harris et al., 2021a), and nests were located in areas where junipers had higher foliage density than what was available at random (Harris et al., 2020). In these areas, nesting at the periphery of nesting trees is predicted to decrease predation risk and therefore, pose a selective advantage. We sought to understand how Gray Vireos compensate for the increased thermal exposure that comes from nesting on the periphery of trees. We found that Gray Vireos may use a combination of nest orientation, nest placement, and nest tree characteristics to reduce nest temperatures and thermal variance throughout the day. We did not find evidence that Gray Vireos use variation in nest morphology to minimize thermal stress.
Although Gray Vireos selected all nest orientations similarly, we found that the orientation of nests was cooler on average than the opposite orientation of the nesting tree, with the greatest difference occurring from 1700–1830 hr. In Albuquerque during the summer months (May–August), the highest ambient temperature occurs between 1600–2000 hr. (https://weatherspark.com/y/3318/Average-weather-in-Albuquerque-New-Mexico-United-States-Year-Round). These findings suggest that the nests had a cooler microclimate during the hottest times of the day than the opposite orientation of the nesting tree, with an average temperature difference of 2°C. This pattern was strongest for north-, east-, and west-facing nests, while south-facing nests were slightly warmer than the north side of nesting trees (Appendix D). Nests also had a smaller range of temperatures throughout the day and began cooling in the evenings faster than the opposite orientation.
The use of nest orientation to optimize microclimate has been shown in a variety of species. Some cavity nesting species, such as Tree Swallow (Tachycineta bicolor), use the orientation of nest openings to maximize wind exposure (Ricklefs and Hainsworth, 1969) and to increase solar exposure during colder mornings (Ardia et al., 2006). Conversely, Horned Larks (Eremophila alpestris) have been shown to nest disproportionately on the north-facing side of their substrate to increase the amount of shade the nest experiences throughout the day (Hartman and Oring, 2003). Some studies have found that Gray Vireos more commonly nest on the south side of the nesting tree (Hargrove and Unitt, 2017; Barlow et al., 2020), however we did not see this trend. Gray Vireos did not show a preference to any one orientation, yet still selected the cooler side of nesting trees. This suggests that Gray Vireos may be able to select the best nest orientation for any given nesting tree. Nest sites were commonly situated in valleys with slopes immediately to the north, east, or south. Such topographic variation could result in shade effects that vary light exposure by time of day. Additionally, unlike the Hargrove and Unitt (2017) study, our nests were in wooded areas, where nesting trees were likely to receive shade from taller adjacent vegetation. These factors may lead to inconsistency in light exposure and temperature by orientation, resulting in Gray Vireos using all nesting orientations.
Although differences in light exposure between LN and LO were observed, almost all differences occurred in morning, when there were no temperature differences. The biggest differences in light exposure were between LN and LS, which suggests that Gray Vireos utilize vegetation structure (more so than orientation) to increase shade and minimize variance in light exposure at nests. Seemingly, relative differences observed in light exposure did not translate into differences in temperatures. Part of this may be accounted for by latency effects, where locations exposed to more light in the mornings maintain greater heat throughout the day. However, this may also be due to differences between ambient and operative temperatures. We measured ambient temperature instead of operative temperature because we were more interested in relative comparisons of thermal conditions than modeling thermal stress. Operative temperature directly accounts for solar radiation and convective heat transfer (Campbell and Norman, 1998). Another explanation for why light exposure did not translate into differences in temperature is that variation between light readings was high, particularly in LO and LS. Light exposure was measured instantaneously every 30 minutes, which could result in high variation between readings. Our study site frequently had high winds and tall, adjacent vegetation which may have resulted in inconsistent shade when collecting instantaneous samples.
When nests were in hotter, south-facing orientations, plasticity in nest placement and nest-tree characteristics could be used to counter greater thermal exposure. If nests were located closer to the interior of nesting trees or if nesting trees had greater foliage density, then nest temperatures were similar or cooler than other orientations. Harris et al. (2020) found that Gray Vireos select nesting areas where the foliage density of junipers was higher than what was randomly available. Although foliage density contributed to cooler microclimates in hotter orientations, we did not find evidence that Gray Vireos disproportionately selected high foliage density when nesting on south- and west-facing orientations. Similarly, south-facing nests were on average closer to the interior of nesting trees than other orientations, although this result was not statistically significant. It may be difficult to statistically detect differences in “distance to edge” because variation in that variable is limited by predation risk, as well. In other words, when nesting on the south side of trees, Gray Vireos seemingly move slightly closer to the interior but are limited in how far they can go by higher predation risk in the interior of nesting trees (Harris et al., 2021a). This example may illustrate why it is difficult to detect negatively interacting selective forces, particularly when one selective force is more heavily weighted than another. As predation risk forces nests outward (Harris et al., 2021a) and microclimate forces nests inward, the total variation in nest placement may be minimal from neutralizing selective forces.
