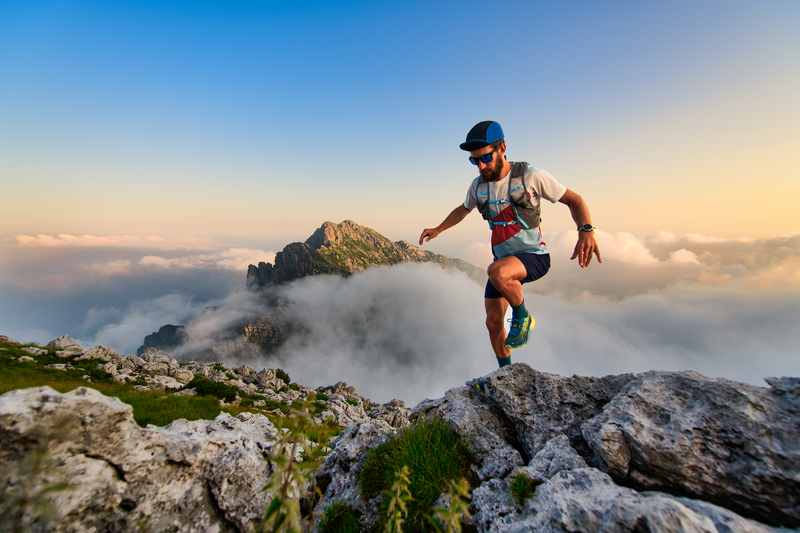
95% of researchers rate our articles as excellent or good
Learn more about the work of our research integrity team to safeguard the quality of each article we publish.
Find out more
ORIGINAL RESEARCH article
Front. Ecol. Evol. , 04 March 2024
Sec. Ecophysiology
Volume 12 - 2024 | https://doi.org/10.3389/fevo.2024.1365549
Heterothermy is considered to be the most effective energy-saving strategy improving survival under natural conditions. Interspecific studies suggest that this strategy is also associated with reduced reproductive output. Yet little is known about the reproductive consequences of heterothermy use at the intraspecific level and thus its repercussions for microevolutionary processes. Moreover, as yet no study has aimed to test if litter size and juvenile mass are affected by torpor use in wild captured animals under undemanding laboratory conditions. Here we tested the hypothesis that intraspecific variation in heterothermy use is associated with different reproductive successes, being the result of the evolution of distinct life histories. We predicted that heterothermy use in winter negatively correlates with litter size and juvenile body mass during the subsequent breeding season. To test this prediction, we used yellow-necked mice from a population in which individuals consistently differ in their use of heterothermy in winter. We measured body size (head width) and body mass, basal metabolic rate, as well as metabolism and body temperature during fasting-induced torpor in wild caught mice in winter. Phenotyped mice were bred in the subsequent summer selectively – males and females with similar heterothermy characteristics were paired, the most to the least heterothermic. Dam body size, but not basal metabolism, was positively correlated with litter size (but not juvenile mass). However, when accounting for this relationship, litter size was negatively while juvenile mass was positively correlated with the average heterothermy use of a given couple. Our study indicates that heterothermy use correlates with specific life-history strategies arising from a fundamental evolutionary trade-off between survival and reproduction.
In natural environments organisms rely on limited resources and must selectively allocate their available energy to a variety of functions, such as growth, reproduction, survival and self-maintenance. The resources that they invest into one function are no longer available to others, leading to a fundamental trade-off and the evolution of distinct life histories (Williams, 1966; Partridge and Harvey, 1988; Stearns, 1992; Roff and Fairbairn, 2007). Animal life-history strategies vary along a slow-fast continuum: species with fast-life histories mature earlier, and have larger reproductive rates and litter sizes, higher mortalities and shorter lifespan than those with slow-life histories (Stearns, 1992; Oli, 2004). It has been argued that in endothermic animals that maintain homeothermy – a high and stable body temperature (Tb) – the slow-fast continuum in the life histories of mammals is associated with the slow-fast continuum in energy metabolism. At the fast end of the life-history continuum, animals have higher metabolic costs of self-maintenance than those at the slow end (Symonds, 1999; Lovegrove, 2003).
Homeothermy enables animals to inhabit a wide range of environments; creates optimal conditions for enzyme function; supports sustained activity and locomotion; increases assimilation capacity; and can improve reproduction (Kemp, 2005). However, the fundamental cost of homeothermy is that the basal metabolic rate (BMR) at the thermoneutral conditions is several times the standard metabolic rate of similarly-sized ectothermic animals (Gillooly et al., 2017), meaning it has substantially higher energy requirements. When energy availability is limited, a high maintenance metabolism can be disadvantageous: the ‘compensatory’ hypothesis predicts that individuals with a low BMR can allocate more energy to growth, survival, and reproduction (Gadgil and Bossert, 1970; Steyermark, 2002; Larivee et al., 2010). By contrast, when food is not limited, the ‘increased intake’ hypothesis predicts that animals with a high BMR reflecting more efficient metabolic machinery can better assimilate energy for growth and reproduction, resulting in higher fitness (Bennett and Ruben, 1979; Hayes et al., 1992).
