- 1Département de biologie, chimie et géographie, Université du Québec à Rimouski, Rimouski, QC, Canada
- 2Groupe de recherche sur les environnements nordiques BORÉAS, Centre d’Études Nordiques, Centre de la Science de la Biodiversité du Québec, Université du Québec à Rimouski, Rimouski, QC, Canada
- 3Department of Integrative Biology, University of Windsor, Windsor, ON, Canada
- 4Department of Biology, Advanced Facility for Avian Research, University of Western Ontario, London, ON, Canada
- 5Department of National Defence, 8 Wing Environment, Astra, ON, Canada
Among birds, several body composition traits typically decrease in size or mass during breeding likely as a result of competing demands during this critical life history stage. However, a recent outdoor captive study in an Arctic-breeding cold-specialist songbird (snow buntings – Plectrophenax nivalis) demonstrated that these birds maintain winter cold acclimatization during the spring and summer, despite facing summer temperatures much warmer than on their Arctic breeding grounds. This suggests that buntings may face a cumulative physiological cost during breeding: having to support a winter phenotype while also upregulating additional traits for reproduction. The current study aimed to test this hypothesis. Between 2016 and 2019, we examined how body composition and metabolic performance (thermogenic capacity and physiological maintenance costs) changed from pre-breeding to chick provisioning in free-living birds captured at the northern limit of their breeding range in the Canadian Arctic (Alert, NU, 82°). While body mass and fat reserves deceased significantly between pre-breeding and territory defense independent of thermal conditions, cold endurance and associated traits remained stable and elevated up to the nestling provisioning period, as long as ambient temperature remained below a threshold level of 0–2°C. These results indicate that snow buntings must maintain a high thermogenic capacity after arrival on the breeding grounds if temperatures remain below freezing, regardless of whether birds are actively breeding or not. In this context, our research suggests that these birds, and possibly other arctic breeding songbirds, may experience cumulative physiological costs during years with a late onset of spring, when breeding activities (i.e., egg production and incubation) begin while temperatures are still below 0–2°C.
1 Introduction
The annual cycle of migratory birds is composed of several life-history stages, including wintering, migratory and reproduction, which typically occur in a determined temporal sequence (Wingfield et al., 1999; Ramenofsky et al., 2003). However, in species experiencing short time windows for reproduction, such as those breeding at high latitudes or altitude, or those with irruptive breeding events, these life-history stages may also overlap in time (Hahn, 1995; Ramenofsky and Wingfield, 2006, 2017). For example, Arctic breeding birds often experience substantial snow accumulation, sub-zero temperatures and unpredictable weather upon arrival on their breeding grounds (e.g., Meltofte, 1983; Walsh et al., 2005; see also Morrison et al., 2007; Wingfield et al., 2011). Under such conditions, behavioral observations show that an overlap between winter and reproductive phenotypes may occur (Ramenofsky and Wingfield, 2006, 2017). Wingfield et al. (2004) have indeed reported winter behaviours, namely high sociability, hyperphagia and high mobility in white crowned sparrows (Zonotrichia leucophrys gambelii) facing harsh environmental conditions upon arrival on their breeding ground at Brook’s Range (68°N), Alaska. These behaviours can be resumed later during the early stages of breeding if spring conditions deteriorate (Meltofte, 1983).
The occurrence of winter behaviors during the pre-breeding or breeding periods likely represents a way to cope with harsh or extreme conditions. However, other than endocrine regulation, which has received substantial attention (Wingfield et al., 2004; Ramenofsky et al., 2017; and see Ramenofsky and Wingfield, 2006 for a review) little is known about the underlying physiological adjustments implemented during these stage overlaps. In fact, taken separately, cold acclimatization and breeding involve significant morphological, physiological and metabolic changes to support energy demanding activities, which may or may not be compatible. For example, birds experiencing declines in ambient temperatures typically respond by increasing heart mass (Zheng et al., 2008; Petit and Vézina, 2014b), blood oxygen carrying capacity (hematocrit, Swanson, 1990b; O’Connor, 1996) and flight muscle size (O’Connor, 1995; Cooper, 2002; Swanson and Vézina, 2015; but see Barceló et al., 2017; Milbergue et al., 2018). Collectively these changes contribute to improving shivering heat production (Swanson, 1990b; Petit and Vézina, 2014a). In contrast, breeding birds typically loose both fat and muscle mass (Morrison et al., 2005; Morrison, 2006; Vézina et al., 2012), experience declines in oxygen carrying capacity (Morton, 1994; Morrison, 2006; Krause et al., 2016a) and develop large reproductive organs (Vézina and Salvante, 2010). At the moment, it is unclear to what degree these critical physiological transformations may overlap in time in support of breeding in cold environments such as the Arctic, especially considering that the timing of breeding can be closely tied to spring temperatures and snow cover (Høye et al., 2007; Moe et al., 2009; Grabowski et al., 2013).
Using captive snow buntings (Plectrophenax nivalis) as a model, Le Pogam et al. (2021b) recently showed that these Arctic cold specialists kept in outdoor aviaries on their wintering grounds (48°N) maintain thermogenic capacity and cold endurance comparable to the peak of winter over most of the summer, which corresponds to their breeding activities in the Arctic. In other words, birds retained a cold acclimated phenotype during summer temperatures that greatly exceeded what is experienced on their Arctic breeding grounds [e.g., mean ambient temperature (Ta) in July and August: 20.3°C, range: 15.9–25.0°C]. This finding contrasts with previous studies showing ambient temperature as the main driver of thermogenic capacity in passerine birds (e.g., Saarela and Heldmaier, 1987; McKechnie and Swanson, 2010; Swanson, 2010). Le Pogam et al. (2021b) thus concluded that the maintenance of winter-like cold endurance throughout summer could provide further downstream advantages given that these birds can face unpredictable and snowy sub-zero conditions while breeding in the Arctic (Meltofte, 1983).
Although observations in captive snow buntings provide evidence for life-history overlap in Arctic breeders (Tinbergen, 1939; Meltofte, 1983; Wingfield and Hunt, 2002; Le Pogam et al., 2021b), the study by Le Pogam et al. (2021b) was nonetheless based on non-breeding, captive individuals. However, investment in a breeding phenotype typically involves considerable physiological changes including the loss of body mass and a reduction in oxygen carrying capacity and muscle mass, which combined could reduce cold endurance (O’Connor, 1995; Cooper, 2002; Dubois et al., 2016). Consequently, while we know that snow buntings maintain cold endurance at winter levels during spring migration and arrival (Le Pogam et al., 2021a), it is currently unclear whether, and if so, for how long, these birds can maintain this level of cold endurance while breeding. In addition, recent evidence has also shown that shivering heat production and cold endurance can vary independently from changes in muscle size (Stager et al., 2015; Milbergue et al., 2018; Le Pogam et al., 2020) or other physiological systems (Petit et al., 2013; Barceló et al., 2017). Therefore, patterns observed in captive birds could still reflect true endogenous cycles in buntings adapted to breed in cold, unpredictable Arctic environments (Le Pogam et al., 2021b).
Based on these recent discoveries, we sought to determine i) how the transition from a post-arrival cold-acclimated phenotype into a breeding phenotype (i.e., pre-breeding, territorial defense and nesting) influences metabolic performance and associated traits in snow buntings, and ii) how spring thermal conditions may influence these patterns. We formulated three hypotheses and related predictions to meet these two objectives. First (Hypothesis 1, Figure 1), snow buntings could maintain a winter-type phenotype for traits related to cold endurance into breeding to safeguard against unpredictable sudden cold conditions (Le Pogam et al., 2021b). In this case, we would expect birds to maintain traits (Table 1) at constant pre-breeding levels as they transition to territorial defense and breeding, independently from environmental conditions (i.e., temperature). Second (Hypothesis 2, Figure 1), cold endurance traits (Table 1) could mainly be driven by thermal conditions (Saarela and Heldmaier, 1987; McKechnie and Swanson, 2010; Swanson, 2010; Swanson et al., 2014), independently from breeding phenotypes. We would then expect performance traits to respond only to temperature, irrespective of the birds’ breeding stages. In this scenario, as winter phenotypes appear to come with spare capacity, expressed as performance trait values plateauing below a certain ambient temperature (Petit and Vézina, 2014b; Swanson and Vézina, 2015; Vézina et al., 2020), one would expect snow buntings to lose cold endurance only when rising ambient temperature reaches a certain threshold value. The birds would then undergo physiological changes and lose cold endurance rapidly in years with early springs, or maintain capacity longer when cold conditions last later into the season. The third hypothesis (Hypothesis 3, Figure 1) posits that snow buntings are unable to maintain a winter-type phenotype while breeding due to physiological changes in traits related to cold endurance (Table 1). In this specific case, one would therefore expect metabolic performance parameters to drop as birds transition from arrival into breeding, irrespective of spring temperatures. To test these three hypotheses, we examined inter-annual variation in phenotypic traits and metabolic performance in free-living buntings captured in the Canadian High Arctic during the pre-breeding stage (i.e., transition stage between arrival and dispersal onto breeding territories), during male territorial establishment, and during breeding, and then examined how temperature variation was associated with changes in phenotypic patterns.
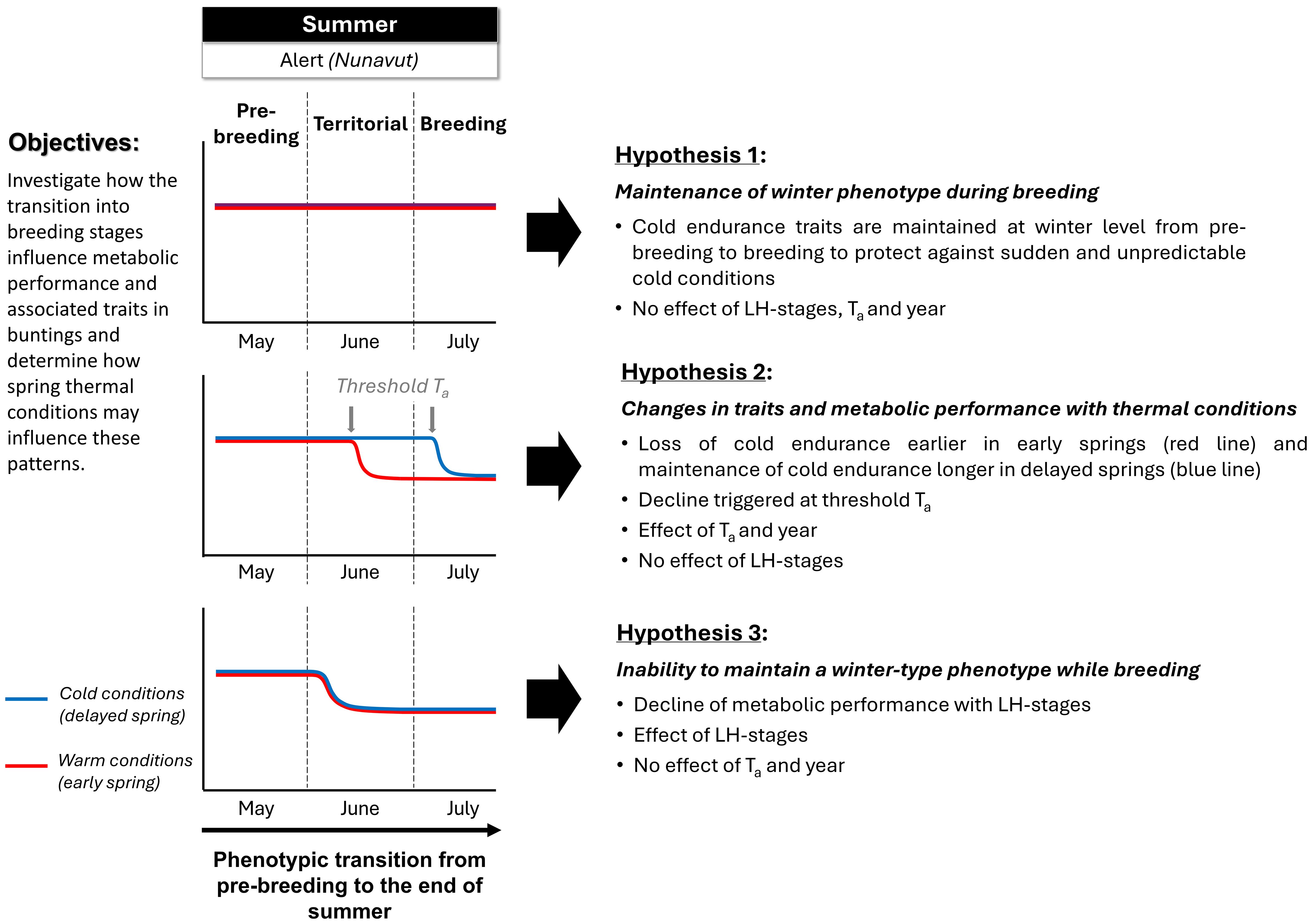
Figure 1 Visual representation of the three hypotheses tested in this study. Snow buntings could either maintain a winter-type phenotype through breeding (hypothesis 1), loose cold endurance when ambient temperature rises above a certain threshold, independently from breeding (hypothesis 2), or be unable to maintain cold endurance while breeding, irrespective of temperature (hypothesis 3). See text in figure and introduction for more details.