Predation of nests has been shown to be the most influential selective pressure on nest-site selection for certain species (Martin, 1988, Martin, 1992, Martin, 1993). Predation risk can act on various scales (Schmidt et al., 2006), which can affect nest-site selection at multiple scales. At the finest scale, nest placement within a substrate can seek to increase visual obstruction and minimize predation risk (Harris et al., 2021a). Consequently, nest predation is considered the primary selective pressure on the evolution of nest placement and structure (Ibáñez-Álamo et al., 2015).This is likely also true for Gray Vireos, given that predation is the most common cause of nest failure (Hargrove and Unitt, 2017; Harris et al., 2021a). Therefore, Gray Vireos likely must consider microclimate secondarily after minimizing predation risk. This may be an example of multifarious or tiered (e.g., primary, secondary) selective forces, where predation risk pushes nests outward toward the exterior of nesting trees, and thermal extremes as a secondary selective force, push nests inwards, particularly for south-facing nests. Microclimate is likely a weaker selective force because the thermal extremes in our study would not likely induce direct mortality of embryos (Webb, 1987). However, Gray Vireo nests did experience temperatures that could affect other aspects of reproductive success and embryonic development. Grenõ et al. (2008) found a negative correlation between nest temperatures and fledgling survival probabilities. Additionally, several studies have shown that nest temperatures greater than 34°C can negatively affect nestling physiology for a variety of songbirds (Ardia, 2013; Cunningham et al., 2013; Rodriguez and Barba, 2016). Specifically, nestlings can experience higher hematocrit levels (Ardia, 2013), stunted growth (Rodriguez and Barba, 2016), and delayed fledging (Cunningham et al., 2013). The threshold of 34°C was reached by 89 of our Gray Vireo nests (95%) and 93 of the opposite orientation samples (99%). Temperatures we measured could have even greater adverse effects on nestling physiology, considering we measured ambient temperature as opposed to operative temperature, which may be higher when accounting for relative humidity (Yahav et al., 1995) and solar radiation (Dzialowski, 2005).
Examples of multifarious selection are rare (Egea-Serrano et al., 2014) and we have not found an example where selective forces are considered tiered. However, selective forces are known to be dynamic (Rodewald et al., 2011), multidimensional (Orsini et al., 2012), and interactive (Egea-Serrano et al., 2014). It is probable that these multidimensional forces are weighted, with weights changing based on dynamic environmental conditions (Rauter et al., 2002). For example, as climates warm, nest microclimate may become the primary selective pressure shaping nest placement, particularly in warmer parts of the species’ ranges. The evolutionary origins of nest placement behavior provide an ideal framework for studying multifarious, tiered, or weighted selective pressures, as these forces manifest in three-dimensional space within a nesting substrate. However, more data are needed to understand the frequency of weather-induced nest failure and if frequencies change through time.
Data availability statement
The raw data supporting the conclusions of this article will be made available by the authors, without undue reservation.
Ethics statement
The animal study was approved by Oklahoma State University Institutional Animal Care and Use Committee. The study was conducted in accordance with the local legislation and institutional requirements.
Author contributions
JH: Conceptualization, Data curation, Formal analysis, Investigation, Methodology, Visualization, Writing – original draft, Writing – review & editing. SM: Conceptualization, Funding acquisition, Investigation, Resources, Supervision, Writing – review & editing.
Funding
The author(s) declare financial support was received for the research, authorship, and/or publication of this article. This work was supported by the U.S. Department of Defense (grant number W9126G-15-2-0044).
Acknowledgments
We thank S Young, J Haughawout, J Kitting, A West, and K Asmus for their help with data collection. We also thank DH Reynolds, AR Cuevas, MB Clark, and the Civil Engineering Division of Kirtland Air Force Base for their administrative and logistic support. We appreciate feedback from S Loss and C Davis on a previous draft of this manuscript.