Many mammals and birds can display substantial temporal and regional variation in Tb that allows them to avoid 70-95% of the energy loss associated with their BMR (Ruf and Geiser, 2015). Thus, many endotherms reduce self-maintenance costs by abandoning homeothermic thermoregulation. This is most likely why many endothermic species have evolved or maintained the ability to be heterothermic, e.g., by being able to enter torpor (Grigg et al., 2004; Lovegrove, 2017). Torpor in endotherms is a controlled process characterized by greatly reduced metabolic rates (MR) and Tb (Ruf and Geiser, 2015), and thus, it is considered to be the most effective energy-saving response to food shortage (Geiser, 2004). Adaptive heterothermy has traditionally been divided into daily torpor and hibernation (multi-day torpor). Daily torpor is shorter and usually reduces MR and Tb less than steady-state hibernation (Geiser and Ruf, 1995). This ancestral trait in mammals (Grigg et al., 2004) likely allowed animals to survive the short- and long-term environmental devastation that occurred during the K-Pg boundary asteroid impact (Lovegrove, 2017), and nowadays may be slowing the extinction rate (Geiser and Turbill, 2009, Hanna and Cardillo, 2014). Heterothermy has also been suggested to be a strategy for avoiding predators (Hoelzl et al., 2015; Turbill and Stojanovski, 2018; Turbill et al., 2019; but see Humphries et al., 2003). Thus, many studies have assumed that heterothermy improves survival, especially under challenging environmental conditions (Ehrhardt et al., 2005; Geiser and Pavey, 2007; Rojas et al., 2014; Dammhahn et al., 2017).
Despite many benefits, heterothermy also has significant physiological costs (Humphries et al., 2003; Landes et al., 2020). Torpor can lead to memory disorder (Millesi et al., 2001), reduced immunocompetence (Bouma et al., 2010), dehydration and compromised ionic balance (Thomas and Geiser, 1997), oxidative stress (Buzadžić et al., 1990) and sleep debt (Daan et al., 1991; Royo et al., 2019). Moreover, in females torpor can be used to enhance body condition before lactation, however lowering Tb during pregnancy significantly delays juvenile development (Racey and Swift, 1981; Willis et al., 2006; Canale et al., 2012). Heterothermy can also affect negatively reproduction in males as normothermy is required for gonadal growth and maturation (Barnes et al., 1986; McAllan and Geiser, 2014). Thus, during reproduction, endotherms minimize torpor use and enter shallow heterothermy states only under energetically stressful conditions (Levesque and Lovegrove, 2014; McAllan and Geiser, 2014). During reproduction, an organism requires high MR and Tb to process and transfer nutrients to growing offspring (Kenagy et al., 1989), which correlates with high levels of reproductive hormones (Hinds et al., 1996). As torpor is associated with very low levels of reproductive hormones and MR, the physiological states of reproduction and torpor are at least partially incompatible (McAllan and Geiser, 2014).
The evolution of heterothermy may have significant implications for other life-history-related traits. The extreme form of heterothermy – hibernation, is also associated with lower basal metabolism (Cooper and Geiser, 2008; Careau, 2013; Boratyński and Szafrańska, 2018) as well as with a slow-living life history (Turbill et al., 2011; Bieber et al., 2018). Since hibernating species have longer life spans and higher survival but lower reproductive rates than non-hibernating species, studies have suggested these contrasting thermoregulatory strategies are associated with an evolutionary trade-off between survival and reproduction (Turbill et al., 2011; Ruf et al., 2012). Heterothermy use consistently differs among individuals within populations (Nespolo et al., 2010; Dammhahn et al., 2017; Boratyński et al., 2019), and it is likely a heritable trait (Lane et al., 2011; Grabek et al., 2019). However, little is known about the fitness consequences of among-individual variation in heterothermy use at the population level (but see Dammhahn et al., 2017).
Here we tested the hypothesis that intraspecific variation in heterothermy use is associated with variation in reproductive success, as a result of the evolution of distinct life histories within a species. To test this prediction, we measured individual use of winter heterothermy during short-term fasting in wild-caught yellow-necked mice Apodemus flavicollis, and then reproduced them selectively. We expected that heterothermy use by individuals in winter would negatively correlate with their litter size and mass during the subsequent reproductive season.
We studied mice from a single population in Białowieża Forest (Eastern Poland) in primaeval stands composed mainly of pedunculate oak Quercus robur and hornbeam Carpinus betulus trees. The wild mice (N=133) were captured at 39 locations at least ~400 m apart using wooden live traps and transferred to a laboratory in the Mammal Research Institute in Białowieża Village (~15km away from sampling sites). Before carrying out laboratory measurements, mice were kept in standard individual rodent cages at 16°C, under natural photoperiod and with access to water and food ad libitum. Before midday on trapping day, each animal was tagged individually with thermosensitive programmable identification transponders (model: IPTT-300, Biomedic Data Systems Inc., Seaford, US). Transponders were pre-calibrated in a water bath at five temperatures (range=18-42°C) against a precise (0.1°C) mercury-in-glass thermometer (Jenatherm N, Germany). Transponders were implanted subcutaneously under a 2% mixture of isoflurane (Iso-Vet) and oxygen anesthetic in the abdomen. While mice were still under anesthesia we measured their head width (HW) as a body size indicator with a caliper, to the nearest 0.1mm (Measy DG, Ecotone, Gdańsk, Poland) and weighed them to the nearest 0.1g (ScoutPro 200, Ohaus, Parsippany, NJ, USA). After a ~week of habituation, BMR (and corresponding body mass) was measured in each mouse. After a second ~week, we measured MR and Tb during 23h fasting experiments aimed at inducing torpor. All phenotypic measurements were done between end of January and the 21st of March in the winters of 2022 or 2023. After measurements, animals that used torpor (defined as entering an evident state of torpor with Tb<32°C) characterized by varied use of heterothermy were selected for breeding (40 individuals from 21 locations: 20 females and 20 males). In mice selected for breeding, we repeated measurements of HW. Between May and September, selected pairs were placed in big breeding cages equipped with branches allowing them to climb, and lined with leaves, wooden shavings, coco fibers and nest-box. The breeding cages were housed in walk-in climatic chambers at Ta=23°C with a natural photoperiod. Paired heterothermic mice were selected based on the degree of heterothermy they used (the most heterothermic male was paired with the most heterothermic female). After three weeks males were separated from females and released at their place of capture. We checked for the presence of juveniles twice a week. In total 17 pairs (each individual was allowed to breed once) with contrasting heterothermy use were successfully bred. One-month-old juveniles were separated from their mothers (and housed in laboratory till natural death), counted, and weighed to the nearest 0.1g. Females were then released at their place of capture.