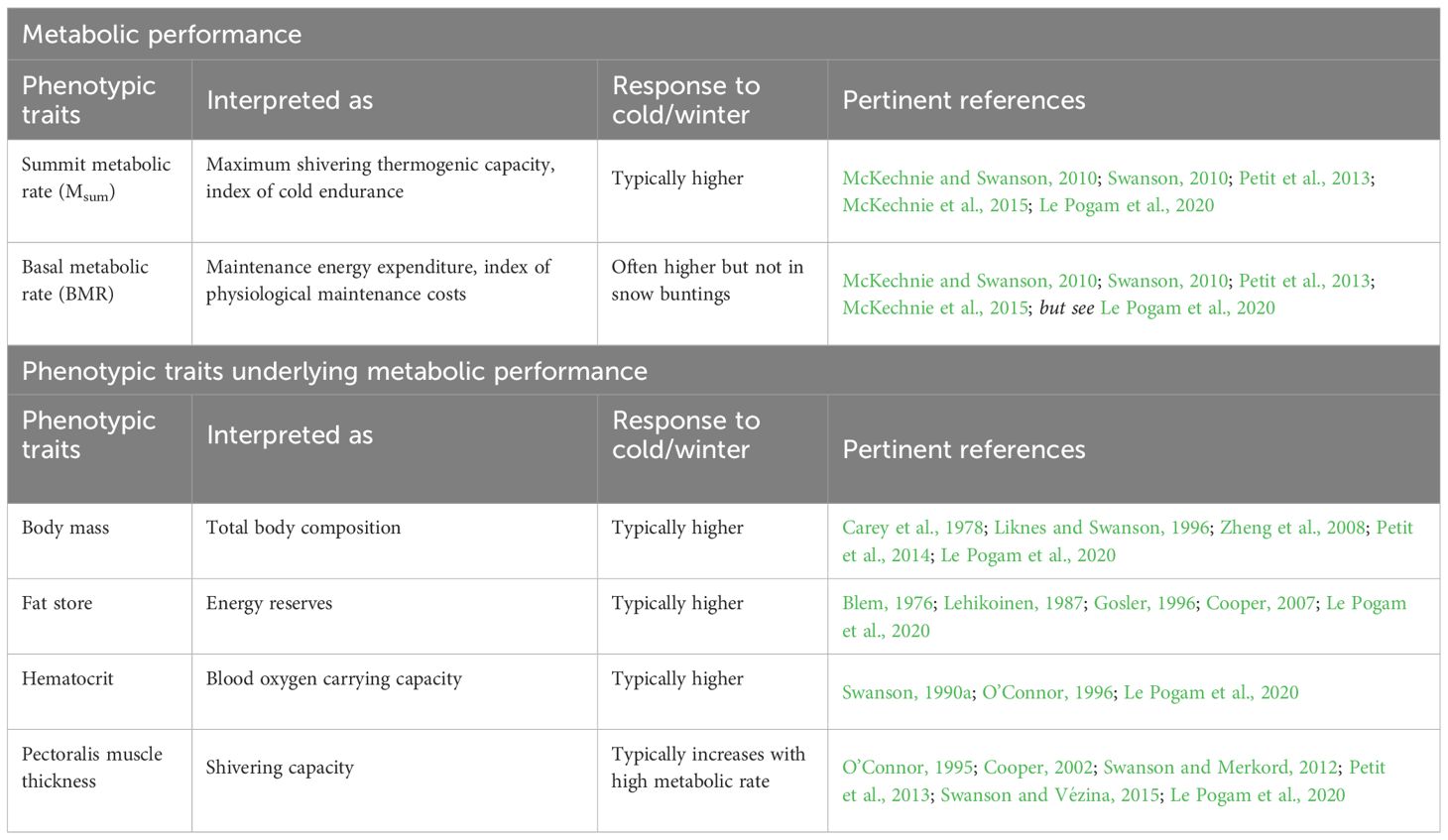
Table 1 List of phenotypic traits measured in this study and their responses to cold and winter in passerine birds.
2 Materials and methods
2.1 Study species
Snow buntings are an Arctic-breeding, migratory passerine known for their ability to endure cold environments (Scholander et al., 1950a; Le Pogam et al., 2020). In the spring, birds migrate through cold winter landscapes (McKinnon et al., 2016; Snell et al., 2018), with males arriving on their breeding grounds to secure territories (up to 83.6°N) up to a month before females (March–April; McKinnon et al., 2016; Snell et al., 2018). Ambient arrival conditions can be comparable or worse than those experienced at the peak of winter, with extensive snow cover and air temperatures (Ta) reaching –30°C (Meltofte, 1983). Buntings are known to arrive on the breeding grounds with winter level cold endurance (Le Pogam et al., 2021a) and can maintain winter-type behaviour for several weeks before dispersing to defend breeding territories (Tinbergen, 1939; Meltofte, 1983).
2.2 Study sites, capture and measurements protocol
Snow buntings were studied in the Arctic during the springs of 2016 to 2019 at Alert, Nunavut, Canada (82°29’58”N, 62°28’5”W). For this study, we distinguished three life-history stages (LH-stages): (1) the transition stage between arrival and dispersal onto breeding territories (hereafter pre-breeding, n = 266 birds), (2) the stage during which males sing and display to defend territories (hereafter territorial, n = 66 birds), and (3) the period during which males and females were paired (hereafter breeding). This last stage includes pair formation (n = 11), nest building (n = 5), egg laying (n = 3), incubation (n = 6), and nestling provisioning (n = 21). Sample sizes for each sub-stage were too small to consider them separately in analyses, and as such they were combined into one breeding stage category (n = 46).
At the pre-breeding stage, birds were caught with walk-in or potter traps (Third Wheel, Devon, England) baited with commercial seed-mix (crushed corn, wheat, sorghum, white millet, red millet and black sunflower, Armstrong, Hagersville, ON, Canada). During the territorial and breeding stages, individuals were attracted to a double potter trap using song playback and a live male bunting as a decoy in one side of the trap. Birds were then captured on the other side of the trap or with spring traps (TWB45 Moudry, Říčany, Czech Republic) set close by.
Immediately after capture, a blood sample (<1% of Mb) was taken from the brachial vein. Blood samples were temporarily kept in cold storage and later centrifuged for 10 minutes at 8,000 RPM to obtain data on hematocrit (i.e., packed red blood cell volume). We then weighed (±0.01g) and sexed birds according to the methods described in Smith (1992). We banded birds with a USGS numbered metal band as well as a unique combination of three darvic color bands to allow for identification from a distance. Morphometric measurements, namely length of head plus beak, tarsus, tail and right wing, were taken to calculate a “structural body size index” (see below). The size of fat stores was also estimated visually using a standard fat score index (from 0 = no visible fat in furculum area to 6 = fat overlapping pectoralis muscles, Love et al., 2012). The birds were then transferred to a field laboratory (less than 6km distance from capture site, transport time < 20 minutes) where we estimated pectoralis muscle thickness non-invasively by ultrasonography (Dietz et al., 1999; Wuenschel et al., 2006; Le Pogam et al., 2020) using a LOGIQe ultrasound scanner fitted with a linear probe (12MHz, GE Healthcare, Wauwatosa, WI, USA). Since the supracoracoideus muscle is very thin at the measured location, muscle thickness values essentially reflect thickness of the pectoralis muscle. However, because ultrasound probe positioning can vary muscle thickness values, we also measured the height of the keel (see Le Pogam et al., 2020). Birds were then kept in cages (76cm W × 46cm D × 45cm H) with ad libitum water and seed (same mix as for captures) until metabolic performance measurements were complete (see below). In total, between 2016 and 2019, 311 males and 67 females were caught and banded during the breeding season (see Supplementary Table 1 for specific sample sizes).
2.3 Metabolic performance
For metabolic performance measurements, we used the set-up and protocol described in detail by Le Pogam et al. (2020, 2021b, 2021a), except that the oxygen analyzers used at Alert were two Sable Systems Foxboxes (Sable Systems, Las Vegas, NV, USA). Key points specific to this study are presented as follows.
Summit metabolic rate (Msum) was measured on a maximum of two birds simultaneously, allowing up to two trials per day. Depending on the time of capture, measurements began between 08h50 and 22h45 (average: 14h45 ± 2.89h; duration: 1h32 ± 0.72h). Measurements took place at least one hour after ultrasound measurements. Birds were placed inside stainless steel metabolic chambers (effective volume 1.5L) and exposed to dry, CO2-free air for 10 minutes at −18°C (or −9°C during the breeding stage) (flow rate of 1,200mL.min−1), before switching to a helox gas mixture (21% oxygen, 79% helium, Rosenmann and Morrison, 1974). The chamber temperature was then lowered by 3°C every 20 minutes until birds became hypothermic (decline of for several minutes and body temperature ≤ 37°C, Swanson and Liknes, 2006) or reached the end of the preprogrammed trial. Six measurements out of 85 (7%) involved birds that were not hypothermic by the end of the trial. However, since hypothermia is not a prerequisite to confirm Msum (Dutenhoffer and Swanson, 1996), we opted to include these individuals in final analyses. Removing them had no influence on results.
We measured basal metabolic rates (BMR) overnight on a maximum of four birds simultaneously. The BMR trials began between 18h25 and 1h26 (average start time: 19h38 ± 5.07h). We insured a minimum 1h of rest after the Msum measurements. Using the same metabolic chambers as for Msum, birds were exposed to 25°C, a temperature within the snow bunting thermoneutral zone (Scholander et al., 1950b) and received dry CO2-free air (flow rate of 650 mL.min−1) for the duration of trials (12h49 ± 5.9h on average). Birds were weighed ( ± 0.01 g) before and after measurements, and average Mb was used in BMR analyses. We used a sampling frequency of 5 secs for Msum and 20 secs for BMR. Both Msum and BMR were calculated from the highest and lowest averaged 10 min trace of , respectively, using equation 10.1 from Lighton (2019), using the instantaneous measurement technique (Bartholomew et al., 1981) for Msum. The duration of BMR trials ensured that birds were post-absorptive at the time of BMR measurement. We estimated energy expenditure for all metabolic measurements using a constant equivalent of 19.8kJ L−1O2 and converted units to Watts (Gessaman and Nagy, 1988).
2.4 Weather data
Weather data were obtained from the Environment and Climate Change Canada Alert weather station (i.e., our study site). More specifically, we extracted daily data for Ta mean and produced 7-day averages that were then used in statistical analyses.
