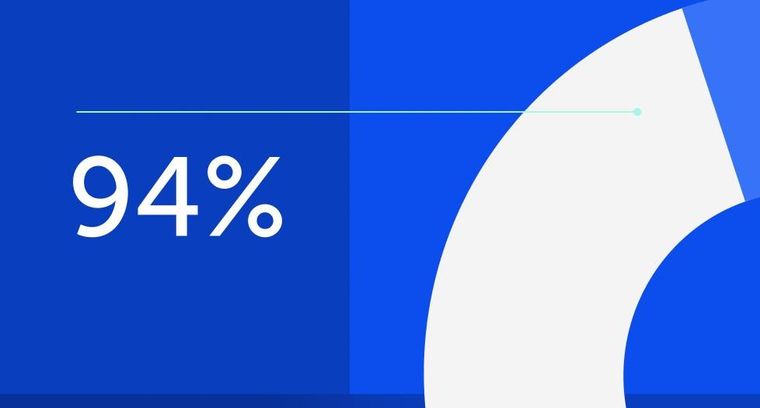
94% of researchers rate our articles as excellent or good
Learn more about the work of our research integrity team to safeguard the quality of each article we publish.
Find out more
ORIGINAL RESEARCH article
Front. Ecol. Evol., 11 July 2024
Sec. Biogeography and Macroecology
Volume 12 - 2024 | https://doi.org/10.3389/fevo.2024.1393376
This article is part of the Research TopicBiodiversity of Antarctic and Subantarctic EcosystemsView all 10 articles
The terrestrial fauna of Antarctica consists of a limited number of species, notably insects, small crustaceans and other micro-invertebrates. Over long periods of evolutionary isolation, these organisms have developed varying degrees of tolerance to multifaceted environmental stresses. Recent molecular biogeographical research highlights the enduring persistence of much of Antarctica’s current terrestrial fauna, with estimates spanning from hundreds of thousands to millions of years. Parochlus steinenii, commonly known as the Antarctic winged midge, stands out as one of the only two insect species native to Antarctica. Distributed across three biogeographic regions, southern South America and the Falkland/Malvinas Islands, sub-Antarctic South Georgia and the Maritime Antarctic South Shetland Islands, this midge raises questions about the temporal isolation of its populations and their divergence. Employing mitochondrial and nuclear genetic markers, we conducted phylogeographic and demographic analyses on 151 individuals of P. steinenii obtained across the three main biogeographic regions including the Magellanic sub-Antarctic Ecoregion (MSE) of southern South America, the sub-Antarctic Island of South Georgia (SG) and the South Shetland Islands (SSI) within the Maritime Antarctic (MA). Our data support the diversification of P. steinenii during the mid-Pleistocene around 1.46 Mya. This period included a branching event between a clade containing only specimens from the MSE and a clade containing individuals from a broader range of locations including the SSI and SG. Based on intraspecific phylogeographic and demographic inferences, we detected strong evolutionary divergence between the three main biogeographic regions. We also detected a signal of population growth during the deglaciation process in SSI and SG, contrary to the pattern seen in the MSE. The different demographic and phylogeographic histories between the sampled biogeographic regions could result from the MA and SG experiencing a strong genetic bottleneck due to a reduction in population size during the Last Glacial Maximum, while the MSE maintained a significant effective population size. The high level of divergence detected between individuals from the MSE and the remaining biogeographic regions supports the hypothesis of a speciation process taking place in P. steinenii.
Today’s Antarctic terrestrial fauna is largely restricted to ice-free areas of the continent, which contribute only ~0.3% of the total continental area, increasing to ~3% in the milder Antarctic Peninsula region. The fauna of the Maritime Antarctic, including the Antarctic Peninsula and Scotia Arc archipelagos, as well as the sub-Antarctic region, exhibits a low diversity characterized by isolated populations. It primarily consists of insects and small crustaceans representing the arthropods, along with tardigrades, rotifers, nematodes, and protozoans (Chown and Convey, 2016; Convey and Biersma, 2024). Over the course of millions of years, this distinct group of organisms has developed and diversified in response to a wide range of environmental stresses. Recent phylogeographic studies strongly suggests that many species within Antarctica’s terrestrial biota have persisted for long periods, with estimated persistence ranging from hundreds of thousands to multi-million year timescales (Convey et al., 2008, 2020; Verleyen et al., 2021; Maturana et al., 2022). Conversely, a smaller number of studies have reported evidence supporting more recent mid- to post-Pleistocene colonization from lower latitudes (van de Wouw et al., 2008; Biersma et al., 2020). Contemporary terrestrial biodiversity in Antarctica therefore consists of a mixture of species that have survived the repeated glacial maxima in local refugia and then recolonized subsequently deglaciated areas, or have arrived more recently through inter-and post-glacial dispersal from lower latitude areas that remained ice-free, or are present through a combination of both mechanisms (Maturana et al., 2022).
The terrestrial fauna of the Antarctic region is closely related to South America, with intimate geographical, biological, geological and glaciological histories (Mercer, 1976; Clapperton and Sugden, 1988; Clapperton, 1990; Rodbell et al., 2009) as evidenced by the presence of shared non-biting midges (Diptera: Chironomidae), and crustaceans (Copepoda and Anostraca). Even though much still remains to be resolved in clarifying the relationships of sub-Antarctic and Antarctic taxa to their sister-groups in South America, the available evidence allows the development of hypotheses and predictions relating to the temporal scale of species divergence and subsequent colonization of the Antarctic region, such as the long-term presence of Antarctic biota and persistence of populations in ice-free areas (Convey et al., 2008), or the dispersal patterns in Belgica antarctica Jacobs, 1900, the endemic wingless Antarctic midge (Allegrucci et al., 2012).
Although there has been significant scientific effort on certain taxonomic groups, understanding in this area remains limited. This lack of knowledge makes it challenging to comprehensively analyze evolutionary patterns in the region. One such poorly studied taxon is Parochlus steinenii (Gercke, 1889) (Diptera: Chironomidae), commonly known as the Antarctic winged midge and one of only two insect species native to the Antarctic continent, along with B. antartica. Parochlus steinenii occurs naturally in southern South America, the Falkland/Malvinas Islands, sub-Antarctic South Georgia, and the South Shetland Islands in Maritime Antarctica (Gañán et al., 2021). Brundin (1966) redescribed the species from adults and pupae collected in Tierra del Fuego, along with the first description of Parochlus steinenii brevipennis Brundin, 1966, a sub-species found in the Andes of Central Chile, south of the Argentinian city of Bariloche (34°S, 893 m.a.s.l.) (Brundin, 1966). Various aspects of the species’ biology have been studied, including occurrences, morphology, phenology and physiology (Brundin, 1966; Edwards and Usher, 1985; Rauschert, 1985; Shimada et al., 1991; Convey et al., 1996; Gañán et al., 2021; Pertierra et al., 2021; Contador et al., 2023) but its genetic diversity, phylogeographic structure and historical demographic processes have yet to be assessed, other than in the very preliminary data presented by Allegrucci et al. (2006).
Despite being capable of flight, P. steinenii may face challenges in dispersing across larger distances due to unfavorable abiotic conditions such as strong winds and cold temperatures. Its different life stages are found in both terrestrial and aquatic environments (Hahn and Reinhardt, 2006; Contador et al., 2023). The larvae and pupae are aquatic, inhabiting deeper permanent lakes where they can avoid entrapment in ice during winter, while the short-lived adults are terrestrial and are found in high abundance and density (approximately 600-800 individuals/m2) during the Antarctic summer at the edge of lakes and streams, where copulation and oviposition occur (Contador et al., 2023). Some freshwater ecosystems in these regions may be adversely affected by predicted future climatic changes, particularly if these lead to local drying or loss of water sources, which could impact the persistence of P. steinenii in its current distribution. In this context, the Antarctic winged midge may act as an effective sentinel of climate change across past, present and future scenarios in Antarctic and sub-Antarctic terrestrial and freshwater ecosystems.