The metabolic rate of mice was measured as oxygen consumption using indirect calorimetry with an open-flow respirometry a ~week after capture. The air used for measurements was pulled from outside, dried (silica gel 2-5mm, Chempur, Piekary Śląskie, Poland) and purified with activated coconut carbon (0.6-2.36mm, Acrivsorb 109, Browin S.zoo, Łódź, Poland). For BMR measurements animals were held in 300mL custom-made glass chambers placed at a thermoneutral zone (~30°C; Cygan 1985) in temperature-controlled cabinets (model: KB 53, Binder GmbH, Tuttlingen, Germany), and the flow through each chamber was set to ~250mL·min-1 and measured incurrently (FlowBar-8, Sable Systems International, Las Vegas USA; henceforth: SSI). The chambers were connected to a two-line multiplexer (MUX, SSI), which allowed us to extract air from two chambers at once and measure six individuals by sampling air automatically. During BMR measurements animals were held in chambers for ~4h, meaning we measured 12 individuals in an 8h work day. The dry air (silica gel 2-5mm, Chempur, Piekary Śląskie, Poland) leaving chamber was sampled at a rate of ~100mL·min-1 to pass through one of two gas analyzers (FC-10a, SSI) connected to one of two lines. The electronic elements of the respirometry system were connected to the computer via an analogue-digital interface (UI-2, SSI), and data were acquired at 1Hz using the software (ExpeData, SSI). During metabolic rate measurements, the air from each chamber was sampled for 140s, every 10min (initial and final baselines were sampled for 10s and 170s, respectively).
To induce torpor we deprived animals of food for ~23h in moderately cold conditions (~16°C). A similar protocol was used successfully by us previously (see: Boratyński et al., 2018; Boratyński et al., 2019; Boratyński et al., 2021). Heterothermy use measurements were conducted after BMR measurements (up to two weeks after capture). Continuous automatic Tb measurements (sampled every few seconds) carried out during short-term fasting were obtained from the transponders with dedicated readers (FSP-7005; Bio Medic Data Systems, Inc., Seaford, USA; henceforth: BMDS) connected to PCs via data acquisition stations (DAS-8010; BMDS). Due to equipment limitations, we measured Tb in five individuals daily. Animals were placed in 850mL chambers constructed of polypropylene containers (model: HPL 808, LocknLock Co., Seoul, Republic of Korea) lined with 4g of shavings. Chambers were connected to a respirometry system (see above), and the air was set to flow through at a rate of ~350mL·min-1. The air leaving each chamber was subsampled at a rate of ~200mL·min-1. This allowed us to measure both Tb and MR over ~23h starting at ~2 pm of the first day and finishing at ~1 pm of the next. One hour was needed to switch animals between measurements. We used the same sampling protocol and respirometry system, as for BMR, to obtain metabolic measurements during fasting.
Oxygen consumption (V̇O2) was calculated following equation 10.2 in Lighton (2008) assuming a 0.8 respiratory exchange ratio. For each individual, in total, we obtained at least 20 and 140 samples of V̇O2 while measuring BMR and torpor use, respectively. We selected only the last 20s (120s were needed to full flush of air in the oxygen analyzers) of each 140s sample as individual metabolic rate measurements. We considered BMR and torpor metabolic rate (TMR) as the lowest values obtained for a given mouse.
Many distinct methods exist to describe thermoregulatory patterns in endotherms (Boyles, 2019). However, most of them are based on just one component (mostly Tb). Here we used principal component analysis (PCA) to obtain a single variable describing an individual’s heterothermy use as a consequence of torpor bout duration (TBD: time spent with Tb<32°C), minimum Tb (Tb-min) during torpor, and corresponding metabolic suppression (for details of physiological parameters see Supplementary Table S1). Since mb-adjusted MR during torpor correlates positively with mb-adjusted BMR (Boratyński and Szafrańska, 2018), we calculated metabolic suppression as maintenance minus torpor metabolism. The relative reduction of metabolism during torpor (RRMT) was calculated as the difference between residual BMR and residual TMR (residuals were calculated based on linear relationships between log10-transformed BMR and log10-transformed mb, and between log10-transformed TMR and log10-transformed mb, respectively).
We calculated relative litter size as the number of juveniles in a given litter divided by the maximum number of juveniles ever recorded in a litter in the local population (eight individuals, Adamczewska, 1961). We calculated best linear unbiased predictions (BLUP) as conditional modes of the random effects from the linear mixed model (juvenile mb was the dependent variable, sex was a factor and couple was a random effect) to estimate the average mb for a litter.
All statistics were done in R v.4.3.1 (https://www.R-project.org/).
To account for all three parameters of heterothermy (TBD, Tb-min, and RRMT) in the future analysis we used PCA to estimate their common vector as the first principal component (PC1). PC1 correlated positively with log10-transformed TBD and RRMT but negatively with log10-transformed Tb-min (see Results).
Since variance in heterothermy use differed between males and females, we compared PC1-heterothermy using a generalized least squares model (GLS) using the R function gls. We used the constant variance function (varIdent) to allow unequal variations in heterothermy use for male and female mice. In the GLS for PC1-heterothermy, we included as covariates residual head width (obtained from the linear model: HW~sex) and body condition (obtained as residuals from the linear model: log10-transformed mb~log10-transformed HW) and sex (a centered continuous predictor) as a factor. In this model, we also included interactions between sex and body condition, and sex and residual HW.
Since relative litter size and BLUP-juvenile mb were correlated (Pearson r=-0.55, P=0.022), we compared them in a bivariate linear model (LM). This model included as covariates dam body size (female head width), dam residual basal metabolic rate (obtained from the linear relationship between BMR and mb), and average PC1-heterothermy for dam and sire.
Function visreg of R was use for visualization of regression models. All continuous variables were scaled using R function scale prior to analysis.
