- 1Programa de Pós-Graduação em Geociências, Departamento de Paleontologia e Estratigrafia, Universidade Federal do Rio Grande do Sul (UFRGS), Porto Alegre, Rio Grande do Sul, Brazil
- 2Department of Paleobiology, Smithsonian Institution, National Museum of Natural History, Washington, DC, United States
- 3Department of Entomology, University of Maryland, College Park, College Park, MD, United States
- 4Capital Normal University, College of Life Science and Academy for Multidisciplinary Studies, Beijing, China
- 5Departamento de Ciências Agrárias e da Terra, Universidade do Estado de Minas Gerais, Passos, Minhas Gerais, Brazil
Introduction: The Sanzenbacher Ranch deposit represents an early Permian plant assemblage in north-central Texas predominantly composed of pteridosperms, of which callipterids are dominant. This study investigates the interactions of a taxonomically broad range of plant hosts with insects, mites, and pathogens to assess the richness and frequency of damage.
Methods: We used standard methods of the functional feeding group--damage type system and analyses graphically illustrated as nonmetric multidimensional scaling.
Results: Of the total surface area represented, we quantified the fraction of the area herbivorized, providing a comparative perspective with regard to several similar studies of herbivory at nearby plant assemblages from the late Paleozoic. To assess insect herbivory, 1,361 plant specimens were analyzed, including fronds, leaves, reproductive structures, axes, and wood impressions. A total of 205 specimens presented one or more instances of insect herbivory (11.17%). Callipterid-peltasperms exhibited the highest number of interactions, with Autunia conferta, Rhachiphyllum schenkii, cf. Lodevia sp., cf. Comia sp., and an unidentified callipterid accounting for 49.34% of all interactions. Medullosans represented 10.53%, followed by lyginopterids with 8.55%. Forty-eight damage types (DTs) were recognized and classified into the nine functional feeding groups of hole feeding, margin feeding, surface feeding, piercing and sucking, oviposition, galling, seed predation, borings, and pathogens.
Discussion: When compared to analogous late Paleozoic plant assemblages in Texas, Sanzenbacher Ranch exhibited almost all herbivore functional feeding groups, high richness of DTs, and low herbivory index of 0.26. This record shows high plant diversity in a seasonally dry environment that supports a trend of an early Permian increase in DT diversity in southwestern Euramerica. Sanzenbacher Ranch, different from other early Permian plant assemblages, sheds light on the dynamic ecological interactions of these ancient ecosystems.
1 Introduction
A remarkable climate change event was recorded at the end of the Paleozoic ra, associated with glacials–interglacial cycles, which resulted in changes in the vegetation of Euramerica (Montañez et al., 2007; DiMichele et al., 2011; Montañez and Poulsen, 2013; Montañez et al., 2016; Griffis et al., 2023). Corresponding to these glacial–interglacial cycles, there was an intercalation of plant assemblages adapted to humid environments (glacial) and plant assemblages tolerant of moisture deficits (interglacial) (DiMichele et al., 2011). An example of this transition can be found in Texas deposits at the end of the Pennsylvanian Subperiod to the early Permian Period, where significant differences in floral composition occurred, transitioning from wetlands to vegetation tolerant of seasonal drought (DiMichele et al., 2004, 2011, 2016; Simon et al., 2016; DiMichele et al., 2018a; Koll and DiMichele, 2021). The latter condition corresponds to climate changes (icehouse–greenhouse turnover) that occurred with the transition from the Carboniferous main glacial phase to the demise of the LPIA (Late Paleozoic Ice Age) through the Permian (Gastaldo et al., 1996; Montañez, 2022).
Occupying these Permian plant assemblages were insect lineages that had been associated with other vascular plants in a variety of environments. Nevertheless, these lineages accommodated to new plants in response to the climate changes that occurred during the Pennsylvanian–Permian transition, resulting insignificant shifts among the herbivorous insects (Schneider and Werneburg, 2006; Rasnitsyn et al., 2013). The insect fossil record shows that several hyperdiverse clades of insects underwent their initial radiation during the beginning of the early Permian (Prokop et al., 2023). Along with an increase in insect taxonomic diversity, there was a contemporaneous increase in the richness and intensity of insect, mite, and pathogen herbivory on plants (Labandeira, 2006b; Labandeira and Prevec, 2014; Xu et al., 2018; Labandeira, 2021). As herbivory expanded, insects developed different feeding strategies (Labandeira, 2019), including ectophytic, or external, consumption of plants through hole feeding, margin feeding, and surface feeding; stylophytic penetration of plant tissues through use of mouthpart stylets in piercing and sucking, or ovipositor blades in oviposition; endophytic, or internal, consumption of tissues from galling, seed predation, and borings; and an increase in necroses induced by pathogens (Labandeira and Wappler, 2023). Studies documenting interactions between insects and plants have proven effective in understanding these largest-scale herbivory patterns involving feeding classes (Labandeira, 2006b; Xu et al., 2018; Labandeira and Wappler, 2023). It is possible to extract data about the plant–insect associational dynamics of late Paleozoic fossil communities, such as the emergence of herbivory with land plants, the types of interactions that were established later during the Permian, and which herbivorous insects used these plants as a food resource (Labandeira, 2002; Labandeira and Wappler, 2023).
Studies of plant–insect interactions from Texan plant assemblages have been well-documented from the Gzhelian of the latest Late Pennsylvanian to the Roadian of the early middle Permian (Beck and Labandeira, 1998; Labandeira and Allen, 2007; Schachat et al., 2014, 2015; Xu et al., 2018; MacCracken and Labandeira, 2020). These studies have revealed that insects developed a spectrum of feeding strategies over time and contended with environmental factors that resulted in variation in the richness and intensity of the damage. The studies also shed light on how interactions changed as the environment also shifted, particularly with the emergence of more specialized herbivores. Documentation for these patterns of herbivory come from the eastern shelf of the Midland Basin of southeastern Texas and adjacent New Mexico, where the Archer City Formation crops out. This unit includes the plant assemblage of Sanzenbacher Ranch (SR), located in north-central Texas. The SR assemblage consists of drought-tolerant taxa that comprises one of the few plant assemblages of Asselian age in Paleozoic Euramerica. This plant assemblage is intercalated with other regional plant assemblages in Texas, providing valuable insights into the changing nature of plant–insect interactions from the latest Pennsylvanian to the early middle Permian. Our research aims to understand the spectrum of insect herbivory at the SR plant assemblage from the beginning of this time interval. In a broader sense, our goal also is to evaluate the composition of interactions between plants and nonvertebrate organisms toward the end of the Paleozoic Era in Euramerica by comparing the richness and frequency of associations of this plant assemblage with other such plant assemblages from north-central Texas during this time interval.
2 Geological paleobotanical context
2.1 The Archer City Formation
In Clay County, of the north-central region of Texas, USA, Pennsylvanian and Permian rock strata predominantly consist of continental deposits (DiMichele et al., 2018b). Alluvial sediments accumulated in the eastern portion of a coastal plain that bordered the eastern platform of the Midland Basin. The geological units identified in Clay County include the Markley Formation (Late Pennsylvanian to lowermost Permian), Archer City Formation (lower Permian, Wolfcampian Series), Nocona Formation (lower Permian, Wolfcampian Series), and Petrolia Formation (lower Permian, Leonardian Series) (Hentz, 1988). Some of these stratigraphic units are time transgressive.
The Archer City Formation, together with the Markley Formation, encompass the Bowie Group (Pennsylvanian–Permian) (Figure 1). The lithological composition of the Archer City Formation primarily consists of clastic deposits, mainly of claystone, with smaller components of siltstone, sandstone, and conglomerate (Hentz, 1988). The thickness of the formation varies from 107 to 122 m and includes strata of sandstone with thicknesses of approximately 15 m. The plants are well-preserved but not to the extent of the somewhat older Williamson Drive material (Xu et al., 2018). Nonetheless, SR plant fossils exceed the threshold for the detection of the most inconspicuous of interactions, punctures made by piercing-and-sucking insects. Compared to other examined plant assemblages from the latest Pennsylvanian to early middle Permian of Texas, the preservation at Sanzenbacher Ranch is somewhat less informative than Williamson Drive (Xu et al., 2018), more informative than Coprolite Bone Bed (Labandeira and Allen, 2007) and South Ash Pasture (Maccracken and Labandeira, 2020), and of similar preservational style as Taint (Beck and Labandeira, 1998), Colwell Creek Pond (Schachat et al., 2014), and Mitchell Creek Flats (Schachat et al., 2015).
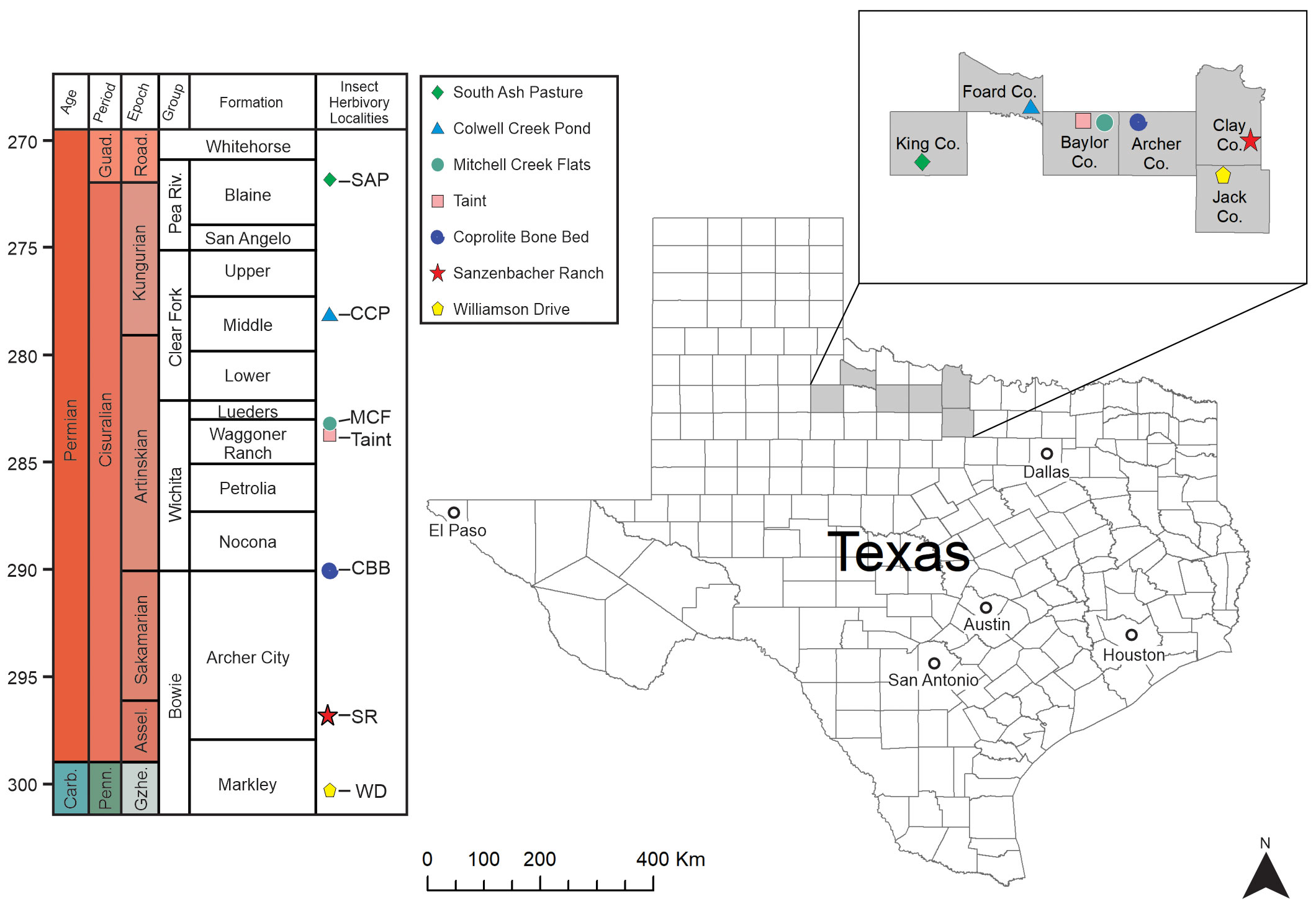
Figure 1 The Sanzenbacher Ranch plant assemblage in north-central Texas. The figure shows geographical and stratigraphic position of all seven Pennsylvanian–Permian plant assemblages that exhibit insect herbivory.
2.2 The Sanzenbacher Ranch plant assemblage
The Sanzenbacher Ranch plant assemblage deposit is situated in the middle region of the Archer City Formation (Figure 1) and is pegged to the Asselian Stage of the early Cisuralian. Based on the field notes of DiMichele et al. (2018a), the Sanzenbacher Ranch plant assemblage is predominantly composed of pteridosperms, followed by conifers. Within the pteridosperms, the callipterid-peltasperms are the most abundant plant group at the outcrop, where Autunia conferta is the most prevalent plant, followed by Rhachiphyllum schenkii and cf. Lodevia. The most abundant medullosan is Neurodontopteris auriculata, along with Odontopteris spp. A lyginopterid is represented by Sphenopteridium germanicum. Coniferopsids constitute the second most abundant plant group, although the identification process of these specimens is challenging. Coniferopsids include Walchia sp. 1, Cordaites sp. 1 and fragments of conifer scales, cones, and bracts (DiMichele et al., 2018a).
Sphenopsids are represented by the calamitaleans Annularia spicata, Annularia carinata, and Asterophyllites equisetiformis; sphenophylls are represented by the Sphenophyllum verticillatum, Sphenophyllum thonii, Sphenophyllum angustifolium, and Sphenophyllum oblongifolium. The reproductive organs for calamitaleans are identified as Calamostachys sp. 1 and Palaeostachya sp. 1 (DiMichele et al., 2018a). The two groups of ferns occurring at the outcrop are arborescent marattialeans (Marattiales) and herbaceous filicaleans (Filicales). Due to the degree of fragmentation of the material, identifications for sterile specimens are provisional, designated, for example, as Pecopteris sp. 1 or as marattialean foliage. Fertile specimens of Asterotheca sp. 1 are also present (DiMichele et al., 2018a). Some specimens have been identified as Pecopteris monyi, Pecopteris hemiltelioides, Pecopteris permica, Oligocarpia gutbieri, Oligocarpia cf. gutbieri, and Sphenopteris sp. 1 (DiMichele et al., 2018a). Other fern fossils also are found in the Sanzenbacher Ranch plant assemblage, with some lacking a well-defined taxonomic affinity, and others possibly associated with seed plants. These other plant fossils include Taeniopteris sp. 1, a likely seed plant, and Yuania, a noeggerathialean, recently shown to be a pteridophyte (Yang and Wang, 2012). Also present are reproductive structures such as Trigonocarpus sp. 1 and various unaffiliated winged seeds (DiMichele et al., 2018a).
3 Materials and methods
3.1 Field collections and fossil preparation
The studied material comes from the Sanzenbacher Ranch locality (Figure 1), and the plant assemblage from this locality is reposited in two collections with three locality numbers: NPL 1161, USNM 40600, and USGS 9999. The specimens were collected during three separate field expeditions. The first collection was made between 1940 and 1941 as part of the Statewide Paleontological–Mineralogical Survey conducted by the Works Progress Administration (WPA), led by Adolph H. Witte (DiMichele et al., 2018a). These specimens are currently at the Non-vertebrate Paleontology Laboratory at the University of Texas at Austin, under the locality number NPL 1161. The second collection, made during the 1960s, was conducted by Sergius H. Mamay and Arthur D. Watt and is cataloged under the locality number USGS 9999. Last, a field trip in 1991, including Mamay, William DiMichele, Dan Chaney, and Robert Hook collected fossils at the same locality as the second collection. Specimens from this collection are cataloged under locality number USNM 40600. The USNM (40600) and the USGS (9999) specimens are housed at the Smithsonian’s National Museum of Natural History, in Washington, DC.
Paleoecological studies have revealed differences among the three collections. The USGS (United States Geological Survey) collection is dominated by a single species, A. conferta, which comprises more than 30% of the specimen occurrences. By contrast, the USNM (United States National Museum, later renamed the National Museum of Natural History or NMNH) collection is dominated by three species with >10% of the occurrences attributable individually to A. conferta, Neurodontopteris auricular, and collectively several species of Pecopteris. The last collection, the NPL material, encompasses four species each accounting for >10% of the occurrences: A. spicata, Calamites stems, Walchia sp. 1, and A. conferta (DiMichele et al., 2018a).
All plant specimens present in the three collections were analyzed, including leaves, fronds, reproductive structures (seeds and cones), stems and other axes, and wood impressions. Photography and data collection were conducted in a Paleobiology laboratory at the NMNH and at the Non-vertebrate Paleontology Laboratory (The University of Texas at Austin) using a Leica StereoZoom 6 Plus stereomicroscope to identify and record instances of herbivory and pathogens. An important attribute in evaluating this herbivory was the presence of a reaction rim and other tissue deformations attributable to herbivory and teratologic structures such as galls (Meyer and Maresquelle, 1983). All specimens were photographed using a Canon EOS 50D and a Canon EOS Rebel T3 camera with a Canon EF-S 60mm macro lens for documentation and assembling additional data for quantitative analyses.
3.2 Qualitative and quantitative analyses
We recorded presence–absence data for damage types (DTs) consistent with production by insects, mites, and pathogens. The damage was classified into functional feeding groups based on the available literature (Labandeira et al., 2007 and subsequent addenda). Nine functional feeding group categories were found: hole feeding, margin feeding, surface feeding, piercing and sucking, oviposition, galling, seed predation, borings, and pathogens. For rare unassignable damage, descriptions were provided. The number of feeding event occurrences (FEOs) were tallied, which indicate how many times a feeding event of a particular DT occurs in on single plant organ such as a leaf (Xiao et al., 2022a, b, c).
The program ImageJ 1.53t (Image processing and analysis in Java Wayne Rasband and contributors, National Institutes of Health, USA) was used to assess the surface areas of the plant specimens. Photos of the specimens were used to calculate the total surface area (TSA) and total herbivorized surface area (THSA) for each specimen and collectively for the plant assemblage. These two measurements, each summed over the entire plant assemblage, were employed to calculate the herbivory index (HI) for the plant assemblage. To evaluate the herbivory index, we applied the method used by Beck and Labandeira (1998):
And for the proportion of herbivorized leaves (PHL):
Herbivory was assessed in terms of richness and intensity of interactions. Richness was determined by DT diversity for the total plant assemblage and for each plant taxon and morphotype. Intensity was determined by how often, or the frequency of, the DTs that were present in the plant assemblage and for each taxon in the plant assemblage.
We evaluated the relationships between functional feeding groups and taxa in the six other plant assemblages from the uppermost Pennsylvanian to early middle Permian interval of north-central Texas. Ordination methods, consistent with those employed by previous authors, were used (Schachat et al., 2015; Xu et al., 2018; MacCracken and Labandeira, 2020; also see Schachat et al., 2022). We constructed a dissimilarity matrix obtained from a Bray–Curtis index that was inputted into a Non-metric Multidimensional Scaling (NMDS) ordination analysis and carried out using the vegan package (Oksanen et al., 2022) in R (R Development Core Team, 2013). This analysis was performed using the “metaMDS” function. The analysis resulted in two separate NMDS plots. The first plot illustrates the relationship between the FFGs and leaf taxa. The second plot shows the relationships of the most herbivorized functional feeding groups and plant taxa for each plant assemblage present during the late Paleozoic interval in Texas.
4 Results
4.1 Composition of the plant assemblage
We analyzed 1,361 specimens from the SR plant assemblage (Supplementary Table S1) across three collections: NPL 1161 collection with 531 specimens, USNM 40600 with 311 specimens, and USGS 9999 with 519 specimens. Each of the three collections exhibited a significantly different taxonomic composition. The NPL collection displayed an elevated presence of calamitaleans, the USNM collection was dominated by A. conferta, and the USGS collection had the highest percentage of morphotype seed sp. 9. Collectively, pteridosperms were the most abundant group, of which A. conferta was the most abundant pteridosperm taxon, followed by R. schenckii. In addition, all three collections contained a significant number of reproductive organs and stems, such as seed morphotype sp. 9 and calamitalean axes, respectively.
Pteridosperms were the most abundant group, accounting for 29.76% of the total plant assemblage organs, and included lyginopterids, medullosans, callipterid peltasperms, and an unidentified pteridosperm (Supplementary Table S2). Peltasperms comprised 19.25% of the plant organs of the total plant assemblage that included callipterid leaves of A. conferta, R. schenkii, cf. Lodevia, cf. and Comia sp., and female reproductive structures of Peltaspermum sp. Medullosans, represented by the genera Neurodontopteris, Odontopteris, and cf. Eusphenopteris made up 6.47%. Lyginopterids, represented solely by Sphenopteridium germanicum, accounted for 3.97%.
Seeds accounted for 25.5% of the total plant assemblage, with all specimens unattached to reproductive structures. The seeds have been classified by the authors into nine seed morphotypes and one identifiable Trigonocarpus sp. 1. Seed sp. 9 was the most abundant morphotype at the Sanzenbacher plant assemblage. Notably, small, isolated, rounded seeds were prevalent, along with two other morphotypes with greater representation: seed sp. 6 and seed sp. 5.
Sphenopsids, mainly composed of whorls foliage of Annularia and stems of Calamites sp. 1, accounted 15.43% of the occurrences, consisting of 210 specimens. The third most abundant group of the Sanzenbacher plant assemblage was comprised of calamitalean stems, followed by A. spicata, which was notably abundant in the NPL 1161 collection. Marattialean, filicalean, and other ferns also made a notable contribution to the plant assemblage, representing 7.42% of all specimens. The most abundant fern was Pecopteris consisting of several species treated here as a single group, followed by Asterotheca sp. 1, and Sphenopteris sp. 1.
Other groups were less represented, each having fewer than 10 specimens. These included cycadopsids, ginkgopsids, and Noeggerathiales. Unidentified specimens found in the USGS 9999 and NPL 1161 localities could not be identified due to fragmentary material and whitish surfaces on some specimens, making it impossible to discern venation or other important foliar features. Lycopsids were absent.