The wide distribution of P. steinenii, and its apparently highly conserved morphology, raise questions about temporal isolation and potential speciation among regional populations. We hypothesize that molecular phylogeographic and demographic analyses using two genetic markers may reveal cryptic divergence and the timescales of persistence of Maritime and sub-Antarctic populations. Our results are important for understanding the long-term evolution of Antarctic terrestrial species and contribute to the debate surrounding evolutionary patterns in the sub- and Maritime Antarctic biota.
Specimens of P. steinenii were collected from freshwater bodies within three recognized biogeographic regions (Figure 1, for more detail see Supplementary Table S1): 1) Magellanic sub-Antarctic Ecoregion (MSE), in particular in Navarino Island, within the Cape Horn Biosphere Reserve; 2) the sub–Antarctic island of South Georgia (SG), including individuals from Bird Island (BI) and the South Georgian mainland (TP, STR and HB); and 3) the Maritime Antarctic (MA) specifically King George Island (KGI), Deception Island (DCI) and Livingston Island (LVI) in the South Shetland Islands (SSI). Adult flies were sampled during the austral summer from 2017 to 2023 from their natural habitats around lakes with the use of an entomological aspirator. Additionally, larvae and adults were also manually collected on Navarino Island from aquatic mosses in a lake near the summit of Bandera Hill, located close to the north coast of the island. All individuals were preserved in 95% ethanol and their geolocation was later included in GBIF (see Gañan et al., 2020). Additional searches were conducted in the Falkland/Malvinas Islands and South Orkney Islands, but no P. steinenii were observed. Taxonomic identity of the specimens was confirmed following Edwards and Usher (1985).
Figure 1 Historical biogeography reconstruction based on 151 cox1 sequences of Parochlus steinenii across the sampling areas. (A) Map of the sampling region in the Magellanic Subantarctic regions (MSE, red); sub-Antarctic Island of South Georgia (SG, orange), and Maritime Antarctic (MA, violet); (B) Bayesian Inference reconstruction using P. steinenii individuals. The values for node support are indicated for posterior probability/bootstrap from BI and ML analyses, respectively.
DNA was extracted using two main protocols, QIAGEN DNeasy Blood & Tissue, following (Maturana et al., 2022), and the QiAMP Extraction Kit, following the manufacturer’s protocols. All individuals were fully submerged in proteinase K+ATL buffer solution for 4 h at 56°C or overnight at 40°C; adults were not crushed to retain them as complete as possible for morphological examination, while larvae were partially or fully crushed before extraction.
Two DNA loci were amplified, two segments of the mitochondrial cytochrome c oxidase subunit I (cox1), and one segment of the nuclear DNA 28S rRNA gene. For the two segments of cox1, we used the universal primers LCO1490 and HCO2198 (Folmer et al., 1994) and UEA5 (AGTTTTAGCAGGAGCAATTACTAT) and UEA10 (TCCAATGCACTAATCTGCCATATTA) (Lunt et al., 1996), while for the 28S rRNA we used rD1.2a (CCCSSGTAATTTAAGCATATTA) and rD5a (GGYGTTGGTTGCTTAAGACAG) (Whiting, 2002). Detailed descriptions of the protocol and techniques used in the Polymerase Chain Reaction are given in Supplementary Material (S2). The products obtained were commercially sequenced by LGC Genomics (Germany) and Macrogen (South Korea).
For the phylogenetic reconstructions we incorporated the sequences of P. steinenii into a comprehensive dataset depicting the main lineages of the genus Parochlus Enderlein1912 (Supplementary Table S3). Homologous DNA sequences were independently aligned for each genetic locus using MAFFT v7.505 (Katoh and Standley, 2013) using the web server (Katoh et al., 2019). We conducted traditional phylogenetic estimations using both Maximum Likelihood (ML) and Bayesian Inference (BI) algorithms. The appropriate sequence evolution model for each genetic locus was determined using bModelTest v1.2.1 (Bouckaert and Drummond, 2017) and ModelFinder (Kalyaanamoorthy et al., 2017). The GTR+Γ model was used for cox1 and the HKY+I+Γ model for 28S rRNA. We prepared a phylogenetic tree for each locus to check congruence between both datasets (Supplementary Figure S4). Subsequently, the sequences were concatenated, and we performed posterior analyses on a partitioned dataset, considering each genetic locus. ML reconstruction was carried out using RAxML v8.2.12 (Stamatakis, 2014) and the node support was assessed through a bootstrap analysis with 1000 pseudo-replicates. The tree was rooted with Zavrelimyia sp. following Cranston et al. (2010). Bayesian reconstruction was performed in MrBayes3 v2.6.7 (Ronquist et al., 2012). The BI analysis was run three times for 100 million generations each time, with tree sampling every 10,000 generations. The consensus tree considered a burn-in period of 25%. For the intraspecific phylogenetic tree using the complete cox1 dataset, we make a midpoint rooted Bayesian tree reconstruction based on GTR+CAT model for ML and GTR+Γ for BI, respectively. Convergence was assessed by checking that split frequencies had an average standard deviation below 0.01 and all parameters had effective sample sizes (ESS) > 200 using Tracer v.1.6 (Rambaut et al., 2014).
Levels of genetic polymorphism were determined for each locus using standard diversity indices including number of haplotypes/alleles (K), number of segregation sites (S), haplotype/allelic diversity (H), average number of pairwise differences (Π) and nucleotide diversity (π) for each of the sampling areas, including the entire MA (KGI, DCI and LVI), sub-Antarctic (TP, BI), and the MSE using DnaSP v6.12.3 (Rozas et al., 2017). We estimated the number of private alleles for each sampling area and region as a proxy to assess the potential existence of refugia (Maggs et al., 2008). Neutrality tests (Tajima’s D and Fu’s FS) were performed to assess deviation from the neutral model. Significant negative values for these tests provide evidence of excess of rare polymorphism in a population, indicating either recent demographic expansion or positive selection. Genealogical relationships were estimated for mitochondrial sequences by constructing a median-joining haplotype network (Bandelt et al., 1999) using PopArt v1.7 (Leigh et al., 2015).
We estimated levels of genetic differentiation among the recognized groups (MSE, SG and SSI), among populations within those groups, and within populations, through mean pairwise differences (ΦST, using Kimura-2P genetic distances) and haplotype frequencies (FST) in ARLEQUIN v3.5.2.2 (Excoffier and Heckel, 2006). To test statistical significance of differentiation, we performed a permutation test (20,000 iterations). The p-value for pairwise ΦST and FST between populations was corrected using the false discovery rate correction (FDR; Benjamini et al., 2005). We used two different clustering methods to determine the spatial genetic structure and the spatial boundaries among them in P. steinenii. First, we used the GENELAND v4.0.7 package (Guillot et al., 2005) in the software R v3.6.1 (R Development Core Team, 2019). This Bayesian approach uses the Markov Chain Monte Carlo (MCMC) procedure to estimate the optimal clustering of samples based on geographic information. The analysis considers that spatially structured clusters are more probable than randomly distributed clusters in space. The most probable number of populations (K) was identified through ten independent MCMC analyses, each consisting of 10 million iterations with a thinning interval of 1000 iterations using the correlated frequency model. The range was limited between K = 1 and K = 5, with a burn-in of 25%. Second, we conducted an analysis of molecular variance (AMOVA) in Arlequin v3.5.2.2 to estimate the proportion of genetic variation explained by partitioning sampling sites into the different demographic units detected. This analysis characterizes spatial genetic structure by partitioning the variance within populations, among populations within groups and among groups.