PC1 explained ~83% of the variance in the three studied parameters of heterothermy and was positively correlated with TBD (r=0.93) and RRMT (r=0.86) but negatively with Tb-min (r=-0.94). Body size and body condition and its interactions with sex did not explain PC1 (heterothermy use), which was only higher in females (PC1: mean ± SE = 0.58 ± 0.94) than males (PC1: mean ± SE = -0.58 ± 1.88; Table 1).
Table 1 Results of the generalized least squares model explaining variation in heterothermy use in male and female yellow-necked mice.
As a result of selective breeding, PC1-heterothermy in dam was highly correlated with PC1-heterothermy in sire (Pearson r=0.64, P=0.006).
Dam residual BMR was not a significant predictor of relative litter size or BLUP-juvenile mb in bivariate the LM (Table 2). Litter size and BLUP-juvenile mb were, however, predicted by dam body size and the heterothermy of males and females that bred together (Table 2). The univariate model for relative litter size indicated it was positively correlated with dam body size (Figure 1A) and negatively with the average of PC1 heterothermy use of dam and sire (Figure 1B, Table 2). The univariate model for BLUP-juvenile mb indicated only a positive correlation with PC1-heterothermy use of dam and sire (Figure 1C, Table 2).
Table 2 Results of the bivariate linear model explaining variation in relative litter size and litter mass (BLUP-juvenile mass: best linear unbiased predictions for the random effect litter) in 17 breeding mouse couples that had contrasting thermoregulatory strategies.
Figure 1 Relationships between relative litter size and (A) dam body size (Dam head width) and (B) heterothermy use of breeding couples (PC1-couple heterothermy); and the relationship between best linear unbiased predictions (BLUP) for juvenile body mass (C) and heterothermy use by breeding couple.
We tested the hypothesis that heterothermy use in winter has long-lasting consequences in the breeding season, under laboratory conditions with unlimited resource availability and non-demanding thermal conditions. We predicted that if heterothermy is associated with long life histories, then more heterothermic animals would have lower reproductive success than less heterothermic ones. We used the yellow-necked mouse as a model for testing these predictions as within a single population of this species we previously found consistent and large variation in thermoregulatory strategies among individuals (Boratyński et al., 2019). We found that the relative litter size was positively correlated with the body size of females, which is consistent with many previous studies (review in: Weller et al., 2023). However, when accounting for this, the relative litter size of a given couple was negatively correlated with the average heterothermy use (a result of torpor depth and length, and corresponding metabolic suppression under heterothermy) of the dam and sire in a couple. This strongly supports previous findings that overall reproductive success (result of litter size and offspring survival) is reduced in most heterothermic individuals within a population (Dammhahn et al., 2017). Thus, our results support the idea that this survival strategy is associated with evolutionary trade-offs and the evolution of distinct life histories.
The ‘assimilation capacity’ hypothesis predicts that higher maternal maintenance metabolism correlates positively with parental investments and as such can improve reproductive success (Koteja, 2000, 2004). Several studies indicate that BMR and the related sustained MR during reproduction can positively affects parental investments, resulting in improved juvenile growth (Glazier, 1985, Derting, 1989; Kam et al., 2003; Speakman et al., 2004; Sadowska et al., 2013). We measured BMR only in the winter season however, several studies indicate that maintenance metabolism is a repeatable trait (Nespolo and Franco, 2007; White et al., 2013; Auer et al., 2016; for yellow-necked mice see: Boratyński et al., 2019; Strijker et al., 2023) indicating that among-individual variation should be consistent over-time. Thus if maintenance metabolism has significant evolutionary consequences, female maintenance metabolism should positively correlate with litter size and/or juvenile mass. In our study however, mouse BMR was not a significant predictor of relative litter size nor of juvenile average body mass (Table 1), meaning these results did not support the ‘assimilation capacity’ hypothesis. Although a link between litter size and mb independent variation in BMR has been found at the interspecific level (Kalcounis-Rueppell, 2007), intraspecific studies have not found support for this relationship (Hayes et al., 1992; Johnson et al., 2001; Johnston et al., 2007; review in: Pettersen et al., 2018; but see also: Boratyński et al., 2013). However, overall reproductive success has been found to correlate positively with BMR in free-ranging bank voles (Boratyński and Koteja, 2010). This suggests that maternal maintenance metabolism affects an interaction between mating, parental investment and offspring survival rather than only litter size.
Several hypotheses have been proposed to explain variation in reproductive fitness parameters (e.g. litter size) and/or maternal investment (e.g. offspring mass) in wild living populations – which is likely shaped by a complex interplay between competition, offspring and parent survival, dam body condition, reproductive plasticity and environmental variability (Lack, 1966; Mountford, 1968; Perrins and Moss, 1975; Reznick, 1985; Boyce and Perrins, 1987; Risch et al., 1995; Morris, 1998; Risch et al., 2007). It is apparent, however, that maternal body size/weight is a key trait associated with litter size and/or litter mass. Body size/mass of adults tends to correlate negatively with litter size across a variety of different-size species (Tuomi, 1980), but both traits are correlated positively at the intraspecific level (Schai-Braun et al., 2021; Weller et al., 2023). The positive correlation between female body size and litter size (Figure 1A) observed in our study is following this general pattern. Body size/mass and litter size/mass can be heritable (Réale et al., 1999; Thomas et al., 2002; Coltman, 2005; Hare and Leighton, 2006), and artificial studies have indicated that selecting for one of these quantitative traits can result in a correlated increase of the other (Bünger et al., 2005). According to the ‘resource availability hypothesis’ the body mass/size is positively correlated with resource availability (Blackburn and Hawkins, 2004; Alhajeri et al., 2020). Since we collected animals from different locations that likely varied in resource availability, the correlation between maternal size and litter size may reflect natural selection constraining allometry limiting female reproductive capacity (e.g. uterine capacity). Alternatively for the natural selection, a similar might be the result of permanent environmental effect, shaping parental phenotype during development.