2.5 Statistical analysis
Analyses proceeded in two steps. First, we used general linear models with Mb, fat score, hematocrit, pectoralis muscle thickness, Msum and BMR as separate response variables. All models included year, LH-stage (i.e., pre-breeding, territorial and breeding), mean ambient temperature (hereafter Ta) and their interactions as predictor variables. We chose to use 7-day mean temperature (6 days prior to capture + day of capture) because earlier reports showed that metabolic performance responds to relatively short term variation in temperature (short and medium term variation as presented by Swanson and Olmstead, 1999). We also added time of day at capture (for Mb and fat score), structural size (for Mb), keel height (for pectoralis muscle thickness) and Mb and length of captivity (for Msum and BMR) as covariates in models. Since several structural size parameters were collected for the same individual, the estimate of “structural body size” was based on the first component obtained from a principal component analysis combining variation in the lengths of head plus beak, wing and tail (Rising and Somers, 1989). Since females do not have a “territorial” stage (only males defend territories; Tinbergen, 1939), the variable “sex” was not include in these models.
In the second step, we further analyzed how pectoralis muscle thickness, hematocrit and mass-independent Msum varied with Ta in interaction with LH-stage and/or year. These variables were the only ones where significant interactions were found (see Results). Linear regression analyses were therefore performed per year and/or per LH-stage. We used piecewise linear regression models to determine whether response variables declined at a given threshold Ta. In this set of analyses, we used the residuals of Msum corrected for body mass (hereafter residual Msum) and residuals of pectoralis muscle thickness corrected for keel height (hereafter residual pectoralis muscle thickness). Visual inspection of residuals confirmed assumptions of normality and homogeneity for all models. All analyses were conducted using JMP pro (14.0.0) and data are presented as mean ± standard error of the mean (s.e.m.). Effects were considered significant and retained in models when P< 0.05.
3 Results
3.1 Weather conditions
At Alert, daily mean Ta exceeded 0°C at the earliest on June 2 in 2019 and at the latest on June 11 in 2018 (Figure 2). The years 2016 and 2019 had the fastest temperature increases (Figure 2, Table 2). However, there was more snow on the ground and rapid snow melt occurred up to 10 days later in 2017 than in other years (Figure 2).
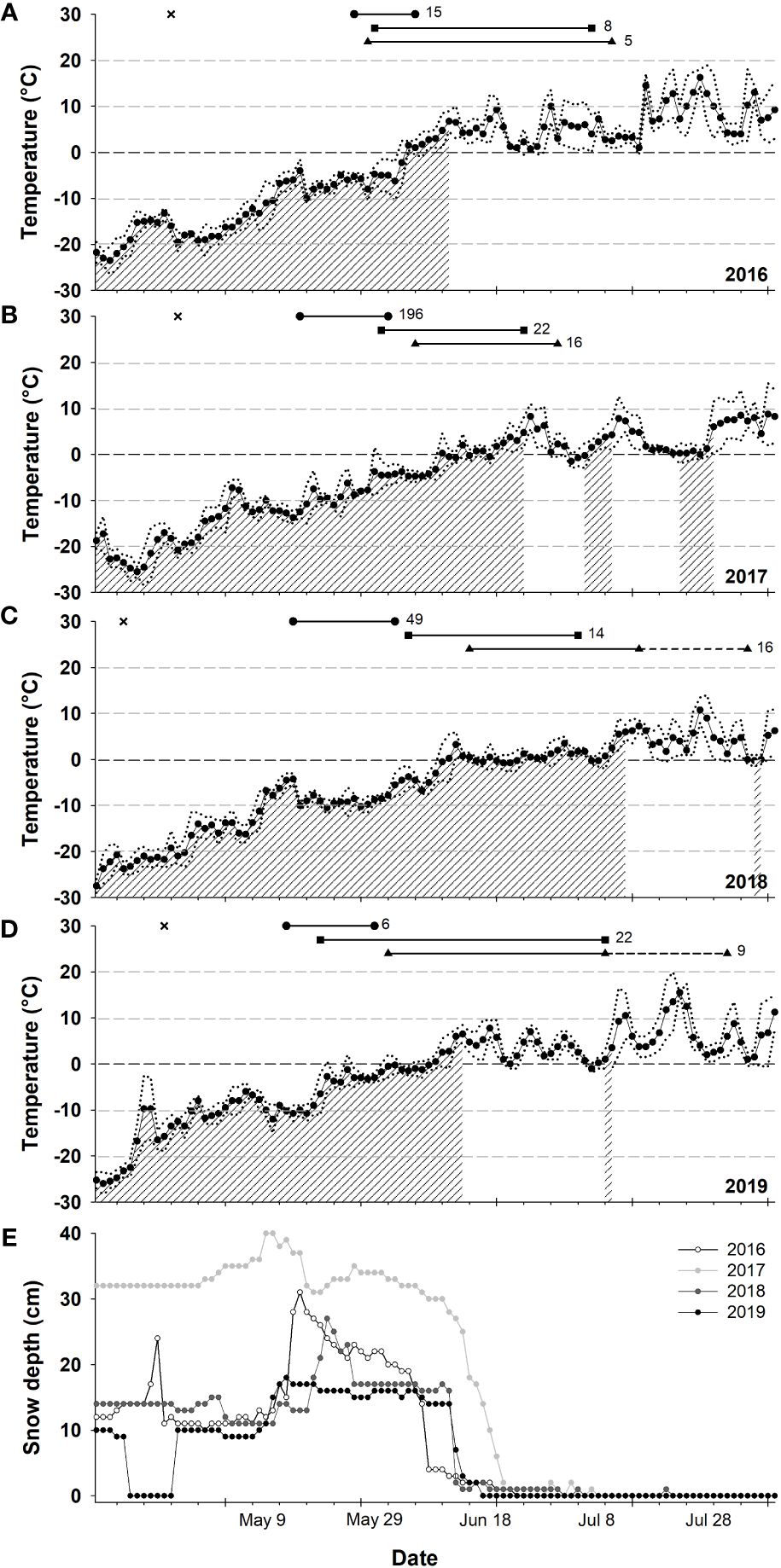
Figure 2 Ambient air temperatures (A–D) and snow depth (E), at Alert, Nunavut, Canada during spring (2016 to 2019). Temperature data show mean daily values (dots) as well as the recorded range of daily minimum and maximum values (doted lines). The hatched period corresponds to the days when the average temperature over 7 days was equal or lower than 1.9°C, the threshold temperature for Msum (see main text). The life history (LH) stages of snow buntings used in this study are represented as lines above each graph (A–D) with the top line being pre-breeding, the middle establishment of territories, and the bottom breeding. The dotted line portion in 2018 and 2019 corresponds to the nestling provisioning stage. The X symbol represent the dates of first observation of birds on the study site. Other symbols represent the first and last capture of prebreeding (•), territorial (▪) and breeding (▴) individuals. Numbers at the right of each line represent sample sizes.
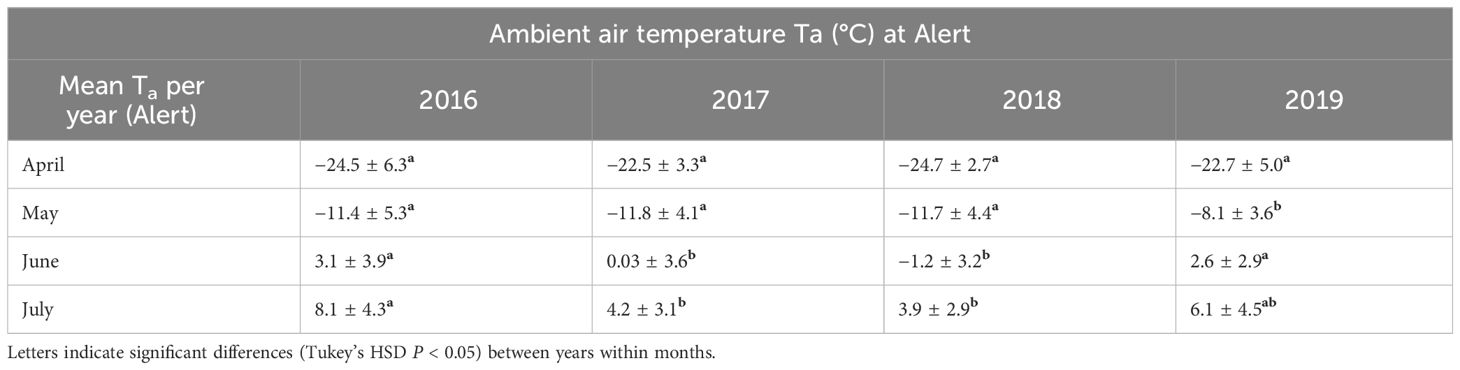
Table 2 Average monthly ambient air temperatures during the breeding season recorded at Alert (NU) between 2016 and 2019.
3.2 Effects of LH-stage and Ta on metabolic performance and associated traits
Controlling for the effect of time at capture and body size, Mb was significantly affected by LH-stage, but not by Ta or year (Table 3). Mb was 14.4% higher during pre-breeding than during territorial defense (Tukey’s HSD P < 0.0001) and Mb at that latter stage did not differ from that measured during breeding (pre-breeding vs breeding: Tukey’s HSD P < 0.0001, Figure 3A). Fat score followed a similar pattern (Table 3), with birds carrying less fat during territorial defense (pre-breeding vs territorial: Tukey’s HSD P < 0.0001) and during breeding (pre-breeding vs breeding: Tukey’s HSD P < 0.005) than at pre-breeding (Figure 3B).
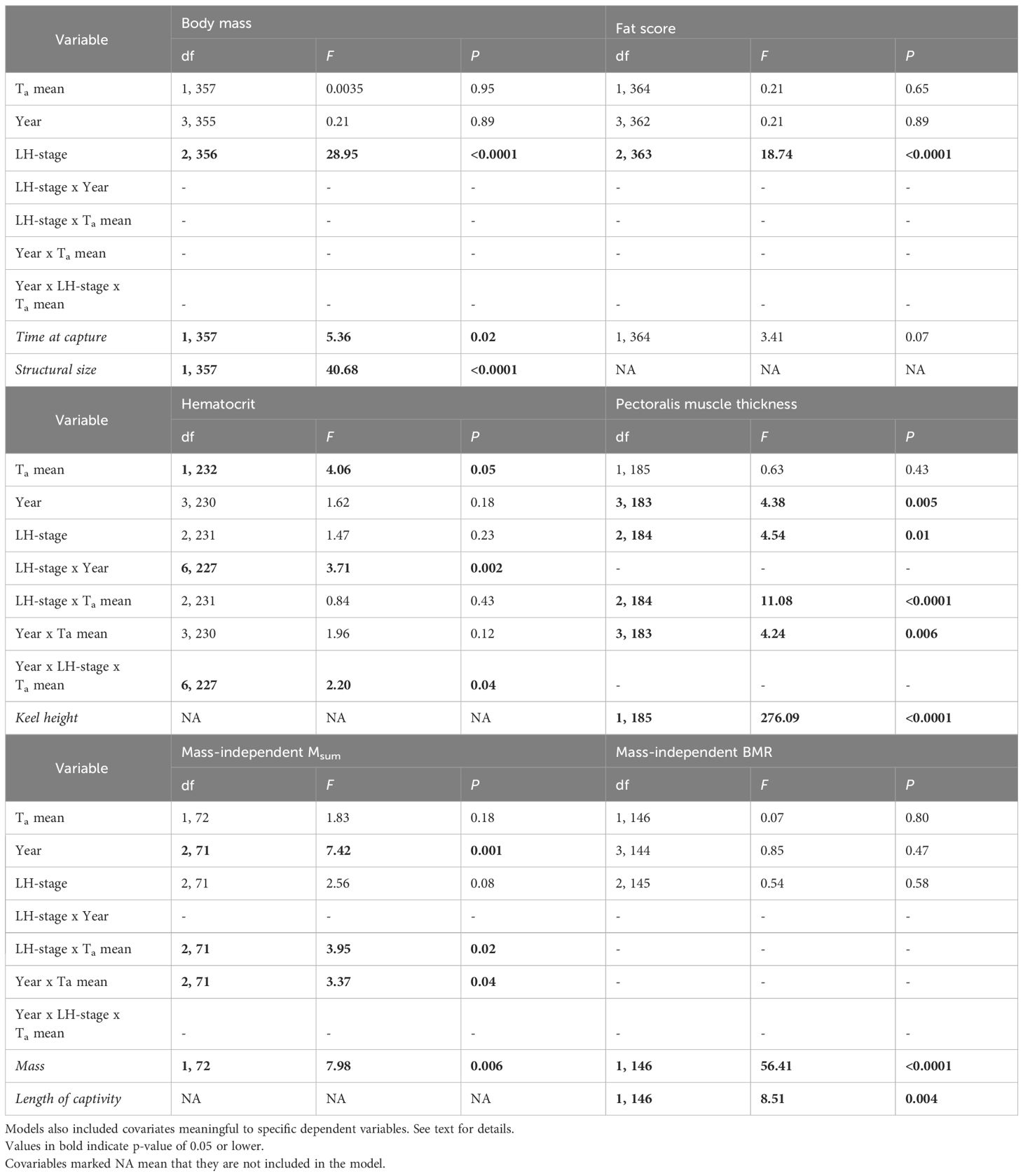
Table 3 Linear effects models comparing phenotypic traits among life-history stages in snow buntings breeding at Alert, Nunavut, Canada.