We examined historical demography of the different previously identified genetic clusters (GENELAND and AMOVA), based on the cox1 marker. First, we compared observed mismatch distributions (Rogers and Harpending, 1992) implemented in DnaSP. We calculated Harpending’s Raggedness index (rH) in Arlequin v3.5.2.2 to test the unimodality of observed data. Second, we estimated population growth trends over time, through Bayesian Skyline Plots (BSP, Drummond and Bouckaert, 2014) implemented in BEAST v2.5 (Bouckaert et al., 2019). The running conditions considered 100 million iterations with parameter sampling every 10,000 steps, discarding the initial 25% of steps in the analysis. We used a population substitution rate of tenfold the evolutionary rate (7.5% per million year, Martin et al., 2002a, b) to estimate the most recent common ancestor (TMRCA) of each genetic group, as this more accurately reflects the rate at which new haplotypes appear (Ho et al., 2011, 2015). This better accounts for the time-dependence of molecular evolution at population level, brings divergence estimates closer to the present and avoids over-estimation of recent divergences when analysing intraspecific lineages.
To estimate divergence times, we utilized BEAST v2.5 with a reduced dataset comprising mitochondrial haplotypes retrieved using DnaSP. We performed a phylogenetic reconstruction combining a phylogenetic mutation rate with a population substitution rate (Ho et al., 2008). We estimated divergence times between the two main clades (Clade A and Clade B) using a phylogenetic rate, as it relates to the substitution rate at which mutation are fixed among clades. This was conducted using an optimized relaxed clock (ORC) model (Douglas et al., 2021) with a substitution rate of 0.75% per million years, a rate proposed for Chironomidae (Martin et al., 2002a, b) and previously used for Parochlus (Allegrucci et al., 2012). For the mutation rate, we used a population rate obtained to estimate the TMRCA of each clade, as it considers the rate at which new haplotypes appear in the clade. Three independent runs of 100 million generations each were conducted, with samples collected every 10,000 iterations. Convergence of results was assessed using Tracer v1.7.2 (Rambaut et al., 2018), and the results were summarized in a single ultrametric tree using TreeAnnotator v2.5.1 (Drummond et al., 2012). The final tree was constructed using the results of both approaches with the Chronos function in ape v5.7-1package (Paradis, 2013) in R, using a sequential secondary age calibration with the time of divergence of the main clades and TMRCA of each lineage (see another example in Pérez-Alvarez et al., 2021).
Within the framework of this study, we sampled from 16 freshwater sites within the species’ reported distribution across Maritime and sub-Antarctic regions and southern South America (Figure 1A, for more details see Supplementary Material S1, and Gañán et al., 2021). Two sites were in the MSE (IN = 9, NI = 12 sequences), four in sub-Antarctic South Georgia (BI = 5, TP = 16, STR = 14, HB = 11), and 10 in the South Shetland Islands (KGI = 40, DCI = 18, LVI = 26). The results of Xia’s test showed low substitution saturation (Iss = 0.2; Iss.c = 0.8; p < 0.05) under the assumption of both symmetrical and asymmetrical tree topologies for all OTU subsets. Similarly, PhyloMad results indicated a low risk of substitution saturation for cox1, considering codon positions 1–2 and position 3 independently. Regarding the simple linear regression plots of genetic distances (uncorrected vs. model corrected) identified deviations for cox1 codon position 3 only (R2 = 0.784). Plots for codons 1 and 2 combined indicated strong linear correlations between uncorrected and model-corrected genetic distances (R2 = 1). When codon 3 was combined with codons 1 and 2, the coefficient of determination remained high (R2 = 0.995), supporting the inclusion of codon 3 for estimating evolutionary relationships. For interspecific phylogenetic tree we used a concatenated data considering one fragment of 699 bp length of cox1 (under the Folmer universal primers) and 28S rRNA, resulting in 1347 bp. This concatenated dataset included 264 and 213 segregating sites for cox1, and 28S rRNA, respectively. For the intraspecific analyses, a total of 151 sequences for cox1 and 26 sequences for 28S rRNA were obtained. However, there was no variation in the 28S rRNA sequences within P. steinenii, so no further population analyses were carried out using this locus. We included a final alignment of two cox1 fragments – considering two mitochondrial fragments obtained from Folmer’s universal and UEA set of primers – of a total length of 1470 bp, with 80 variable sites (5.4%), and did not include insertions/deletions or stop codons.
The haplotype tree topology of P. steinenii identified the divergence of two main clades, (A) and (B), with high posterior probability (PP) and bootstrap support (BS) (Figure 1B). Clade B showed a well-supported SG clade, separated from remaining but unresolved SSI specimens (Figure 1B). The concatenated 28S rRNA and cox1 phylogenetic reconstruction recovered the relationships within the subfamily Podonominae and the monophyly of P. steinenii (Figure 2). At the same time, individuals from the Maritime Antarctic (MA) populations were more closely related to those from sub-Antarctic South Georgia (SG) than to those from the MSE (Allegrucci et al., 2006). Within the Parochlus clade, MA, MSE and SG groups formed a monophyletic group together with previously published sequences of P. steinenii from SG (Cranston et al., 2010). This clade was recovered in an early branching event relative to the other available Parochlus species sequences from northern latitudes of South America (Peru, Chile, Argentina) and New Zealand (Figure 2).
Figure 2 Phylogenetic reconstruction of Podonominae based on concatenated data. Maximum Likelihood reconstruction, including members of the subfamily Podonominae with emphasis on Parochlus spp. Information in brackets represent the sequence code used for the analysis (within P. steinenii), and sampling site for each sequence. Values for the nodes support are indicated for posterior probability/bootstrap, respectively.
Global mitochondrial haplotype diversity (Hd) was 0.922, with no substantial variation among the three biogeographic regions analyzed (Table 1). The MSE population exhibited the highest level of diversity, in terms of nucleotide diversity (Π = 7.44) and fewer haplotypes (K = 11), despite having a smaller sample size (n = 21). The sub-Antarctic SG and the Maritime Antarctic SSI populations showed similar genetic diversity patterns in terms of nucleotide diversity (Π = 3.04 and 1.2, respectively) and numbers of haplotypes (K = 27 and 28, respectively).
Table 1 Diversity indices and neutrality tests for Parochlus steinenii sampled across three biogeographic regions.
The median-joining haplotype network showed three main groups, without any shared haplotypes (Figure 3). The MSE was the most distinct haplogroup, separated by 10 mutational steps from MA and SG. It also displayed a more expanded genealogy, with several mutational steps between each haplotype, contrasting with those in MA and SG, which displayed a star-like topology and short genealogy.
Figure 3 Haplotype network for Parochlus steinenii based on 151 mtDNA cox1 sequences spanning the species’ distribution. Neighbor-joining network illustrating the distribution of haplotypes across lakes in the Magellanic Subantarctic Ecoregion, South Georgia and the South Shetland Islands. Circles sizes are proportional to haplotype frequency.