When accounting for the relationship between dam body size and litter size, parents’ heterothermy explained the variation in number of juveniles. Heterothermy use in winter among individuals from a single population has previously been found to correlate negatively with overall reproductive success in summer in free-ranging eastern chipmunks Tamias striatus (Dammhahn et al., 2017). This suggests the existence of a trade-off between this energy-saving strategy for increasing survival and reproductive investments. However, overall reproductive success is the result of mating intensity, litter size and offspring survival; thus, it is unclear from that study which of these traits (if any alone) negatively correlates with heterothermy. As we bred mice under laboratory conditions with access to unlimited food resources and at undemanding thermal conditions, the reproductive success we observed can be considered their reproductive potential (likely close to maximal). Thus, our study suggests that the reduced reproductive success observed in free-ranging animals using heterothermy does not necessarily result from losing mating opportunities or from other traits (as suggested by Dammhahn et al., 2017), but could be the direct result of highly heterothermic animals having reduced reproductive capacity. We also observed that bred couples’ heterothermy use was positively correlated with the body mass of one-month-old juveniles. Since higher juvenile body mass can improve survival (Ringsby et al., 1998; Brown et al., 2022), this result suggests that heterothermic animals invest more into offspring quality than quantity. However, there was also a negative correlation between average juvenile mass and juvenile number. Thus, lower juvenile mass in less heterothermic animals does not necessarily have to be correlated with parents’ physiologies but could be constrained by higher litter size itself.
The modern theoretical model for adaptive thermoregulation suggests the existence of a continuum of thermoregulatory strategies (Angilletta et al., 2006; 2010). Since the overall area under the theoretical performance curve is expected to be maximized (but also finite), thermoregulatory specialists (e.g. strictly homeothermic animals) operating at a narrow Tb range should perform better at optimal temperatures than thermoregulatory generalists – heterothermic animals characterized by a wide Tb range (Angilletta et al., 2006, 2010). This varying thermosensitivity can underlie/cause (Boyles and Warne, 2013; Seebacher and Little, 2017) intraindividual variation in the performance of skeletal muscles (Choi et al., 1998; Rojas et al., 2012; James et al., 2015), immune function (Butler et al., 2013), and generally complex molecular networks (Schwartz and Bronikowski, 2013). Finally, the specialist-generalist model predicts that, as a result of the cost-benefit trade-off, different thermoregulatory strategies should promote distinct (reproduction or survival) fitness components (Angilletta et al., 2010). If heterothermy improves survival (Turbill et al., 2011; Dammhahn et al., 2017; Geiser and Ruf, 2023) but simultaneously impairs reproduction (Dammhahn et al., 2017; this study), then both these strategies can coexist among individuals within a single population as a result of the fundamental evolutionary trade-off.
The evolutionary consequences of the different thermoregulatory strategies could have important implications for mouse population dynamics, which is strongly affected by food availability (Pucek et al., 1993; Bogdziewicz et al., 2016). Typically, the mouse population increases in the spring and summer following masting (synchronized seed production of forest trees) and crashes two years later (Pucek et al., 1993; Zwolak et al., 2016). While the post-mast increase in rodent abundance is most likely driven by an extended breeding season, winter breeding and high overwinter survival (Pucek et al., 1993; Zwolak et al., 2016), the factors affecting the post-outbreak crash are less well-known but probably involve density-dependent inhibition of maturation and breeding (Elias et al., 2006), predation (Pucek et al., 1993), disease, and parasites (Pedersen and Greives, 2008). The results of our experiment provide another explanation for the observed steep decline in mouse abundances after the post-masting peak. The proportion of individuals using heterothermy in winter increases when food resources become scarce (Dammhahn et al., 2017; Boratyński et al., 2021), which likely contributes to the much lower reproduction rates in the following breeding season.
The raw data supporting the conclusions of this article will be made available by the authors, without undue reservation.
The animal study was approved by Local Committee for Ethics in Animal Research in Olsztyn (decision no. 67/2020). The study was conducted in accordance with the local legislation and institutional requirements.
JB: Conceptualization, Data curation, Formal analysis, Funding acquisition, Investigation, Methodology, Project administration, Resources, Software, Validation, Visualization, Writing – original draft, Writing – review and editing. KI: Investigation, Writing – review and editing. KZ: Conceptualization, Funding acquisition, Investigation, Supervision, Writing – review and editing.
The author(s) declare that financial support was received for the research, authorship, and/or publication of this article. The study was supported by a grant from the Polish National Science Center awarded to JB based on decision number 2019/35/D/NZ8/03626.
We would like to thank Tom Diserens for providing language corrections. We also thank Zbyszek Boratyński and two reviewers for comments and suggestions that helped us to improve this version of the manuscript.
The authors declare that the research was conducted in the absence of any commercial or financial relationships that could be construed as a potential conflict of interest.
All claims expressed in this article are solely those of the authors and do not necessarily represent those of their affiliated organizations, or those of the publisher, the editors and the reviewers. Any product that may be evaluated in this article, or claim that may be made by its manufacturer, is not guaranteed or endorsed by the publisher.