Conflict of interest
The authors declare that the research was conducted in the absence of any commercial or financial relationships that could be construed as a potential conflict of interest.
Publisher’s note
All claims expressed in this article are solely those of the authors and do not necessarily represent those of their affiliated organizations, or those of the publisher, the editors and the reviewers. Any product that may be evaluated in this article, or claim that may be made by its manufacturer, is not guaranteed or endorsed by the publisher.
Supplementary material
The Supplementary Material for this article can be found online at: https://www.frontiersin.org/articles/10.3389/fevo.2024.1417573/full#supplementary-material
Footnotes
- ^ Lumens (lm) is the unit for luminous flux, which is a measure for visible light according to the International System of Units (SI).
References
Amat-Valero M., Calero-Torralbo M. A., Václav R., Valera F. (2014). Cavity types and microclimate: implications for the ecological, evolutionary, and conservation studies. Int. J. Biometeorology 58, 1983–1994. doi: 10.1007/s00484-014-0801-0
Ardia D. R., Perez J. H., Clotfelter E. D. (2006). Nest box orientation affects internal temperature and nest site selection by Tree Swallows. J. Field Ornithology 77, 339–344. doi: 10.1111/j.1557-9263.2006.00064.x
Ardia D. R. (2013). The effects of nestbox thermal environment on fledging success and haematocrit in tree swallows. Avian Biol. Res. 6, 99–103.
Barlow J. C., Leckie S. N., Baril C. T. (2020). Gray vireo (Vireo vicinior), version 1.0. In Birds of the World. Eds. Poole A. F., Gill F. B. (Ithaca, NY, USA: Cornell Lab of Ornithology). doi: 10.2173/bow.gryvir.01
Bent A. C. (1965). Life histories of North American wagtails, shrikes, vireos, and their allies (Washington D.C., USA: Smithsonian Institution).
Burton N. H. K. (2006). Nest orientation and hatching success in the tree pipit Anthus trivialis. J. Avian Biol. 37, 312–317. doi: 10.1111/j.2006.0908-8857.03822.x
Campbell G. S., Norman J. M. (1998). An introduction to environmental biophysics. 2nd ed. (New York, New York, USA: Springer Science & Business Media).
Carroll J. M., Davis C. A., Elmore R. D., Fuhlendorf S. D. (2015). A ground-nesting Galliform’s response to thermal heterogeneity: implications for ground-dwelling birds. PLoS One 10, e0143676. doi: 10.1371/journal.pone.0143676
Cunningham S. J., Martin R. O., Hojem C. L., Hockey P. A. R. (2013). Temperatures in excess of critical thresholds threaten nestling growth and survival in a rapidly-warming arid savanna: a study of common fiscals. PLoS One 8, e74613. doi: 10.1371/journal.pone.0074613
Dzialowski E. M. (2005). Use of operative temperature and standard operative temperature models in thermal biology. J. Thermal Biol. 30, 317–334. doi: 10.1016/j.jtherbio.2005.01.005
Egea-Serrano A., Hangartner S., Laurila A., Räsänen K. (2014). Multifarious selection through environmental change: acidity and predator-mediate adaptive divergence in the moor frog (Rana arvalis). Proc. R. Soc. B 281, 20133266. doi: 10.1098/rspb.2013.3266
Goguen B., Curson D. R. (2020). Plumbeous vireo (Vireo plumbeus), version 1.0. Ed. Poole A. F. (Ithaca, NY, USA: Cornell Lab of Ornithology). Birds of the World. doi: 10.2173/bow.plsvir.01
Grenõ J. L., Belda E. J., Barba E. (2008). Influence of temperatures during the nestling period on post-fledging survival of great tit Parus major in a Mediterranean habitat. J. Avian Biol. 39, 41–49. doi: 10.1111/j.0908-8857.2008.04120.x