The Bayesian analysis of the number of genetic groups and the spatial clustering algorithm obtained with GENELAND detected three genetic clusters (K = 3, PP = 0.65, Figure 3), in agreement with the three biogeographic regions (i.e., MSE, SG and MA). The posterior probabilities associated with the definition of populations was on average 0.7, corresponding to the boundary between these clusters across the Polar Front (PF), which is located northern of SSI and SG (Figure 4). Similarly, AMOVA of comparison of pairwise differences (Supplementary Table S5), also confirmed the greatest differentiation between MSE and the combination of SG and SSI (70.41%, FCT = 0.704, p < 0.01), while differentiation within groups only explained 2.72% (FSC = 0.092, p < 0.01) of the total genetic variance. Pairwise comparison based on two statistics related to genetic (FST) and phylogeographic (ΦST) differentiation, showed significant structure across the three main biogeographic areas (Supplementary Table S6). In particular, the levels of genetic differentiation within SSI and SG were very low (SSI: FST = 0.145, ΦST = 0.052; SG: FST = 0.106, ΦST = 0.199, for more details see Supplementary Table S7).
Figure 4 Spatial genetic structure of Parochlus steinenii from the spatial model in Geneland across the three biogeographic regions sampled. Higher posterior probabilities of population membership are indicated in yellow for each sampling site (A) MSE, (B) SG, (C) MA. Black circles indicate the relative position of the sampling localities. Posterior probabilities of membership were plotted with the shapefiles of Scotia Arc coastline available in the repository in the Antarctic digital database from the British Antarctic survey (BAS). https://add.data.bas.ac.uk.
As expected for star-like genealogy, both neutrality tests Tajima’s D and Fu’s Fs were highly negative and significant (Table 1) for MA and SG, but positive and not significant for the MSE. The distribution of pairwise differences analyzed through Mismatch Analyses (MMA), varied between the different demographic units of P. steinenii. The MA and SG showed a typical unimodal curve and smooth distribution (Figure 5), representing a classical population expansion. In case of the SG populations, the curve moved to the right, indicating more mutation accumulation over time. As expected, the Harpending’s raggedness index was not significant in SG (rH = 0.027, p > 0.05) or MA (rH = 0.079, p > 0.05). Likewise, the analysis revealed a non-significant SSD value in SG (SSD = 0.001, p > 0.05) and SSI (SSD = 0.003, p > 0.05), supporting a spatial expansion for both populations In contrast, the MSE population exhibited a multimodal distribution (Figure 5) and its Harpending’s raggedness index was significant (rH = 0.099, p < 0.05). Likewise, the analysis revealed a significant SSD value (SSD = 0.060, p < 0.05), refuting the demographic model of spatial expansion for the South American population and, rather, supporting population equilibrium. Based on Bayesian Inference phylogenetic reconstruction, haplotype network and the genetic differentiation index, we identified the three haplogroups aligning with the three specific geographic areas as separated and independent demographic units. Bayesian Skyline Plot analyses were conducted using the 10× corrected substitution rate (Figure 5), providing historical population dynamic patterns and dates for TMRCA for the three demographic and evolutionary units (MA, SG and MSE). MSE had the oldest TMRCA of ca. 112,000 YBP (95% highest posterior density 95%HPD = 47.8 – 200 ka), followed by SG (ca. 50,000 YBP; 95%HPD: 19.6 – 92.1 ka), and finally MA (ca. 30,000 YBP; 95%HPD: 10.2 – 65.9 ka). The date of the onset of population expansion in SSI was estimated at ca. 10,000 YBP, while SG expanded earlier at ca. 20,000 YBP. For the MSE population, we did not detect a population expansion signal. Values of TMRCA were within the same range values obtained from the τ = 2μt values from the Mismatch Analyses (MSE: τ = 4.005, 36,035 YBP; SG: τ = 3.043, 13,500 YBP; MA: τ = 1.295, 11,655 YBP) (Figure 5).
Figure 5 Historical demographic trajectories of Parochlus steinenii within its distribution in the Magellanic Subantarctic Ecoregion (red), South Georgia (yellow) and South Shetland Islands (violet). Left panels: Past demographic changes constructed using Bayesian Skyline Plot approach based on cox1 haplotypes. The y-axis is the product of the effective population sizes (Ne) and generation length in a log scale. The x-axis is the time before present (Myr). The median estimate (solid black line) and 95% highest probability density (HPD) limits (colored area) are shown. The thick dashed line represents the time of the most recent common ancestor (TMRCA). Right panels: Distribution of pairwise differences of cox1 for each demographic unit. Values of Tau are shown.
The haplotype phylogenetic reconstruction based on cox1 shows a clear assortment of haplotypes according to their geographic origin (MSE, SG and MA), as previously observed in the concatenated phylogenetic reconstruction (Figure 1), and the haplotype network (Figure 2). Using sequential calibration points, the divergence times estimated among the main clades of Parochlus suggest the MSE lineage as the first clade-group to diverge (Clade A), around 1.46 Mya (95%HPD): 0.87 – 2.10 Mya), followed by the divergence between MA and SG lineages (Clade B) around 0.84 Mya (95%HPD: 0.50 – 1.25 Mya). The age for each population node was obtained from the TMRCA in BSP analyses (Figure 6).
Figure 6 Phylogenetic reconstruction based on haplotypes cox1 data showing divergence times of Parochlus steinenii across its distribution in sub- and maritime Antarctica. Nodes ages are the median values from both Bayesian Molecular Clock analyses and TMRCA of each clade estimated with Bayesian Skyline Plot. In each clade of interest (MSE, SG and MA), nodes bar indicated the 95%HPD. The colored tip represents the colors of the sampling region.
Our molecular data are consistent with previous phylogenetic analyses of Parochlus (Allegrucci et al., 2006; Cranston et al., 2010), in suggesting a sub-Antarctic and Antarctic clade that is distinct from all remaining Parochlus species. We identified two distinct lineages currently distributed in Clade (A) MSE, and Clade (B) MA and SG. Additionally, we detected a second branching event within Clade B, differentiating specimens from SG and MA. Based on intraspecific phylogeographic, demographic inference and genetic structure, this finding suggests three distinct evolutionary and demographic units, matching the three sampled biogeographic regions.
Given the existence of two clades whose origin is during the Pliocene (1.46 Mya), the presence of private alleles in each population, the deeply diverged haplogroups/clades and distinct reciprocally monophyletic cluster, suggest the presence of separated and multiple refugia within the present distribution of the species. The diversification of P. steineni agrees with the start of the intensification of the glacial cycles during the Pliocene–Pleistocene (Zachos et al., 2001). These results support the long-term persistence of P. steinenii in sub-Antarctic and Maritime Antarctic regions, adding further supporting evidence to the recurring pattern of long-term persistence across a range of Antarctic terrestrial and freshwater invertebrates (see Convey et al., 2020 and citation therein; Verleyen et al., 2021; Maturana et al., 2022; Short et al., 2022). Though phylogeographic approaches can identify one of the outcomes of isolation from ancestral panmixia due to refugia (allopatry among populations), we must also consider factors that maintain regional distinction during interglacial periods, such as physical, environmental or selective mechanisms that may limit gene flow among populations (Maggs et al., 2008).
The representatives of Clade A were collected on Navarino Island in the Cape Horn Biosphere Reserve (CHBR), south of Tierra del Fuego. The lake sampled is located below Bandera Hill at 700 m a.s.l, and represents the only population sampled for genetic analyses within the MSE. The species has also been recorded at Horn Island, slightly further south in the Cape Horn archipelago, from a lake at similar altitude (see Gañan et al., 2020). The MSE population is characterized by high genetic diversity, an extended network topology and older TMRCA, with no signal of past demographic growth. Such genetic and demographic patterns suggest that this population maintained a significant effective population size during the intensification of glacial cycles throughout the Pleistocene, and has not experienced an interglacial population expansion unlike SG and MA. Additionally, the MSE population had a positive neutrality test, meaning a deficit of low frequency haplotypes and high divergence between them. Therefore, we proposed that P. steinenii is a polar/cold-adapted species with effective interglacial refugia at high altitude in MSE (see cryptic southern refugia section, Stewart et al., 2010), as described for other terrestrial examples in the Northern Hemisphere (Hirao et al., 2015). This plausible scenario of P. steinenii as a cold-adapted species allows us also to explain 1) the disjunct distribution and high percentage of divergence from representatives from SG and MA (which represent polar refugia at higher southern latitudes); 2) the persistence in high altitude ecosystems such as small rivers and marginal meltwater channels, similar to the habitats in which they are found today in their current MSE distribution range (Contador et al., 2023); and 3) the abundance and frequency of occurrences within South Shetland Islands, in particular Kitiesh Lake in King George Island where the population density is extremely high (T. Contador personal observations).