4.2 Composition of plant–insect and plant–pathogen interactions
The SR plant assemblage had 152 specimens with at least one instance of a DT caused by insects, mites, or pathogens (11.17%). We observed 48 different DTs, 205 DT occurrences, and 547 feeding event occurrences (FEOs) across 31 plant morphotypes (Table 1). The callipterids collectively hosted nearly half of the recorded DTs, comprising 49.34% of the total (Supplementary Table S2). The taxon with the greatest DT richness was A. conferta (18 DTs and 58 DT occurrences), followed by R. schenkii (16 DTs and 41 DT occurrences). The lyginopterid S. germanicum was the third group with the greatest richness (11 DTs and 17 DT occurrences), with 8.55% of the total. Cordaites sp. 1 and N. auriculata presented nine DTs, whereas cf. Lodevia sp. had eight DTs, represented by 7.24%, 6.58%, and 3.95%, respectively. The Sanzenbacher plant assemblage had a total surface area of 8,687.86 cm2 and an herbivorized surface area (HSA) of 22.48 cm2 (Supplementary Table S1). The taxon with the greatest surface area was A. conferta, with 5,212.77 cm2, 60% of the total, and an HSA of 6.11 cm2, comprising 27.2% of the total herbivorized area. In the second place is R. schenckii, which had 551.43 cm2 (6.35%) and an HSA of 5.811 cm2 (25.84%). (More information regarding the SA and HSA are available at the Supplementary Table S5).
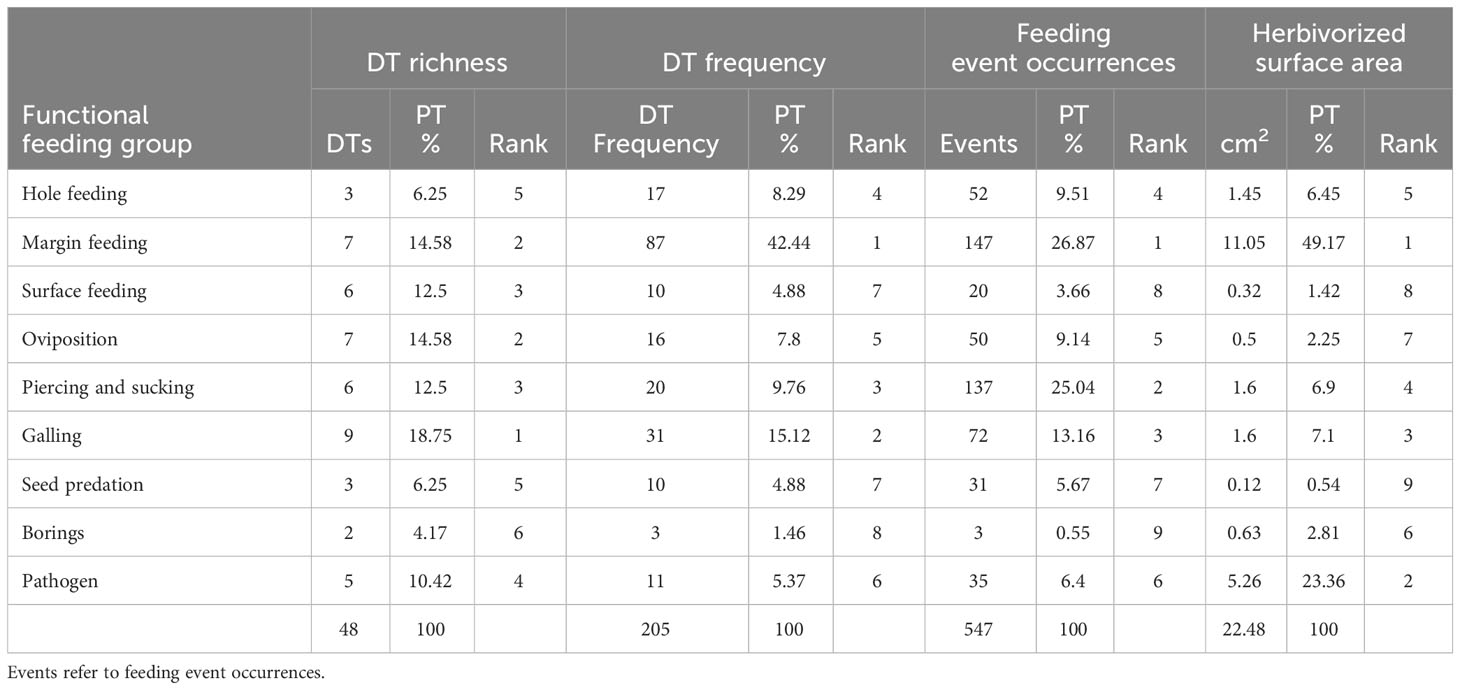
Table 1 Functional feeding groups for the Asselian Sanzenbacher Ranch plant assemblage from Texas and their rank position by damage type (DT) richness, DT frequency, feeding event occurrences, and herbivorized surface area.
4.2.1 Hole feeding
Definition and natural history. Hole feeders are insects with mandibulate mouthparts and a chewing feeding style that are responsible for incisions surrounded by plant reaction tissue. Hole feeding is characterized by complete consumption of the leaf thickness, resulting in rounded, ellipsoidal, polylobate, and irregular incision shapes. The insect feeds locally on all tissues of the leaf blade, removing the entire thickness and thus causing small to large incisions.
DTs present. DT01 (Figures 2L–O, 3F, G, M-O, T-V, 4A, C); DT02 (Figures 2J, K, 3A, B, P, Q); and DT08 (Figures 2A–G, I, 3R, S).
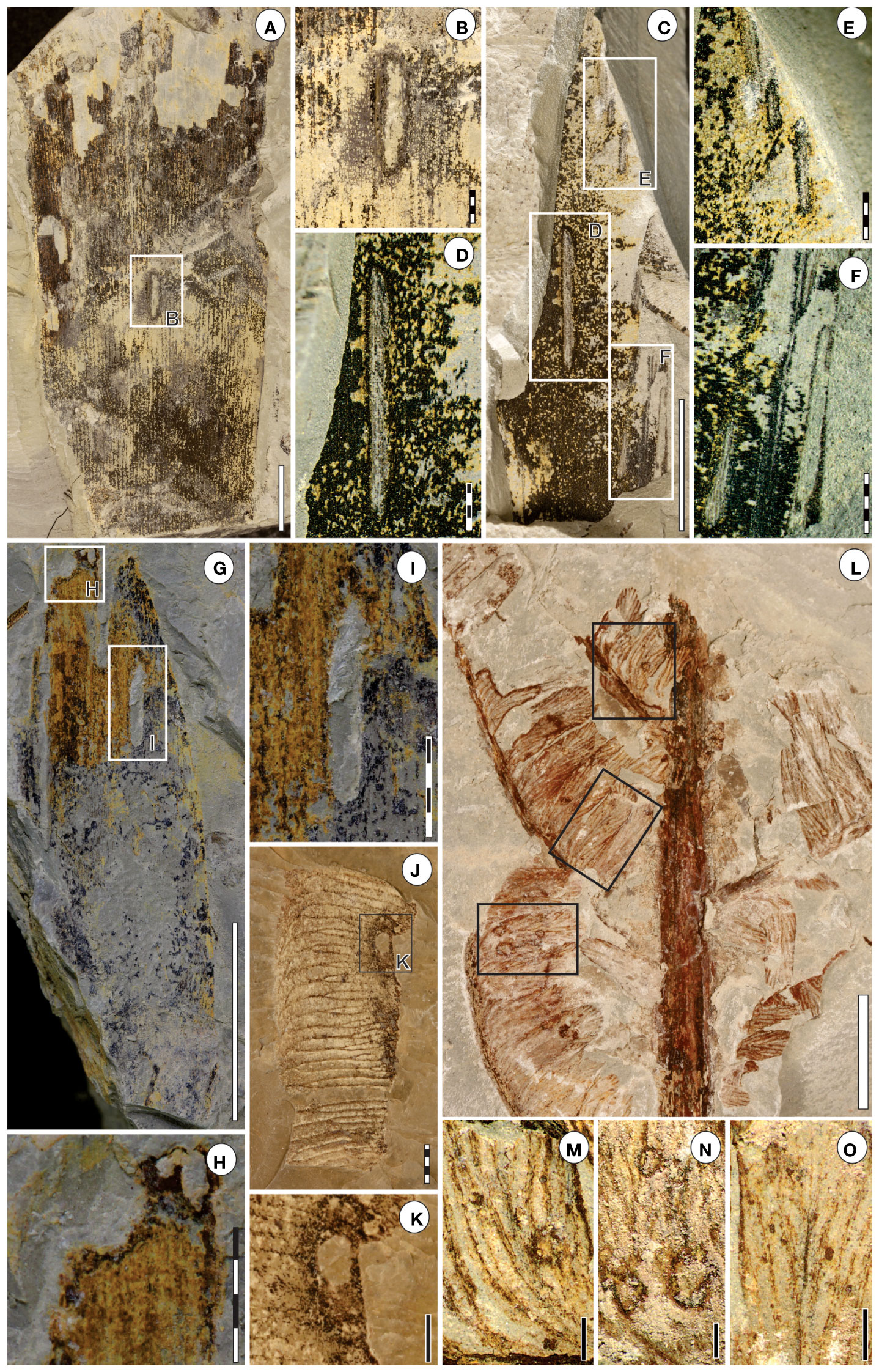
Figure 2 Hole feeding (DT01, DT02, and DT08) and piercing-sucking (DT46) from localities USNM 40600, USGS 9999, and NPL 1161. (A) Cordaites sp. 1 with hole feeding DT08 resulting in slot-like incisions (USNM 774410), magnified in (B). (C) Cordaites sp. 1 with hole feeding DT08 (USNM 535880b), detailed in (D–F). Note approximately seven slot feeding incisions on this leaf. (G) Cordaites sp. 1 with hole feeding DT08 and margin feeding DT12 (NPL TX000001161.119b); DT12 is enlarged in (H), and DT08 is enlarged in (I). Note that this slot damage occupies two interveinal spaces. (J) Taeniopteris sp. 1 with hole feeding DT02 (USGS 612415), detailed in (K), displaying a wide swath of surrounding necrotic tissue. (L) Rhachiphyllum schenkii with hole feeding DT01 and piercing-and-sucking DT46 (USNM 536560). DT01 shows veinal stringers in the incisions, enlarged in (M, N); four punctures of DT46 is detailed in (O). Scale bars: white, 10 mm; black and white, 2 mm; black, 1 mm.
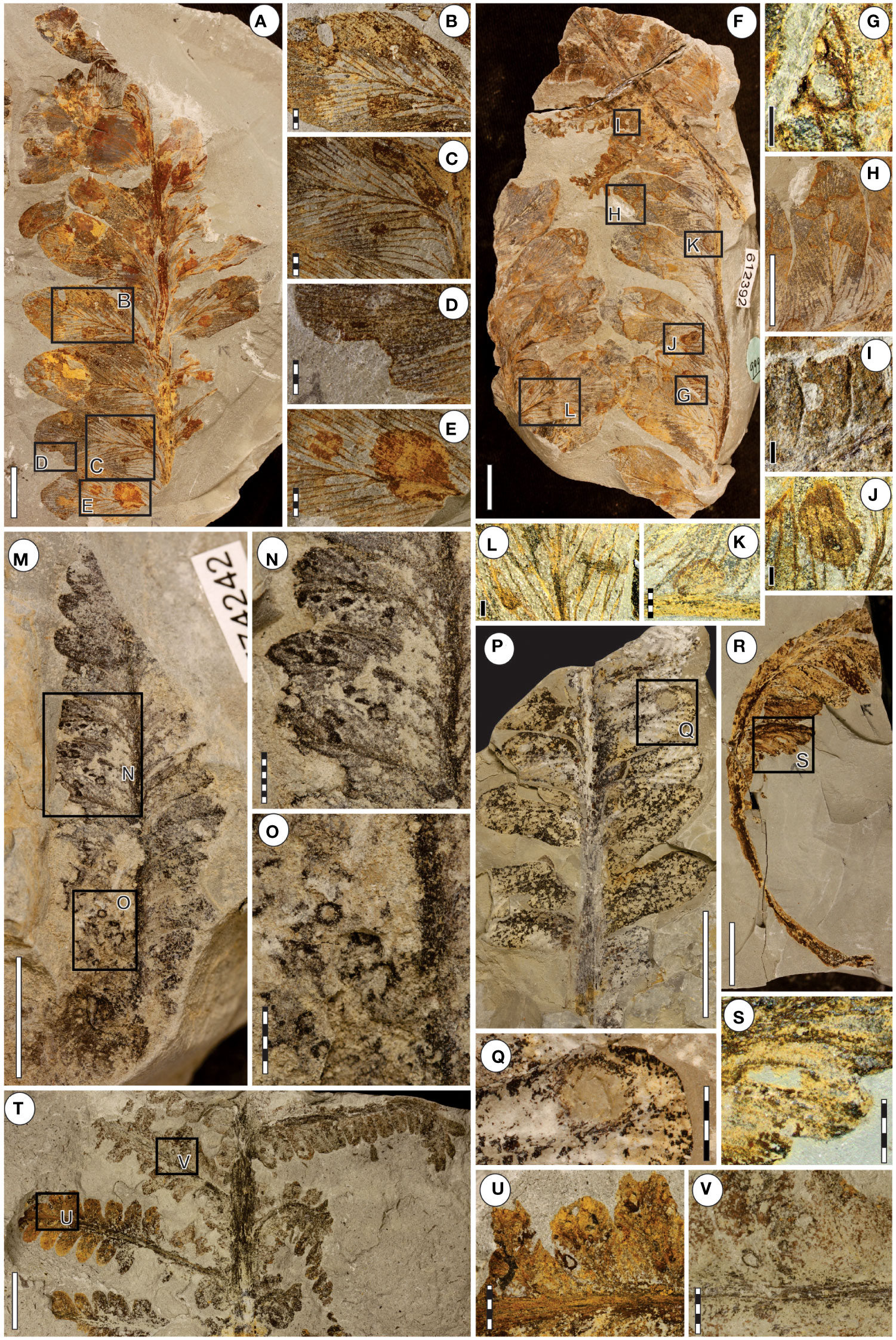
Figure 3 Hole Feeding (DT01, DT02, and DT08), margin feeding (DT12 and DT13), a gall (DT34), and pathogens (DT174) from localities USNM 40600 and USGS 9999. (A) Rhachiphyllum schenkii with hole feeding DT02, pathogen DT174, gall DT34, and margin feeding DT12 (USGS 774576a). DT02 is detailed in the upper left of (B); multiple examples of DT34 is illustrated in (C); DT12 is shown in (D); and DT174 is at (E). (F) Rhachiphyllum schenkii with several functional feeding groups, including hole feeding DT01 enlarged in (G), margin feeding DT13 at (H), the gall DT34 at (L), and pathogen DT174 (USNM 612392a) at (J, K). In addition, at (F) is Autunia conferta (USNM 612392b) with margin feeding DT12 at (I). (M) Autunia conferta displaying DT01 hole feeding (USNM 774242), detailed in (N, O). (P) Autunia conferta with DT02 hole feeding (USGS 536566), detailed at (Q), showing a thick reaction rim. (R) Rhachiphyllum schenkii with DT08 hole feeding (USNM 635896), detailed at (S). (T) Autunia conferta with DT01 hole feeding (USNM 635900), detailed in (U, V), showing small, delicate incisions. Scale bars: white, 10 mm; black and white, 2 mm; and black, 1 mm.
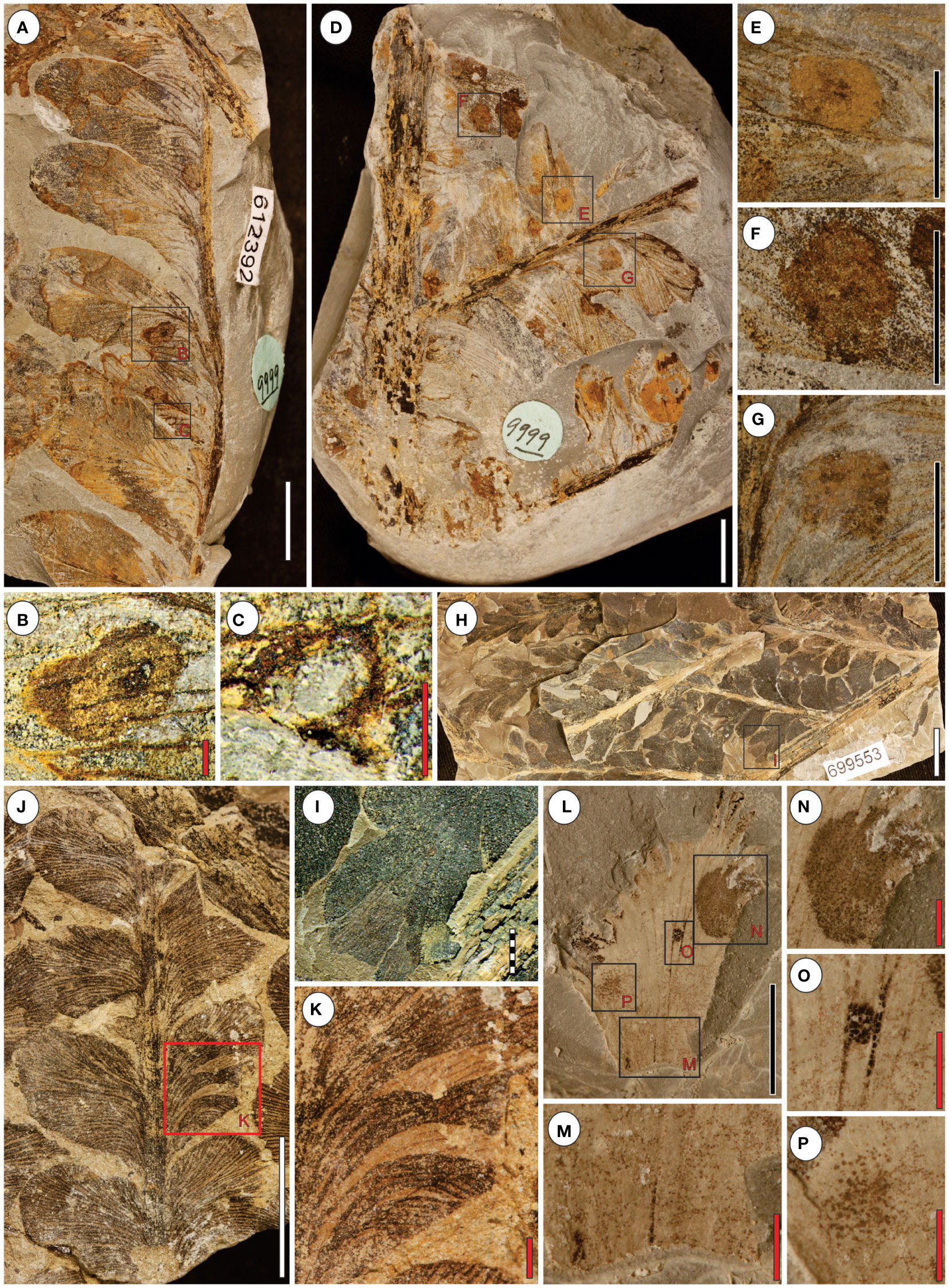
Figure 4 Pathogens (DT66, DT75, DT97, and DT174), hole feeding (DT01), and a gall (DT34) from USNM localities 40600, USGS 9999, and NPL 1161. (A) Cordaites sp.1 with DT97 pathogen (USNM 774369a) enlarged in (B) and DT01 hole feeding enlarged in (C). (D) Rhachiphyllum schenkii with three examples of DT174 (USGS 774572a), detailed in (E–G). (H) Sphenopteridium germanicum with V-shaped DT97 (USGS 699553a), detailed in (I), showing necrotic tissue at lower left surrounded by a reaction front. (J) Odontopteris subcrenulata with U-shaped DT75 (USGS 612381), detailed in (K) and exhibiting a prominent reaction front. (L) Sphenophyllum thonii with DT66 pathogen, DT34 gall, and a cluster of punctures that form DT281 (USGS 774582). (M) shows punctures clusters DT281; (N) shows pathogen DT66 consisting of three or four concentric circles of miniscule fructification with each dot representing a separate fructification; the detail of DT34 is in (O); (P) provide an enlargement of the puncture clusters DT281. Scale bars: white, 10 mm; black, 5 mm; black and white, 2 mm; red 1, mm.
Plant hosts. Pteridosperms: A. conferta (DT01, DT02); R. schenkii (DT01, DT02, DT08); and cf. Lodevia sp. 1 (DT02). Coniferophytes: Cordaites sp. 1 (DT08). Ferns: Pecopteris hemitelioides (DT01). Incertae sedis: Taeniopteris sp. 1 (DT02).
Sanzenbacher Ranch DT occurrences. Hole feeding consisted of three DTs representing 6.25% of all DTs at SR. We recorded 17 DT occurrences and 52 FEOs (Table 1), hosted by six fossil plant species (Supplementary Table S4). Rhachiphyllum schenkii had the highest occurrence of DTs, followed by A. conferta and Cordaites sp. 1. Within hole feeding, DT01 was most common compared to the other hole-feeding DTs, present in two callipterids and one marattialean fern. DT08 was abundant on Cordaites sp. 1, with four occurrences; DT02 was observed only in pteridosperms and was less abundant than DT01. Hole feeding represented 6.45% of the herbivorized surface area removed and was the fifth most represented functional feeding group (Table 1).
Paleozoic DT occurrences. Hole feeding has been reported in Gondwanan plant assemblages, present in leaves of Cordaites, Gangamopteris spp., Glossopteris spp., and Botrychiopsis sp (Adami-Rodrigues et al., 2004; Pinheiro et al., 2012; Gallego et al., 2014; Pinheiro et al., 2015; Cariglino, 2018; Edirisooriya et al., 2018). Reports of hole feeding in Cathaysian plant assemblages mention considerable hole-feeding on gigantopterid foliage (Liu et al., 2020; Zhou et al., 2020; Ma et al., 2023), while other taxa were less representative in hole feeding. For Euramerican plant assemblages, such as those in Texas and New Mexico, hole feeding occurs in abundance. There is a record of hole-feeding DTs on Macroneuropteris scheuchzeri and Charliea manzanitana for the latest Pennsylvanian (Xu et al., 2018; Donovan and Lucas, 2021) and an extensive record on Permian gigantopterids, callipterids, cycadophytes, and cordaitaleans from early Permian plant assemblages of north-central Texas (Beck and Labandeira, 1998; Labandeira and Allen, 2007; Schachat et al., 2014, 2015; Xu et al., 2018; MacCracken and Labandeira, 2020).