The Supplementary Material for this article can be found online at: https://www.frontiersin.org/articles/10.3389/fevo.2024.1365549/full#supplementary-material
Adamczewska K. A. (1961). Intensity of reproduction of the Apodemus flavicollis (Melchior 1834) during the period 1954-1959. Acta Theriol. 5, 1–21. doi: 10.4098/0001-7051
Alhajeri B. H., Porto L. M., Maestri R. (2020). Habitat productivity is a poor predictor of body size in rodents. Curr. Zool. 66, 135–143. doi: 10.1093/cz/zoz037
Angilletta M. J., Bennett A. F., Guderley H., Navas C. A., Seebacher F., Wilson R. S. (2006). Coadaptation: aunifying principle in evolutionary thermal biology. Physiol. Biochem. Zool. 79, 282–294. doi: 10.1086/499990
Angilletta M. J., Cooper B. S., Schuler M. S., Boyles J. G. (2010). The evolution of thermal physiologyinendotherms. Front. Biosci. 2, 861–881. doi: 10.1016/S0306-4565(01)00094-8
Auer S. K., Bassar R. D., Salin K., Metcalfe N. B. (2016). Repeatability of metabolic rate is lower for animals living under field versus laboratory conditions. J. Exp. Biol. 219, 631–634. doi: 10.1242/jeb.133678
Barnes B. M., Kretzmann M., Licht P., Zucker I. (1986). The influence of hibernation on testis growth and spermatogenesis in the golden-mantled ground squirrel, Spermophilus lateralis. Biol. Reprod. 35, 1289–1297. doi: 10.1095/biolreprod35.5.1289
Bennett A. F., Ruben J. A. (1979). Endothermy and activity in vertebrates. Science 206, 649–654. doi: 10.1126/science.493968
Bieber C., Turbill C., Ruf T. (2018). Effects of aging on timing of hibernation and reproduction. Scient. Rep. 8, 13881. doi: 10.1038/s41598-018-32311-7
Blackburn T. M., Hawkins B. A. (2004). Bergmann’s rule and the mammal fauna of northern North America. Ecography 27, 715–724. doi: 10.1111/j.0906-7590.2004.03999.x
Bogdziewicz M., Zwolak R., Crone E. E. (2016). How do vertebrates respond to mast seeding? Oikos 125, 300–307. doi: 10.1111/oik.03012
Boratyński J. S., Iwińska K., Bogdanowicz W. (2018). Body temperature variation in free-living and food-deprived yellow-necked mice sustains an adaptive framework for endothermic thermoregulation. Mammal Res. 63, 493–500. doi: 10.1007/s13364-018-0392-y
Boratyński J. S., Iwińska K., Bogdanowicz W. (2019). An intra-population heterothermy continuum: notable repeatability of body temperature variation in food-deprived yellow-necked mice. J. Exp. Biol. 222, 1–10. doi: 10.1242/jeb.197152
Boratyński J. S., Iwińska K., Szafrańska P. A., Chibowski P., Bogdanowicz W. (2021). Continuous growth through winter correlates with increased resting metabolic rate but does not affect daily energy budgets due to torpor use. Curr. Zool. 67, 131–145. doi: 10.1093/cz/zoaa047
Boratyński Z., Koteja P. (2010). Sexual and natural selection on body mass and metabolic rates in free-living bank voles. Func. Ecol. 24, 1252–1261. doi: 10.1111/j.1365-2435.2010.01764.x
Boratyński J. S., Szafrańska P. A. (2018). Does basal metabolism set the limit for metabolic downregulation during torpor? Physiol. Biochem. Zoo. 91, 1057–1067. doi: 10.1086/699917
Boratyński Z., Koskela E., Mappes T., Schroderus E. (2013). Quantitative genetics and fitness effects of basal metabolism. Evol. Ecol. 27, 301–314. doi: 10.1007/s10682-012-9590-2
Bouma H. R., Strijkstra A. M., Boerema A. S., Deelman L. E., Epema A. H., Hut R. A., et al. (2010). Blood cell dynamics during hibernation in the European Ground Squirrel. Vet. Immunol. Immunopathol. 136, 319–323. doi: 10.1016/j.vetimm.2010.03.016
Boyce M. S., Perrins C. M. (1987). Optimizing great titclutch size in a fluctuating environment. Ecology 68, 142–153. doi: 10.2307/1938814
Boyles J. G. (2019). A brief introduction to methods for describing body temperature in endotherms. Physiol. Biochem. Zool. 92, 365–372. doi: 10.1086/703420
Boyles J. G., Warne R. W. (2013). A novel framework for predicting the use of facultative heterothermy by endotherms. J. Theor. Biol. 336, 242–245. doi: 10.1016/j.jtbi.2013.08.010
Brown T. J., Dugdale H. L., Hammers M., Komdeur J., Richardson D. S. (2022). Seychelles warblers with silver spoons: Juvenile body mass is a lifelong predictor of annual survival, but not annual reproduction or senescence. Ecol. Evol. 12, e9049. doi: 10.1002/ece3.9049
Bünger L., Lewis R. M., Rothschild M. F., Blasco A., Renne U., Simm G. (2005). Relationships between quantitative and reproductive fitness traits in animals. Philos. Trans. R. Soc Lond. B Biol. Sci. 360, 1489–1502. doi: 10.1098/rstb.2005.1679
Butler M. W., Stahlschmidt Z. R., Ardia D. R., Davies S., Davies J., Guillette L. J., et al. (2013). Thermal sensitivity of immune function: evidemce against ageneralist-specialist trade-off among endothermic and ectothermic vertebrates. Am. Nat. 181 761, 774. doi: 10.1086/670191
Buzadžić B., Spasić M., Saičić Z. S., Radojičić R., Petrović V. M., Halliwell B. (1990). Antioxidant defenses in the ground squirrel Citellus citellus 2. The effect of hibernation. Free Radic. Biol. Med. 9, 407–413. doi: 10.1016/0891-5849(90)90017-D
Canale C. I., Perret M., Henry P. Y. (2012). Torpor use during gestation and lactation in a primate. Naturwissenschaften 99, 159–163. doi: 10.1007/s00114-011-0872-2