Conversely, the contemporary distribution of clade B included a broader allocation (SG, MA). In both populations, we found low levels of nucleotide diversity, unimodal curves in their Mismatch Analyses, and significant demographic expansion. Such a demographic pattern may reflect past contraction-expansion processes in response to climate oscillations, as has been frequently cited in phylogeographic studies of high-latitudes affected by the LGM (Provan and Bennett, 2008; Marko et al., 2010; Maturana et al., 2020). Considering that both SG and SSI are located south of the oceanic Antarctic Polar Front (APF), they would experience greater impact from ice-sheet advances during the LGM (Fraser et al., 2012; Hodgson et al., 2014). In consequence, these populations may have experienced strong bottlenecks due to drastic reductions in their population sizes. Further and ongoing research at small geographic scale, the use of more molecular markers (e.g. mitochondrial genomes), or more contemporary and polymorphic markers (e.g. SNPs), will be required to generate a possible scenarios with greater resolution for P. steinenii.
The archipelagoes of the Scotia Arc form a geological connection between the southern part of South America and the Antarctic Peninsula. The region is also one of Earth’s major ocean gateways and is critical for understanding the behavior of the main oceanographic currents and their influence on Southern Hemisphere biodiversity (Livermore et al., 2004; Scher and Martin, 2006). It is particularly complex in terms of its geology, glacial processes and the spatial distribution of biodiversity (Terauds et al., 2012). Studies based on available distribution records of vegetation (primarily bryophyte and lichen species) and certain groups of terrestrial invertebrates erected 16 distinct Antarctic Conservation Biogeographic Regions (ACBR, Terauds et al., 2012) in the region of Antarctic Treaty governance, three of which cover the western Antarctic Peninsula, South Shetland Islands and South Orkney Islands. This terrestrial biogeographic classification does not extend to the maritime Antarctic South Sandwich Islands, sub-Antarctic South Georgia, or the Magellan sub-Antarctic Ecoregion, contrary to the complex marine biogeographic regions, which have identified patterns and linkages across the Scotia Arc region (De Broyer and Koubbi, 2014; Koubbi et al., 2014; González-Wevar et al., 2022). Understanding the extent to which these biogeographical regions apply to any particular taxon, and how distributions may change given the appearance of new ice-free areas (Lee et al., 2022), are key to informing how biosecurity protocols should be implemented to minimize risks of anthropogenic transfer of native biota between different parts of this region (van Vuuren et al., 2018; Cukier et al., 2023) and in particular in freshwater ecosystems, which have not previously been included in such studies.
Based on our P. steinenii mtDNA sequences obtained from 16 locations across Maritime Antarctica (SSI) and different sub-Antarctic regions (SG, MSE), we detected strong genetic and phylogeographic differentiation combined with ancient population divergence. Our data support the deep divergence between Clade A and B, which may provide evidence of a speciation processes (genealogical lineages). This hypothesis of species differentiation within the overall distribution was also noted and discussed by Allegrucci et al. (2006). However, speciation processes must be considered carefully, since the nDNA did not detect any differentiation between both clades. The lack of any differentiation in the nuclear 28S rRNA gene versus the mtDNA is likely to reflect lower mutation rates in the nuclear gene and should be addressed in future studies by the inclusion of more nuclear genes.
Finally, our phylogeographic and genetic divergence estimates for P. steinenii are comparable to those reported in studies of other sympatric Maritime Antarctic invertebrates (McGaughran et al., 2010; van Vuuren et al., 2018; McGaughran et al., 2019), and the endemic Antarctic midge B. antarctica (Allegrucci et al., 2012; Edgington et al., 2023). Additionally, these divergence time estimates are consistent with those recently reported for the freshwater copepod, Boeckella poppei, based on cox1 sequences obtained across the same three biogeographic regions (Maturana et al., 2022).
The populations of P. steinenii examined in this study exhibited strong genetic and phylogeographic structure across the Maritime Antarctic, South Georgia and the Magellanic sub-Antarctic Ecoregion. This structure is also reflected in the phylogenetic reconstruction carried out, in which we found two main clades separating the MSE and SG/MA, with a subsequent branching between the latter. Based on the contrasting phylogeographic and demographic patterns between southern South America and Antarctica, we propose that P. steinenii is a cold-adapted polar species with interglacial refugia in the high mountains of southern Patagonia. The strong genetic and phylogeographic structure, as well as the timing of divergence, are in agreement with patterns reported in other sympatric terrestrial invertebrates. Further analyses focusing on multiple nuclear loci are required to confirm the possible speciation processes and document contemporary fine scale patterns within these three regions to fully understand the connectivity and dispersal mechanisms behind the different biogeographic scenarios proposed for P. steinenii. The movement of individuals between local populations is a crucial factor in the persistence and dynamics of entire meta-populations, especially in changing, fragmented and extreme environments (Lakovic et al., 2015). Understanding past and present connectivity between populations of the Antarctic winged midge can provide valuable insights into how resilient or responsive the species may be in the face of ongoing climate change.
The original contributions presented in the study are publicly available. This data can be found here: NCBI GenBank, accession PP824188–PP824338.
The animal study was approved by Instituto Antartico Chileno Instituto de Biodiversidad Antártica y Subantártica. The study was conducted in accordance with the local legislation and institutional requirements.
CSM: Conceptualization, Data curation, Formal analysis, Methodology, Writing – original draft, Writing – review & editing, Investigation, Software, Visualization. TCM: Funding acquisition, Investigation, Project administration, Resources, Supervision, Writing – review & editing. FS: Data curation, Writing – review & editing. MAV: Methodology, Software, Visualization, Writing – review & editing. PV: Data curation, Writing – review & editing. MG: Visualization, Writing – review & editing. CG-W: Funding acquisition, Validation, Writing – review & editing. EP: Investigation, Resources, Validation, Writing – review & editing. CS: Validation, Writing – review & editing. PC: Funding acquisition, Investigation, Supervision, Validation, Writing – original draft, Writing – review & editing.
The author(s) declare financial support was received for the research, authorship, and/or publication of this article. This work was funded by ANID – Millennium Science Initiative Program – ICN2021_002, ANID/BASAL FB210018 from Cape Horn International Centre (CHIC), FONDECYT project 11130451, the Chilean Antarctic Institute (project RT_48_16), ANID-Postdoctoral Grant 2110063 to CSM, 3220352 to MAV and NERC core funding to the BASE Ecosystems and Biodiversity, Evolution and Adaptation Teams to PC. FS's PhD scholarship was supported by CNPQ and the Cambridge Trust (Process no. 233923/2014-4).
Maritime Antarctic and South Georgia material was collected under permits from INACH and the Government of South Georgia and the South Sandwich Islands. We thank the Captain, officers, crew and expedition staff of Commandant Charcot and, in particular, Science Officer Geoffroy Dekersauson, from Ponant expedition LEG CC120323. We thank Javier Rendoll Cárcamo, Simón Castillo, Ramiro Bustamante, Gilliam Graham and Gonzalo Arriagada for their help in the different stages of this project.