4.2.2 Margin feeding
Definition and natural history. Margin feeding is attributed to arthropods with mandibulate mouthparts and a chewing feeding style. The process of margin feeding results in consumption of the entire leaf blade thickness along the leaf edge, typically as cuspate or deeper U-shaped excisions. Margin feeding, like hole feeding, often exhibits dark reaction tissue along the cut edge, but often, other features such as veinal stringers and necrotic flaps of tissue are present. Margin-feeding arthropods primarily feed along the lateral regions of foliage but also initiate their attacks at foliage apices and bases.
DTs present. DT12 (Figures 2G, H, 3A, D, I, 5A, H); DT13 (Figures 3F, H, 5A–E, I-K, P–X); DT14 (Figures 5A, F, G, I, J, 6L, O); DT15; DT81 (Figures 3F, I); DT162 (Figures 5L, M); and DT348 (Figures 5N, O).
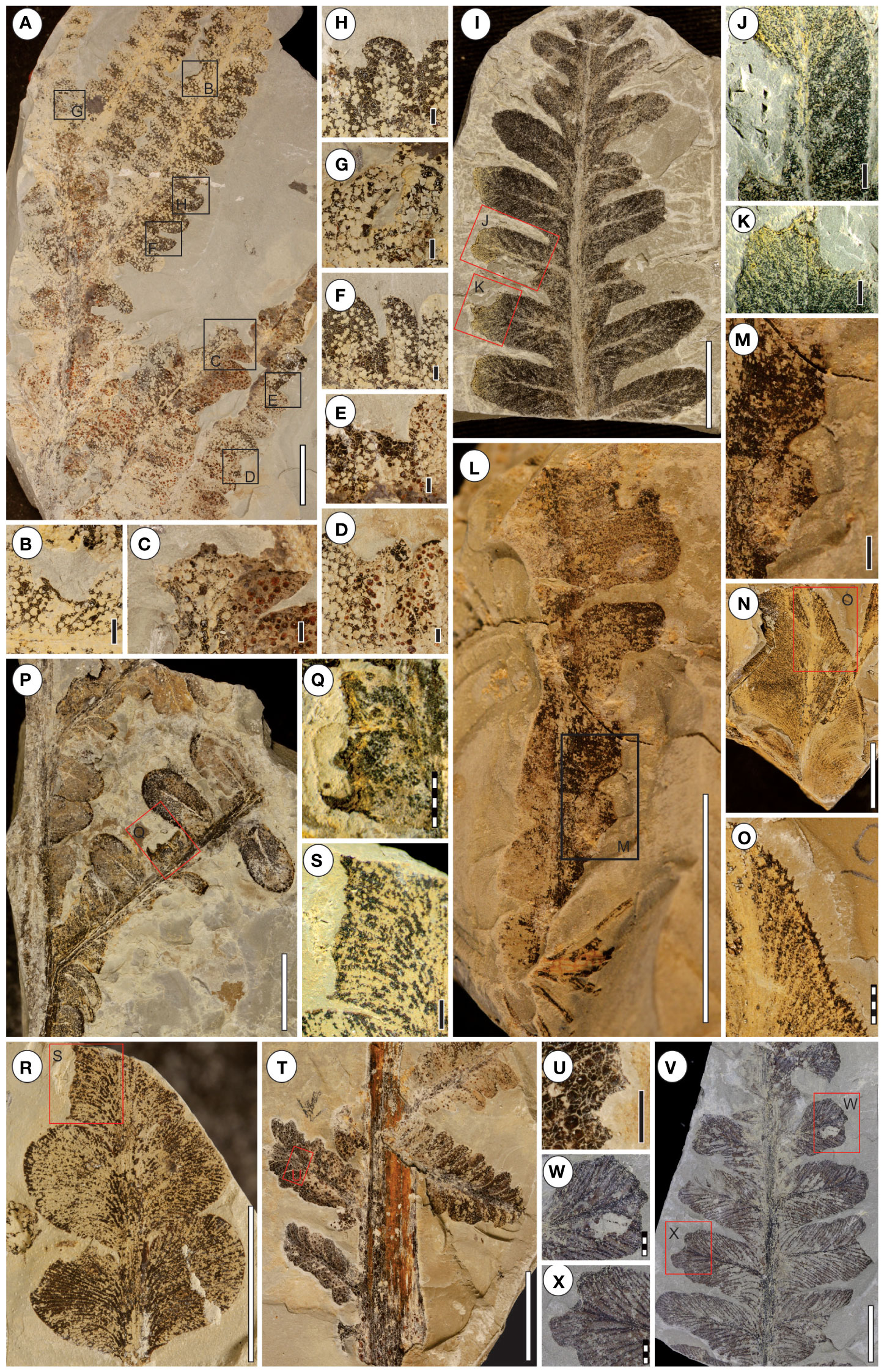
Figure 5 Margin feeding (DT12, DT13, DT14, DT162, and DT348), from localities USNM 40600, USGS 9999, and NPL 1161. (A) Autunia conferta with DT12, DT13, and DT14 (USNM 536556); enlargements of DT13 are in (B–E); DT14 in (F, G); and DT12 in (H). (I) Rhachiphyllum schenkii with DT13 and DT14 (USGS 612365); enlargements of DT14 is in (J) and DT13 in (K). (L) Taeniopteris sp. 1, showing margin feeding, DT162, along the right side of the leaf (NPL TX000001161.89), displaying extensive margin feeding, detailed in (M). (N) Neurodontopteris auriculata with DT348 (774431), showing distinctive veinal stringers along the excised leaf margin at (O). (P) Autunia conferta with DT13 (USGS 612403), displaying features of the excised margin in (Q). (R) Odontopteris sp. 1 with DT13 (USGS 774512), the excised margin, with cuspules, detailed at (S). (T) Autunia conferta with DT13 (USGS 774573), magnified at (U). (V) Rhachiphyllum schenkii with DT13 (NPL TX000001161.136), magnified in (W, X). Scale bars: white, 10 mm; black and white, 2 mm; black, 1 mm.
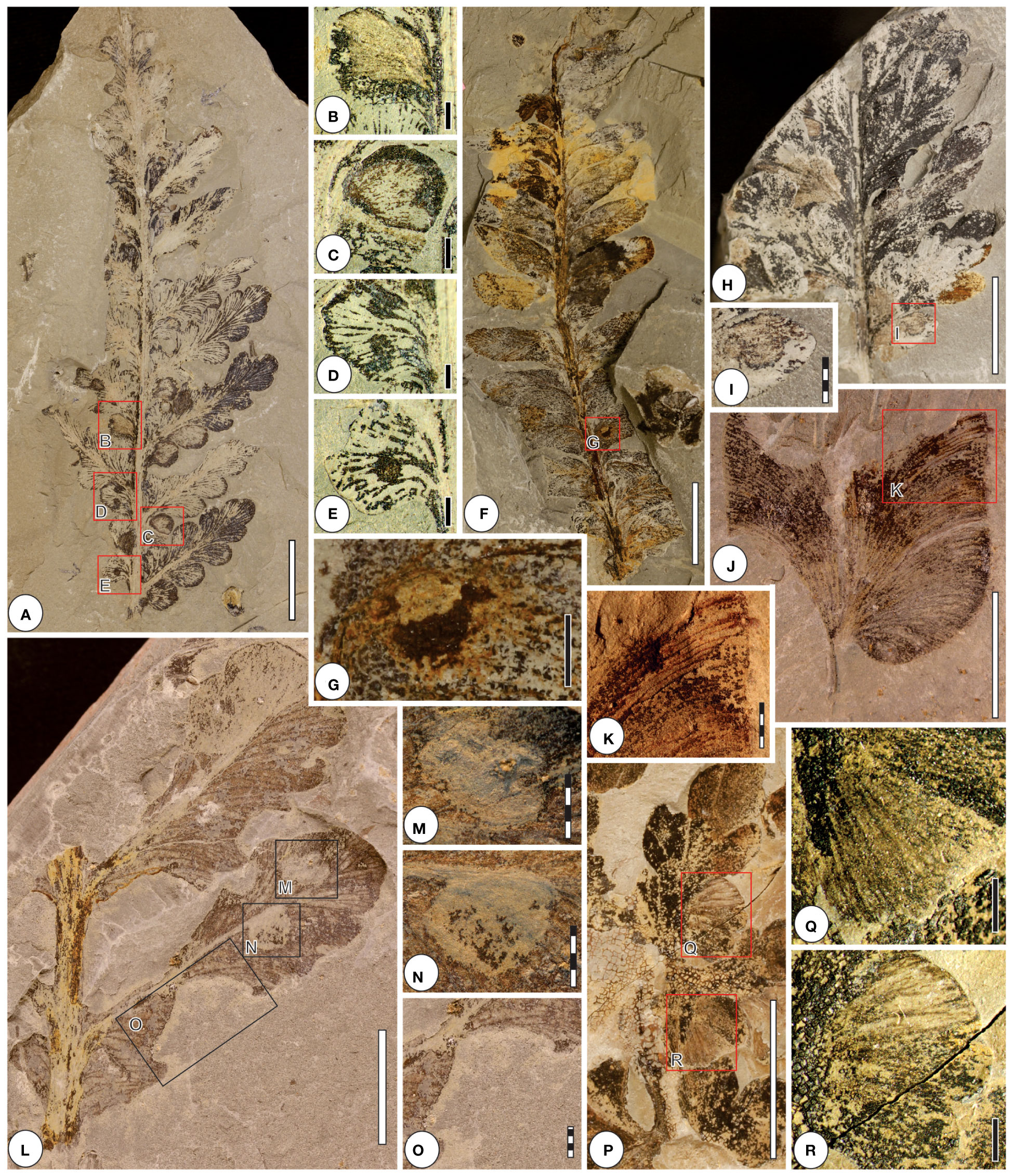
Figure 6 Surface feeding (DT25, DT27, DT29, DT30, and DT238), a gall (DT80), and margin feeding (DT14) from localities USNM 40600, USGS 9999, and NPL 1161. (A) Sphenopteridium germanicum with surface feeding DT29 and gall DT80 (USGS 699552); DT29 is enlarged in (B, C); and DT80, appearing as opaque circular structures with a lightly hued center, is detailed in (D, E). (F) Rhachiphyllum schenkii with DT30 surface feeding (USNM 635915), displaying a dark center, magnified in (G). (H) Sphenopteridium germanicum with DT30 surface feeding (USNM 774232a), showing a discernable reaction rim, is detailed in (I). (J) Neurodontopteris auriculata with DT25 surface feeding DT25 (NPL TX000001161.12), showing a narrow channel of consumed leaf adjacent a vein displayed at (K). (L) cf. Lodevia sp. 1 (NPL TX000001161.03a) with DT238 surface feeding in the vein axils, detailed in (M, N), and DT14 margin feeding extending to a major vein in (O). (P) Sphenopteridium germanicum with DT27 surface feeding DT27 (USGS 774433a), detailed in (Q, R), exhibiting a lighter hue than the adjacent, unherbivorized, leaf surface Scale bars: white, 10 mm; black and white, 2 mm; black, 1 mm.
Plant hosts. Pteridosperms: A. conferta (DT12, DT13, DT14, DT15, DT81); R. schenkii (DT12, DT13, DT14, DT15); cf. Lodevia sp. (DT12, DT13, DT14); Sphenopteridium germanicum (DT12, DT13, DT14); Odontopteris subcrenulata (DT13); Odontopteris sp. (DT13); N. auriculata (DT12, DT348); callipterid (DT13); neuropterid (DT12); and unidentified pteridosperm (DT12, DT13). Conifers: Cordaites sp. 1 (DT13). Sphenopsids: S. thonii (DT13); and Annularia cf. carinata (DT13). Ferns: cf. Oligocarpia sp. (DT12, DT13); and Pecopteris sp. (DT13, DT14). Incertae sedis: Taeniopteris sp. (DT162); and unidentified specimens (DT13).
Sanzenbacher Ranch DT occurrences. Margin feeding was the group with the second highest DT diversity, representing 7 out of 48 DTs (14.58%) across 17 plant morphotaxa and species (Supplementary Table S4). We recorded 87 DT occurrences and 147 FEOs (Table 1). A. conferta represented 40.23% of all margin feeding DT occurrences, and R. schenkii 19.54%. In addition, S. germanicum and cf. Lodevia sp. 1 had 10.34% and 8.04%, respectively, of margin feeding DT occurrences. Notably there was an elevated presence of DT12, DT13, and DT14. Margin feeding was represented by 49.17% of the total surface area removed and was the most represented functional feeding group (Table 1).
Paleozoic DT occurrences. Margin feeding is the most abundant functional feeding group in Paleozoic floras, and during the Permian, it was present in the floras of Gondwana, Euramerica, and Cathaysia. This type of damage is found in many plant groups in Gondwana but is most pronounced in glossopterids (Cariglino and Gutiérrez, 2011; Pinheiro et al., 2012; Gallego et al., 2014; Cariglino, 2018; Edirisooriya et al., 2018; Fernández and Chiesa, 2019). For Cathaysian floras, it is common to find margin feeding preferentially on gigantopterids (Glasspool et al., 2003; Liu et al., 2020; Zhou et al., 2020) and less common in other plant groups (Liu et al., 2020). Margin feeding occurs with abundance in Euramerican plant assemblages, especially on callipterids, gigantopterids, and medullosans (Beck and Labandeira, 1998; Labandeira and Allen, 2007; Schachat et al., 2014, 2015; Xu et al., 2018; MacCracken and Labandeira, 2020; Donovan and Lucas, 2021).
4.2.3 Surface feeding
Definition and natural history. Surface feeding is characterized by partial consumption of the leaf blade thickness. This type of feeding involves delamination or abrasion of one or more but not all tissue layers of a leaf. Often insect surface feeders have a special modification of their mouthparts, such as mandible incisiform teeth that are altered into scooping or abrading structures.
DTs present. DT25 (Figures 6J, K); DT27 (Figures 6P-R); DT29 (Figures 6A-C); DT30 (Figures 6F-I); DT201; and DT238 (Figures 6L-N).
Plant hosts. Pteridosperms: A. conferta (DT201); R. schenkii (DT30); cf. Lodevia sp. (DT238); Sphenopteridium germanicum (DT27, DT29, DT30); N. auriculata (DT25); and Odontopteris sp. 1 (DT25). Sphenopsids: Sphenophyllum sp. 1 (DT25).
Sanzenbacher Ranch DT occurrences. Surface feeding is represented by six out of 48 DTs (12.5%), occurring on seven plant morphotaxa and species (Supplementary Table S4). We recorded a frequency of 10 DT occurrences and 20 FEOs (Table 1). Sphenopteridium germanicum had three DTs; callipterids, medullosans, and sphenopsids presented one DT each. DTs with the most occurrences were DT25 and DT30. Surface feeding represented 1.42% of the total surface area removed and was the eighth most represented functional feeding group (Table 1).
Paleozoic DT occurrences. Surface feeding is present in Gondwanan plant assemblages, and this type of damage is present on Glossopteris, Gangamopteris, Sphenophyllum, and Euryphyllum (Pinheiro et al., 2012; Gallego et al., 2014; Cariglino, 2018; Fernández and Chiesa, 2019). There also are occurrences of surface feeding from Cathaysian plant assemblages on Pecopteris, Nemejcopteris, Pterophyllum, and Taeniopteris (Feng et al., 2021; Ma et al., 2023). For Euramerica, two Late Pennsylvanian plant assemblages have surface feeding damage on Annularia, Alethopteris, Comia, Lilpopia, Macroneuropteris, Neuropteris, Pseudomariopteris, Sigillaria, and Sphenopteridium (Xu et al., 2018; Donovan and Lucas, 2021). Surface feeding was observed in early and early middle Permian plant assemblages from north-central Texas, particularly on Auritifolia, Cathaysiopteris, Evolsonia, Johniphyllum, Taeniopteris, and Zeilleropteris (Beck and Labandeira, 1998; Labandeira and Allen, 2007; Schachat et al., 2014, 2015; Xu et al., 2018; MacCracken and Labandeira, 2020).
4.2.4 Oviposition
Definition and natural history. Although oviposition is not a form of plant consumption, it is included as a nominal functional feeding group due to the considerable use of live plant tissues as a resource and extensive reports in the literature of insect ovipositional damage going back to the Early Pennsylvanian (Béthoux et al., 2004; Labandeira, 2006a; Laaß and Hauschke, 2019). Endophytic oviposition is characterized by the insertion of eggs into the plant by a piercing or more often a slicing ovipositor, producing a lesion with a surrounding scar and internally disturbed tissue. Exophytic oviposition is the deposition of eggs on the plant surface, with minimal, if any, surface damage and is rare in the fossil record. Endophytic oviposition involves penetration of the plant surface between the epidermis and hypodermis and extending to subsurface tissues, or even reaching inner, parenchymatous, and vascular tissue (Renwick and Chew, 1994; Constant et al., 1996).
DTs present. DT72, DT76 (Figures 7F–K, N-Q); DT100; DT101 (Figures 7L, M); DT108; DT245 (Figures 7A-E); and DT294 (Figures 7R-T).
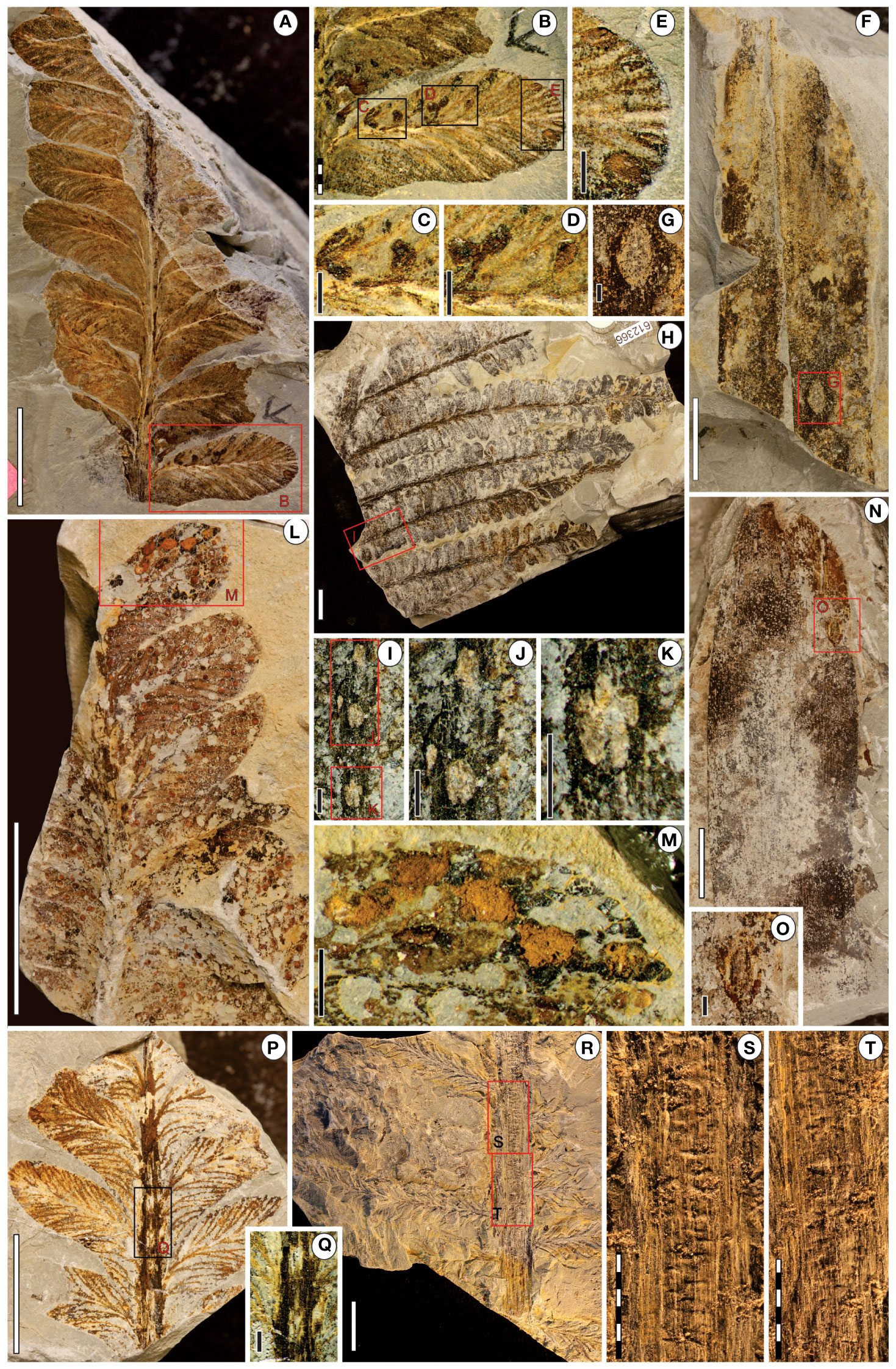
Figure 7 Oviposition (DT76, DT228, DT245, and DT294) from localities USNM 40600, USGS 9999, and NPL 1161. (A) Rhachiphyllum schenkii with DT245 oviposition (USGS 536558), detailed in (B–E) showing ovoidal lesions along the leaf edge. (F) Cordaites sp. 1 with DT76 oviposition (USNM 774293), enlarged in (G), adjacent the leaf midrib. (H) Autunia conferta with DT76 oviposition (USGS 612366), detailed in (I–K) and showing two lesions with prominent reaction scars and lobate interiors with disturbed tissue. (L) Rhachiphyllum schenkii with DT228 oviposition (USGS 774335), detailed in (M) and consisting of a linear series of five ovoidal lesions. (N) Cordaites sp. 1 with DT76 oviposition (USNM 774265), showing a lenticular lesion with a prominent surrounding scar and inner disrupted tissue, detailed in (O). (P) Rhachiphyllum schenkii with DT76 oviposition (USGS 612361), detailed in (Q), displaying two, separate lesions along a midrib. (R) Walchia sp. 1 with DT294 oviposition (NPL TX000001161.80), enlarged in (S, T), displaying interconnected, U-shape lesions along a major branch. Scale bars: white, 1 cm; black and white, 2 mm; black, 1 mm.