Careau V. (2013). Basal metabolic rate, maximum thermogenic capacity and aerobic scope in rodents: interaction between environmental temperature and torpor use. Biol. Let. 9, 20121104. doi: 10.1098/rsbl.2012.1104
Choi I. H., Cho Y., Oh Y. K., Jung N. P., Shin H. C. (1998). Behavior and muscle performance in heterothermicbats. Physiol. Biochem. Zool. 71, 257–266. doi: 10.1086/515915
Coltman D. W. (2005). Testing marker-based estimates of heritability in the wild. Mol. Ecol. 14, 2593–2599. doi: 10.1111/j.1365-294X.2005.02600.x
Cooper C. E., Geiser F. (2008). The “minimal boundary curve for endothermy” as a predictor of heterothermy in mammals and birds: a review. J. Comp. Physiol. B 178, 1–8. doi: 10.1007/s00360-007-0193-0
Daan S., Barnes B. M., Strijkstra A. M. (1991). Warming up for sleep?—ground squirrels sleep duringarousalsfrom hibernation. Neurosci. Lett. 128, 265–268. doi: 10.1016/0304-3940(91)90276-Y
Dammhahn M., Landry-Cuerrier M., Réale D., Garant D., Humphries M. M. (2017). Individual variation in energy-saving heterothermy affects survival and reproductive success. Func. Ecol. 31, 866–875. doi: 10.1111/1365-2435.12797
Derting T. L. (1989). Metabolism and food availability as regulators of production in juvenile cotton rats. Ecology 7, 587–595. doi: 10.2307/1940210
Ehrhardt N., Heldmaier G., Exner C. (2005). Adaptive mechanisms during food restriction in Acomys russatus: the use of torpor for desert survival. J. Comp. Physiol. B. 175, 193–200. doi: 10.1007/s00360-005-0475-3
Elias S. P., Witham J. W., Hunter M. L. Jr. (2006). A cyclic redbacked vole (Clethrionomys gapperi) population and seedfall over 22 years in Maine. J. Mammal. 87, 440–445. doi: 10.1644/05-MAMM-A-170R1.1
Gadgil M., Bossert W. H. (1970). Life historical consequences of natural selection. Am. Nat. 104, 1–24. doi: 10.1086/282637
Geiser F. (2004). Metabolic rate and body temperature reduction during hibernation and daily torpor. Annu. Rev. Physiol. 66, 239–274. doi: 10.1146/annurev.physiol.66.032102.115105
Geiser F., Pavey C. R. (2007). Basking and torpor in a rock-dwelling desert marsupial: survival strategies in a resource-poor environment. J. Comp. Physiol. B. 177, 885–892. doi: 10.1007/s00360-007-0186-z
Geiser F., Ruf T. (2023). Long-term survival, temperature, and torpor patterns. Scient. Rep. 13, 6673. doi: 10.1038/s41598-023-33646-6
Geiser F., Ruf T. (1995). Hibernation versus daily torpor in mammals and birds: physiological variables and classification of torpor patterns. Physiol. Zool. 68, 935–966. doi: 10.1086/physzool.68.6.30163788
Geiser F., Turbill C. (2009). Hibernation and daily torpor minimize mammalian extinctions. Naturwissenschaften 96, 1235–1240. doi: 10.1007/s00114-009-0583-0
Gillooly J. F., Gomez J. P., Mavrodiev E. V. (2017). A broadscale comparison of aerobic activitylevelsinvertebrates: endotherms versus ectotherms. Proc. R. Soc B. 264, 20162328. doi: 10.1098/rspb.2016.2328
Glazier D. S. (1985). Relationship between metabolic rate and energy expenditure for lactation in Peromyscus. Comp. Biochem. Physiol. A 80, 587–590. doi: 10.1016/0300-9629(85)90417-7
Grabek K. R., Cooke T. F., Epperson L. E., Spees K. K., Cabral G. F., Sutton S. C., et al. (2019). Genetic variation drives seasonal onset of hibernation in the 13-lined ground squirrel. Commun. Biol. 2, 478. doi: 10.1038/s42003-019-0719-5
Grigg G. C., Beard L. A., Augee M. L. (2004). The evolution of endothermy and its diversity in mammalsandbirds. Physiol. Biochem. Zool. 77, 982–997. doi: 10.1086/425188
Hanna E., Cardillo M. (2014). Clarifying the relationship between torpor and anthropogenic extinction risk in mammals. J. Zool. 293, 211–217. doi: 10.1111/jzo.12136
Hare E., Leighton E. A. (2006). Estimation of heritability of litter size in Labrador Retrievers and German Shepherd dogs. J. Vet. Beh. 1, 62–66. doi: 10.1016/j.jveb.2006.06.001
Hayes J. P., Garland J. T., Dohm M. R. (1992). Individual variation in metabolism and reproduction of Mus: are energetics and life history linked? Func. Ecol. 6, 5–14. doi: 10.2307/2389765
Hinds L. A., Fletcher T. P., Rodger J. P. (1996). Hormones of oestrus and ovulation and their manipulationinmarsupials. Reprod. Fertil. Dev. 8, 661–672. doi: 10.1071/RD9960661
Hoelzl F., Bieber C., Cornils J. S., Gerritsmann H., Stalder G. L., Walzer C., et al. (2015). How to spend the summer? Free-living dormice (Glis glis) can hibernate for 11 months in non-reproductive years. J. Comp. Physiol. B. 185, 931–939. doi: 10.1007/s00360-015-0929-1
Humphries M. M., Thomas D. W., Kramer D. L. (2003). The role of energy availability in mammalian hibernation: a cost-benefit approach. Physiol. Biochem. Zool. 76, 165–179. doi: 10.1086/367950
James R. S., Tallis J., Angilletta M. J. (2015). Regional thermal specialisation in a mammal: temperature affects power output of core muscle more than that of peripheral muscle in adult mice (Mus musculus). J. Comp. Physiol. B. 185, 135–142. doi: 10.1007/s00360-014-0872-6