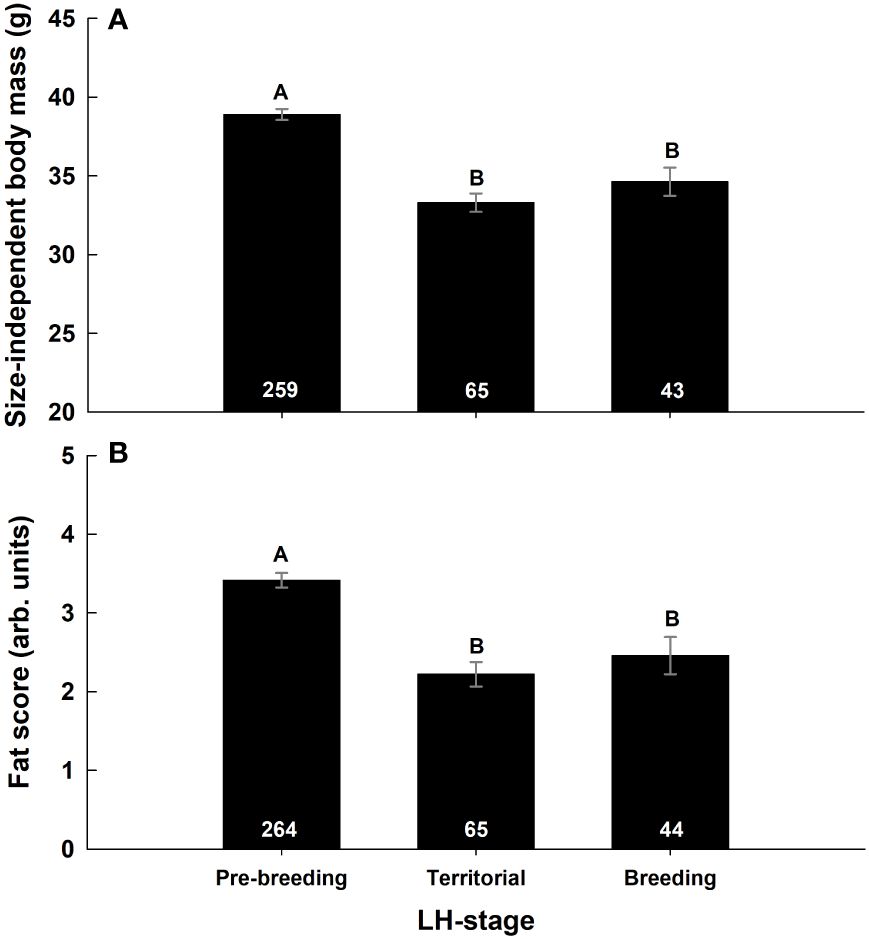
Figure 3 The effect of life history (LH) stage on size-independent body mass (A) and fat score (B) in snow buntings breeding at Alert, Nunavut, Canada. Letters indicate significant differences (Tukey’s HSD P < 0.05). Numbers within bars indicate sample size for each group. Values presented as mean ± SEM.
The influence of temperature on hematocrit was dependent on both LH-stage and year (see interaction terms in Table 3). Regressions performed within LH-stages showed that hematocrit decreased as Ta increased, but only during breeding (Figure 4). However, piecewise linear regression combining all data showed no inflection point for hematocrit.
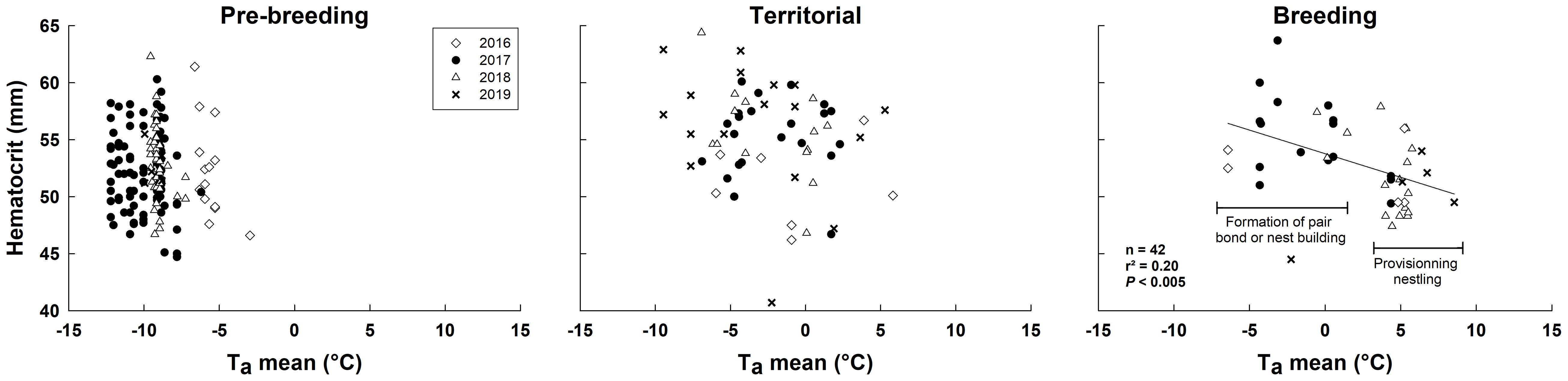
Figure 4 Relationship between hematocrit and ambient air temperature in relation to life history stages in snow buntings breeding at Alert, Nunavut, Canada.
Pectoralis muscle thickness varied among both years and LH-stages (Table 3). However, these effects occurred in interaction with Ta (see interaction terms in Table 3). Regression analyses per LH-stages revealed that residual pectoralis muscle thickness decreased with warming Ta only during breeding (Figure 5). Regressions per year showed a significant negative relationship between residual pectoralis muscle thickness and Ta, but only in 2017 (Figure 5). Piecewise regression combining all data revealed an inflection point at 0.1 ± 1.6°C (Figure 6A). Above that temperature, residual pectoralis muscle thickness declined with increasing Ta (Figure 6A).
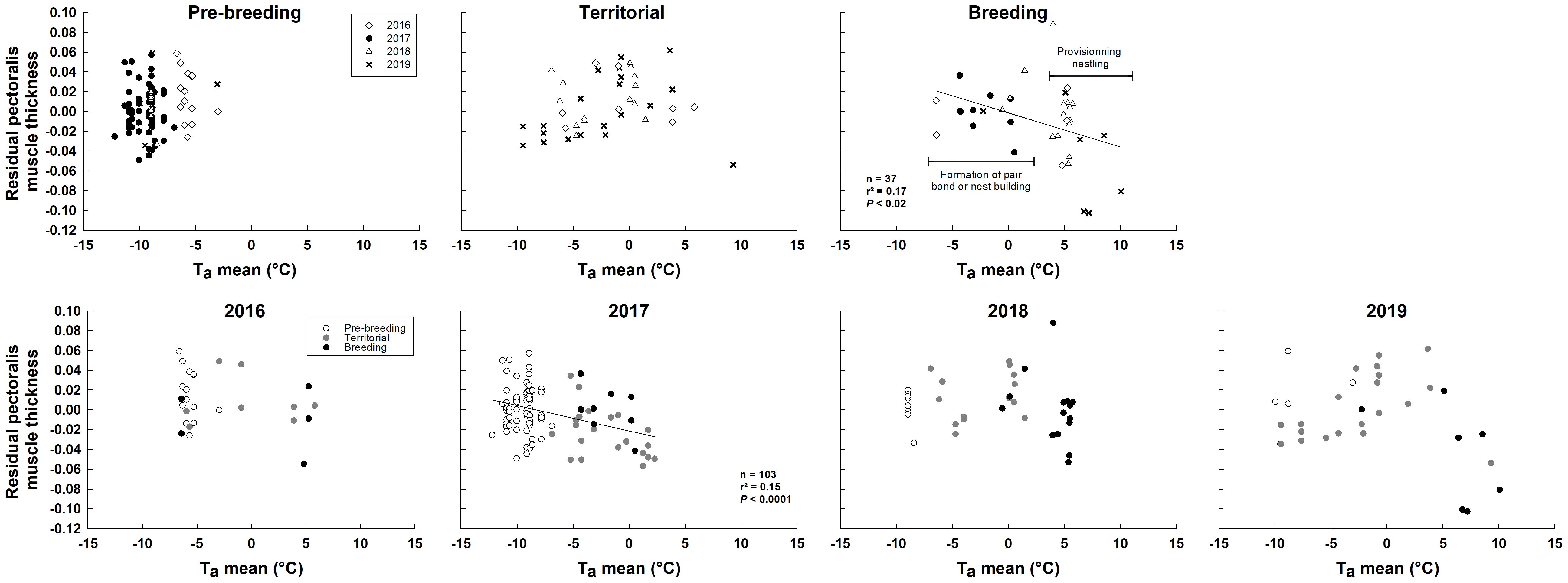
Figure 5 Relationships between residuals of pectoralis muscle thickness (pectoralis muscle thickness corrected for probe positioning) and ambient air temperature in relation to life-history stages and year in snow buntings at Alert, Nunavut, Canada.
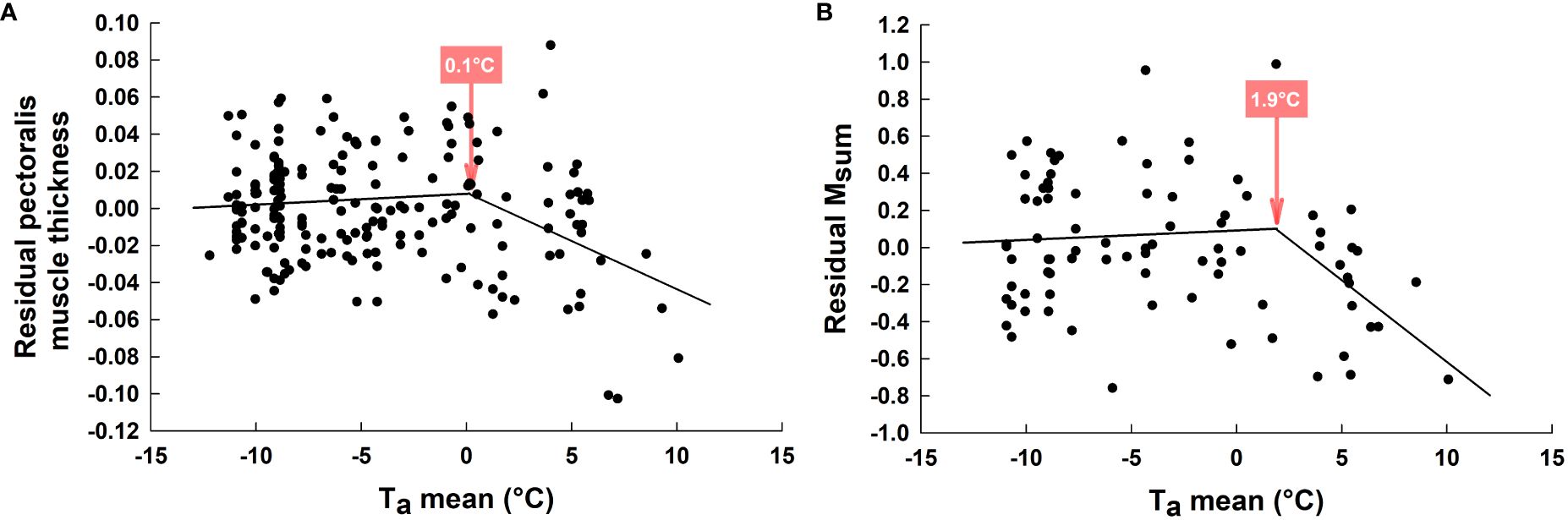
Figure 6 Relationship between residuals of muscle thickness (pectoralis muscle thickness corrected for probe positioning) and ambient temperature (A) and between residuals of Msum (Msum corrected for body mass) and ambient air temperature (B) in snow buntings at Alert, Nunavut, Canada. Temperature values and arrows show the inflexion points for these two parameters.