Plant hosts. Pteridosperms: A. conferta (DT76, DT100, DT101, DT108) and R. schenkii (DT76, DT245). Coniferophytes: Cordaites sp. 1 (DT54, DT100, DT296); Walchia sp. 1 (DT76, DT294) and miscellaneous axes (DT72).
Sanzenbacher Ranch DT occurrences. Oviposition was observed in seven out of 48 DTs, representing 14.58% of the total DT occurrences across five plant morphotypes and species (Supplementary Table S4). We recorded 10 DT occurrences and 50 FEOs (Table 1). Among these, A. conferta displayed four DTs (DT76, DT100, DT101, and DT108), while Cordaites sp., R. schenkii, and Walchia sp. exhibited two DTs each. Notably, callipterids A. conferta and R. schenkii displayed the highest degree of oviposition, with five and four instances, respectively. DT245 showed the most damage, with three occurrences. Other plant hosts had two or less DTs. Oviposition represented 2.25% of the total surface area removed and ranked as the seventh most represented functional feeding group (Table 1).
Paleozoic DT occurrences. Several authors have reported ovipositional damage from the Gondwanan Glossopteris flora, particularly on Euryphyllum, Gangamopteris, Glossopteris, and Kladistamuos (Adami-Rodrigues et al., 2004; Prevec et al., 2009; McLoughlin, 2011; Srivastava and Agnihotri, 2011; Pinheiro et al., 2012; Cariglino and Gutiérrez, 2011; Gallego et al., 2014; Cariglino, 2018; Edirisooriya et al., 2018; Fernández and Chiesa, 2019). For the Cathaysian flora, authors have reported ovipositional damage in calamitaleans, gigantopterids, and cycadophytes (Feng et al., 2021; Ma et al., 2023). There are reports of oviposition scars in Pursongia and Phylladoderma for the Angaran flora (Vassilenko, 2011; Vassilenko and Karasev, 2020). The most extensive record is for the Euramerican flora. Euramerican ovipositional damage is found in lycophytes, sphenophytes, cycadophytes, medullosans, peltasperms, gigantopterids, and unidentified plant axes (Schachat et al., 2014, 2015; Xu et al., 2018; MacCracken and Labandeira, 2020; Donovan and Lucas, 2021).
4.2.5 Piercing and sucking
Definition and natural history. Piercing-and-sucking insects and mites forage for sap on all plant tissues, targeting vasculature, parenchyma, mesophyll, epidermis, seed embryonic and nutritive tissues, and even protoplasts of spores and pollen (Dolling, 1991; Schuh and Slater, 1995; Wang et al., 2009). After piercing the surface tissue of a plant host with stylate mouthparts, the insect or mite extracts sap in the form of protoplasts within cells or forms a feeding pool into which the sap from damaged tissue is deposited (Labandeira and Phillips, 1996a). The damage caused by piercer and suckers often form patterns that indicate the type of tissue consumed, such as a row of small perforations along a major veins indicating that the mouthpart stylets were accessing phloem and avoiding associated structural tissues (Xu et al., 2018). In contrast to the simple punctures or patterned clusters of punctures mentioned above, a second major type of piercing-and-sucking feeding mode are scale insect impression marks, which are present on plant surfaces and represent direct evidence of the scale insect responsible for the interaction (Harris et al., 2007; Xiao et al., 2021). Many piercing-and-sucking insects are considered specialists in imbibing phloem sap and, in the process, can minimize plant defense responses (Thompson and Goggin, 2006).
DTs present. DT46 (Figures 8A, C-G); DT47 (Figures 8I, J); DT48 (Figures 8K, L); DT211 (Figures 8M, N); DT281; and DT286 (Figures 8O-V).
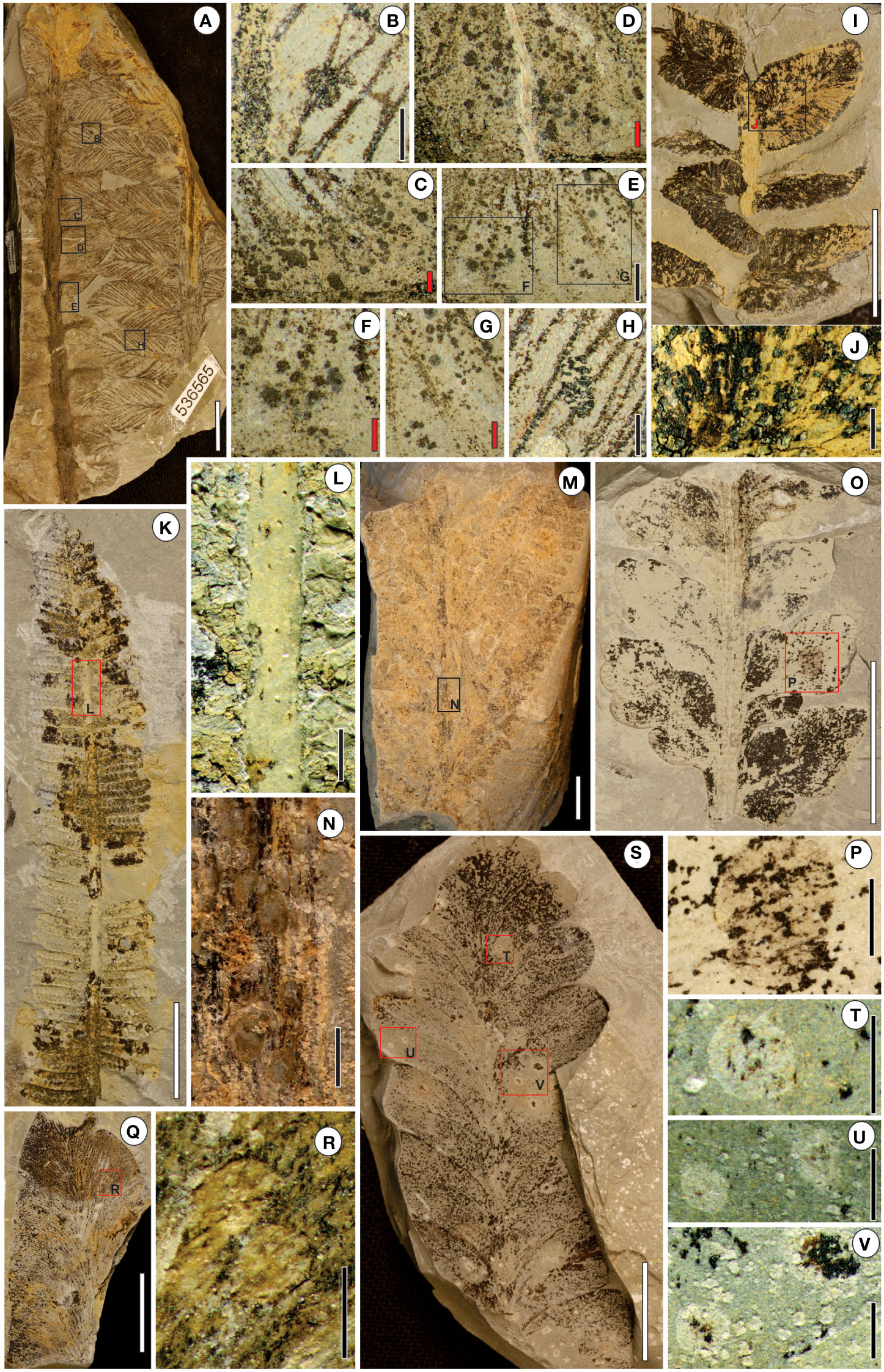
Figure 8 Piercing and sucking (DT46, DT47, DT48, DT211, and DT286) and a gall (DT34) from localities USNM 40600, USGS 9999, and NPL 1161. (A). Autunia conferta with DT34 gall occurring on secondary veins, enlarged in (B) (USNM 536565); DT46 piercing and sucking punctures cratered and circular in cross section, enlarged in (C–G), and DT34 gall enlarged in (H). (I), Neurodontopteris auriculata with DT47 piercing and sucking punctures bulbous and circular in cross section (USGS 536567a), enlarged in (J). (K) Pecopteris sp. 1 with DT48 piercing and sucking punctures elliptical in cross-section (USNM 635868), enlarged in (L). (M) Autunia conferta with DT211, displaying elliptical scale insect impression marks of substantial height (NPL TX000001161.74c), expanded in (N). (O) Sphenopteridium germanicum with DT286, consisting of circular, flat scale insect impression marks (USNM 774420b), an example expanded in (P). (Q) Neurodontopteris auriculata with another example of a DT286 scale insect impression mark (USGS 774516a), but on a different host and expanded in (R). (S) Autunia conferta with DT286 scale insect impression marks (USGS 774579), detailed in (T–V). Scale bars: white, 10 mm: black and white, 2 mm; black, 1 mm; red, 5 μm.
Plant hosts. Pteridosperms: A. conferta (DT46, DT48, DT211, DT286); R. schenkii (DT46, DT286); cf. Lodevia sp. (DT46, DT47); Sphenopteridium germanicum (DT286); N. auriculata (DT47, DT286); O. subcrenulata (DT286); and Sphenopteris sp. 1 (DT46). Coniferophytes: Cordaites sp. 1 (DT47, DT281). Sphenopsids: S. thonii (DT281) and Calamitalean axes (DT48). Ferns: Pecopteris sp. (DT48) and miscellaneous unidentified (DT286).
Sanzenbacher Ranch DT occurrences. Piercing and sucking damage constituted six of the 48 DTs, representing 12.5% of the total occurrences across 12 plant morphospecies (Supplementary Table S2). We recorded 20 DT occurrences and 137 FEOs (Table 1). A. conferta exhibited four DTs (DT46, DT48, DT211, and DT286) and four DT occurrences. This was followed by N. auriculata and cf. Lodevia sp., which had two DTs each (DT47 and DT286, and DT46 and DT47, respectively), and accordingly with three and two instances of those DTs. DT286, a scale insect impression mark, was found on six plant morphotypes and species, five of which were pteridosperms, resulting in six DT occurrences. DT46 was the second most frequently occurring DT, with four instances. The remaining damage was associated with three or fewer DTs. Piercing and sucking represented 6.9% of the total surface area removed and was the fourth most represented functional feeding group (Table 1).
Paleozoic DT occurrences. Piercing-and-sucking damage in Gondwanan floras overwhelmingly targeted of glossopterid foliage and to a lesser extent lycophyte microphylls (Cariglino, 2018; Edirisooriya et al., 2018; Fernández and Chiesa, 2019). By contrast, damage on Cathaysian floras was documented on cycadophytes and gigantopterids (Feng et al., 2021; Ma et al., 2023). Damage has been noted in Late Pennsylvanian plant assemblages of Euramerica (Donovan and Lucas, 2021), occurrences of which have matched the expansion of this functional feeding group mentioned at the end of the Pennsylvanian (Xu et al., 2018). In Euramerica, piercing-and-sucking DTs have been reported from north-central Texas on cycadophytes, peltasperms, gigantopterids, and conifers (Schachat et al., 2014, 2015; MacCracken and Labandeira, 2020).
4.2.6 Galling
Definition and natural history. Gallers are specialized organisms, typically viruses, bacteria, nematodes, and especially mites and insects that cause abnormal swellings or growths in the tissues of plants (Meyer, 1987). Galling insects and mites secrete substances such as amino acids, phenolic compounds, enzymes, and hormones at oviposition sites that result in “abnormal” growths, or teratologies, causing the differentiation of tissues by hypertrophy (increases in cellular size) and hyperplasia (excess proliferation of tissue) (Hori, 1992). This process of induced tissue differentiation results typically in the establishment of four structural elements in a typical insect or mite gall. They are (i) gall vascular tissue that attaches to the vascular tissue of the host plant; (ii) an innermost chamber that houses the galler; (iii) a zone of nutritive tissue that supplies food to the galler; and (iv) an outermost wall of hardened tissue that provides protection to the gall (Dreger-Jauffret and Shorthouse, 1992). Galling organisms overwhelmingly present highly honed, parasitic associations with their host, stimulating the production of excess leaf tissue such that their offspring can develop within, while obtaining food and protection (Maderspacher, 2021).
DTs present. DT34 (Figures 3A, C, F, L; Figures 4L, O; Figures 8A, B, G, H); DT80 (Figures 6A, D, E; Figures 9A-E); DT85 (Figures 9H, I); DT121 and DT125 (Figures 9F, G); DT197, DT247, DT304, and DT415 (Figures 9J-N).
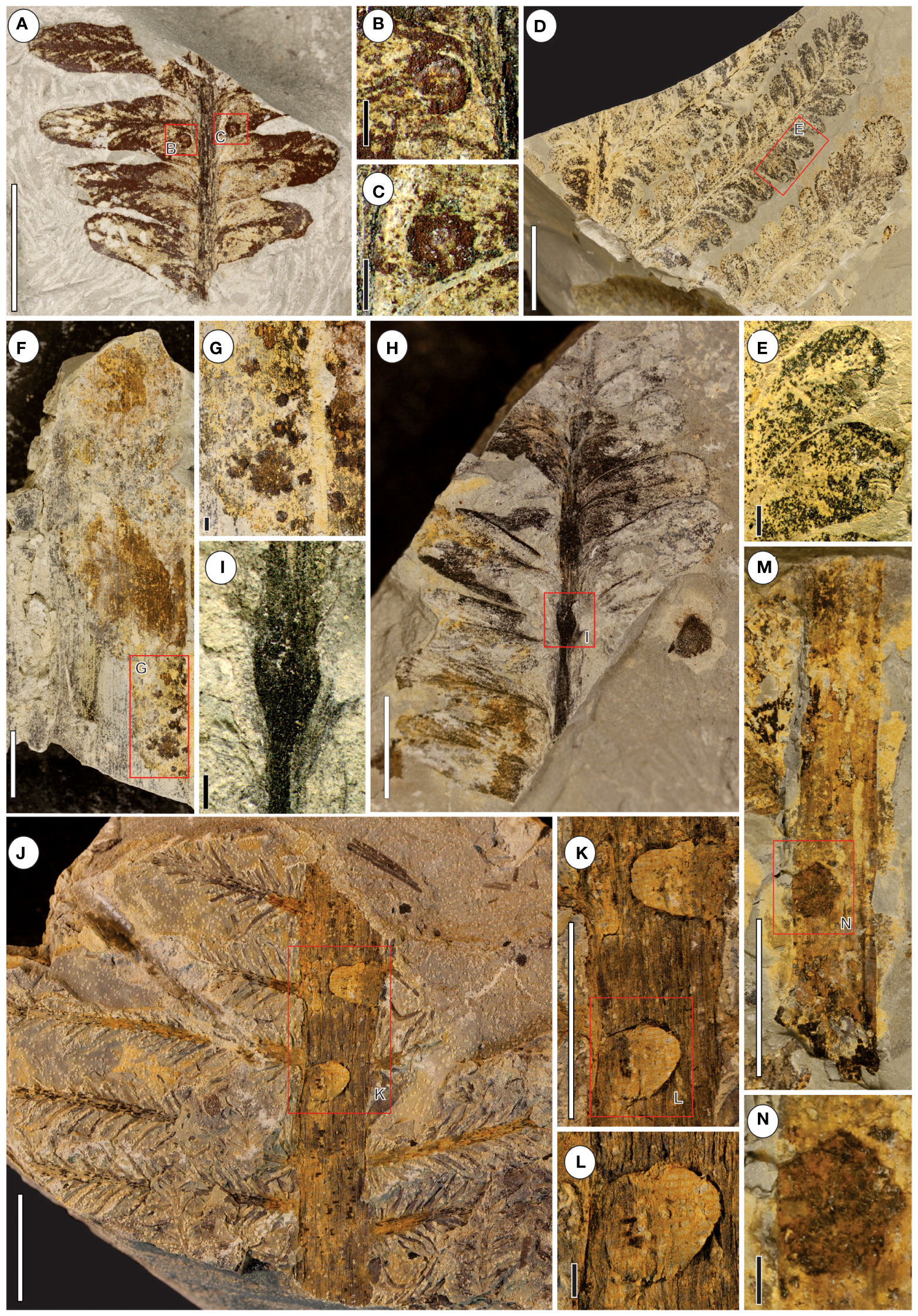
Figure 9 Galling (DT80, DT85, DT125, and DT415) from localities USNM 40600, USGS 9999, and NPL 1161. (A) Rhachiphyllum schenkii with gall DT80 (USNM 774401), enlarged in (B, C). (D) Autunia conferta with DT80 (USGS 774308), three galls of which are enlarged in (E). (F) Cordaites sp. 1with the compound gall DT125 (USNM 774296), enlarged in (G). (H) Rhachiphyllum schenkii with a midrib gall of DT85 (USNM 774218), enlarged in (I). (J) New DT type occurring in Walchia sp. 1, with the new DT415 stem gall (NPL TX000001161.140). showing in enlarged view at (K, L), with center pustules and an outer rim. (M) Neurodontopteris auriculata with new stem gall DT415 (USNM 774274), enlarged in (N) showing rounded exterior rim and pustulose interior. Scale bars: white, 10 mm; black, 1 mm.
Plant hosts. Pteridosperms: A. conferta (DT34, DT80); R. schenkii (DT34, DT80, DT85); cf. Lodevia sp. (DT80); O. subcrenulata (DT197); N. auriculata (DT247, DT415); neuropterid (DT304); cf. Comia sp. (DT80); and S. germanicum (DT80, DT85, DT247). Coniferophytes: Cordaites sp. 1 (DT125) and Walchia sp. 1 (DT415). Sphenopsids: Asterophyllites equisetiformis (DT121); S. thonii (DT34); and a calamitalean stem (DT415).
Sanzenbacher Ranch DT occurrences. Galling was the most diverse functional feeding group recorded for the Sanzenbacher plant assemblage, amounting to nine out of 48 DTs (18.75%) across 13 plant morphotypes and species (Supplementary Table S4). We recorded 31 DTs and 72 FEOs (Table 1). Rhachiphyllum schenkii and S. germanicum had three different DTs each. A. conferta and N. auriculata displayed two different DTs each. DT34 was the most abundant DT with 11 occurrences, followed by DT80 with nine occurrences. Galling represented 7.1% of the total surface area removed and was the third most represented functional feeding group (Table 1). New damage type DT415 (Supplementary Material) is present in the medullosan N. auriculata, the conifer Walchia sp. 1 (Figures 9J-N), and sphenophyte Calamitalean axes, occurring unusually on a broad spectrum of host plants. DT415 is similar to the gall of the modern twig gall wasp (Gross, 2007), although it considerably antedates this lineage.
Paleozoic DT occurrences. The first report of galls, probably of fungal origin, dates back to the Middle Devonian, with the presence of DT32 on a liverwort from Euramerica (Labandeira et al., 2013). In the younger Paleozoic, galls have been reported from Gondwanan, Cathaysian, and Euramerican floras. In Gondwanan deposits, galls are present especially from the late Permian on sphenopsids, ferns, and glossopterids (Adami-Rodrigues et al., 2004; McLoughlin, 2011; Pinheiro et al., 2012; Cariglino, 2018; Fernández and Chiesa, 2019). The presence of galls in the Cathaysian flora ranges from the early to late Permian on sphenophytes, ferns, noeggerathialeans, gigantopterids, and cycadophytes (Liu et al., 2020; Feng et al., 2021; Ma et al., 2023). A variety of galls have been documented in Euramerican plant assemblages from the Middle Devonian to the middle Permian on sphenopsids, ferns, gigantopterids, medullosans, callipterids, and cycadophytes. (Labandeira and Phillips, 1996b; Labandeira and Allen, 2007; Labandeira and Prevec, 2014; Schachat et al., 2014, 2015; Xu et al., 2018; MacCracken and Labandeira, 2020). One notable trend in the Paleozoic gall record is that virtually all Pennsylvanian age galls are on vegetative and reproductive axes of free-sporing plant lineages, whereas Permian galls are overwhelmingly on foliage of seed plants (Labandeira, 2021).
4.2.7 Seed predation
Definition and natural history. Seed predation is characterized by the removal of the internal embryonic and nutritive tissues and external integument of reproductive structures, mostly seeds, causing the inviability or death of the embryo. Seed predation affects the reproductive structure of the targeted plant, causing subsequent changes in the plant distribution and community composition (Janzen, 1971; House and Brust, 1989; Crawley, 2000). Seed predation may occur at the predispersal or postdispersal stages, in which seed mortality rates may range up to 80% in forests and grasslands (Janzen, 1971, 1980). The taxonomic affiliations of seed predators are variable in time and space and likely involved insects such as the paleodictyopteroid Diaphanopterodea during the early Permian (Sharov, 1973; Zherikhin, 2002), trophically analogous to true bugs of the present day (Krugman and Koerber, 1969; Zhang, 1993). Seed predation can result from surface feeders, piercer and suckers, borers, and the activities of oviposition (Dos Santos et al., 2020).
DTs present. DT73 (Figures 10E, F, I); DT74 (Figures 10A-D); and DT124 (Figures 10G, H).