Griffith S. C., Wainwaring M. C., Sorato E., Beckman C. (2016). High atmospheric temperatures and “ambient temperature“ drive embryonic development and lead to earlier hatching in a passerine bird. R. Soc. Open Scient 3, 150371. doi: 10.1098/rsos.150371
Grisham B. A., Godar A. J., Boal C. W., Haukos D. A. (2016). Interactive effects between nest microclimate and nest vegetation structure confirm microclimate thresholds for lesser prairie-chicken nest survival. Condor 118, 728–746. doi: 10.1650/CONDOR-16-38.1
Grzybowski J. A. (2020). Black-capped vireo (Vireo atricapilla), version 1.0. Eds. Poole A. F., Gill F. B. (Ithaca, NY, USA: Cornell Lab of Ornithology). Birds of the World. doi: 10.2173/bow.bkcvir1.01
Hargrove L., Unitt P. (2017). Poor reproductive success of Gray Vireos in a declining California population. J. Field Ornithology 88, 16–19. doi: 10.1111/jofo.2017.88.issue-1
Harris J. P., Smith L. M., McMurry S. T. (2020). A multiscale analysis of gray vireo (Vireo vicinior) nest-site selection in central New Mexico. Avian Conserv. Ecol. 15, 12. doi: 10.5751/ACE-01540-150112
Harris J. P., Smith L. M., McMurry S. T. (2021a). Fine-scale plasticity in nest placement can compensate for poor quality junipers as nesting trees for gray vireos. PeerJ 9, e12477. doi: 10.7717/peerj.12477
Harris J. P., Smith L. M., McMurry S. T. (2021b). Interacting gradients of selection and survival probabilities to estimate habitat quality: an example using the gray vireo (Vireo vicinior). Ecol. Indic. 131, 108210. doi: 10.1016/j.ecolind.2021.108210
Hartman C. A., Oring L. W. (2003). Orientation and microclimate of Horned Lark nests: the importance of shade. Condor 105, 158–163. doi: 10.1093/condor/105.1.158
Ibáñez-Álamo J. D., Magrath R. D., Oteyza J. C., Chalfoun A. D., Haff T. M., Schmidt K. A., et al. (2015). Nest predation research: recent findings and future perspectives. J. Ornithology 156, 246–262. doi: 10.1007/s10336-015-1207-4
Kubel J. E., Yahner R. H. (2007). Detection probability of Golden-winged Warblers during point counts with and without playback recordings. J. Field Ornithology 78, 195–205. doi: 10.1111/j.1557-9263.2006.00094.x
Kus B., Hopp S. L., Johnson R. R., Brown B. T. (2020). Bell's vireo (Vireo bellii), version 1.0. Ed. Poole A.F.P. (Ithaca, NY, USA: Cornell Lab of Ornithology). Birds of the World. doi: 10.2173/bow.belvir.01
Lenth R., Singmann H., Love J., Buerkner P., Herve M. (2020). “emmeans: estimated marginal means,” in R package version 1.0.23.1 (R Foundation for Statistical Computing, Vienna, Austria). Available at: https://CRAN.R-project.org/package=bbmle.
Mainwaring M. C., Hartley I. R., Lanmbrechts M. M., Deeming D. C. (2014). The design and function of birds’ nests. Ecol. Evol. 20, 3909–3928. doi: 10.1002/ece3.1054
Martin T. E. (1988). Processes organizing open-nesting bird assemblages: competition or nest predation? Evolutionary Ecol. 2, 37–50. doi: 10.1007/BF02071587
Martin T. E. (1992). Interaction of nest predation and food limitation in reproductive strategies. Curr. Ornithology 9, 163–197.
Martin T. E. (1993). Nest predation and nest sites: new perspectives on old patterns. Bioscience 43, 523–532. doi: 10.2307/1311947
Martin T. E. (1995). Avian life history evolution in relation to nest sites, nest predation, and food. Ecol. Monogr. 65, 101–127. doi: 10.2307/2937160
Marzluff J. M. (1988). Do pinyon jays alter nest placement based on prior experience? Anim. Behav. 36, 1–10. doi: 10.1016/S0003-3472(88)80244-6
Moreras A., Tolvanen J., Morosinotto C., Bussiere E., Forsman J., Thomson R. L. (2021). Choice of nest attributes as a frontline defense against brood parasitism. Behav. Ecol. 32, 1285–1295.