Summit metabolic rate, whether considered whole (not shown) or corrected for Mb, differed among years and LH-stages, but both these effects depended on Ta (interaction terms in Table 3). As with hematocrit and pectoralis muscle thickness, regressions within LH-stages showed a negative linear relationship between residual Msum and Ta only during breeding (Figure 7). Intra-annual regression analyses also showed a negative relationship between residual Msum and Ta, but only in 2019 (Figure 7). Piecewise regression performed on all data highlighted an inflection point at 1.9 ± 2.6°C (Figure 6B). Above that temperature, residual Msum declined with warming temperature (Figure 6B).
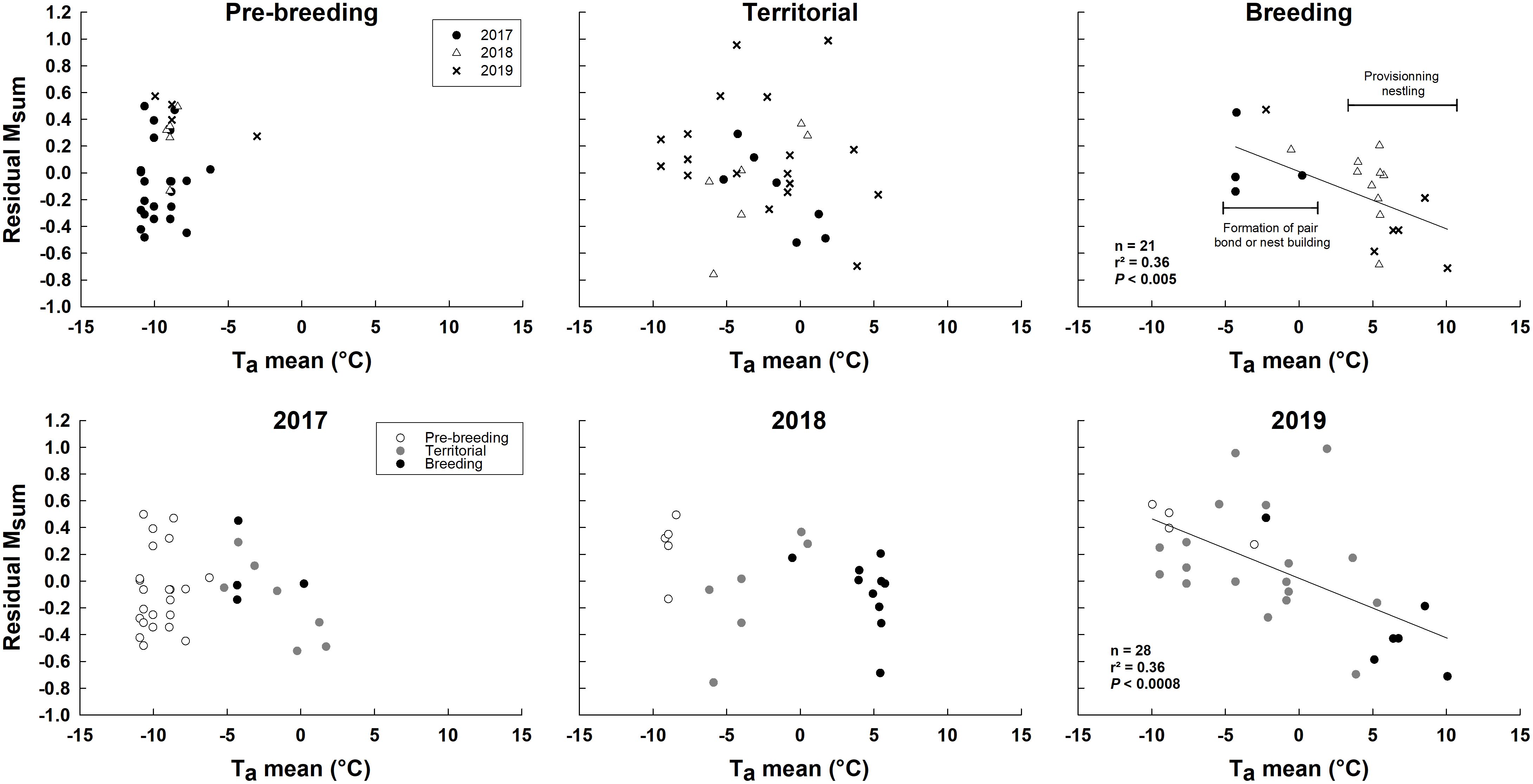
Figure 7 Relationship between residuals of Msum (Msum corrected for body mass) and ambient air temperature according to life-history stages and year in snow buntings at Alert, Nunavut, Canada.
Whole basal metabolic rate varied with LH-stage (F2,146 = 21.7, P < 0.001, not shown), but this effect was driven by the loss of Mb among stages (Figure 3). Considering the significant influence of Mb (in addition to that of length of captivity), we found no significant influence of year, Ta or LH-stage on BMR (Table 3). Maintenance costs remained constant at 0.56 ± 0.01W from spring to end of summer.
4 Discussion
The objective of this study was to examine whether metabolic performance and associated traits in snow buntings remained at post-arrival level (i.e., winter level; Le Pogam et al., 2021a) across three Life History (LH) stages on their breeding grounds, or whether performance declined in response to temperature and/or breeding stages. Overall, although all traits did not show the same pattern, our results suggest that in snow buntings, thermogenic capacity declines during active breeding, but only if ambient temperatures rise above freezing.
4.1 Body mass and fat storage decline as birds begin to breed
After considering the effects of structural body size and time at capture, both body mass and fat scores were only affected by life history stages in snow buntings. Indeed, mass and fat scores both declined from pre-breeding to the territorial stage and then remained low thereafter during breeding. As this finding was independent from the effects of temperature and year, these results support the idea that birds could not maintain mass and energy stores at winter levels while breeding (Hypothesis 3, Figure 1).
A decline in body mass and fat stores during the establishment of breeding territories is common in passerines (e.g., Ramenofsky and Wingfield, 2006; Krause et al., 2016b). For species breeding at high latitudes, this phenomenon generally coincides with the dispersal of post-arrival flocks and the secretion of reproductive hormones (see Ramenofsky and Wingfield, 2006 for a review). Snow buntings also become very active as they initiate breeding activities. For example, the period of territorial defense is characterized by high rates of singing in males, courtship displays where the birds climb in altitude and sing while gliding down as well as physical fights between competing males (Romero et al., 1998; Guindre-Parker et al., 2013). Once breeding pairs have formed, males and females are highly mobile during nest building and both adults maintain high rates of nestling provisioning after the eggs have hatched (Tinbergen, 1939; Lyon et al., 1987), with daily resting periods typically being limited to 3–5h per day (Hussell, 1972). In fact, nestling growth rates in snow buntings are among the highest in passerines of that body size (i.e., 11.5–13% of adult body weight gain per day; Hussell, 1972), with only 8–10 days from hatching to fledging, with nestlings unable to fly at fledging (Hussell, 1972). With the high energy cost of flapping flight in birds (Hedenstrom, 1993) and the busy schedule of breeding snow buntings, it may simply not be possible for these birds to maintain body mass and fat stores at winter levels, even if thermal conditions can be highly unpredictable in the Arctic (Table 2). Alternatively, but not exclusively, maintaining a lower body mass at these stages could also be adaptive as it reduces flight costs during a period of high energy demand (Merkle and Barclay, 1996; Nagy et al., 2007; Boyle et al., 2012).
While the variation in body mass and fat stores reported here is consistent with that of other free-living species, it contrasts with previous observations in captive snow buntings (Navarro and Gutiérrez, 1995; Le Pogam et al., 2021b). Both Navarro and Gutiérrez (1995) and Le Pogam et al. (2021b) observed that when maintained at their wintering latitude throughout summer, this species maintains body mass and fat stores above their winter average until at least the end of June. Similar observations have also been made in other captive migrant species (Eyster, 1954; Gwinner and Czeschlik, 1978; Schwabl and Farner, 1989), which have led others to hypothesize that different endocrine responses, lack of visual cues or reproductive opportunities in captivity (Gwinner and Czeschlik, 1978; Schwabl and Farner, 1989) could trigger this effect. Although this is possible, captive studies have also shown strong endogenous regulation of seasonal cycles in migrant species (Ramenofsky and Wingfield, 2006; Vézina et al., 2011; Karagicheva et al., 2016). It could therefore also be that snow buntings are pre-programmed, via their endogenous circannual cycle, to maintain high energy intake rates during breeding as this occurs at a time of very high daily energy expenditure (Vézina et al., 2011; Karagicheva et al., 2016). In free-living breeding conditions, this would be enough to balance energy budgets and maintain stable (but lower) body mass, while in captivity maintaining high intake rates in birds not able to breed could lead to fat stores and body mass comparable to or above wintering levels.
4.2 Maintenance costs decline with breeding, but cold endurance traits respond to warming temperatures
Basal metabolic rate is interpreted as a measure of physiological maintenance costs and is thought to reflect changes in the activity and amount of tissues forming an animal (Piersma and Lindström, 1997; Piersma et al., 2004; Swanson et al., 2017a). We found that total maintenance costs declined as birds lost body mass with territorial defense and breeding, but that tissue metabolic intensity (mass corrected BMR) remained constant. We also found that these patterns were independent of variation in ambient temperature. Since territorial defense and nestling provisioning are energetically demanding activities for snow buntings, lower overall maintenance costs could be beneficial to balance energy budgets at that time (Swanson et al., 2017b). This finding contrasts with an earlier report of increasing BMR in shorebirds breeding at Alert (Vézina et al., 2012), although a large portion of this variation was attributed to migration recovery in recently arrived birds. Female birds have also been shown to have higher BMR during egg development (Chappell et al., 1999; Salvante et al., 2010; Vézina and Salvante, 2010). However, our data did not include measurements at that stage.
Our data on hematocrit, pectoralis muscle thickness and Msum showed comparable patterns regarding life-history stages and ambient temperature. Values for all three variables remained relatively stable during the pre-breeding and territorial stages, but declined with warming temperatures during breeding. In other words, warming temperature led to a loss of cold endurance as predicted under Hypothesis 2, but only when the birds were actively breeding, also providing support for Hypothesis 3. In fact, although sample size limitations prevented us from testing for within-breeding-stage effects (e.g., nest building, incubation and provisioning) Figures 4–7 indicate that the temperature-related decline in these traits appears to occur during the highly active period of nestling provisioning. However, this stage also coincides with temperatures above 0°C in all years. Results further suggest that in some years temperature may also act on muscle size (in 2017, Figure 5) and Msum (in 2019, Figure 7) independently from life-history stage. Although the overlap in time makes the independent influence of temperature and breeding stages difficult to tease apart, several lines of evidence, together with previous studies, lead us to posit that variation in ambient temperature, rather than the direct effects of breeding, is the main driver of the observed decline in cold endurance in breeding snow buntings.