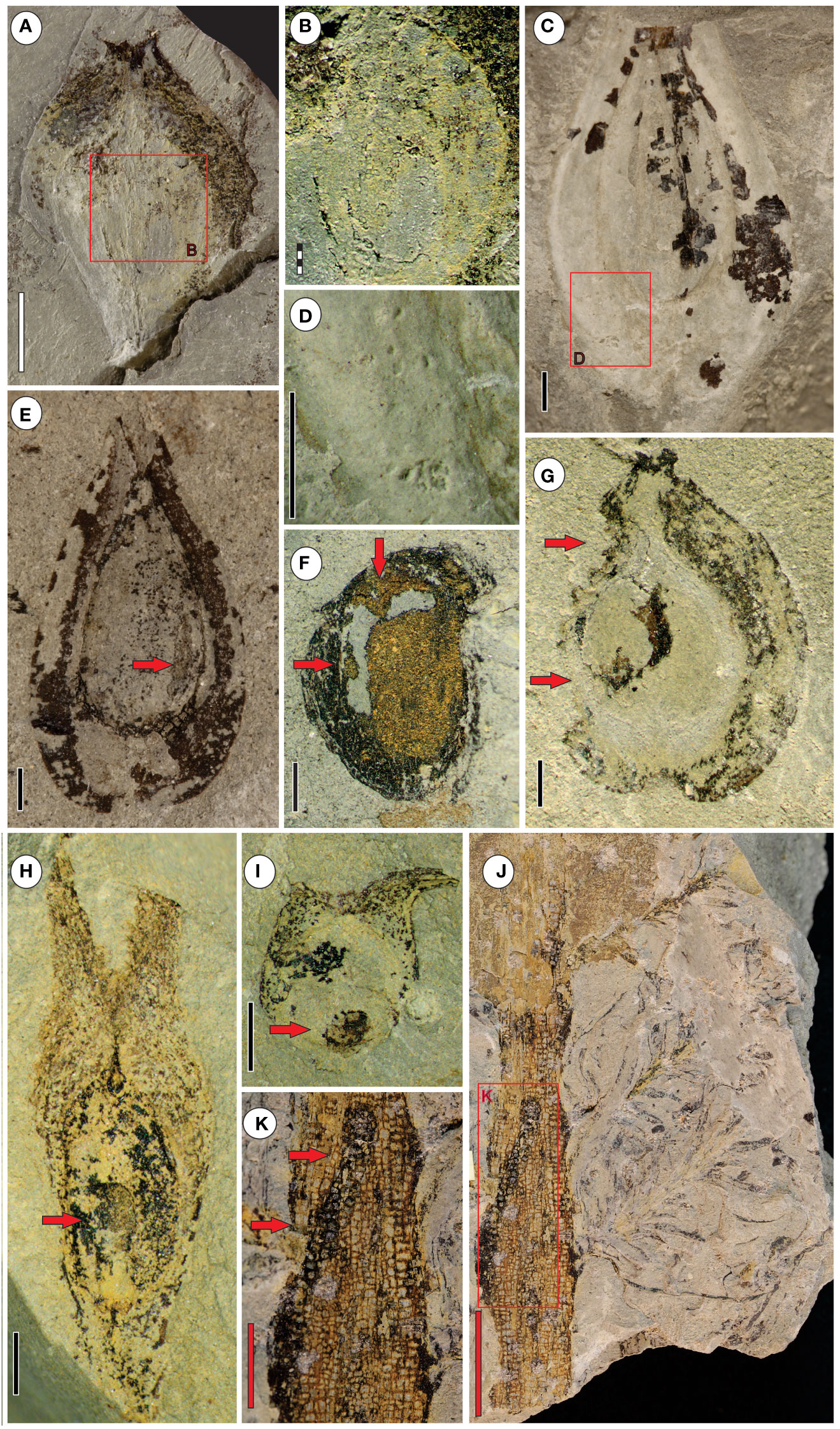
Figure 10 Seed predation (DT73, DT74, and DT124) and borings (DT160) from localities USNM 40600 and USGS 9999. (A) Seed sp. 1 with DT74 (USNM 635902b), detailed in (B), illustrating round punctures. (C) Seed sp. 2 with DT74 (USNM 774260), detailed in (D). illustrating round punctures. (E) Seed sp. 2 with DT73 (USGS 635912a), illustrating a linear cluster of elliptical punctures. (F) Seed sp. 6 with DT73 (USGS 612432g), illustrating dispersed elliptical punctures. (G) Seed sp. 1 with DT124 (USNM 774231b), illustrating the removal of tissues along the seed main body. (H) Seed sp. 9 with DT124 (USNM 794296), illustrating the excavation of tissues within the seed main body. (I) Seed sp. 5 with two occurrences of DT73 (USGS 612432j). (J) Walchia sp. with borings of DT160 (NPL TX000001161.52a), illustrated in (K). Scale bars: red, 10 mm; white, 2 mm; black, 1 mm; black and white, 500 μm.
Plant hosts. Seed sp. 1 (DT74, DT124); seed sp. 2 (DT73, DT74); seed sp. 5 (DT73); seed sp. 6 (DT73); and seed sp. 9 (DT73, DT74, DT124).
Sanzenbacher Ranch DT occurrences. Seed predation is present in three out of 48 DTs (0.06%). We recorded 10 DTs and 31 FEOs (Table 1). Five seed morphotypes exhibited damage; seed sp. 9 displayed three DTs, seed sp. 1 and seed sp. 2 displayed two DTs each, and seed sp. 5 and seed sp. 6 had one DT each. The DTs observed in the seeds were DT73, DT74, and DT124, representing 10 instances of herbivory and predation. DT73 is identified by lenticular to elliptical punctures in the seed body, which is typically are present in the central body, either isolated or overlapping with each other but not present on the wings. DT73 appeared four times in the plant assemblage. DT74 is distinguished by small perforations on the seed main body and wings, consisting of circular to ovate pits with reaction rims. DT74 was present in four instances in the plant assemblage. DT124 is characterized by an uninterrupted removal or major region of embryonic and associated nutritive tissue. Seed predation represents 0.54% of the total surface area removed and the ranked ninth (last) in representation of a functional feeding group (Table 1).
Paleozoic DT occurrences. For the Gondwanan flora, there is a record of seed predation for Cordaicarpus sp., Samaropsis sp., and cupule-attached seeds with DTs 74, 399, 400, and 401 (Dos Santos et al., 2020). There is an extensive record for the Euramerican flora of a variety of predation among seeds such as Trigonocarpus, Samaropsis, unknown spermatophyte seeds, and indeterminate seeds with DT73, DT74, DT124, and DT257 (Sharov, 1973; Jennings, 1974; Scott and Taylor, 1983; Shcherbakov, 2008; Labandeira et al., 2013; Schachat et al., 2014, 2015; Labandeira et al., 2016).
4.2.8 Borings
Definition and natural history. The damage caused by borings is characterized by burrows, tunnels, galleries, and engravings often in wood but also in other tissues such as bark, cambia, pith parenchyma, collenchyma, and sclerenchyma. Insects can consume wood but cannot metabolically produce enzymes to digest cellulose, relying instead on a variety of gut microbes and external fungi to accomplish the decomposition of cellulose. More nutritious sources of food are other, minimally indurated plant tissues such as cambial meristematic tissue between the bark and trunk of seed plants, and pith parenchyma in ferns and monocots (Hickin, 1975; Solomon, 1995). For consumers of wood, phloem, xylem (sapwood), and cambium are the primary sources of nutrition (Bouget et al., 2005).
DTs present. DT160 (Figures 10J, K) and DT284.
Plant hosts. Conifers: Walchia sp. 1; Sphenopsids: Calamites sp. 1; and an unknown wood impression.
Sanzenbacher Ranch DT occurrences. This functional feeding group is represented by two out of 48 DTs (0.04%). We recorded three DTs and three FEOs (Table 1). We report borings in three specimens: one calamitalean, a conifer, and an unidentified wood impression. DT284 appeared twice and DT160 once. Borings represented 2.81% of the total surface area removed and ranked sixth in functional feeding=group representation (Table 1).
Paleozoic DT occurrences. The record of borings is common in three-dimensionally permineralized wood but uncommon in largely two-dimensionally preserved impression and compression material. Although uncommon, Xu et al. (2018) observed DTs of borings in Calamites stems from the latest Pennsylvanian of Texas, including DT284, then a new damage type, also observed in this study. Also occurring in the early Permian of north-central Texas is a record of DT243, consisting of a boring in an indeterminate axis and a petiole (Schachat et al., 2014). For the Cathaysian flora, there is a record of an unassigned boring in a Nemejcopteris sp. axis (Feng et al., 2021).
4.2.9 Pathogens
Definition and natural history. Pathogens principally include viruses, bacteria, oomycetes, nematodes, and especially fungi that cause plant disease and other disorders infecting live plant tissues, Pathogens are considered a form of herbivory (Whyte et al., 2011) in that the necroses infecting live tissue is surrounded by the outer gradational zone of a reaction front, which differs from a distinct, often prominent, reaction rim that ends in a sharp break with adjacent, unaltered tissue. Some fungal pathogens have distinctive fructifications that are placed centrally amid necrotic tissue that also differ in shape, size, and surface ornamentation (Stephenson, 2010). The record of pathogens extends to the Paleozoic (Labandeira and Prevec, 2014), but the richness of pathogens is best developed in Early Cretaceous angiosperm-dominated plant assemblages (Labandeira and Prevec, 2014; Xiao et al., 2022a, b).
DTs present. DT58; DT66 (Figures 4K, M); DT75 (Figures 4I, J); DT97 (Figures 4A, B, G, H); and DT174 (Figures 3A, E, F, J, K; Figures 4C-F).
Plant hosts. Pteridosperms: R. schenkii (DT174); N. auriculata (DT75, DT97); O. subcrenulata (DT75); S. germanicum (DT97); and unidentified pteridosperm (DT58). Conifers: Cordaites sp. (DT58, DT97). Sphenopsids: S. thonii (DT66).
Sanzenbacher Ranch DT occurrences. The pathogens functional feeding group is represented by five out of 48 DTs (10.42%) among seven plant species. We recorded 11 DTs and 35 FEOs (Table 1). We included pathogen damage in this study due to their substantial presence and inclusion in other such studies (Xiao et al., 2022b), although this functional feeding group has not been documented for some Paleozoic plant assemblages from north-central Texas. Pteridosperms were the group that presented the highest instances of pathogen herbivory and were represented by three DTs for R. schenckii, two DTs for N. auriculata, and one DT each for O. subcrenulata and S. germanicum. The most abundant damage was DT174 observed in three R. schenckii specimens, and DT97 noted on Cordaites sp. 1, N. auriculata, and S. germanicum. The other DTs appeared once or twice. Neurodontopteris auriculata and Cordaites sp. 1 were the second group with the greatest DT occurrences, with the necroses of DT97 on both hosts, DT75 on N. auriculata, and DT58 on Cordaites sp. 1. Pathogens represent 23.36% of the total surface area removed and ranked as second in functional-feeding-group representation (Table 1).
Paleozoic DT occurrences. Of the Euramerican flora, the latest Pennsylvanian (Gzhelian) Williamson Drive plant assemblage had the greatest presence of documented pathogens, consisting of DT58, DT75, and DT97 (Xu et al., 2018). The early Permian (Kungurian) Colwell Creek Pond plant assemblage records DT58 and DT97 (Schachat et al., 2014), and a study by Liu et al. (2020) recorded DT97. However, pathogens DT75 and DT97 occur in Middle to Late Pennsylvanian Macroneuropteris scheuchzeri across Euramerica, particularly at the Mazon Creek plant assemblage (Moscovian) in Northwestern Illinois (Scott and Taylor, 1983; Labandeira, 2006a). Donovan and Lucas (2021) documented and considered DT75 and DT97 as a pathogen; all previous studies considered DT75 and DT97 as surface feeding instead of pathogenic in origin.
5 Discussion
5.1 Functional feeding group and DT comparisons among Texan, Paleozoic plant assemblages
This study reports the presence of nine functional feeding groups, and 48 DTs for a plant assemblage with a herbivory index of 0.26. The same functional feeding groups have been described for the nearby early Kungurian, Colwell Creek Pond (CCP) plant assemblage (Figure 11), where margin feeding, hole feeding, surface feeding, piercing and sucking, oviposition, galling, seed predation, and borings were present (Schachat et al., 2014). The Williamson Drive (WD) plant assemblage presented a similar suite of functional feeding groups, except for the absence of seed predation and the additional presence of rare skeletonization (Xu et al., 2018). The WD and CCP plant assemblages consist of two suites that have a high richness of DTs during the late Gzhelian to early Kungurian stages. The Sanzenbacher Ranch (SR) plant assemblage is of intermediate late Asselian age and is consistent with a pattern of a high DT richness from the latest Pennsylvanian to the early Permian.
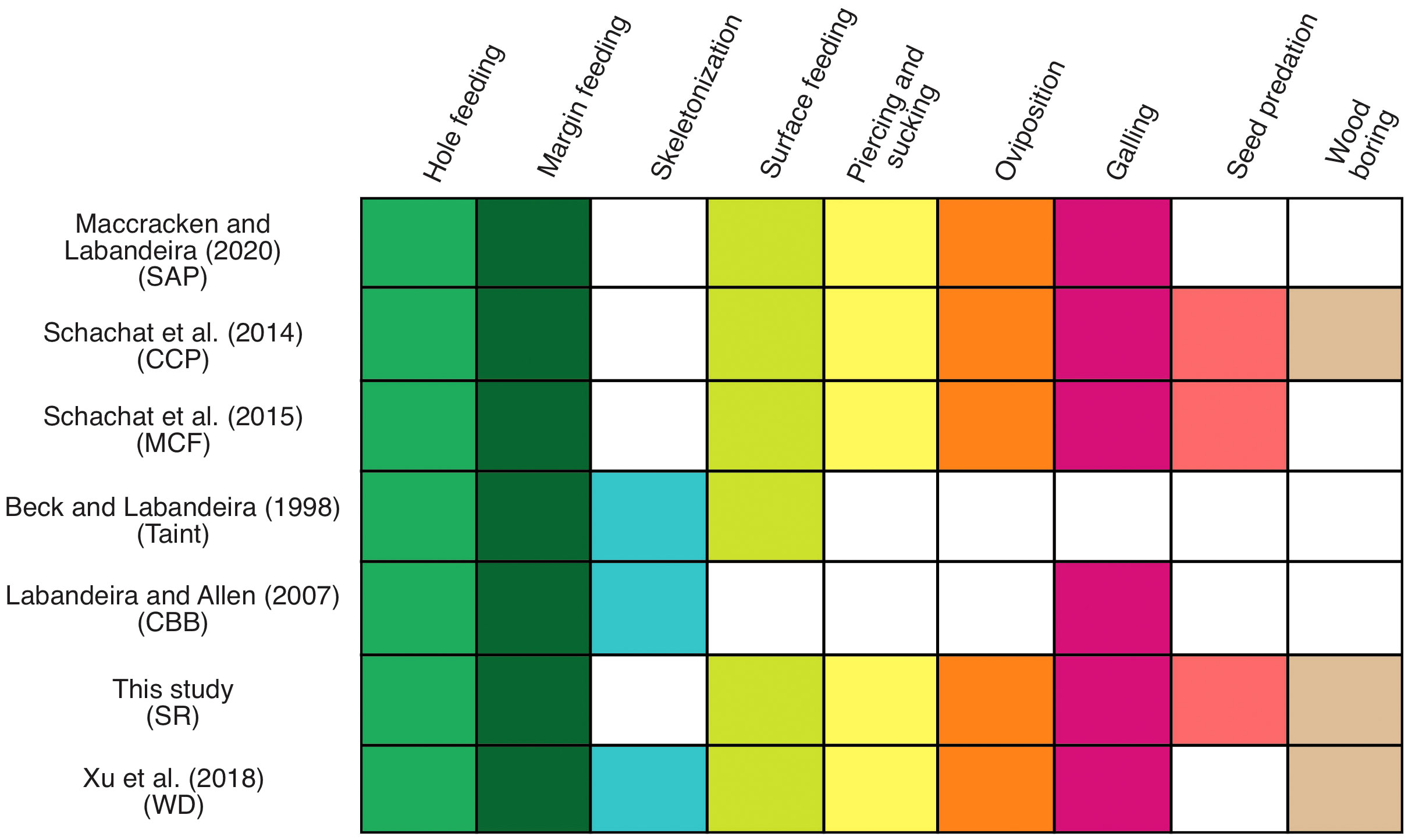
Figure 11 Functional-feeding groups observed for different Paleozoic plant assemblages in north-central Texas. The figure is based on Schachat et al. (2015).
External foliage feeding, consisting of the three functional feeding groups of hole feeding, margin feeding, and surface feeding, constituted the most abundant source of herbivory at SR, compared to the remaining functional feeding groups. Other Permian plant assemblages displayed a similar pattern with these three externally feeding functional feeding groups, although there is a different composition regarding DTs within these plant assemblages. The younger plant assemblages of Mitchell Creek Flats (MCF), CCP, and South Ash Pasture (SAP) present the same external foliage feeding functional feeding groups as shown at SR. Alternatively, the three older plant assemblages of WD, Coprolite Bone Bed (CBB), and Taint displayed the presence of all four functional feeding groups of external foliage feeding, which notably includes skeletonization (Figure 11). There is a similarity regarding the prominent presence of margin feeding in most Permian plant assemblages.
The individual functional feeding groups of external foliage feeding shows several patterns. Margin feeding had the highest scores for the SAP, SR, MCF, and CBB plant assemblages (Table 2). The CBB plant assemblage notably documented 70.07% of all DT occurrences as margin feeding (Labandeira and Allen, 2007). By contrast, the SR plant assemblage had 42.44% of all DT occurrences allocated to margin feeding, accounting for less than half of all DT occurrences. The SAP and MCF plant assemblages had margin feeding percentages of 36.59% and 22.41%, respectively (Schachat et al., 2015; MacCracken and Labandeira, 2020), whereas the CCP and WD plant assemblages displayed 11.08% and 4.04% margin feeding, respectively. For all late Paleozoic plant assemblages, the most common damage was DT12 and DT13, consisting of a broad (level 1) damage-type functional breadth, formerly considered as “generalized”. The updated host specificity categories are defined as follows: broad damage-type functional breadth (level 1), intermediate damage-type functional breadth (level 2), and narrow damage-type functional breadth (level 3), based on a revision of the categories of generalist–intermediate–specialist (Labandeira et al., 2007) used previously. In the present study of SR, we report the presence of DT162 and DT348 on Taeniopteris sp. and N. auriculata, whose damage is classified as level 3, or “specialized”, or more properly narrow damage-type functional breadth. Hole feeding at SR was more representative of the CBB plant assemblage (24.09%), followed by SAP and MCF, which had lower numbers of damaged plants (12.19% and 12.07%). Sanzenbacher Ranch and remaining plant assemblages had values under 9%, indicating that of all the DT occurrences (Table 2), SR had fewest hole feeding DT occurrences compared to margin feeding. Surface feeding had an elevated presence at WD, with 34.19% of all occurrences, contrasting with less hole feeding and margin feeding. The SAP, MCF, and CCP plant assemblages had <10% of all DT occurrences as hole feeding, and the same pattern was reported in this study at SR. Notably, SR, MCF, CCP, and SAP lacked evidence of skeletonization (Figure 4).
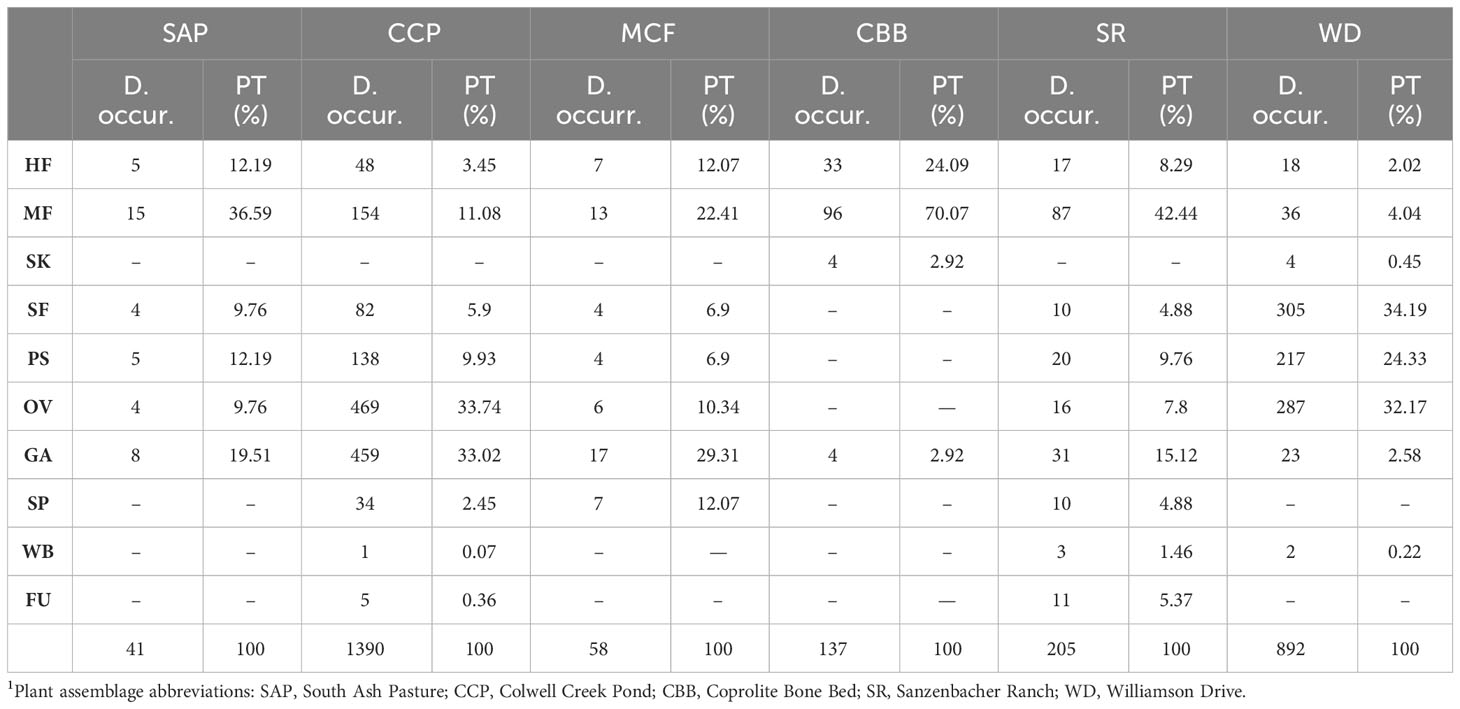
Table 2 Number of the total damage (DT) occurrences for plant assemblages SAP, CCP, MCF, CBB, SR, WD, and their respective percentages from latest Pennsylvanian to early middle Permian plant assemblages from north-central Texas 1.