The authors declare that the research was conducted in the absence of any commercial or financial relationships that could be construed as a potential conflict of interest.
The author(s) declared that they were an editorial board member of Frontiers, at the time of submission. This had no impact on the peer review process and the final decision.
All claims expressed in this article are solely those of the authors and do not necessarily represent those of their affiliated organizations, or those of the publisher, the editors and the reviewers. Any product that may be evaluated in this article, or claim that may be made by its manufacturer, is not guaranteed or endorsed by the publisher.
The Supplementary Material for this article can be found online at: https://www.frontiersin.org/articles/10.3389/fevo.2024.1393376/full#supplementary-material
Allegrucci G., Carchini G., Convey P., Sbordoni V. (2012). Evolutionary geographic relationships among orthocladine chironomid midges from marine Antarctic and sub-Antarctic islands. Biol. J. Linn. Soc 106, 258–274. doi: 10.1111/bij.2012.106.issue-2
Allegrucci G., Carchini G., Todisco V., Convey P., Sbordoni V. (2006). A molecular phylogeny of antarctic chironomidae and its implications for biogeographical history. Polar Biol. 29, 320–326. doi: 10.1007/s00300-005-0056-7
Bandelt H. J., Forster P., Rohl A. (1999). Median-joining networks for inferring intraspecific phylogenies. Mol. Biol. Evol. 16, 37–48. doi: 10.1093/oxfordjournals.molbev.a026036
Benjamini Y., Krieger A. M., Yekutieli D. (2005). Adaptive linear step-up procedures that control the false discovery rate. Biometrika 93, 491–507. doi: 10.1093/biomet/93.3.491
Biersma E. M., Torres-Diaz C., Molina-Montenegro M. A., Newsham K. K., Vidal M. A., Collado G. A., et al. (2020). Multiple late-Pleistocene colonisation events of the Antarctic pearlwort Colobanthus quitensis (Caryophyllaceae) reveal the recent arrival of native Antarctic vascular flora. J. Biogeogr. 47, 1663–1673. doi: 10.1111/jbi.13843
Bouckaert R. R., Drummond A. (2017). Bmodeltest: bayesian phylogenetic site model averaging and model comparison. BMC Evol. Biol. 17, 42. doi: 10.1186/s12862-017-0890-6
Bouckaert R., Vaughan T. G., Barido-Sottani J., Duchene S., Fourment M., Gavryushkina A., et al. (2019). BEAST 2.5: An advanced software platform for Bayesian evolutionary analysis. PloS Comput. Biol. 15, e1006650. doi: 10.1371/journal.pcbi.1006650
Brundin L. (1966). Transantarctic relationship and their significance, as evidenced by chironomid midges. With a monograph of the subfamilies Podonominae and Aphroteniinae and the austral Heptagyiae. K. Svenska Vetensk Akad. Handl. 11, 1–472.
Chown S. L., Convey P. (2016). Antarctic entomology. Annu. Rev. Entomol. 61, 119–137. doi: 10.1146/annurev-ento-010715-023537
Clapperton C. M. (1990). Quaternary glaciation in the Southern Ocean and Antarctic Peninsula area. Quater. Sci. Rev. 9, 229.252. doi: 10.1016/0277-3791(90)90020-B
Clapperton C. M., Sugden D. E. (1988). Holocene glacier fluctuations in South America and Antarctica. Quater. Sci. Rev. 7, 185–198. doi: 10.1016/0277-3791(88)90005-4
Contador T., Ganan M., Rendoll-Carcamo J., Maturana C. S., Benitez H. A., Kennedy J., et al. (2023). A polar insect’s tale: Observations on the life cycle of Parochlus steinenii, the only winged midge native to Antarctica. Ecology 104, e3964. doi: 10.1002/ecy.3964
Convey P., Biersma E. M. (2024). “Antarctic Ecosystems,” in Encyclopedia of Biodiversity, 3rd ed. Ed. Scheiner S. M. (Elsevier, Oxford), 133–148.
Convey P., Biersma E. M., Casanova-Katny A., Maturana C. S. (2020). “Refuges of Antarctic Diversity,” in Past Antarctica. Ed. Oliva M. (Elsevier (Barcelona, Barcelona).
Convey P., Gibson J. A., Hillenbrand C. D., Hodgson D. A., Pugh P. J., Smellie J. L., et al. (2008). Antarctic terrestrial life – challenging the history of the frozen continent? Biol. Rev. Cambridge Philos. Soc. 83, 103–117. doi: 10.1111/j.1469-185X.2008.00034.x
Convey P., Greenslade P., Richard K. J., Block W. (1996). The terrestrial arthropod fauna of the Byers Peninsula, Livingston Island, South Shetland Islands - Collembola. Polar Biol. 16, 257–259. doi: 10.1007/s003000050052
Cranston P. S., Hardy N. B., Morse G. E., Puslednik L., McCluen S. R. (2010). When molecules and morphology concur: the ‘Gondwanan’ midges (Diptera: Chironomidae). Syst. Entomol. 35, 636–648. doi: 10.1111/j.1365-3113.2010.00531.x
Cukier S., Fudala K., Bialik R. J. (2023). Are Antarctic aquatic invertebrates hitchhiking on your footwear? J. Nat. Conserv. 72, 126354. doi: 10.1016/j.jnc.2023.126354
De Broyer C., Koubbi P. (2014). “Chapter 1.1: The Biogegraphy of the Southern Ocean,” in Biogeographic Atlas of the Southern Ocean. Eds. De Broyer C., Koubbi P., Griffiths H. J., U. Raymond B. (Scientific Committee on Antarctic Research, Cambridge), 2–5.
Douglas J., Zhang R., Bouckaert R. (2021). Adaptive dating and fast proposals: Revisiting the phylogenetic relaxed clock model. PloS Comput. Biol. 17, e1008322. doi: 10.1371/journal.pcbi.1008322
Drummond A. J., Bouckaert R. R. (2014). Bayesian evolutionary analysis with BEAST 2 (Cambridge: C.U. Press).
Drummond A. J., Suchard M. A., Xie D., and Rambaut A. (2012). Bayesian Phylogenetics with BEAUti and the BEAST 1.7. Mol. Biol. Evol. 29, 1969–1973. doi: 10.1093/molbev/mss075
Edgington H., Pavinato V. A. C., Spacht D., Gantz J. D., Convey P., Lee R. E., et al. (2023). Genetic history, structure and gene flow among populations of Belgica Antarctica, the only free-living insect in the western Antarctic Peninsula. Polar Sci. 36. doi: 10.1016/j.polar.2023.100945
Edwards M., Usher M. B. (1985). The winged Antarctic midge Parochlus steinenii (Gerke) (Diptera: Chironomidae) in the South Shetland Islands. Biol. J. Linn. Soc 26, 83–93. doi: 10.1111/j.1095-8312.1985.tb01553.x
Excoffier L., Heckel G. (2006). Computer programs for population genetics data analysis: a survival guide. Nat. Rev. Genet. 7, 745–758. doi: 10.1038/nrg1904
Folmer O., Black M., Hoeh W., Lutz R., Vrijenhoek R. C. (1994). DNA primers for amplification of mitochondrial cytochrome c oxidase subunit I from diverse metazoan invertebrates. Mol. Mar. Biol. Biotechnol. 3, 294–299.