We observed a decline in pectoralis muscle thickness as temperature increased during breeding, with the lowest values recorded in birds provisioning nestlings. It is possible that the high locomotor activity required during foraging and provisioning led to muscle loss, as is often observed in other species during migration (e.g., Battley et al., 2000; Bauchinger et al., 2005), and to a correlated decline in Msum since shivering heat production covaries with muscle size in buntings (Dubois et al., 2016; Le Pogam et al., 2020; Le Pogam et al., 2021b). However, migration-related muscle loss results from unbalanced protein turnover due to a negative energy budget during flights (i.e., no or little replacement for degraded proteins as birds lose mass; Bauchinger and McWilliams, 2010, 2010; but see Gerson and Guglielmo, 2011). In our study, buntings did not show signs of negative energy balance once territorial defense had been initiated. In fact, both body mass and fat scores remained stable between territorial and breeding stages (Figure 3). Furthermore, experimental studies have shown that exercise in birds leads to an increase in pectoralis muscle mass and not a decrease as we observed here (Zhang et al., 2015, 2018). Additionally, seasonal declines in muscle mass and Msum have been observed at the end of winter as temperatures warm up in non-migrant species coming out of cold wintering conditions (e.g., Petit et al., 2014). Such a pattern has also been observed in outdoor captive snow buntings exposed to summer temperatures on their wintering grounds (Le Pogam et al., 2021b). Indeed, although Le Pogam et al. (2021b) did not test for an influence of temperature on muscle thickness per se, they nonetheless reported muscle sizes comparable to winter in March and April (corresponding to migration), and a relatively slow, but constant, decline through the rest of summer that was paralleled by a reduction in Msum. Therefore, as these captive birds could not breed, we believe that changes in muscle size in breeding buntings, combined with the parallel changes in Msum, are more likely to result from rapidly improving thermal conditions than from a consequence of the birds’ breeding activity.
We also found a negative relationship between hematocrit level and ambient temperature, but only during breeding when thermal conditions had improved. Breeding related changes in avian hematocrit have been reported before (Morton, 1994; Fair et al., 2007; Krause et al., 2016a). For example, female birds typically experience hematocrit declines prior to egg-laying due to an increase in plasma estrogen inhibiting erythrocyte synthesis (Wagner et al., 2008; Williams et al., 2012), but this effect disappears during active nestling provisioning, the period matching the decline observed in this study (Morton, 1994; Williams et al., 2004; Krause et al., 2016a and see Fair et al., 2007 for a review). In males, however, hematocrit is positively related to testosterone (Buttemer and Astheimer, 2000) and testosterone does decline when males begin to provision nestlings in buntings (Romero et al., 1998). Nevertheless, although hematocrit typically increases with exercise (Bairlein and Totzke, 1992; Morton, 1994; Piersma et al., 1996), it has consistently been found to correlate negatively with ambient temperature in birds (DeGraw et al., 1979; Rehder and Bird, 1983; Fair et al., 2007). This marker of oxygen carrying capacity is also directly and positively correlated with thermogenic capacity (Swanson, 1990a; Petit and Vézina, 2014a), including in snow buntings (Le Pogam et al., 2020; Le Pogam et al., 2021b). It is therefore likely that the hematocrit reduction observed in breeding buntings results from increasingly favorable thermal conditions. As blood oxygen carrying capacity and cardiac function appears to support maximal shivering heat production (Petit et al., 2014; Vézina et al., 2017) this effect probably underlies part of the observed temperature dependent changes in Msum.
4.3 Snow buntings may be paying cumulative physiological costs in late spring years
Our observations suggest that variation in oxygen carrying capacity, muscle size and Msum may be more strongly influenced by warming summer temperatures than by breeding activities in snow buntings. These findings support results from several studies on avian cold endurance published since the 1990s showing the proximate influence of temperature on thermogenic capacity (Swanson and Olmstead, 1999; McKechnie and Swanson, 2010; Swanson, 2010 for reviews). In fact, combining data across years and breeding stages (Figure 6), we observed that pectoralis muscle thickness began declining once ambient temperatures warmed above 0°C. Thermogenic capacity followed closely and began to decline at temperatures above 2°C (Figure 6). Therefore, our results for traits underlying cold endurance are consistent with the Msum reaction norm reported in other birds (Petit and Vézina, 2014b; Swanson and Vézina, 2015) and support the hypothesis that snow buntings maintain thermogenic capacity after their arrival if temperatures remain below freezing, whether they are actively breeding or not (Hypothesis 2, Figure 1).
This finding of a threshold temperature effect on the maintenance of thermogenic capacity is important because it suggests that snow buntings likely incur cumulative physiological costs in years with a late onset of spring where breeding activities may begin at temperatures well below 0–2°C. The timing of our own data collection confirms this idea (see Figure 2). While nestling provisioning clearly occurs at temperatures above freezing, other important stages such as egg production and incubation may begin (2016, 2019) or occur almost completely (2017, 2018) at temperatures requiring the maintenance of winter levels of thermogenic capacity and cold endurance. Other species, such as the Canada jay (Perisoreus canadensis) are known to initiate breeding at sub-zero temperatures (e.g., −10°C); however, these birds are larger than snow buntings and rely on considerable amounts of cached food to support their energy requirements (Whelan et al., 2016). Experimental studies with birds breeding in the cold have shown reduced rates of egg production, delayed laying and smaller clutches (Salvante et al., 2007), as well as reduced locomotor activity and potential energy reallocation among physiological systems when birds must combine cold acclimation and breeding (Salvante et al., 2010). The extent to which maintaining winter level cold endurance in late Arctic springs may or may not be restrictive in actively breeding snow buntings will therefore require more research.
Data availability statement
The raw data supporting the conclusions of this article will be made available by the authors, without undue reservation.
Ethics statement
The animal study was approved by the animal care committee of the Université du Québec à Rimouski (CPA-61-15-163 and CPA-71-17-194) and was conducted under scientific (SC-48) and banding (10889E) permits from Environment and Climate Change Canada. The study was conducted in accordance with the local legislation and institutional requirements.
Author contributions
ALP: Conceptualization, Data curation, Formal analysis, Investigation, Methodology, Visualization, Writing – original draft, Writing – review & editing. RO: Writing – original draft, Writing – review & editing. OL: Funding acquisition, Resources, Supervision, Writing – original draft, Writing – review & editing. KY: Data curation, Investigation, Writing – review & editing. JD: Data curation, Investigation, Writing – review & editing. LR: Data curation, Investigation, Writing – review & editing. GR: Data curation, Investigation, Writing – review & editing. FR: Data curation, Investigation, Writing – review & editing. DB: Resources, Writing – review & editing. AT: Funding acquisition, Resources, Writing – review & editing. FV: Conceptualization, Funding acquisition, Methodology, Project administration, Resources, Supervision, Validation, Writing – original draft, Writing – review & editing.
Funding
The author(s) declare financial support was received for the research, authorship, and/or publication of this article. This research benefited from a generous donation from the Kenneth M. Molson Foundation. It was also supported by NSERC Discovery grants to FV and OL, Canada Foundation for Innovation (CFI) awards to FV and OL, Canada Research Chair funding to OL, as well as logistical support and funding from the Department of National Defense to FV and DB.
Acknowledgments
We thank François Fournier from Environment and Climate Change Canada for help with logistical support in the initial Alert phase of this project. We thank Jonathan Coudé for technical support and Alain Caron for statistical advice. We thank Chris McRae and Nathan Koutroulides for help with logistics and the personnel from CFS Alert for their support during fieldwork. We are grateful to the field team for their punctual help in 2019: Emilie Desjardins, Sandra Lai, Marie-Pierre Poulin, Marie-Jeanne Rioux and Jacob Caron Carrier. We thanks the reviewers for their constructive comments on the earlier versions of this article. This research is part of the PhD thesis of A. Le Pogam at Université du Québec à Rimouski (Le Pogam, 2021).
Conflict of interest
The authors declare that the research was conducted in the absence of any commercial or financial relationships that could be construed as a potential conflict of interest.
Publisher’s note
All claims expressed in this article are solely those of the authors and do not necessarily represent those of their affiliated organizations, or those of the publisher, the editors and the reviewers. Any product that may be evaluated in this article, or claim that may be made by its manufacturer, is not guaranteed or endorsed by the publisher.
Supplementary material
The Supplementary Material for this article can be found online at: https://www.frontiersin.org/articles/10.3389/fevo.2024.1369761/full#supplementary-material
References
Bairlein F., Totzke U. (1992). New aspects on migratory physiology of trans-Saharan passerine migrants. Ornis. Scandinavica. 23, 244–250. doi: 10.2307/3676645
Barceló G., Love O. P., Vézina F. (2017). Uncoupling basal and summit metabolic rates in white-throated sparrows: Digestive demand drives maintenance costs, but changes in muscle mass are not needed to improve thermogenic capacity. Physiol. Biochem. Zool. 90, 153–165. doi: 10.1086/689290
Bartholomew G. A., Vleck D., Vleck C. M. (1981). Instantaneous measurements of oxygen consumption during pre-flight warm-up and post-flight cooling in sphingid and saturniid moths. J. Exp. Biol. 90, 17–32. doi: 10.1242/jeb.90.1.17
Battley P. F., Piersma T., Dietz M. W., Tang S., Dekinga A., Hulsman K. (2000). Empirical evidence for differential organ loss during trans-oceanic bird flight. Proc. R. Soc. London. B. 267, 191–195. doi: 10.1098/rspb.2000.0986
Bauchinger U., McWilliams S. R. (2010). Carbon turnover in tissues of a passerine bird: Allometry, isotopic clocks, and phenotypic flexibility in organ size. Physiol. Biochem. Zool. 83, 1032–1032. doi: 10.1086/657659
Bauchinger U., Wohlmann A., Biebach H. (2005). Flexible remodeling of organ size during spring migration of the garden warbler (Sylvia borin). Zoology 108, 97–106. doi: 10.1016/j.zool.2005.03.003
Blem C. R. (1976). Patterns of lipid storage and utilization in birds. Am. Zool. 16, 671–684. doi: 10.1093/icb/16.4.671