Contrasting with external foliage feeding, galling for a few plant assemblages was the first or second functional feeding group most represented, based on DT occurrences. The MCF locality had galling that ranked as the first FFG, representing 29.31% of all DT occurrences (Table 2), and also with the greatest richness. Our study showed that galling as the second group in occurrences and the first in DT diversity, accounting for nine out of 48 DTs (18.75%) (Table 1). Schachat et al. (2015) indicated that the high diversity of galls could be indicative of aridification in the MCF environment, and the SR locality has drought-tolerant taxa, such as the conifer Walchia sp., the medullosan Neurodontopteris, and callipterid peltasperms (DiMichele et al., 2018a). Such a condition could be indicative of the expansion of aridification in Euramerica due to glacial–interglacial cycles. The Archer City Formation has a low percentage of carbonates that would have formed the original soil, which may be indicative of soil moisture deficiency and water stress (Griffis et al., 2023). A current example is Neotropical Dry Forest, where there is an association between gall-inducing insects and aridity, involving factors such as ontogenetic stage, plant density, and life forms present in the forest (Cuevas-Reyes et al., 2004). SR had an elevated number of galling DTs in A. conferta and R. schenkii, the most abundant taxa at the locality, which indicate the prevalence of galler arthropods attacking both plant hosts. Additionally, for CCP and SAP, both representing xeromorphic environments, galling was their second most abundant FFG. Concerning the damage at SR, DT34 was the most common gall observed. DT34 damage also was observed at SAP, CCP, and WD. The galls DT85 and DT121 were reported for the CBB plant assemblage (Labandeira and Allen, 2007) and at SR. We observed DT85 on the SR callipterid R. schenkii and lyginopterid S. germanicum, the latter also occurring at the CBB plant assemblage along A. conferta. DT121 was present in the conifer Walchia piniformis at the CBB plant assemblage and also occurs on the calamitalean A. equisetiformis at SR. At SR, a new type of gall on the woody axes of the voltzialean conifer Walchia sp. 1 is DT415 (Supplementary Material). The Taint plant assemblage lacked the galling functional feeding group, in contrast to other Paleozoic plant assemblages in Texas (Figure 11), consistent with its occupation near a stream drainage system.
The piercing-and-sucking functional feeding group expresses the same degree of richness as that observed for surface feeding at SR. We observed that most pteridosperms, including Autunia, Rhachiphyllum, cf. Lodevia sp., Odontopteris, Neurodontopteris, and Sphenopteridium, collectively had 13 DTs, representing 65% of all piercing-and-sucking DTs at SR. Xu et al. (2018) reported the expansion of the piercing-and-sucking functional feeding group during the latest Pennsylvanian corresponds to 24.33% of all DT occurrences at WD. In addition, WD exhibited nine DTs, including distinctive DT286 and DT287 that are scale insect impression marks. At SR, we noticed that DT286 occurred only on pteridosperms, whereas at WD, there is a high incidence of DT286 on sphenophytes. The piercing-and-sucking data at SR is consistent with an expansion of a particular type of herbivory on a variety of taxa during the Pennsylvanian–Permian transition. DTs 46, 47, and 48 are the most common in the Paleozoic plant assemblages and are considered of intermediate damage-type functional breadth. These three DTs are present in several major plant lineages, including lycophytes, sphenophytes, marattialean ferns, gigantopterids, pteridosperms, and coniferaleans. Notably, the Taint and CBB plant assemblages lack this functional feeding group (Figure 11).
The oviposition record is well documented for Paleozoic floras, even though the insertion of eggs into plant tissues is a type of resource use different from feeding. Paleozoic ovipositional damage from Angara, Cathaysia, Euramerica, and Gondwana have been broadly reported (Pinheiro et al., 2012; Vassilenko and Karasev, 2020; Donovan and Lucas, 2021; Ma et al., 2023), and plant assemblages such as CCP and WD are noted for their high level of oviposition, consisting of 33.74 and 32.17%, respectively, of all DT occurrences (Schachat et al., 2014; Xu et al., 2018). The SR plant assemblage has a proportional representation of ovipositional damage comparable to SAP, with somewhat <10% of all DT occurrences (Table 2). Sanzenbacher shares seven oviposition DTs with the other Texan plant assemblages. DT101 is the most common in these Paleozoic floras and is present in almost all the sites, followed by DT76 and DT100. One DT that is exclusive to SR is DT294, which occurs in a Walchia sp. 1 stem.
Seed predation occurs in a few Paleozoic plant assemblages from the Early Pennsylvanian to middle Permian (Sharov, 1973; Jennings, 1974; Scott and Taylor, 1983; Shcherbakov, 2008; Labandeira et al., 2013; Schachat et al., 2014, 2015; Labandeira et al., 2016; Dos Santos et al., 2020). A few studies found a sizeable number of interactions in mostly platyspermic but also cylindrospermic seeds (Schachat et al., 2014, 2015; Dos Santos et al., 2020). DT73 and DT74 has been reported for the MCF and CCP plant assemblages that targeted two and three seed morphotypes, respectively. Additionally, the presence of DT124 was reported for the MCF plant assemblage (Schachat et al., 2015). For SR, predation was noticed on five seed morphotypes. Seed sp. 9 harbored four DTs, the most prominent of which is DT74 with two DT occurrences, seed sp. 1 had DT74 and DT124, and seed sp. 2 exhibited DT73 and DT74. Two other seeds, seed sp. 5 and seed sp. 6, displayed DT73. Although seed predation showed multiple DT occurrences at SR, such interactions have not been recorded at the WD, CBB, Taint, and SAP plant assemblages.
The Paleozoic record of borings is scarce, although there are rare exceptions (Naugolnykh and Ponomarenko, 2010; Feng et al., 2017). Borings have been observed previously at two plant assemblages from the late Paleozoic interval of north-central Texas. Two DTs of borings were documented on axes from the latest Pennsylvanian (Gzhelian) WD plant assemblage (Xu et al., 2018), in which DT284 was present on Calamites sp. 1 and DT160 was present on the conifer Walchia sp.1. We also recorded DT284 on Calamites sp. 1 at SR. Moreover, Schachat et al. (2014) observed that DT243 borings from the early Permian (Kungurian) CCP plant assemblage were present on an unaffiliated plant. This study expands the knowledge of the borings functional feeding group for the early Permian.
Pathogens were not assessed for all late Paleozoic plant assemblages in north-central Texas but were recorded for the WD, CCP, and SR plant assemblages. The oldest locality, WD, established the presence of DT58, and DT75 and DT97 that preferentially occurred on medullosan foliage (Xu et al., 2018). At CCP, DT58 and DT97 were present (Schachat et al., 2014). All three pathogen DTs were present at SR. Donovan and Lucas (2021) recorded DT75 and DT97 pathogen damage at an earlier Late Pennsylvanian assemblage at the Kinney Brick Quarry in New Mexico. It appears that DT75 and DT97 was a persistent pathogen present on seed fern, mostly medullosan, foliage, throughout the Middle Pennsylvanian (Labandeira and Beall, 1990) to earliest Permian.
Comparisons of DTs among these plant assemblages were analyzed using an ordination that was represented by non-metric multidimensional scaling (NMDS) (Figures 11, 12). The results show that, in comparisons of functional feeding groups, the SR plant assemblage is most closely associated with the SAP and MCF plant assemblages. Gigantopterids and callipterids from these three plant assemblages exhibited a strong association with the FFG external foliage feeding and with galling. The high prevalence of galling in these three plant assemblages and their association with habitats where moisture is limiting (Table 3) supports the hypothesis proposed by Fernandes and Price (1988) that harsh environments are occupied by gallers. Subsequent studies by these and other authors (Fernandes and Price, 1992; Price et al., 1998) provide additional support from the modern record that gall richness increases in dry sites compared to riparian sites.
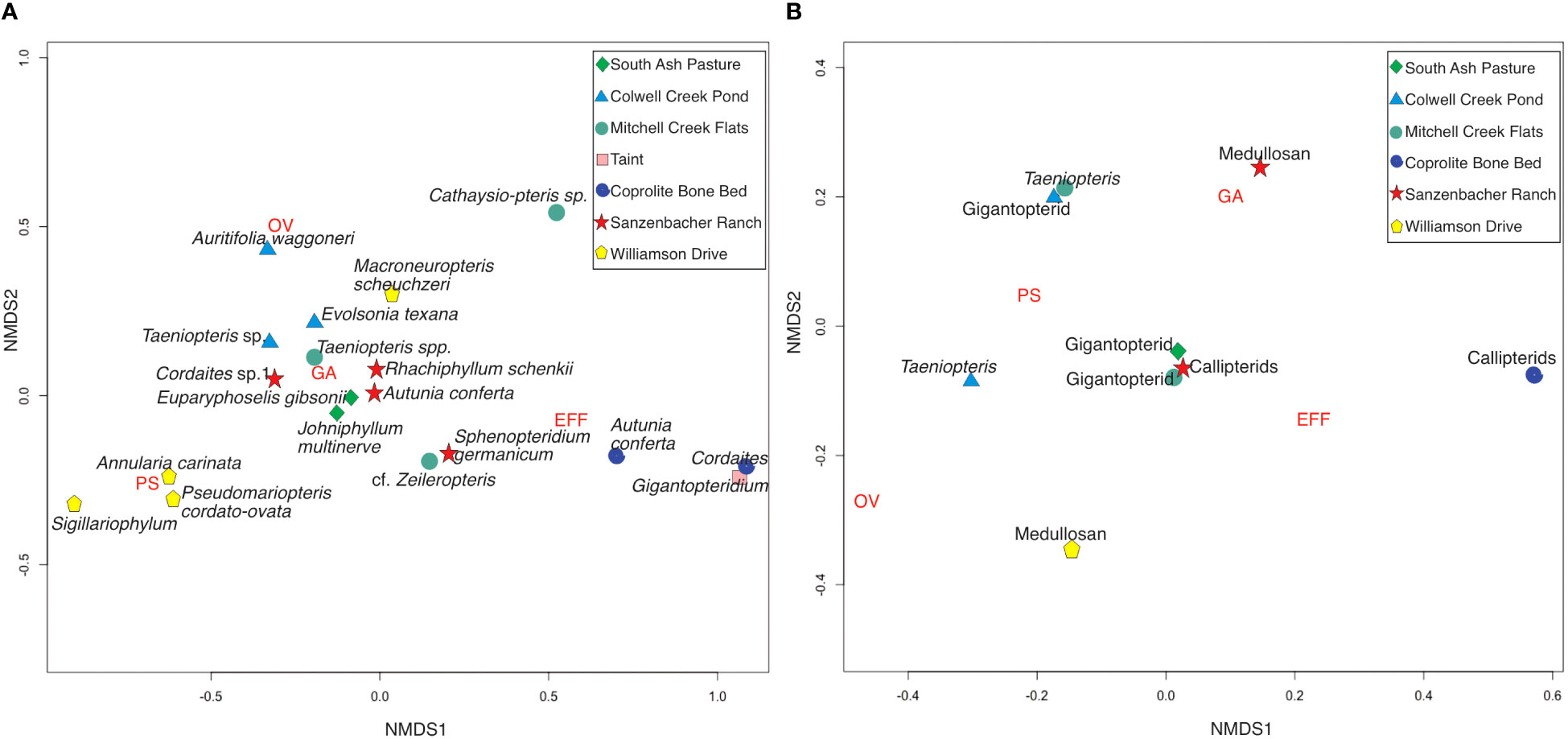
Figure 12 A non-metric multidimensional scaling (NMDS) ordination for the functional feeding groups that were present at the Late Paleozoic in Texas. On the left (A) is an NMDS for the most herbivorized taxa in each plant assemblage. On the right (B) is the most herbivorized taxa for all plant assemblages. Each legend ordinates the plant assemblages from the oldest (bottom) to the youngest (top). Pathogens are not included, as they were not examined and recorded in all plant assemblages.
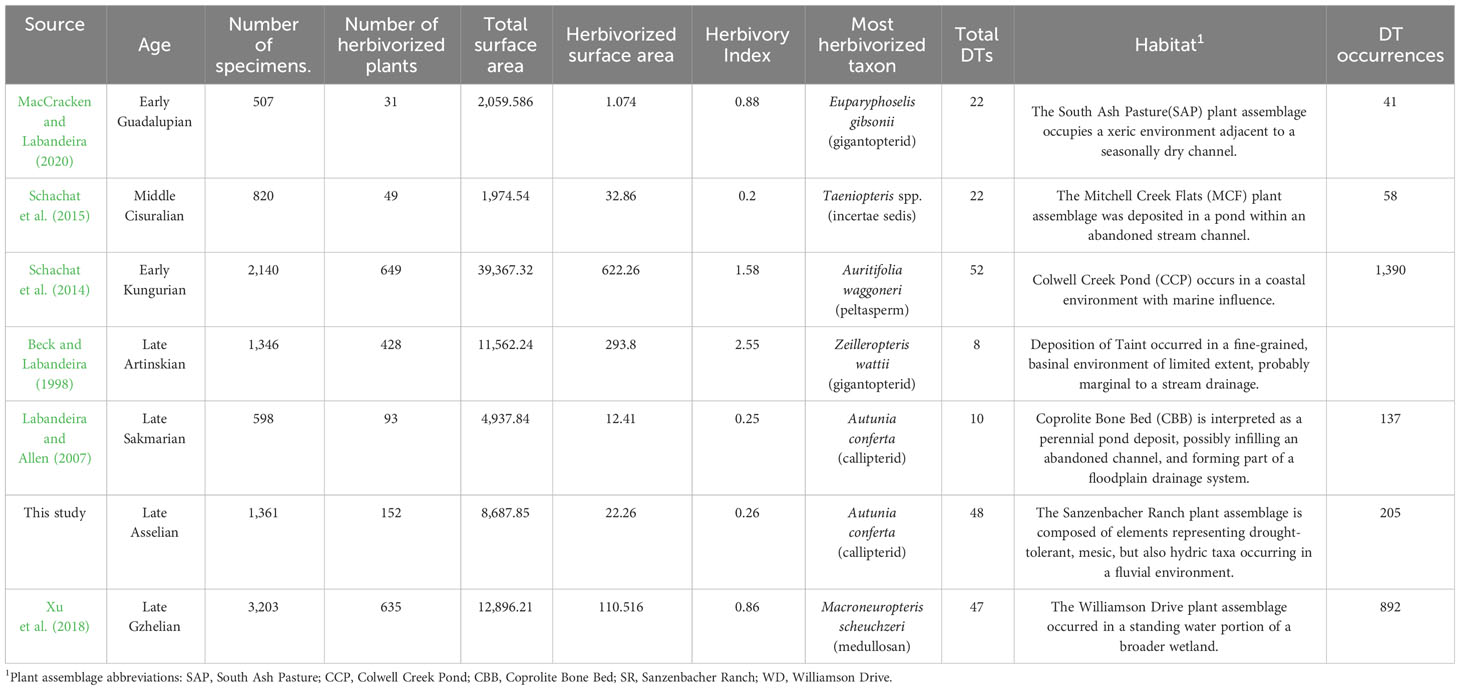
Table 3 Paleozoic plant–insect interaction data recorded from latest Pennsylvanian to early Middle Permian plant assemblages from north-central Texas.
5.2 Possible insect culprits
The only fossil insects known from the Sanzenbacher Ranch locality consists of five wings of cockroaches (Blattodea) in the NPL 1161 collection and one in the USGS 9999 collection, the latter probably representing species of Phyloblatta. In addition, a caterpillar-like holometabolous larva was found that currently remains undescribed.
Insects with mandibulate mouthparts are principally responsible for causing external foliage feeding in plants. Plant damage caused by the functional feeding groups of hole feeders (DT01, DT02, and DT08), margin feeders (DT12, DT14, DT15, DT162, and DT348), and surface feeders (DT25, DT27, DT29, and DT30) could have been inflicted by four major groups of Permian insects. They are (i) orthopteroid insects such as grasshopper-like Oedischiidae of the Orthoptera (Sharov, 1971); (ii) the elongate Protophasmatidae and spinose Geraridae of the “Eoblattida” (Rasnitsyn, 2002); (iii) caterpillar-like, larval holometabolans Metabolarva and Srokalarva (Nel et al., 2013; Haug et al., 2015); and (iv) possibly early beetle lineages such as Tshekardocoleidae and Permocupedidae (Ponomarenko, 1969). The first three of these groups possessed downwardly directed (hypognathous) mouthparts typically associated with herbivory, unlike the forwardly directed (prognathous) mouthparts of early Permian predatory insects (Labandeira, 2019). More direct evidence of a possible culprit is the three-dimensionally preserved caterpillar-like larva, a likely herbivore, found in the NMNH Sanzenbacher Ranch collection. The dietary spectrum of early beetle lineages is less clear; virtually, all Permian lineages have been associated with wood (Zhao et al., 2021), a conclusion buttressed by distinctive galleries present in late Permian conifer wood (Feng et al., 2017). However, although the associations of beetles with wood during the Permian often have been noted (Zhao et al., 2021), there is evidence that some beetle taxa consumed live and dead plants (Dmitriev and Ponomarenko, 2002).
The Pennsylvanian and Permian record of endophytic oviposition is extensive, and several insect taxa have been suggested as culprits. Based on external ovipositor structure and the size and shape of oviposition lesions on plant surfaces (Lin et al., 2019), several groups of early Permian ovipositing insects are implicated (Lin et al., 2019). Evidence indicates that endophytic oviposition was inflicted by insects of medium to large size whose females possessed large, piercing or slicing ovipositors (Bechly, 2001), often blade-like and with sawtooth edges. Damage of larger, lenticular lesions (DT72, DT76) are consistent with slicing ovipositors. The distinctive damage pattern of DT100 is similar to the gymnosperm leaf Pursongia and a related species described from the Permian of Russia and attributed to kennedyine dragonflies (Meganeurina: Kennedyidae), ecological analogs of certain extant damselflies (Odonata: Zygopteran) (Vassilenko, 2011; Aristov et al., 2013). The structure of DT108, representing coalesced, large, circular to polylobate lesions, likely represents a damage from the slicing ovipositor of a medium sized orthopteran insect such as the Oedischiidae (Sharov, 1971). The small, ovoidal lesions of DT228 and DT245 are best attributed to Archescytinidae, an early Permian hemipteran lineage that possessed small, piercing, ovipositors (Shcherbakov and Popov, 2002).
The piercing-and-sucking functional feeding group expanded during the Late Pennsylvanian (Xu et al., 2018). This expansion included newly emergent lineages such as Archescytinidae, Prosbolidae, Prosbolopseidae, Coleoscytidae, and Ingruidae, insects with stylate mouthparts and body lengths of generally 4–10 mm (Shcherbakov and Popov, 2002). These five lineages were capable of producing the single punctures of DT46, DT47, and DT48, or the clustered pattern of DT281. By contrast, the fabricators of the scale insect impression scars of DT211 and DT286 remain a mystery, as they do for similar impression scars for the three-million-year earlier, latest Pennsylvanian, WD plant assemblage (Xu et al., 2018). DT211 occurs on foliar midveins, is ellipsoidal, and shows substantial height; by contrast, DT286 occurs on a leaf blade, is circular, and flat. Both DTs are morphologically similar to various modern soft scales (Hemiptera: Coccidae), although the earliest occurrence of the more inclusive clade of scale insects, Coccomorpha, has an earliest body-fossil occurrence in the Late Triassic (Shcherbakov and Popov, 2002).
Galls on plants have been recorded since the Middle Devonian (Labandeira and Prevec, 2014), and additional gall DTs have been reported in several late Paleozoic plant assemblages (Labandeira, 2021), The nine gall DTs at Sanzenbacher Ranch is one of the most diverse for the Paleozoic and exhibits the general shift of galls being hosted on plant axes during the Pennsylvanian to being hosted on plant foliage during the Permian (Labandeira, 2021). Sanzenbacher galls can be divided into axial galls (DT121 and DT415), generalized, featureless galls (DT34, DT80, and DT125), midrib galls (DT85 and DT304), and galls with distinctive features such as ornamentation (DT197 and DT247). The gall with the greatest resolution as to a culprit is DT121 (Labandeira and Allen, 2007), a conifer gall attributable to a pine aphid (Hemiptera: Adelgidae) or precursor lineage similar to the extant Cypress Twig Gall Midge (Lynch, 2017). Most of the generalized, featureless galls—DT34, DT80, and the compound gall DT125—likely were made by small, galling hemipterans such as Protopsyllidiidae, or by thripids such as Lophioneuridae that had the requisite small bodies important for galling during the Permian (Schachat and Labandeira, 2015).
Seed predation damage has been reported in Gondwanan and Euramerican floras during the Pennsylvanian and Permian (Zherikhin, 2002; Labandeira, 2006b; Dos Santos et al., 2020). Suggested culprits for DT73 and DT74 are hemipterans such as Archescytinidae and Protopsyllidiidae, the thripid Lophioneuridae, and the permothemistid Diathemidae, a Paleodictyopteroidea lineage of small body size that evolved stylate mouthparts and penetrating ovipositors distinct from Hemipteroidea (Labandeira, 2006b; Schachat and Labandeira, 2015; also see Sharov, 1973). The culprit for DT124 possibly is an early beetle lineage (Coleoptera: Tshekardocoleidae), based on the damage that indicates a chewing insect with robust, prognathous, mandibulate mouthparts (Ponomarenko, 1969) primarily may have had habits of boring into wood (xylophagy) (Zhao et al., 2021).
Other than small oribatid mites, insects producing borings into early Permian wood of cordaites and conifers, larger borings almost always would involve larger archostematan beetles (Zherikhin, 2002; Zhao et al., 2021). DT160, consisting of linear tunnels approximately circular in cross section and packed with frass, and DT284 consisting of more irregular galleries with adjacent tunnels bordered by partitions, likely involved culprits such as Tshekardocoleidae and Permocupedidae (Zhao et al., 2021). The consistency of wood tunneled by beetles would range from punky and in the initial stages of decomposition (Naugolnykh and Ponomarenko, 2010), to highly indurated secondary xylem (Feng et al., 2017).
Pathogens are poorly represented in the Permian, and little modern evidence has been provided for their taxonomic identifications. Most evidence for pathogenic damage comes from glossopterid plant assemblages of the late Permian (Labandeira and Prevec, 2014). At SR, DT66 and DT174 are distinctive as they house fructifications. The circular pattern of DT66 is similar to the ascomycotan Tar Spot Disease (Rhytismatales: Rhytismataceae) (Conrad, 2009), although this pattern of three to four concentric rings of miniscule fructifications is not well developed. The distinctive ovoidal blotch of DT174 that contains a central ring of small fructifications is reminiscent of a basidiomycotan rust fungus (Uredinales: Phragmidiaceae) that occurs on a variety of modern angiosperm hosts (Coombs, 2018). The remaining pathogens—DT58, DT75, and DT97—have minimal identifying features and can be attributed to various unidentifiable leaf necroses such as leaf spot and leaf blight.