Fraser C. I., Ruzzante D. E., Waters J. M. (2012). Poleward bound: biological impacts of Southern Hemisphere glaciation. Trends Ecol. Evol. 27, 462–471. doi: 10.1016/j.tree.2012.04.011
Gañán M., Contador T., Rendoll J., Simoes F., Carolina P., Graham G., et al. (2021). Records of Parochlus steinenii in the Maritime Antarctic and sub-Antarctic regions. Zookeys 1011, 63–71. doi: 10.3897/zookeys.1011.56833
Gañan M., Contador T., Rendoll J., Simoes F., Pérez C., Graham G., et al. (2020). Records of Parochlus steinenii in the Maritime Antarctic and sub-Antarctic regions. doi: 10.15468/2cfwd7
González-Wevar C. A., Segovia N. I., Rosenfeld S., Maturana C. S., Jeldres V., Pinochet R., et al. (2022). Seven snail species hidden in one: Biogeographic diversity in an apparently widespread periwinkle in the Southern Ocean. J. Biogeogr. 49, 1521–1534. doi: 10.1111/jbi.14453
Guillot G., Mortier F., Estoup A. (2005). Geneland: a computer package for landscape genetics. Mol. Ecol. Notes 5, 712–715. doi: 10.1111/j.1471-8286.2005.01031.x
Hahn S., Reinhardt K. (2006). Habitat preference and reproductive traits in the Antarctic midge Parochlus steinenii (Diptera: Chironomidae). Antarct. Sci. 18, 175–181. doi: 10.1017/S0954102006000204
Hirao A. S., Watanabe Y. Y., Liu Q.-J., Li X., Masuzawa T., Ohara M., et al. (2015). Low genetic diversity and high genetic divergence in southern rear edge populations of Dryas octopetala in the high mountains of far East Asia. Japan. Soc. Plant System. 66, 11–22.
Ho S. Y., Duchene S., Molak M., Shapiro B. (2015). Time-dependent estimates of molecular evolutionary rates: evidence and causes. Mol. Ecol. 24, 6007–6012. doi: 10.1111/mec.13450
Ho S. Y., Lanfear R., Bromham L., Phillips M. J., Soubrier J., Rodrigo A. G., et al. (2011). Time-dependent rates of molecular evolution. Mol. Ecol. 20, 3087–3101. doi: 10.1111/j.1365-294X.2011.05178.x
Ho S. Y., Saarma U., Barnett R., Haile J., Shapiro B. (2008). The effect of inappropriate calibration: three case studies in molecular ecology. PloS One 3, e1615. doi: 10.1371/journal.pone.0001615
Hodgson D. A., Graham A. G. C., Roberts S. J., Bentley M. J., Cofaigh C.Ó., Verleyen E., et al. (2014). Terrestrial and submarine evidence for the extent and timing of the Last Glacial Maximum and the onset of deglaciation on the maritime-Antarctic and sub-Antarctic islands. Quater. Sci. Rev. 100, 137–158. doi: 10.1016/j.quascirev.2013.12.001
Kalyaanamoorthy S., Minh B. Q., Wong T. K. F., von Haeseler A., Jermiin L. S. (2017). ModelFinder: fast model selection for accurate phylogenetic estimates. Nat. Methods 14, 587–589. doi: 10.1038/nmeth.4285
Katoh K., Rozewicki J., Yamada K. D. (2019). MAFFT online service: multiple sequence alignment, interactive sequence choice and visualization. Brief Bioinform. 20, 1160–1166. doi: 10.1093/bib/bbx108
Katoh K., Standley D. M. (2013). MAFFT multiple sequence alignment software version 7: improvements in performance and usability. Mol. Biol. Evol. 30, 772–780. doi: 10.1093/molbev/mst010
Koubbi P., De Broyer C., Griffiths H. J., Raymond B., d’Udekem d’Acoz C., Van de Putte A. P., et al. (2014). “Chapter 12: Conclusions: Present and future of Southern Ocean Biogeography,” in Biogeographic atlas of the Southern Ocean. Eds. Koubbi P., De Broyer C., Griffiths H. J., Raymond B., d’Udekem d’Acoz C., Van de Putte A. P., D.B. Danis B., G.J. Grant S., Held C., Hosie G., Huettmann F., Post A., Ropert- Coudert Y., Stoddart M., Swadling K. M. (Scientific Committee on Antarctic Research, Cambridge).
Lakovic M., Poethke H. J., Hovestadt T. (2015). Dispersal timing: emigration of insects living in patchy environments. PloS One 10, e0128672. doi: 10.1371/journal.pone.0128672
Lee J. R., Waterman M. J., Shaw J. D., Bergstrom D. M., Lynch H. J., Wall D. H., et al. (2022). Islands in the ice: Potential impacts of habitat transformation on Antarctic biodiversity. Glob Chang Biol. 28, 5865–5880. doi: 10.1111/gcb.16331
Leigh J. W., Bryant D., Nakagawa S. (2015). popart: full-feature software for haplotype network construction. Methods Ecol. Evol. 6, 1110–1116. doi: 10.1111/2041-210X.12410
Livermore R., Eagles G., Morris P., Maldonado A. (2004). Shackleton Fracture Zone: No barrier to early circumpolar ocean circulation. Geology 32, 797. doi: 10.1130/G20537.1
Lunt D. H., Zhang D. X., Szymura J. M., Hewitt G. M. (1996). The insect cytochrome oxidase I gene: evolutionary patterns and conserved primers for phylogenetic studies. Insect Mol. Biol. 5, 153–165. doi: 10.1111/j.1365-2583.1996.tb00049.x
Maggs C. A., Castilho R., Foltz D., Henzler C., Jolly M. T., Kelly J., et al. (2008). Evaluating signatures of glacial refugia for North Atlantic benthic marine taxa. Ecology 89, S108–S122. doi: 10.1890/08-0257.1
Marko P. B., Hoffman J. I., Emme S. A., Mcgovern T. M., Keever C. C., Cox L. N. (2010). The ‘Expansion–Contraction’ model of Pleistocene biogeography: rocky shores suffer a sea change? Mol. Ecol. 19, 146–169. doi: 10.1111/mec.2009.19.issue-1
Martin J., Guryev V., Blinov A. (2002a). Population variability in Chironomus (Camptochironomus) species (Diptera, Nematocera) with a Holarctic distribution: evidence of mitochondrial gene flow. Insect Mol. Biol. 11, 387–397. doi: 10.1046/j.1365-2583.2002.00348.x
Martin J., Guryev V., Blinov A., Edward D. H. D. (2002b). A molecular assessment of the extent of variation and dispersal between Australian populations of the genus Archaeochlus Brundin (Diptera: Chironomidae). Invertebr. Syst. 16, 599–603. doi: 10.1071/IT01040
Maturana C. S., Biersma E. M., Díaz A., González-Wevar C., Contador T., Convey P., et al. (2022). Survivors and colonizers: Contrasting biogeographic histories reconciled in the Antarctic freshwater copepod Boeckella poppei. Front. Ecol. Evol. 10. doi: 10.3389/fevo.2022.1012852
Maturana C. S., Segovia N. I., González-Wevar C. A., Díaz A., Rosenfeld S., Poulin E., et al. (2020). Evidence of strong small-scale population structure in the Antarctic freshwater copepod Boeckella poppei in lakes on Signy Island, South Orkney Islands. Limnol. Oceanogr. 65, 2024–2040. doi: 10.1002/lno.11435
McGaughran A., Terauds A., Convey P., Fraser C. I. (2019). Genome-wide SNP data reveal improved evidence for Antarctic glacial refugia and dispersal of terrestrial invertebrates. Mol. Ecol. 28 (22), 4941–4957. doi: 10.1111/mec.15269
McGaughran A., Torricelli G., Carapelli A., Frati F., Stevens M. I., Convey P., et al. (2010). Contrasting phylogeographical patterns for springtails reflect different evolutionary histories between the Antarctic Peninsula and continental Antarctica. J. Biogeogr. 37, 103–119. doi: 10.1111/j.1365-2699.2009.02178.x
Mercer J. H. (1976). Glacial history of southermost South America. Quat. Res. 6, 125–166. doi: 10.1016/0033-5894(76)90047-8
Paradis E. (2013). Molecular dating of phylogenies by likelihood methods: a comparison of models and a new information criterion. Mol. Phylogenet. Evol. 67, 436–444. doi: 10.1016/j.ympev.2013.02.008
Pérez-Alvarez M., Kraft S., Segovia N. I., Olavarría C., Nigenda-Morales S., Urbán R J., et al. (2021). Contrasting phylogeographic patterns among northern and southern hemisphere fin whale populations with new data from the southern Pacific. Front. Mar. Sci. 8. doi: 10.3389/fmars.2021.630233
Pertierra L. R., Escribano-Álvarez P., Olalla-Tárraga M. Á. (2021). Cold tolerance is similar but heat tolerance is higher in the alien insect Trichocera maculipennis than in the native Parochlus steinenii in Antarctica. Polar Biol. 44, 1203–1208. doi: 10.1007/s00300-021-02865-w
Provan J., Bennett K. D. (2008). Phylogeographic insights into cryptic glacial refugia. Trends Ecol. Evol. 23, 564–571. doi: 10.1016/j.tree.2008.06.010
Rambaut A., Drummond A. J., Xie D., Baele G., Suchard M. A. (2018). Posterior summarization in bayesian phylogenetics using tracer 1.7. Syst. Biol. 67, 901–904. doi: 10.1093/sysbio/syy032
Rambaut A., Suchard M. A., Xie D., Drummond A. J. (2014). Tracer v1.6. Available at: http://beast.bio.ed.ac.uk/Tracer.