Boyle W. A., Winkler D. W., Guglielmo C. G. (2012). Rapid loss of fat but not lean mass prior to chick provisioning supports the flight efficiency hypothesis in tree swallows. Funct. Ecol. 26, 895–903. doi: 10.1111/j.1365-2435.2012.01997.x
Buttemer W. A., Astheimer L. B. (2000). Testosterone does not affect basal metabolic rate or blood parasite load in captive male white-plumed honeyeaters Lichenostomus penicillatus. J. Avian Biol. 31, 479–488. doi: 10.1034/j.1600-048X.2000.310407.x
Carey C., Dawson W. R., Maxwell L. C., Faulkner J. A. (1978). Seasonal acclimatization to temperature in cardueline finches II. Changes in body composition and mass in relation to season and acute cold stress. J. Comp. Physiol. 125, 101–113. doi: 10.1007/BF00686746
Chappell M. A., Bech C., Buttemer W. A. (1999). The relationship of central and peripheral organ masses to aerobic performance variation in house sparrows. J. Exp. Biol. 202, 2269–2279. doi: 10.1242/jeb.202.17.2269
Cooper S. J. (2002). Seasonal metabolic acclimatization in mountain chickadees and juniper titmice. Physiol. Biochem. Zool. 75, 386–395. doi: 10.1086/342256
Cooper S. J. (2007). Daily and seasonal variation in body mass and visible fat in mountain chickadees and juniper titmice. Wilson. J. Ornithol. 119, 720–724. doi: 10.1676/06-183.1
DeGraw W. A., Kern M. D., King J. R. (1979). Seasonal changes in the blood composition of captive and free-living white-crowned sparrows. J. Comp. Physiol. B. 129, 151–162. doi: 10.1007/BF00798180
Dietz M. W., Dekinga A., Piersma T., Verhulst S. (1999). Estimating organ size in small migrating shorebirds with ultrasonography: An intercalibration exercise. Physiol. Biochem. Zool. 72, 28–37. doi: 10.1086/316648
Dubois K., Hallot F., Vézina F. (2016). Basal and maximal metabolic rates differ in their response to rapid temperature change among avian species. J. Comp. Physiol. B. 186, 919–935. doi: 10.1007/s00360-016-1001-5
Dutenhoffer M. S., Swanson D. L. (1996). Relationship of basal to summit metabolic rate in passerine birds and the aerobic capacity model for the evolution of endothermy. Physiol. Zool. 69, 1232–1254. doi: 10.1086/physzool.69.5.30164255
Eyster M. (1954). Quantitative measurement of the influence of photoperiod, temperature, and season on the activity of captive songbirds. Ecol. Monogr. 24, 1–28. doi: 10.2307/1943509
Fair J. M., Whitaker S., Pearson B. (2007). Sources of variation in haematocrit in birds. Ibis, 149(3), 535–552. doi: 10.1111/j.1474-919X.2007.00680.x
Gerson A. R., Guglielmo C. G. (2011). Flight at low ambient humidity increases protein catabolism in migratory birds. Science 333, 1434–1436. doi: 10.1126/science.1210449
Gessaman J. A., Nagy K. A. (1988). Energy metabolism: Errors in gas-exchange conversion factors. Physiol. Zool. 61, 507–513. doi: 10.1086/physzool.61.6.30156159
Gosler A. G. (1996). Environmental and social determinants of winter fat storage in the great tit Parus major. J. Anim. Ecol. 65, 1–17. doi: 10.2307/5695
Grabowski M. M., Doyle F. I., Reid D. G., Mossop D., Talarico D. (2013). Do Arctic-nesting birds respond to earlier snowmelt? A multi-species study in north Yukon, Canada. Polar. Biol. 36, 1097–1105. doi: 10.1007/s00300-013-1332-6
Guindre-Parker S., Gilchrist H. G., Baldo S., Love O. P. (2013). Alula size signals male condition and predicts reproductive performance in an Arctic-breeding passerine. J. Avian Biol. 44, 209–215. doi: 10.1111/j.1600-048X.2012.05817.x
Gwinner E., Czeschlik D. (1978). On the significance of spring migratory restlessness in caged birds. Oikos 30, 364–372. doi: 10.2307/3543485
Hahn T. P. (1995). Integration of photoperiodic and food cues to time changes in reproductive physiology by an opportunistic breeder, the red crossbill, Loxia curvirostra (Aves: Carduelinae). J. Exp. Zool. 272, 213–226. doi: 10.1002/jez.1402720306
Hedenstrom A. (1993). Migration by soaring or flapping flight in birds: The relative importance of energy cost and speed. Philos. Trans. R. Soc. B.: Biol. Sci. 342, 353–361. doi: 10.1098/rstb.1993.0164
Høye T. T., Post E., Meltofte H., Schmidt N. M., Forchhammer M. C. (2007). Rapid advancement of spring in the High Arctic. Curr. Biol. 17, 449–451. doi: 10.1016/j.cub.2007.04.047
Hussell D. J. T. (1972). Factors affecting clutch size in Arctic passerines. Ecol. Monogr. 42, 317–364. doi: 10.2307/1942213
Karagicheva J., Rakhimberdiev E., Dekinga A., Brugge M., Koolhaas A., Ten Horn J., et al. (2016). Seasonal time keeping in a long-distance migrating shorebird. J. Biol. Rhythms. 31, 509–521. doi: 10.1177/0748730416655929
Krause J. S., Németh Z., Pérez J. H., Chmura H. E., Ramenofsky M., Wingfield J. C. (2016a). Annual hematocrit profiles in two subspecies of white-crowned sparrow: A migrant and a resident comparison. Physiol. Biochem. Zool. 89, 51–60. doi: 10.1086/684612
Krause J. S., Pérez J. H., Chmura H. E., Sweet S. K., Meddle S. L., Hunt K. E., et al. (2016b). The effect of extreme spring weather on body condition and stress physiology in Lapland longspurs and white-crowned sparrows breeding in the Arctic. Gen. Comp. Endocrinol. 237, 10–18. doi: 10.1016/j.ygcen.2016.07.015
Lehikoinen E. (1987). Seasonality of the daily weight cycle in wintering passerines and its consequences. Ornis. Scandinavica. 18, 216–226. doi: 10.2307/3676769
Le Pogam A. (2021). Ajustements phénotypiques en réponse aux contraintes associées à l’hiver, la migration et la reproduction chez le plectrophane des neiges (Montréal: Université du Québec à Montréal).
Le Pogam A., Love O. P., Régimbald L., Dubois K., Hallot F., Milbergue M., et al. (2020). Wintering snow buntings elevate cold hardiness to extreme levels but show no changes in maintenance costs. Physiol. Biochem. Zool. 93, 417–433. doi: 10.1086/711370
Le Pogam A., O’Connor R. S., Love O. P., Drolet J., Régimbald L., Roy G., et al. (2021a). Snow buntings maintain winter-level cold endurance while migrating to the High Arctic. Front. Ecol. Evol. 9, 724876. doi: 10.3389/fevo.2021.724876
Le Pogam A., O’Connor R. S., Love O. P., Petit M., Régimbald L., Vézina F. (2021b). Coping with the worst of both worlds: Phenotypic adjustments for cold acclimatization benefit northward migration and arrival in the cold in an Arctic-breeding songbird. Funct. Ecol. 35, 1240–1254. doi: 10.1111/1365-2435.13793
Lighton J. R. B. (2019). Measuring Metabolic Rates: A manual for scientists. 2nd ed (New-York: Oxford University Press).
Liknes E. T., Swanson D. L. (1996). Seasonal variation in cold tolerance, basal metabolic rate, and maximal capacity for thermogenesis in white-breasted nuthatches Sitta carolinensis and downy woodpeckers Picoides pubescens, two unrelated arboreal temperate residents. J. Avian Biol. 27, 279–288. doi: 10.2307/3677259
Love O. P., Macdonald C. A., Mckinnon E. A. (2012). Canadian snow bunting banding network protocol. doi: 10.6084/m9.figshare.1588581
Lyon B. E., Montgomerie R. D., Hamilton L. D. (1987). Male parental care and monogamy in snow buntings. Behav. Ecol. Sociobiol. 20, 377–382. doi: 10.1007/BF00300684
McKechnie A. E., Noakes M. J., Smit B. (2015). Global patterns of seasonal acclimatization in avian resting metabolic rates. J. Ornithol. 156, 367–376. doi: 10.1007/s10336-015-1186-5
McKechnie A. E., Swanson D. L. (2010). Variability in basal metabolic rate of a long-distance migrant shorebird (red knot, Calidris canutus) reflects shifts in organ sizes. Physiol. Zool. 56, 741–758. doi: 10.1038/35131
McKinnon E. A., Macdonald C. A., Gilchrist H. G., Love O. P. (2016). Spring and fall migration phenology of an Arctic-breeding passerine. J. Ornithol. 157, 681–693. doi: 10.1007/s10336-016-1333-7
Meltofte H. (1983). Arrival and pre-nesting period of the snow bunting Plectrophenax nivalis in East Greenland. Polar. Res. 1, 185–198. doi: 10.1111/j.1751-8369.1983.tb00702.x
Merkle M. S., Barclay R. M. R. (1996). Body mass variation in breeding mountain bluebirds sialia currucoides: Evidence of stress or adaptation for flight? J. Anim. Ecol. 65, 401–413. doi: 10.2307/5776
Milbergue M. S., Blier P. U., Vézina F. (2018). Large muscles are beneficial but not required for improving thermogenic capacity in small birds. Sci. Rep. 8, 14009–14009. doi: 10.1038/s41598-018-32041-w
Moe B., Stempniewicz L., Jakubas D., Angelier F., Chastel O., Dinessen F., et al. (2009). Climate change and phenological responses of two seabird species breeding in the high-Arctic. Mar. Ecol. Prog. Ser. 393, 235–246. doi: 10.3354/meps08222
Morrison R. I. G. (2006). Body transformations, condition, and survival in red knots Calidris canutus travelling to breed at Alert, Ellesmere Island, Canada. Ardea 94, 607–618.
Morrison R. I. G., Davidson N. C., Piersma T. (2005). Transformations at high latitudes: Why do red knots bring body stores to the breeding grounds? Condor. 107, 449–457. doi: 10.1093/condor/107.2.449
Morrison R. I. G., Davidson N. C., Wilson J. R. (2007). Survival of the fattest: Body stores on migration and survival in red knots Calidris canutus islandica. J. Avian Biol. 38, 479–487. doi: 10.1111/j.2007.0908-8857.03934.x
Morton M. L. (1994). Hematocrits in montane sparrows in relation to reproductive schedule. Condor. 96, 119–126. doi: 10.2307/1369069
Nagy L. R., Stanculescu D., Holmes R. T. (2007). Mass loss by breeding female songbirds: Food supplementation supports energetic stress hypothesis in black-throated blue warblers. Condor 109, 304–311. doi: 10.1650/0010-5422(2007)109[304:MLBBFS]2.0.CO;2
Navarro I., Gutiérrez J. (1995). Fat reserves in snow buntings. Biochem. Mol. Biol. Fishes. 54, 1051–1063. doi: 10.1016/S1873-0140(06)80020-2
O’Connor T. P. (1995). Metabolic characteristics and body composition in house finches: Effects of seasonal acclimitatization. J. Comp. Physiol. B. 165, 298–305. doi: 10.1007/BF00367313
O’Connor T. P. (1996). Geographic variation in metabolic seasonal acclimatization in house finches. Condor. 98, 371–381. doi: 10.2307/1369155
Petit M., Lewden A., Vézina F. (2013). Intra-seasonal flexibility in avian metabolic performance highlights the uncoupling of basal metabolic rate and thermogenic capacity. PloS One 8, e68292–e68292. doi: 10.1371/journal.pone.0068292
Petit M., Lewden A., Vézina F. (2014). How does flexibility in body composition relate to seasonal changes in metabolic performance in a small passerine wintering at Northern latitude? Physiol. Biochem. Zool. 87, 539–549. doi: 10.1086/676669
Petit M., Vézina F. (2014a). Phenotype manipulations confirm the role of pectoral muscles and haematocrit in avian maximal thermogenic capacity. J. Exp. Biol. 217, 824–830. doi: 10.1242/jeb.095703
Petit M., Vézina F. (2014b). Reaction norms in natural conditions: How does metabolic performance respond to weather variations in a small endotherm facing cold environments? PloS One 9, 1–17. doi: 10.1371/journal.pone.0113617
Piersma T., Everaarts J. M., Jukema J. (1996). Build-up of red blood cells in refuelling bar-tailed godwits in relation to individual migratory quality. Condor. 98, 363–370. doi: 10.2307/1369154
Piersma T., Gessaman J. A., Dekinga A., Visser G. H. (2004). Gizzard and other lean mass components increase, yet basal metabolic rates decrease, when red knots Calidris canutus are shifted from soft to hard-shelled food. J. Avian Biol. 35, 99–104. doi: 10.1111/j.0908-8857.2004.03259.x
Piersma T., Lindström Å. (1997). Rapid reversible changes in organ size as a component of adaptative behaviour. Trends Ecol. Evol. 12, 134–138. doi: 10.1016/S0169-5347(97)01003-3
Ramenofsky M., Agatsuma R., Barga M., Cameron R., Harm J., Landys M. M., et al. (2003). “Migratory behavior: new insights from captive studies,” in Avian migration. Ed. G. E. Berthold P. (Springer, Berlin Heidelberg, New-York), 97–111.