5.3 Damage-type richness and intensity in Late Paleozoic, Texan plant assemblages
This study recorded 48 DTs for the SR plant assemblage. Compared to other late Paleozoic plant assemblages from north-central Texas, we noticed a high DT richness at SR. Such elevated richness also was observed for the CCP and WD plant assemblages (Schachat et al., 2014; Xu et al., 2018), where the presence of 52 and 47 DTs, respectively, was reported. Nonetheless, by comparison, a considerably lower number of DTs were recorded for the remaining plant assemblages, ranging between 8 and 22 DTs (Beck and Labandeira, 1998; Labandeira and Allen, 2007; Schachat et al., 2015; MacCracken and Labandeira, 2020). Late Pennsylvanian to early middle Permian plant assemblages presented higher levels of DT occurrences, as observed at WD with 892 and at CCP with 1,390 DT occurrences (Schachat et al., 2014; Xu et al., 2018). By contrast, SR had 205 DT occurrences, and CBB, of similar age, reported 137 DT occurrences. The SAP and MCF plant assemblages showed 41 and 58 DT occurrences, respectively. Studies conducted in modern forests show that environmental conditions and plant community structure could influence damage composition, referred to as herbivorous diversity (Cuevas-Reyes et al., 2004; Espírito-Santo et al., 2012). A study published by Leal et al. (2016) showed that, in modern dry tropical forests, the vegetation structure can largely define the herbivore composition in terms of richness and abundance, which is based on tree richness.
There are three discrete patterns defined by the herbivory index (HI) for the seven plant assemblages (Table 3). First, plant assemblages with an HI of 0.2%–0.3% are at SR, CBB, and MCF and have a taeniopterid or a callipterid as the most herbivorized plant host. Second, plant assemblages with an HI of 0.8%–0.9% are WD and SAP and have a gigantopterid or medullosan as the most herbivorized plant host. Third, plant assemblages with an HI of 1.5%–2.6% are Taint and CCP and have a gigantopterid or a peltasperm as the most herbivorized plant host. In all instances, these data indicate that seed plants are the most herbivorized host, and overall environmental conditions, especially access to water sources, may be the prime determinant of the HI.
5.4 Most herbivorized plant hosts and their environments
The seven plant assemblages from the latest Pennsylvanian to early middle Permian of north-central Texas exhibit similarities and differences regarding the most herbivorized taxa (Table 3). For the early Permian plant assemblages of SR and CBB, a callipterid and A. conferta are the most herbivorized taxa. At CBB A. conferta accounted for 335 specimens, 85 of which express at least one instance of herbivory, representing 91.4% of the total herbivorized specimens of the plant assemblage (Labandeira and Allen, 2007). For SR, we observed 174 specimens, 42 of which show herbivory, representing 27.63% of the plant specimens. When comparing herbivory on Autunia, the values are similar between the two plant assemblages: CBB shows 25.37% of the specimens were herbivorized, and SR shows 24.13% of the specimens have been herbivorized. Labandeira and Allen (2007) documented at CBB the functional feeding groups of hole feeding, margin feeding, skeletonization, and galling for A. conferta, with an herbivorized area of 10.32 cm2 compared to a total foliar area of 2420.34 cm2. In contrast, at the SR plant assemblage, A. conferta exhibited the functional feeding groups of hole feeding, margin feeding, surface feeding, piercing and sucking, oviposition, and galling, with an herbivorized area of 6.11 cm2 out of a total surface area of 5,212.77 cm2. The difference in total herbivorized area between these plant assemblages could be attributed to a wide variation in the DT composition of damage. Whereas CBB showed a higher presence of functional feeding groups such as margin feeding and hole feeding, the SR plant assemblage had a small number of DTs attributable to piercing and sucking and oviposition (Supplementary Table S4). Additionally, the second most herbivorized taxa in our study was the callipterid R. schenkii, representing 15.79% of the total herbivorized specimens. The functional feeding groups observed on R. schenkii were hole feeding, margin feeding, surface feeding, piercing and sucking, oviposition, galling, and pathogens (Supplementary Table S4). Callipterids, such as Autunia, can have fronds up to 80 cm long (Kerp, 1988) and a thick cuticle in the adaxial portion that thins out considerably toward the abaxial region with the added presence of epidermal papillae (Kerp, 1988; Kerp and Barthel, 1993). The presence of thick cuticle and papillae could be protection from herbivory by arthropods and pathogens (Glover, 2000; Peeters, 2002).
Medullosans were extensively herbivorized at the latest Pennsylvanian WD plant assemblage. This plant assemblage featured Macroneuropteris scheuchzeri as the most herbivorized plant host, comprising 52.44% of all interactions (Xu et al., 2018). The authors reported for M. scheuchzeri a diversity of functional feeding groups that encompassed all four subgroups of external foliage feeding, and piercing and sucking, oviposition, galling, and pathogens. Pathogens were developed on M. scheuchzeri that included especially an elevated presence of DT75 and DT97. In our examination of SR, Neurodontopteris auriculata was the most herbivorized medullosan. As observed previously (Xu et al., 2018), DT75 and DT97 were also documented on this plant host (Supplementary Table S6). Whereas we assigned these DTs to pathogen damage based on the latest version of the Damage Guide (Labandeira et al., in prep), Xu et al. (2018) mentioned in their study that both DTs were probably caused by a combination of factors and were the result of an opportunistic pathogen.
The MCF and CCP plant assemblages show that the abundantly represented Taeniopteris spp. as the most herbivorized group of plants (Schachat et al., 2014, 2015). SR had five taeniopterid specimens, two of which showed at least one instance of herbivory. We observed one specimen with DT02 hole feeding and the other specimen with DT162 margin feeding. DT02 was recorded at the CPP and MCF plant assemblages, and DT162 is the first instance of this DT reported for a Paleozoic assemblage.
The late Artinskian and the early Guadalupian plant assemblages of Taint and SAP present gigantopterids as the most herbivorized taxa (Figure 1, Table 3). The Taint plant assemblage shows that Zeilleropteris wattii (Beck and Labandeira, 1998) and the SAP plant assemblage shows that Euparyphoselis gibsonii (MacCracken and Labandeira, 2020) were extensively herbivorized. In contrast, the SR plant assemblage lacked gigantopterids, although a similar level of overall herbivory was observed, similar to the latest Pennsylvanian WD and the late Sakmarian CBB plant assemblages (Labandeira and Allen, 2007; Xu et al., 2018) (Figure 1). Apparently, gigantopterids were not abundant during the early Permian in Euramerica but became more abundant in its southwestern region during the uppermost Sakmarian to the earliest Guadalupian (Mamay, 1988; Mamay et al., 1988; DiMichele et al., 2005; DiMichele et al., 2018b).
Herbivore preference for targeting and preferring specific taxa could be associated with the presence of particular plant groups in certain environments. Callipterids and medullosans were abundant at the end of the Pennsylvanian and beginning of the Permian, as observed in this study and in other plant assemblages (Labandeira and Allen, 2007; DiMichele et al., 2013, 2015; Xu et al., 2018; DiMichele et al., 2018a; Luthardt et al., 2021). From the Sakmarian Stage onwards, there is a noticeable shift in both the plant assemblages and in the environments they inhabited (Table 3). This transition shifted from humid to seasonally dry environments (DiMichele et al., 2004; MacCracken and Labandeira, 2020), a shift that increased the diversity and abundance of gigantopterids and accordingly their arthropod herbivores.
6 Conclusions
The early Permian (Asselian) Sanzenbacher Ranch plant assemblage from the north-central region of Texas is composed of drought-tolerant taxa. This plant assemblage primarily consists of callipterid peltasperms and other pteridosperms, along with calamitaleans, coniferophytes, and an abundance of reproductive organs. We recorded the nine functional feeding groups of hole feeding, margin feeding, surface feeding, piercing and sucking, oviposition, galling, seed predation, borings, and pathogens, collectively consisting of 48 distinctive damage types (DTs). In contrast to other late Paleozoic plant assemblages, the Sanzenbacher Ranch plant assemblage lacks evidence of skeletonization. The NMDS analysis revealed that the composition of functional feeding groups at Sanzenbacher Ranch is closely associated with the South Ash Pasture and Mitchell Creek Flat plant assemblages, exhibiting a high prevalence of external foliage feeding and galling. The Sanzenbacher Ranch plant assemblage demonstrates a high richness of DTs, similar to that observed in the series of latest Pennsylvanian to early Kungurian plant assemblages at, for example, Williamson Drive and Colwell Creek Pond. There is a notable diversity of galls at the Sanzenbacher Ranch plant assemblage, consistent with other seasonally dry plant assemblages in xeric environments such as Mitchell Creek Flats. This study contributes to an understanding of how changes in arthropod and pathogen interactions with plants during this time interval were likely attributable to changes in the environments caused by glacial–interglacial cycles.
Data availability statement
The original contributions presented in the study are included in the article/Supplementary Material. Further inquiries can be directed to the corresponding author.
Author contributions
TB: Conceptualization, Data curation, Formal analysis, Investigation, Writing – original draft, Writing – review & editing, Funding acquisition. CL: Conceptualization, Formal Analysis, Funding acquisition, Supervision, Writing – review & editing. ER: Formal analysis, Writing – review & editing. RI: Formal analysis, Funding acquisition, Supervision, Writing – review & editing.
Funding
The author(s) declare financial support was received for the research, authorship, and/or publication of this article. This work was funded by the Co-ordination for the Improvement of Higher Education Personnel (CAPES process 88887.584278/2020-00), the National Council for Scientific and Technological Development (CNPq process 141156/2020-1 and PQ 313946/2021-3), and The Benson Fund Grant provided by the Paleobiology Department (Smithsonian National Museum of Natural History).
Acknowledgments
We thank W. A. DiMichele for permitting access to the Sanzenbacher Ranch collection, his help in plant identifications, providing information about the locality, and reviewing the manuscript. Thanks go to L. Boucher for all her support during the visit to the Non-Vertebrate Laboratory fossil plant collection at the University of Texas in Austin. We thank Renan S. Pittella for helping with the script for the statistical analysis. All the text was generated by the author, and we used artificial intelligence (AI) to correct potential English mistakes.
Conflict of interest
The authors declare that the research was conducted in the absence of any commercial or financial relationships that could be construed as a potential conflict of interest.
Publisher’s note
All claims expressed in this article are solely those of the authors and do not necessarily represent those of their affiliated organizations, or those of the publisher, the editors and the reviewers. Any product that may be evaluated in this article, or claim that may be made by its manufacturer, is not guaranteed or endorsed by the publisher.
Supplementary material
The Supplementary Material for this article can be found online at: https://www.frontiersin.org/articles/10.3389/fevo.2024.1368174/full#supplementary-material
References
Adami-Rodrigues K., Iannuzzi R., Pinto I. D. (2004). Permian plant–insect interactions from a Gondwana flora of southern Brazil. Foss. Strata 51, 106–125. doi: 10.18261/9781405169851-2004-07
Aristov D. S., Bashkuev A. S., Golubev V. K., Gorochov A. V., Karasev E. V., Kopylov D. S., et al. (2013). Fossil insects of the middle and upper Permian of European Russia. Paleontol. J. 47, 641–832. doi: 10.1134/S0031030113070010
Bechly G. (2001). Die faszinierende Evolution der Insekten. Stutt. Beitr. Naturk. C 49, 1–94. Available at: https://bechly.lima-city.de/C49.pdf.
Beck A. L., Labandeira C. C. (1998). Early Permian insect folivory on a gigantopterid-dominated riparian flora from north-central Texas. Palaeogeogr. Palaeoclim. Palaeoecol. 142, 139–173. doi: 10.1016/S0031-0182(98)00060-1
Béthoux O., Galtier J., Nel A. (2004). Earliest evidence of insect endophytic oviposition. Palaios 19, 408–413. doi: 10.1669/0883-1351(2004)019<0408:EEOIEO>2.0.CO;2
Bouget C., Brustel H., Nageleisen L. M. (2005). Nomenclature des groupes écologiques d’insectes liés au bois: synthèse et mise au point sémantique. Compt. Rend. Biol. 328, 936–948. doi: 10.1016/j.crvi.2005.08.003
Cariglino B. (2018). Patterns of insect-mediated damage in a Permian Glossopteris flora from Patagonia (Argentina). Palaeogeogr. Palaeoclim. Palaeoecol. 507, 39–51. doi: 10.1016/j.palaeo.2018.06.022
Cariglino B., Gutiérrez P. R. (2011). Plant–insect interactions in a Glossopteris flora from the la Golondrina Formation (Guadalupian –Lopingian), Santa Cruz Province, Patagonia, Argentina. Ameghiniana 48, 103–112. doi: 10.5710/AMGH.v48i1(321)
Conrad J. (2009). Excerpts from Jim Conrad’s Naturalist Newsletter – Fungal “Black Dots” on Leaves. Available at: http://www.backyardnature.net/n/x/tar-spot.htm (Accessed March of 2018).
Constant B., Grenier S., Febvay G., Bonnot G. (1996). Host plant hardness in oviposition of Macrolophus caliginosus (Hemiptera: Miridae). J. Econ. Entomol. 89, 1446–1452. doi: 10.1093/jee/89.6.1446
Coombs E. (2018). IPM Images – bramble leaf rust (Phragmidium violaceum). Available at: https://www.ipmimages.org/browse/detail.cfm?imgnum=5435665 (Accessed November of 2019).
Crawley M. J. (2000). “Seed predators and plant population dynamics,” in Seeds: The Ecology of Regeneration in Plant Communities. Eds. Fenner M. (CABI, Wallingford), 167–182.
Cuevas-Reyes P., Quesada M., Hanson P., Dirzo R., Oyama K. (2004). Diversity of gall-inducing insects in a Mexican tropical dry forest: the importance of plant species richness, life-forms, host plant age and plant density. J. Ecol. 92, 707–716. doi: 10.1111/j.0022-0477.2004.00896.x
DiMichele W. A., Cecil C. B., Chaney D. S., Elrick S. D., Lucas S. G., Lupia R., et al. (2011). “Pennsylvanian–Permian vegetational changes in tropical Euramerica,” in Harper J. A. (Ed.) Geology of the Pennsylvanian–Permian in the Dunkard Basin: Guidebook, 76th Annual Field Conference of Pennsylvania Geologists, Washington, D. C., 60–102.
DiMichele W. A., Chaney D. A., Falcon-Lang H., Kerp H., Looy C. V., Lucas S. G., et al. (2015). “A compositionally unique voltzian conifer-callipterid flora from a carbonate-filled channel, Lower Permian, Robledo Mountains, New Mexico, and its broader significance,” in Carboniferous-Permian Transition in the Robledo Mountains, Southern New Mexico, vol. 65 . Eds. Lucas S. G., DiMichele W. A. (Albuquerque: New Mexico Mus. Nat. Hist. Sci. Bull), 123–128.
DiMichele W. A., Hook R. W., Kerp H., Hotton C. L., Looy C. V., Chaney D. S. (2018a). “Lower Permian Flora of the Sanzenbacher Ranch, Clay County, Texas,” in Transformative Paleobotany. Eds. Krings M., Harper C. J., Cúneo N. R., Rothwell G. W. (Elsevier), 95–126. Available at: https://www.sciencedirect.com/science/article/abs/pii/B9780128130124000061
DiMichele W. A., Hook R. W., Nelson W. J., Chaney D. S. (2004). An unusual Middle Permian flora from the Blaine Formation (Pease River Group: Leonardian–Guadalupian Series) of King County, West Texas. J. Paleontol. 78, 765–782. doi: 10.1666/0022-3360(2004)078<0765:AUMPFF>2.0.CO;2
DiMichele W. A., Kerp H., Krings M., Chaney D. (2005). The Permian peltasperm radiation: Evidence from the southwestern United States. New Mexico Mus. Nat. Hist. Sci. Bull. 30, 67–79. Available at: https://scholar.google.com/scholar?hl=en&as_sdt=0%2C9&q=Lower+Permian+Flora+of+the+Sanzenbacher+Ranch%2C&btnG=.
DiMichele W. A., Kerp H., Sirmons R., Fedorko N., Skema V., Blake B. M. Jr., et al. (2013). Callipterid peltasperms of the Dunkard Group, Central Appalachian Basin. Int. J. Coal Geol. 119, 56–78. doi: 10.1016/j.coal.2013.07.025
DiMichele W. A., Lucas S. G., Chaney D. S., Donovan M. P., Kerp H., Koll R. A., et al. (2018b). Early Permian flora, Doña Ana Mountains, Southern New Mexico, with special consideration of taxonomic issues and arthropod damage. New Mexico Mus. Nat. Hist. Sci. Bull. 79, 165–205.
DiMichele W. A., Schneider J. W., Lucas S. G., Eble C. F., Falcon-Lang H. J., Looy C. V., et al. (2016). “Megaflora and palynoflora associated with a Late Pennsylvanian coal bed (Bursum Formation, Carrizo Arroyo, New Mexico, U.S.A.) and paleoenvironmental significance,” in New Mexico Geological Society Guidebook, 67th Field Conference., Geology of the Belen Area. Albuquerque, 351–368.
Dmitriev V. Y., Ponomarenko A. G. (2002). “General features of insect history,” in History of Insects. Eds. Rasnitsyn A. P., Quicke D. L. J. (Kluwer, Dordrecht), 325–436.
Donovan M. P., Lucas S. (2021). Insect herbivory on the Late Pennsylvanian Kinney Brick Quarry Flora, New Mexico, USA. New Mexico Mus. Nat. Hist. Sci. Bull. 84, 193–207. Available at: https://books.google.com/books?hl=en&lr=&id=rtUxEAAAQBAJ&oi=fnd&pg=PA193&ots=CoFUGOm5DB&sig=EDlajB9Ay2W3kSL33HYEVivhxf8#v=onepage&q&f=false
Dos Santos T. B., Pinheiro E. R. S., Iannuzzi R. (2020). First evidence of seed predation by arthropods from Gondwana and its early Paleozoic history (Rio Bonito Formation, Paraná Basin, Brazil). Palaios 35, 292–301. doi: 10.2110/palo.2020.004
Dreger-Jauffret F., Shorthouse J. D. (1992). “Diversity of gall-inducing insects and their galls,” in Biology of Insect-Induced Galls. Eds. Shorthouse J. D., Rohfritsch O. (Oxford University Press, New York), 8–33.
Edirisooriya G., Dharmagunawardhane H. A., McLoughlin S. (2018). The first record of the Permian Glossopteris flora from Sri Lanka: implications for hydrocarbon source rocks in the Mannar Basin. Geol. Mag. 155, 907–920. doi: 10.1017/S0016756816001114
Espírito-Santo M. M., Neves F. S., Fernandes G. W., Silva J. O. (2012). Plant phenology and absence of sex-biased gall attack on three species of Baccharis. PloS One 7, e46896. doi: 10.1371/journal.pone.0046896
Feng Z., Wang J., Rößler R., Ślipiński A., Labandeira C. C. (2017). Late Permian wood borings reveal an intricate network of ecological relationships. Nat. Commun. 8, 556. doi: 10.1038/s41467-017-00696-0
Feng Z., Wang J., Zhou W., Wan M., Pšenička J. (2021). Plant–insect interactions in the early Permian Wuda Tuff Flora, North China. Rev. Palaeobot. Palynol. 294, 104269. doi: 10.1016/j.revpalbo.2020.104269
Fernandes G. W., Price P. W. (1988). Biogeographical gradients in galling species richness: test of hypothesis. Oecologia 76, 161–167. doi: 10.1007/BF00379948
Fernandes G. W., Price P. W. (1992). The adaptive significance of insect gall distribution: survivorship of species in xeric and mesic habitats. Oecologia 90, 14–20. doi: 10.1007/BF00317803
Fernández J. A., Chiesa J. O. (2019). Plant-insect interactions in the fossil flora of the Bajo de Veliz Formation (Gzhelian–Asselian): San Luis, Argentina. Ichnos 27, 156–166. doi: 10.1080/10420940.2019.1697263
Gallego J., Cúneo R., Escapa I. (2014). Plant–arthropod interactions in gymnosperm leaves from the Early Permian of Patagonia, Argentina. Geobios 47, 101–110. doi: 10.1016/j.geobios.2014.01.002
Gastaldo R. A., DiMichele W. A., Pfefferkorn H. W. (1996). Out of the icehouse into the greenhouse; a late Paleozoic analog for modern global vegetational change. GSA Today 6, 1–7. Available at: https://repository.si.edu/bitstream/handle/10088/7161/paleo_1996_Gastaldo_Pfefferkorn_DiMichele_GSAToday.pdf
Glasspool I., Hilton J., Collinson M., Wang S. (2003). Foliar herbivory in Late Palaeozoic Cathaysian gigantopterids. Rev. Palaeobot. Palynol. 127, 125–132. doi: 10.1016/S0034-6667(03)00107-6
Glover B. J. (2000). Differentiation in plant epidermal cells. J. Expt. Bot. 51, 497–505. doi: 10.1093/jexbot/51.344.497
Griffis N., Tabor N. J., Stockli D., Stockli L. (2023). The Far-Field imprint of the late Paleozoic Ice Age, its demise, and the onset of a dust-house climate across the Eastern Shelf of the Midland Basin, Texas. Gondwana Res. 115, 17–36. doi: 10.1016/j.gr.2022.11.004
Gross J. (2007). CalPhotos – Photo Database – Callirhytis perdens; Ruptured Twig Gall Wasp – Shown with Quercus kelloggii. Available online at: https://calphotos.berkeley.edu/cgi/img_query?enlarge=1111+1111+2222+1368 (Accessed April of 2023).
Harris A. C., Bannister J. M., Lee D. E. (2007). Fossil scale insects (Hemiptera, Coccoidea, Diaspididae) in life position on an angiosperm leaf from an early Miocene lake deposit, Otago, New Zealand. J. R. Soc N. Z. 37, 1–13. doi: 10.1080/03014220709510531
Haug J. T., Labandeira C. C., Santiago-Blay J. A., Haug C., Brown S. (2015). Life habits, hox genes, and affinities of a 311 million-year-old holometabolan larva. BMC Evolution. Biol. 15, 208. doi: 10.1186/s12862-015-0428-8
Hentz T. F. (1988). Lithostratigraphy and paleoenvironments of upper Paleozoic continental red beds, North-Central Texas: Bowie (new) and Wichita (revised) Groups. Univ. Texas Bur. Econ. Geol. Rept. Investig. 170, 1e55. doi: 10.23867/RI0170D
Hickin N. E. (1975). The Insect Factor in Wood Decay. 3rd ed. (New York: St. Martin’s Press). 383 p.