Muff S., Nilsen E. B., O’Hara R. B., Nater C. R. (2022). Rewriting results sections in the language of evidence. Trends Ecol. Evol. 37, 203–210. doi: 10.1016/j.tree.2021.10.009
New Mexico Climate Center (2019). Albuquerque International Airport weather station. Available online at: https://weather.nmsu.edu/coop/request/station/290234/data/. (Accessed September 1, 2019)
Orsini L., Spanier K. I., Meester L. D. (2012). Genomic signature of natural and anthropogenic stress in wild populations of the weaterflea Daphnia magna: validation in space, time and experimental evolution. Mol. Ecol. 21, 2160–2175. doi: 10.1111/j.1365-294X.2011.05429.x
Perez D. M., Gardner J. L., Medina I. (2020). Climate as an evolutionary driver of nest morphology in birds: a review. Front. Ecol. Evol. 8, 424. doi: 10.3389/fevo.2020.566018
Pinheiro J., Bates D., DebRoy S., Sarkar D., R Core Team (2020). nlme: linear and nonlinear mixed effects models. (R package version 3), 1–148. Available at: https://CRAN.R-project.org/package=nlme.
Rauter C. M., Reyer H. U., Bollmann K. (2002). Selection through predation, snowfall and microclimate on nest-site preferences in the Water Pipit Anthus spinoletta. Ibis 144, 433–444. doi: 10.1046/j.1474-919X.2002.00013.x
R Core Team (2016). R: A language and environment for statistical computing. (Vienna, Austria: R Foundation for Statistical Computing). Available at: http://www.R-project.org.
Ricklefs R. E., Hainsworth F. R. (1969). Temperature regulation in nestling cactus wrens: the nest environment. Condor 71, 32–37. doi: 10.2307/1366045
Rodewald A. D., Shustack D. P., Jones T. M. (2011). Dynamic selective environments and evolutionary traps in human-dominated landscapes. Ecology 92, 1781–1788. doi: 10.1890/11-0022.1
Rodriguez S., Barba E. (2016). Nestling growth is impaired by heat stress: an experimental study in a Mediterranean great tit population. Zoological Stud. 55, 40. doi: 10.6620/ZS.2016.55-40
Schlossberg S. (2006). Abundances and habitat preferences of gray vireos (Vireo vicinior) on the Colorado plateau. Auk 123, 33–44. doi: 10.1093/auk/123.1.33
Schmidt K. A., Ostfeld R. S., Smyth K. N. (2006). Spatial heterogeneity in predator activity, nest survivorship, and nest-site selection in two forest thrushes. Oecologia 148, 22–29. doi: 10.1007/s00442-005-0340-9
Tieleman B. I., Van Noordwijk H. J., Williams J. B. (2008). Nest site selection in a hot desert: trade-off between microclimate and predation risk. Condor 110, 116–124. doi: 10.1525/cond.2008.110.1.116
US Air Force (2012). Integrated natural resources management plan for Kirtland Air Force Base (Kirtland AFB, New Mexico, USA: Department of Defense).
Webb D. R. (1987). Thermal tolerance of avian embryos: a review. Condor 89, 874–898. doi: 10.2307/1368537
Wilson R. W., Cooper R. J. (1998). Acadian flycatcher nest placement: does placement influence reproductive success? Condor 100, 673–679. doi: 10.2307/1369748
Wikum D. A., Shanholtzer G. F. (1978). Application of the Braun-Blanquet cover-abundance scale for vegetation analysis in land development studies. Environ. Manag. 2, 323–329.
Keywords: Gray Vireo, microclimate, multifarious selection, natural selection, nest-site selection, tiered selective forces, weighted selection
Citation: Harris JP and McMurry ST (2024) Nest placement accounts for thermal exposure secondarily: insights on multifarious selection. Front. Ecol. Evol. 12:1417573. doi: 10.3389/fevo.2024.1417573
Received: 15 April 2024; Accepted: 19 August 2024;
Published: 13 September 2024.
Edited by:
Mark A. Elgar, The University of Melbourne, AustraliaReviewed by:
Gustavo Liñan, Spanish National Research Council (CSIC), SpainAlejandro Corregidor-Castro, University of Padua, Italy
Copyright © 2024 Harris and McMurry. This is an open-access article distributed under the terms of the Creative Commons Attribution License (CC BY). The use, distribution or reproduction in other forums is permitted, provided the original author(s) and the copyright owner(s) are credited and that the original publication in this journal is cited, in accordance with accepted academic practice. No use, distribution or reproduction is permitted which does not comply with these terms.
*Correspondence: Jonathan P. Harris, anBoYXJyQGlhc3RhdGUuZWR1