Ramenofsky M., Campion A. W., Pérez J. H., Krause J. S., Németh Z. (2017). Behavioral and physiological traits of migrant and resident whitecrowned sparrows: A common garden approach. J. Exp. Biol. 220, 1330–1340. doi: 10.1242/jeb.148171
Ramenofsky M., Wingfield J. C. (2006). Behavioral and physiological conflicts in migrants: The transition between migration and breeding. J. Ornithol. 147, 135–145. doi: 10.1007/s10336-005-0050-4
Ramenofsky M., Wingfield J. C. (2017). Regulation of complex behavioural transitions: Migration to breeding. Anim. Behav. 124, 299–306. doi: 10.1016/j.anbehav.2016.09.015
Rehder N. B., Bird D. M. (1983). Annual profiles of blood packed cell volumes of captive American kestrels. Can. J. Zool. 61, 2550–2555. doi: 10.1139/z83-337
Rising J. D., Somers K. M. (1989). The measurement of overall body size in birds. Auk. 106, 666–674. doi: 10.2307/4087673
Romero L. M., Soma K. K., O’Reilly K. M., Suydam R., Wingfield J. C. (1998). Hormones and territorial behavior during breeding in snow buntings (Plectrophenax nivalis): An arctic-breeding songbird. Hormones. Behav. 33, 40–47. doi: 10.1006/hbeh.1997.1432
Rosenmann M., Morrison P. (1974). Maximum oxygen consumption and heat loss facilitation in small homeotherms by He-O2. Am. J. Physiol. 226, 490–495. doi: 10.1152/ajplegacy.1974.226.3.490
Saarela S., Heldmaier G. (1987). Effect of photoperiod and melatonin on cold resistance, thermoregulation and shivering/nonshivering thermogenesis in Japanese quail. J. Comp. Physiol. B. 157, 625–633. doi: 10.1007/BF00700983
Salvante K. G., Vézina F., Williams T. D. (2010). Evidence for within-individual energy reallocation in cold-challenged, egg-producing birds. J. Exp. Biol. 213, 1991–2000. doi: 10.1242/jeb.036319
Salvante K. G., Walzem R. L., Williams T. D. (2007). What comes first, the zebra finch or the egg: temperature-dependent reproductive, physiological and behavioural plasticity in egg-laying zebra finches. J. Exp. Biol. 210, 1325–1334. doi: 10.1242/jeb.02745
Scholander P. F., Hock R., Walters V., Johnson F. (1950a). Heat regulation in some Arctic and tropical mammals and birds. Biol. Bull. 99, 237–258. doi: 10.2307/1538741
Scholander P. F., Walters V., Hock R., Irving L. (1950b). Body insulation of some Arctic and tropical mammals and birds. Biol. Bull. 99, 225–236. doi: 10.2307/1538740
Schwabl H., Farner D. S. (1989). Endocrine and environmental control of vernal migration in male white-crowned sparrows, Zonotrichia leucophrys gambelil. Physiol. Zool. 62, 1–10. doi: 10.1086/physzool.62.1.30159994
Smith R. D. (1992). Age determination, wing-feather colour and wing-length change in snow buntings Plectrophenax nivalis. Ringing. Migration. 13, 43–51. doi: 10.1080/03078698.1992.9674014
Snell K. R. S., Stokke B. G., Moksnes A., Thorup K., Fossoy F. (2018). From Svalbard to Siberia: Passerines breeding in the High Arctic also endure the extreme cold of the Western steppe. PloS One 13, e0202114–e0202114. doi: 10.1371/journal.pone.0202114
Stager M., Swanson D. L., Cheviron Z. A. (2015). Regulatory mechanisms of metabolic flexibility in the dark-eyed junco (Junco hyemalis). J. Exp. Biol. 218, 767–777. doi: 10.1242/jeb.113472
Swanson D. L. (1990a). Seasonal acclimatization to temperature in Cardueline finches I. Insulative and metabolic adjustments. J. Comp. Physiol. 112, 1578–1592. doi: 10.2307/1368185
Swanson D. L. (1990b). Seasonal variation in cold hardiness and peak rates of cold-induced thermogenesis in the dark-eyed junco (Junco hyemalis). Auk. 107, 561–566. doi: 10.1093/auk/107.3.561
Swanson D. L. (2010). “Seasonal metabolic variation in birds: functional and mechanistic correlates,” in Current Ornithology. Ed. Thompson C. F. (Springer-Verlag, New-York), 75–129. Available at: www.springer.com/series/5816.
Swanson D. L., King M. O., Culver W. I., Zhang Y. (2017a). Within-winter flexibility in muscle masses, myostatin, and cellular aerobic metabolic intensity in passerine birds. Physiol. Biochem. Zool. 90, 210–222. doi: 10.1086/688956
Swanson D. L., Liknes E. T. (2006). A comparative analysis of thermogenic capacity and cold tolerance in small birds. J. Exp. Biol. 209, 466–474. doi: 10.1242/jeb.02024
Swanson D. L., McKechnie A. E., Vézina F. (2017b). How low can you go? An adaptive energetic framework for interpreting basal metabolic rate variation in endotherms. J. Comp. Physiol. B. 187, 1039–1056. doi: 10.1007/s00360-017-1096-3
Swanson D. L., Merkord C. (2012). Seasonal phenotypic flexibility of flight muscle size in small birds: A comparison of ultrasonography and tissue mass measurements. J. Ornithol. 154, 119–127. doi: 10.1007/s10336-012-0877-4
Swanson D. L., Olmstead K. L. (1999). Evidence for a proximate influence of winter temperature on metabolism in passerine birds. Physiol. Biochem. Zool. 72, 566–575. doi: 10.1086/316696
Swanson D. L., Vézina F. (2015). Environmental, ecological and mechanistic drivers of avian seasonal metabolic flexibility in response to cold winters. J. Ornithol. 156, 377–388. doi: 10.1007/s10336-015-1192-7
Swanson D. L., Zhang Y., Liu J.-S., Merkord C., King M. O. (2014). Relative roles of temperature and photoperiod as drivers of metabolic flexibility in dark-eyed juncos. J. Exp. Biol. 217, 866–875. doi: 10.1242/jeb.096677
Vézina F., Cornelius Ruhs E., O’Connor E. S., Le Pogam A., Régimbald L., Love O. P., et al. (2020). Consequences of being phenotypically mismatched with the environment: Rapid muscle ultrastructural changes in cold-shocked black-capped chickadees (Poecile atricapillus). Am. J. Physiol. Regulatory. Integr. Comp. Physiol. 318, R274–R283. doi: 10.1152/ajpregu.00203.2019
Vézina F., Dekinga A., Piersma T. (2011). Shorebirds’ seasonal adjustments in thermogenic capacity are reflected by changes in body mass: how preprogrammed and instantaneous acclimation work together. Integr. Comp. Biol. 51, 394–408. doi: 10.1093/icb/icr044
Vézina F., Gerson A. R., Guglielmo C. G., Piersma T. (2017). The performing animal: Causes and consequences of body remodeling and metabolic adjustments in red knots facing contrasting thermal environments. Am. J. Physiol. - Regulatory. Integr. Comp. Physiol. 313, R120–R131. doi: 10.1152/ajpregu.00453.2016
Vézina F., Salvante K. G. (2010). Behavioral and physiological flexibility are used by birds to manage energy and support investment in the early stages of reproduction. Curr. Zool. 56, 767–792. doi: 10.1093/czoolo/56.6.767
Vézina F., Williams T. D., Piersma T., Morrison R. I. G. (2012). Phenotypic compromises in a long-distance migrant during the transition from migration to reproduction in the High Arctic. Funct. Ecol. 26, 500–512. doi: 10.1111/j.1365-2435.2011.01955.x
Wagner E. C., Prevolsek J. S., Wynne-Edwards K. E., Williams T. D. (2008). Hematological changes associated with egg production: Estrogen dependence and repeatability. J. Exp. Biol. 211, 400–408. doi: 10.1242/jeb.011205
Walsh J. E., Shapiro I., Shy T. L. (2005). On the variability and predictability of daily temperatures in the Arctic. Atmosphere. - Ocean. 43, 213–230. doi: 10.3137/ao.430302
Whelan S., Strickland D., Morand-Ferron J., Norris D. R. (2016). Male experience buffers female laying date plasticity in a winter-breeding, food-storing passerine. Anim. Behav. 121, 61–70. doi: 10.1016/j.anbehav.2016.08.014
Williams T. D., Challenger W. O., Christians J. K., Evanson M., Love O. P., Vézina F. (2004). What causes the decrease in haematocrit during egg production? Funct. Ecol. 18, 330–336. doi: 10.1111/j.0269-8463.2004.00829.x
Williams T. D., Fronstin R. B., Otomo A., Wagner E. (2012). Validation of the use of phenylhydrazine hydrochloride (PHZ) for experimental manipulation of haematocrit and plasma haemoglobin in birds. Ibis 154, 21–29. doi: 10.1111/j.1474-919X.2011.01195.x
Wingfield J. C., Hunt K. E. (2002). Arctic spring: Hormone – behavior interactions in a severe environment. Comp. Biochem. Physiol. Part B.: Biochem. Mol. Biol. 132, 275–286. doi: 10.1016/s1096-4959(01)00540-1
Wingfield J. C., Jacobs J. D., Tramontin A. D., Perfito N., Meddle S., Maney D. L., et al. (1999). “Toward and ecological basis of hormone-behavior interactions in reproduction of birds,” in Reproduction in context: environmental and social influences on reproductive behavior and physiology. Eds. Wallen K., Schneider J. E. (MIT press, Cambridge), 85–128.
Wingfield J. C., Kelley J. P., Angelier F. (2011). What are extreme environmental conditions and how do organisms cope with them? Curr. Zool. 57, 363–374. doi: 10.1093/czoolo/57.3.363
Wingfield J. C., Owen-Ashley N., Benowitz-Fredericks Z., Lynn S. E., Hahn T., Wada H., et al. (2004). Arctic spring: The arrival biology of migrant birds. Acta Zool. Sin. 50, 948–960.
Wuenschel M. J., Jugovich A. R., Hare J. A. (2006). Estimation of muscle mass by ultrasonography differs between observers and life states of models in small birds. Trans. Am. Fisheries. Soc. 135, 379–385. doi: 10.1577/T04-233.1
Zhang Y., Eyster K., Liu J.-S., Swanson D. L. (2015). Cross-training in birds: Cold and exercise training produce similar changes in maximal metabolic output, muscle masses and myostatin expression in house sparrows (Passer domesticus). J. Exp. Biol. 218, 2190–2200. doi: 10.1242/jeb.121822
Zhang Y., Yap K. N., Williams T. D., Swanson D. L. (2018). Experimental increases in foraging costs affect pectoralis muscle mass and myostatin expression in female, but not male, zebra finches (Taeniopygia guttata). Physiol. Biochem. Zool. 91, 849–858. doi: 10.1086/697153
Keywords: Arctic birds, body composition, breeding, carry-over, cold acclimatization, life history stages, phenotypic flexibility, thermoregulation
Citation: Le Pogam A, O’Connor RS, Love OP, Young KG, Drolet J, Régimbald L, Roy G, Robitaille F, Berteaux D, Tam A and Vézina F (2024) Phenotypic constraints at the top of the world: an Arctic songbird faces the cumulative cost of maintaining a winter-like phenotype during breeding. Front. Ecol. Evol. 12:1369761. doi: 10.3389/fevo.2024.1369761
Received: 12 January 2024; Accepted: 05 March 2024;
Published: 03 April 2024.
Edited by:
Lin Zhang, Hubei University of Chinese Medicine, ChinaReviewed by:
Yang Wang, Hebei Normal University, ChinaShuping Zhang, Minzu University of China, China
Copyright © 2024 Le Pogam, O’Connor, Love, Young, Drolet, Régimbald, Roy, Robitaille, Berteaux, Tam and Vézina. This is an open-access article distributed under the terms of the Creative Commons Attribution License (CC BY). The use, distribution or reproduction in other forums is permitted, provided the original author(s) and the copyright owner(s) are credited and that the original publication in this journal is cited, in accordance with accepted academic practice. No use, distribution or reproduction is permitted which does not comply with these terms.
*Correspondence: Audrey Le Pogam, YXVkcmV5LmxlcG9nYW1AdXFhci5jYQ==