Hori K. (1992). “Insect secretions and their effect on plant growth, with special reference to hemipterans,” in Biology of Insect-Induced Galls. Eds. Shorthouse J. D., Rohfritsch O. (Oxford University Press, New York), 157–170.
House G. J., Brust G. E. (1989). Ecology of low-input, no-tillage agroecosystems. Agric. Ecosys. Environ. 27, 331–345. doi: 10.1016/0167-8809(89)90096-0
Janzen D. H. (1971). Seed predation by animals. Annu. Rev. Ecol. Syst. 2, 465–492. doi: 10.1146/annurev.es.02.110171.002341
Janzen D. H. (1980). Specificity of seed-attacking beetles in a Costa Rican deciduous forest. J. Ecol. 68, 929–952. doi: 10.2307/2259466
Jennings J. R. (1974). Lower Pennsylvanian plants of Illinois I: a flora from the Pounds Sandstone Member of the Caseyville Formation. J. Paleontol. 48, 459–473. Available at: https://www.jstor.org/stable/1303133
Kerp J. H. F. (1988). Aspects of Permian palaeobotany and palynology. X. The West and Central European species of the genus Autunia Krasser emend. (Peltaspermaceae) and the form-genus Rhachiphyllum Kerp (callipterid foliage). Rev. Palaeobot. Palynol. 54, 249–360. doi: 10.1016/0034-6667(88)90017-6
Kerp H., Barthel M. (1993). Problems of cuticular analysis in pteridosperms. Rev. Palaeobot. Palynol. 78, 1–18. doi: 10.1016/0034-6667(93)90014-L
Koll R. A., DiMichele W. A. (2021). Dominance-diversity architecture of a mixed hygromorphic-to-xeromorphic flora from a botanically rich locality in western equatorial Pangea (lower Permian Emily Irish site, Texas, USA. Palaeogeogr. Palaeoclimatol. Palaeoecol. 563, 110132. doi: 10.1016/j.palaeo.2020.110132
Krugman S. L., Koerber W. (1969). Effect of cone feeding by Leptoglossus occidentalis on ponderosa pine seed development. For. Sci. 13, 104–111. Available at: https://academic.oup.com/forestscience/article-abstract/15/1/104/4709786.
Laaß M., Hauschke N. (2019). Earliest record of exophytic insect oviposition on plant material from the latest Pennsylvanian (Gzhelian, Stephanian C) of the Saale Basin, Germany. Palaeogeogr. Palaeoclimat. Palaeoecol. 534, 109337. doi: 10.1016/j.palaeo.2019.109337
Labandeira C. C. (2002). “The history of associations between plants and animals,” in Plant-Animal Interactions: An Evolutionary Approach, vol. 26–74 . Eds. Herrera C. M., Pellmyr O. (Blackwell Science, Oxford, U. K), 248–261.
Labandeira C. C. (2006a). Silurian to Triassic plant and insect clades and their associations: new data, a review, and interpretations. Arthropod. System. Phyl. 64, 53–94. doi: 10.3897/asp.64.e31644
Labandeira C. C. (2006b). The four phases of plant-arthropod associations in deep time. Geol. Acta 4, 409–438. Available at: https://scholar.google.com/scholar?hl=en&as_sdt=0%2C9&q=The+four+phases+of+plant-arthropod+associations&btnG=.
Labandeira C. C. (2019). “The fossil record of insect mouthparts: Innovation, functional convergence and associations with other organisms,” in Insect Mouthparts—Form, Function, Development and Performance, vol. 5 . Ed. Krenn H., 567–671. Zool. Monogr. Springer: Cham, Switzerland.
Labandeira C. C. (2021). Ecology and evolution of gall-inducing arthropods: The pattern from the terrestrial fossil record. Front. Ecol. Evol. 9, 632449. doi: 10.3389/fevo.2021.632449
Labandeira C. C., Allen E. G. (2007). Minimal insect herbivory for the Lower Permian Coprolite Bone Bed site of north-central Texas USA, and comparison to other Late Paleozoic floras. Palaeogeogr. Palaeoclim. Palaeoecol. 247, 197–219. doi: 10.1016/j.palaeo.2006.10.015
Labandeira C. C., Beall B. S. (1990). “Arthropod terrestriality,” in Arthropod Paleobiology, vol. 3 . Ed. Mikulic D., 214–256. Short Courses in Paleontology. The Paleontological Society: Knoxville, Tennessee.
Labandeira C. C., Kustatscher E., Wappler T. (2016). Floral assemblages and patterns of insect herbivory during the Permian to Triassic of Northeastern Italy. PloS One 11, e0165206. doi: 10.1371/journal.pone.0165205
Labandeira C. C., Phillips T. L. (1996a). Insect fluid-feeding on Upper Pennsylvanian tree ferns (Palaeodictyoptera, Marattiales) and the early history of the piercing-and-sucking functional feeding group, Ann. Entomol. Soc Am. 89, 157–183. doi: 10.1093/aesa/89.2.157
Labandeira C. C., Phillips T. L. (1996b). A Carboniferous insect gall: Insight into early ecologic history of the Holometabola. Proc. Natl. Acad. Sci. U.S.A. 93, 8470–8474. doi: 10.1073/pnas.93.16.8470
Labandeira C. C., Prevec R. (2014). Plant paleopathology and the roles of pathogens and insects. Int. J. Paleopath. 4, 1–16. doi: 10.1016/j.ijpp.2013.10.002
Labandeira C. C., Tremblay S. L., Bartowski E. E., Hernick L. V. (2013). Middle Devonian liverwort herbivory and antiherbivore defence. New Phytol. 202, 247–258. doi: 10.1111/nph.12643
Labandeira C. C., Wappler T. (2023). Arthropod and pathogen damage on fossil and modern plants: exploring the origins and evolution of herbivory on land. Annu. Rev. Entomol. 68, 341–361. doi: 10.1146/annurev-ento-120120-102849
Labandeira C. C., Wilf P., Johnson K. R., Marsh F. (2007). Guide to Insect (and Other) Damage Types on Compressed Plant Fossils. Version 3.0 (Washington D.C.: Smithsonian Institution). 25p.
Leal C. R. O., Silva J. O., Sousa-Souto L., Neves F. S. (2016). Vegetation structure determines insect herbivore diversity in seasonally dry tropical forests. J. Insect Conserv. 20, 979–988. doi: 10.1007/s10841-016-9930-6
Lin X. D., Labandeira C. C., Ding Q. L., Meng Q. M., Ren D. (2019). Exploiting nondietary resources in deep time: Patterns of oviposition on mid-Mesozoic plants from Northeastern China. Internat. J. Pl. Sci. 180, 411–457. doi: 10.1086/702641
Liu H., Wei H., Chen J., Guo Y., Zhou Y., Gou X., et al. (2020). A latitudinal gradient of plant–insect interactions during the late Permian in terrestrial ecosystems? New evidence from Southwest China. Global Planet. Change 192, 103248. doi: 10.1016/j.gloplacha.2020.103248
Luthardt L., Galtier J., Meyer-Berthaud B., Mencl V., Rößler R. (2021). Medullosan seed ferns of seasonally-dry habitats: old and new perspectives on enigmatic elements of Late Pennsylvanian–early Permian intramontane basinal vegetation. Rev. Palaeobot. Palynol. 288, 104400. doi: 10.1016/j.revpalbo.2021.104400
Lynch P. (2017). Alamy stock photos – Cypress twig gall midge Taxodiomyia cupressiananassa gall forming on bald cypress tree leaves. Available at: http://www.alamy.com/stock-photo-cypress-twig-gall-midge-taxodiomyia-cupressiananassa-gall-forming-24487601.html (Accessed February of 2018).
Ma F. J., Luo D. D., Liu S., Zhang C. Q., Wang Q. J., Li B. X., et al. (2023). Local provincialism of late Permian plant–arthropod associations in South Cathaysia: Evidence of arthropod-mediated damages in a Wuchiapingian assemblage of South China. J. Asian Ear. Sci. 254, 105729. doi: 10.1016/j.jseaes.2023.105729
MacCracken S. A., Labandeira C. C. (2020). The Middle Permian South Ash Pasture assemblage of north-central Texas: coniferophyte and gigantopterid herbivory and longer-term herbivory trends. Int. J. Plant Sci. 181, 342–362. doi: 10.1086/706852
Maderspacher F. (2021). Cecidology: anatomy of a biohack. Curr. Biol. 21, R430–R458. doi: 10.1016/j.cub.2021.03.095
Mamay S. H., Miller J. M., Rohr D. M., Stein J. W.E. (1988). Foliar morphology and anatomy of the gigantopterid plant Delnortea abbottiae, from the Lower Permian of west Texas. Am. J. Bot. 75, 1409–1433. doi: 10.1002/j.1537-2197.1988.tb14202.x
McLoughlin S. (2011). New records of leaf galls and arthropod oviposition scars in Permian–Triassic Gondwanan gymnosperms. Austral. J. Bot. 59, 156–169. doi: 10.1071/BT10297
Montañez I. P. (2022). Current synthesis of the penultimate icehouse and its imprint on the Upper Devonian through Permian stratigraphic record. Geol. Soc. London Special Public. 512, 213–245. doi: 10.1144/SP512-2021-124
Montañez I. P., McElwain J. C., Poulsen C. J., White J. D., DiMichele W. A., Wilson J. P., et al. (2016). Climate, pCO2 and terrestrial carbon cycle linkages during late Palaeozoic glacial-interglacial cycles. Nat. Geosci. 9, 824–828. doi: 10.1038/ngeo2822
Montañez I. P., Poulsen C. J. (2013). The late Paleozoic ice age: an evolving paradigm: Annu. Rev. Ear. Planet. Sci. 41, 629–656. doi: 10.1146/annurev.earth.031208.100118
Montañez I. P., Tabor N. J., Niemeier D., DiMichele W. A., Frank T. D., Fielding C. R., et al. (2007). CO2-forced climate and vegetation instability during Late Paleozoic deglaciation. Science 315, 87–91. doi: 10.1126/science.1134207
Naugolnykh S. V., Ponomarenko A. G. (2010). Possible traces of feeding by beetles in coniferophyte wood from the Kazanian of the Kama River Basin. Paleontol. J. 44, 468–474. doi: 10.1134/S0031030110040131
Nel A., Roques P., Nel P., Prokin A. A., Bourgin T., Prokop J., et al. (2013). The earliest known holometabolous insects. Nature 503, 257–261. doi: 10.1038/nature12629
Oksanen J., Simpson G., Blanchet F., Kindt R., Legendre P., Minchin P., et al. (2022). vegan: Community Ecology Package. R package version 2.6-4. Available at: https://CRAN.R-project.org/package=vegan.
Peeters P. J. (2002). Correlations between leaf structural traits and the densities of herbivorous insect guilds. Biol. J. Linn. Soc 77, 43–65. doi: 10.1046/j.1095-8312.2002.00091.x
Pinheiro E. R. S., Gallego J., Iannuzzi R., Cúneo R. (2015). First report of feeding traces in Permian Botrychiopsis leaves from Western Gondwana. Palaios 30, 613–619. doi: 10.2110/palo.2014.091
Pinheiro E. R. S., Iannuzzi R., Tybusch G. P. (2012). Specificity of leaf damage in the Permian “Glossopteris Flora”: A quantitative approach. Rev. Palaeobot. Palynol. 174, 113–121. doi: 10.1016/j.revpalbo.2012.01.002
Ponomarenko A. G. (1969). Historical development of archostematan beetles. Tr. Paleontol. Inst. 25, 1–240. Available at: https://scholar.google.com/scholar_lookup?title=Historical%20development%20of%20Archostemata%20beetles&publication_year=1969&author=A.G.%20Ponomarenko.
Prevec R., Labandeira C. C., Neveling J., Gastaldo R. A., Looy C. V., Bamford M. (2009). Portrait of a Gondwanan ecosystem: A new late Permian fossil locality from KwaZulu-Natal, South Africa. Rev. Palaeobot. Palynol. 156, 454–493. doi: 10.1016/j.revpalbo.2009.04.012
Price P. W., Fernandes G. W., Lara A. C. F., Brawn J., Barrios H., Wright M. G., et al. (1998). Global pattern in local number of insect galling species. J. Biogeogr. 25, 581–591. doi: 10.1046/j.1365-2699.1998.2530581.x
Prokop J., Nel A., Engel M. S. (2023). Diversity, form, and postembryonic development of Paleozoic insects. Annu. Rev. Entomol. 68, 401–429. doi: 10.1146/annurev-ento-120220-022637
Rasnitsyn A. P. (2002). “Class Insecta Linné, 1758, The insects,” in History of Insects. Eds. Rasnitsyn A. P., Quicke D. L. J. (Kluwer, Dordrecht), 65–324.
Rasnitsyn A. P., Aristov D. S., Rasnitsyn D. A. (2013). Dynamics of insect diversity during the Early and Middle Permian. Paleontol. J. 49, 1282–1309. doi: 10.1134/S0031030115120102
R Development Core Team. (2013). R: A language and environment for statistical computing (Vienna, Austria: R Foundation for Statistical Computing). Available at: https://www.R-project.org/.
Renwick J. A. A., Chew F. S. (1994). Oviposition behavior in Lepidoptera. Annu. Rev. Entomol. 39, 377–400. doi: 10.1146/annurev.en.39.010194.002113
Schachat S. R., Labandeira C. C. (2015). Evolution of a complex behavior: the origin and initial diversification of foliar galling by Permian insects. Sci. Nat. 202, 14. doi: 10.1007/s00114-015-1266-7
Schachat S., Labandeira C. C., Chaney D. S. (2015). Insect herbivory from early Permian Mitchell Creek Flats of north-central Texas: opportunism in a balanced component community. Palaeogeogr. Palaeoclim. Palaeoecol. 440, 830–847. doi: 10.1016/j.palaeo.2015.10.001
Schachat S., Labandeira C. C., Gordon J., Chaney D. S., Levi S., Halthore M. N., et al. (2014). Plant–insect interactions from early Permian (Kungurian) Colwell Creek Pond, north-central Texas: The early spread of herbivory in riparian environments. Internat. J. Plant Sci. 175, 855–890. doi: 10.1086/677679
Schachat S. R., Payne J. L., Boyce C. K., Labandeira C. C. (2022). Generating and testing hypotheses about the fossil record of insect herbivory with a theoretical ecospace. Rev. Palaeobot. Palynol. 297, 104564. doi: 10.1016/j.revpalbo.2021.104564
Schneider J. W., Werneburg R. (2006). “Insect biostratigraphy of the Euramerican continental Late Pennsylvanian and Early Permian,” in Non-Marine Permian Biostratigraphy and Biochronology, vol. 265 . Eds. Lucas S. G., Cassinis G., Schneider J. W. (Geol. Soc. Lond. Spec. Publ), 325–336.
Schuh R. T., Slater J. A. (1995). True Bugs of the World (Hemiptera: Heteroptera) – Classification and Natural History (Ithaca and London: Cornell University Press). 336 p.
Scott A. C., Taylor T. N. (1983). Plant/animal interactions during Upper Carboniferous. Bot. Rev. 49, 259–307. doi: 10.1007/BF02861089
Sharov A. G. (1971). Phylogeny of the Orthopteroidea. Jerusalem: Israeli Program for Scientific Translation Vol. 118. Ed. Salkind J. (Russian: Paleontologischeskogo Instituta), 251.
Sharov A. G. (1973). “Morphological features and mode of life of the Palaeodictyoptera,” in Readings in the Memory of Nicolaj Aleksandrovich Kholodovskij. Ed. Bei–Benko G. Y. (Leningrad: Academy of Sciences), 49–63.
Shcherbakov D. E. (2008). On Permian and Triassic insect faunas in relation to biogeography and the Permian–Triassic crisis. Paleontol. J. 42, 15–31. doi: 10.1134/S0031030108010036
Shcherbakov D. E., Popov Y. A. (2002). “Superorder Cimicidea Laicharting 1781 ORDER HEMIPTERA Linné, 1758. The Bugs, Cicadas, Plantlice, Scale Insects, etc. (-Cimicida Laicharting 1781, =Homoptera Leach 1815+ Heteroptera Latreille 1810),” in History of Insects. Eds. Rasnitsyn A. P., Quicke D. L. J. (Kluwer, Dordrecht), 143–157.
Simon S. S. T., Gibling M. R., DiMichele W. A., Chaney D. S., Looy C. V., Tabor N. J. (2016). An abandoned-channel fill with exquisitely preserved plants In redbeds of the Clear Fork Formation, Texas, USA: An Early Permian water-dependent habitat in the arid plains of Pangea. J. Sed. Res. 86, 944–964. doi: 10.2110/jsr.2016.60
Solomon J. D. (1995). Guide to Insect Borers in North American Broadleaf Trees and Shrubs (U. S. D. A. For. Serv. Agric. Hdbk. AH-706). 735 p.
Srivastava A. K., Agnihotri D. (2011). Insect traces on early Permian plants of India. Paleontol. J. 45, 200–206. doi: 10.1134/S0031030111020171
Thompson G. A., Goggin F. L. (2006). Transcriptomics and functional genomics of plant defense induction by phloem-feeding insects. J. Expt. Bot. 57, 755–766. doi: 10.1093/jxb/erj135
Vassilenko D. V. (2011). The first record of endophytic insect oviposition from the Tartarian of European Russia. Paleontol. J. 45, 333–334. doi: 10.1134/S0031030111030154
Vassilenko D. V., Karasev E. V. (2020). First report of insect endophytic oviposition from the upper permian of the Pechora Basin, on a leaf of Phylladoderma (Peltaspermopsida: Cardiolepidaceae). Paleontol. J. 54, 371–374. doi: 10.1134/S0031030120040140
Wang J., Labandeira C. C., Zhang Z.-F., Bek J., Pfefferkorn H. W. (2009). Permian Circulipuncturites discinisporis Labandeira, Wang, Zhang, Bek et Pfefferkorn gen. et spec. nov. (formerly Discinispora) from China, an ichnotaxon of punch-and-sucking insect on Noeggeranthialean spores. Rev. Palaeobot. Palynol. 156, 277–282. doi: 10.1016/j.revpalbo.2009.03.006
Whyte G., Howard K., St. J. Hardy G. E., Burgess T. L. (2011). Foliar pests and pathogens of Eucalyptus dunnii plantations in southern Queensland. Austral. For. 74, 161–169. doi: 10.1080/00049158.2011.10676359
Xiao L., Labandeira C. C., Ben-Dov Y., Maccracken S. A., Shih C., Dilcher D. L., et al. (2021). Early Cretaceous mealybug herbivory on a laurel highlights the deep-time history of angiosperm–scale insect associations. New Phytol. 232, 1414–1423. doi: 10.1111/nph.17672
Xiao L., Labandeira C. C., Dilcher D. L., Ren D. (2022b). Arthropod and fungal herbivory at the dawn of angiosperm diversification: The Rose Creek plant assemblage of Nebraska, U.S.A. Cret. Res. 131, 105088. doi: 10.1016/j.cretres.2021.105088
Xiao L., Labandeira C. C., Dilcher D. L., Ren D. (2022c). Data, metrics, and methods for “Arthropod and fungal herbivory at the dawn of angiosperm diversification: The Rose Creek plant assemblage of Nebraska, U.S.A”. Data Brief 42, 108170. doi: 10.1016/j.dib.2022.108170
Xiao L., Labandeira C. C., Ren D. (2022a). Insect herbivory immediately before the eclipse of the gymnosperms. Ins. Sci. 29, 1483–1520. doi: 10.1111/1744-7917.12988
Xu Q., Jin J., Labandeira C. C. (2018). Williamson Drive: herbivory from a north-central Texas flora of latest Pennsylvanian age shows discrete component community structure, expansion of piercing and sucking, and plant counterdefenses. Rev. Palaeobot. Palynol. 251, 28–72. doi: 10.1016/j.revpalbo.2018.01.002
Yang G. X., Wang H. S. (2012). Yuzhou Flora—A hidden gem of the Middle and Late Cathaysian Flora. Sci. China Earth Sci. 55, 1601–1619. doi: 10.1007/s11430-012-4476-2
Zhang J. (1993). Biology of Harpalus rufipes DeGeer (Coleoptera: Carabidae) in Maine and dynamics of seed predation. Master’s thesis, University of Maine, Orono.
Zhao X. Y., Yu Y. L., Clapham M. E., Yan E., Chen J., Jarzembowski E. A., et al. (2021). Early evolution of beetles regulated by the end-Permian deforestation. eLife 10, e72692. doi: 10.7554/eLife.72692
Zherikhin V. V. (2002). “Insect trace fossils,” in History of Insects. Eds. Rasnitsyn A. P., Quicke D. L. J. (Kluwer, Dordrecht), 303–324.
Zhao X., Yu Y., Clapham M. E., Yan E., Chen J., Jarzembowski E. A., Zhao X., Wang B. (2021). Early evolution of beetles regulated by the end-Permian deforestation. eLife 10, e72692. doi: 10.7554/eLife.72692
Keywords: Autunia, damage types, functional feeding groups, herbivory, peltasperms, seed plants
Citation: Dos Santos TB, Labandeira CC, Pinheiro ERdS and Iannuzzi R (2024) Plant interactions with arthropods and pathogens at Sanzenbacher Ranch, early Permian of Texas, and implications for herbivory evolution in Southwestern Euramerica. Front. Ecol. Evol. 12:1368174. doi: 10.3389/fevo.2024.1368174
Received: 10 January 2024; Accepted: 09 February 2024;
Published: 12 March 2024.
Edited by:
Juan Manuel Robledo, Centro de Ecología Aplicada del Litoral (CONICET), ArgentinaReviewed by:
Silvia Gnaedinger, Centro de Ecología Aplicada del Litoral (CONICET), ArgentinaPhilippe Moisan, Universidad de Atacama, Chile
Copyright © 2024 Dos Santos, Labandeira, Pinheiro and Iannuzzi. This is an open-access article distributed under the terms of the Creative Commons Attribution License (CC BY). The use, distribution or reproduction in other forums is permitted, provided the original author(s) and the copyright owner(s) are credited and that the original publication in this journal is cited, in accordance with accepted academic practice. No use, distribution or reproduction is permitted which does not comply with these terms.
*Correspondence: Thamiris Barbosa Dos Santos, thamiris.barbosa.santos@gmail.com