Rauschert M. (1985). Beobachtungen an der Chironomide Parochlus steineni auf der Insel King George (Südshetlandinseln, Antarktis)(Diptera, Chironomidae). Dtsch. Entomol. Z. 32, 183–188. doi: 10.1002/mmnd.v32:1/3
R Development Core Team. (2019). R: A Language and Environment for Statistical Computing (Vienna, Austria: R Foundation for Statistical Computing).
Rodbell D. T., Smith J. A., Mark B. G. (2009). Glaciation in the andes during the lateglacial and holocene. Quater. Sci. Rev. 28, 2165–2212. doi: 10.1016/j.quascirev.2009.03.012
Rogers A. R., Harpending H. (1992). Population growth makes waves in the distribution of pairwise genetic differences. Mol. Biol. Evol. 9, 552–569. doi: 10.1093/oxfordjournals.molbev.a040727
Ronquist F., Teslenko M., van der Mark P., Ayres D. L., Darling A., Hohna S., et al. (2012). MrBayes 3.2: efficient Bayesian phylogenetic inference and model choice across a large model space. Syst. Biol. 61, 539–542. doi: 10.1093/sysbio/sys029
Rozas J., Ferrer-Mata A., Sanchez-DelBarrio J. C., Guirao-Rico S., Librado P., Ramos-Onsins S. E., et al. (2017). DnaSP 6: DNA sequence polymorphism analysis of large data sets. Mol. Biol. Evol. 34, 3299–3302. doi: 10.1093/molbev/msx248
Scher H. D., Martin E. E. (2006). Timing and Climatic consequences of the opening of Drake Passage. Science 312, 428–430. doi: 10.1126/science.1120044
Shimada K., Ohyama Y., Pan C. (1991). Cold-hardiness of the Antarctic winged midge Parochlus steinenii during the active season at King George Island. Polar Biol. 11, 311–314. doi: 10.1007/BF00239023
Short K. A., Sands C. J., McInnes S. J., Pisani D., Stevens M. I., Convey P. (2022). An ancient, Antarctic-specific species complex: large divergences between multiple Antarctic lineages of the tardigrade genus Mesobiotus. Mol. Phylogenet. Evol. 170, 107429. doi: 10.1016/j.ympev.2022.107429
Stamatakis A. (2014). version 8: a tool for phylogenetic analysis and post-analysis of large phylogenies. Bioinformatics 30, 1312–1313. doi: 10.1093/bioinformatics/btu033
Stewart J. R., Lister A. M., Barnes I., Dalen L. (2010). Refugia revisited: individualistic responses of species in space and time. Proc. Biol. Sci. 277, 661–671. doi: 10.1098/rspb.2009.1272
Terauds A., Chown S. L., Morgan F., Peat H. J., Watts D. J., Keys H., et al. (2012). Conservation biogeography of the Antarctic. Divers. Distrib. 18, 726–741. doi: 10.1111/j.1472-4642.2012.00925.x
van de Wouw M., van Dijk P., Huiskes A. H. L. (2008). Regional genetic diversity patterns in Antarctic hairgrass (Deschampsia Antarctica Desv.). J. Biogeogr. 35, 365–376. doi: 10.1111/j.1365-2699.2007.01784.x
van Vuuren B. J., Lee J. E., Convey P., Chown S. L. (2018). Conservation implications of spatial genetic structure in two species of oribatid mites from the Antarctic Peninsula and the Scotia Arc. Antarct. Sci. 30, 105–114. doi: 10.1017/S0954102017000529
Verleyen E., Van de Vijver B., Tytgat B., Pinseel E., Hodgson D. A., Kopalová K., et al. (2021). Diatoms define a novel freshwater biogeography of the Antarctic. Ecography 44, 548–560. doi: 10.1111/ecog.05374
Whiting M. F. (2002). Mecoptera is paraphyletic: multiple genes and phylogeny of Mecoptera and Siphonaptera. Zool. Scr. 31, 93–104. doi: 10.1046/j.0300-3256.2001.00095.x
Keywords: Antarctic–Magellan connection, Diptera, Cape Horn, insect, past climate oscillations, phylogeography, speciation
Citation: Maturana CS, Contador Mejias T, Simões FL, Valladares MA, Vidal PM, Gañán M, González-Wevar CA, Poulin E, Sands CJ and Convey P (2024) Ancient diversification in extreme environments: exploring the historical biogeography of the Antarctic winged midge Parochlus steinenii (Diptera: Chironomidae). Front. Ecol. Evol. 12:1393376. doi: 10.3389/fevo.2024.1393376
Received: 29 February 2024; Accepted: 13 June 2024;
Published: 11 July 2024.
Edited by:
Mauro Fois, University of Cagliari, ItalyReviewed by:
Cristián E. Hernández, University of Concepcion, ChileCopyright © 2024 Maturana, Contador Mejias, Simões, Valladares, Vidal, Gañán, González-Wevar, Poulin, Sands and Convey. This is an open-access article distributed under the terms of the Creative Commons Attribution License (CC BY). The use, distribution or reproduction in other forums is permitted, provided the original author(s) and the copyright owner(s) are credited and that the original publication in this journal is cited, in accordance with accepted academic practice. No use, distribution or reproduction is permitted which does not comply with these terms.
*Correspondence: Tamara Contador Mejias, dGFtYXJhLmNvbnRhZG9yQHVtYWcuY2w=
Disclaimer: All claims expressed in this article are solely those of the authors and do not necessarily represent those of their affiliated organizations, or those of the publisher, the editors and the reviewers. Any product that may be evaluated in this article or claim that may be made by its manufacturer is not guaranteed or endorsed by the publisher.
Research integrity at Frontiers
Learn more about the work of our research integrity team to safeguard the quality of each article we publish.