Johnson M. S., Thomson S. C., Speakman J. R. (2001). Limits to sustained energy intake: II. Inter-relationships between resting metabolic rate, life-history traits and morphology in Mus musculus. J. Exp. Biol. 204, 1937–1946. doi: 10.1242/jeb.204.11.1937
Johnston S. L., Souter D. M., Erwin S. S., Tolkamp B. J., Yearsley J. M., Gordon I. J., et al. (2007). Associations between basal metabolic rate and reproductive performance in C57BL/6J mice. J. Exp. Biol. 210, 65–74. doi: 10.1242/jeb.02625
Kalcounis-Rueppell M. C. (2007). Relationship of basal metabolism and life history attributes in Neotomine—Peromyscine rodents (Cricetidae: Neotominae). Ecoscience 14, 347–356. doi: 10.2980/1195-6860(2007)14[347:ROBMAL]2.0.CO;2
Kam M., Cohen-Gross S., Khokhlova I. S., Degen A. A., Geffen E. (2003). Average daily metabolic rate, reproduction and energy allocation during lactation in the Sundevall Jird Meriones crassus. Funct. Ecol. 17, 496–503. doi: 10.1046/j.1365-2435.2003.00754.x
Kemp T. S. (2005). The Origin And Evolution Of Mammals (Oxford: Oxford University Press). doi: 10.1093/oso/9780198507604.001.0001
Kenagy G. J., Sharbaugh S. M., Nagy K. A. (1989). Annual cycle of energy and time expenditureinagolden-mantled groundsquirrel population. Oecologia 78, 269–282. doi: 10.1007/BF00377166
Koteja P. (2000). Energy assimilation, parental care and the evolution of endothermy. Proc. R. Soc B. 267, 479–484. doi: 10.1098/rspb.2000.1025
Koteja P. (2004). The evolution of concepts on the evolution of endothermy in birds and mammals. Physiol. Biochem. Zool. 77, 1043–1050. doi: 10.1086/423741
Landes J., Pavard S., Henry P. Y., Terrien J. (2020). Flexibility is costly: hidden physiological damage from seasonal phenotypic transitions in heterothermic species. Front. Physiol. 11, 985. doi: 10.3389/fphys.2020.00985
Lane J. E., Kruuk L. E. B., Charmantier A., Murie J. O., Clotman D. W., Buoro M., et al. (2011). A quantitative genetic analysis of hibernation emergence date in a wild population of Columbian ground squirrels. J. Evolut. Biol. 24, 1949–1959. doi: 10.1111/jeb.2011.24.issue-9
Larivee M. L., Boutin S., Speakman J. R., McAdam A. G., Humphries M. M. (2010). Associations between over-winter survival and resting metabolic rate in juvenile North American red squirrels. Func. Ecol. 24, 597–607. doi: 10.1111/j.1365-2435.2009.01680.x
Levesque D. L., Lovegrove B. G. (2014). Increased homeothermy during reproduction in a basal placentalmammal. J. Exp. Biol. 217, 1535–1542. doi: 10.1242/jeb.098848
Lovegrove B. G. (2003). The influence of climate on the basal metabolic rate of small mammals: a slow-fastmetabolic continuum. J. Comp. Physiol. B. 173, 87–112. doi: 10.1007/s00360-002-0309-5
Lovegrove B. G. (2017). A phenology of the evolution of endothermy in birds and mammals. Biol. Rev. 92, 1213–1240. doi: 10.1111/brv.12280
McAllan B. M., Geiser F. (2014). Torpor during reproduction in mammals and birds: dealing with anenergeticconundrum. Am. Zool. 54, 516–532. doi: 10.1093/icb/icu093
Millesi E., Prossinger H., Dittami J. P., Fieder M. (2001). Hibernation effects on memory in European ground squirrels (Spermophilus citellus). J. Biol. Rhythms. 16, 264–271. doi: 10.1177/074873001129001971
Morris D. W. (1998). State-dependent optimization of litter size. Oikos. 83, 518–528. doi: 10.2307/3546679
Mountford M. D. (1968). The significance of litter size. J. Anim. Ecol. 37, 363–367. doi: 10.2307/2953
Nespolo R. F., Franco M. (2007). Whole-animal metabolic rate is a repeatable trait: a meta-analysis. J. Exp. Biol. 210, 2000–2005. doi: 10.1242/jeb.02780
Nespolo R. F., Verdugo C., Cortés P. A., Bacigalupe L. D. (2010). Bioenergetics of torpor in the microbiotheridmarsupial, monito del monte (Dromiciops gliroides): the role of temperature and food availability. J. Comp. Physiol. B. 180, 767–773. doi: 10.1007/s00360-010-0449-y
Oli M. K. (2004). The fast–slow continuum and mammalian life-history patterns: an empirical evaluation. Basic App. Ecol. 5, 449–463. doi: 10.1016/j.baae.2004.06.002
Partridge L., Harvey P. H. (1988). The ecological context of life history evolution. Science 241, 1449–1455. doi: 10.1126/science.241.4872.1449
Pedersen A. B., Greives T. J. (2008). The interaction of parasites and resources cause crashes in a wild mouse population. J. Anim. Ecol. 77, 370–377. doi: 10.1111/j.1365-2656.2007.01321.x
Perrins C. M., Moss D. (1975). Reproductive rates in thegreat tit. J. Anim. Ecol. 44, 695–706. doi: 10.2307/3712
Pettersen A. K., Marshall D. J., White C. R. (2018). Understanding variation in metabolic rate. J. Exp. Biol. 221, jeb166876. doi: 10.1242/jeb.166876
Pucek Z., Jędrzejewski W., Jędrzejewska B., Pucek M. (1993). Rodent population dynamics in a primeval deciduous forest (Białowieża National Park) in relation to weather, seed crop, and predation. Acta Theriol. 38, 199–232. doi: 10.4098/0001-7051
Racey P. A., Swift S. M. (1981). Variations in gestation length in a colony of pipistrelle bats (Pipistrelluspipistrellus) from year to year. Reproduction. 61, 123–129. doi: 10.1530/jrf.0.0610123
Réale D., Festa-Bianchet M., Jorgenson J. T. (1999). Heritability of body mass varies with age and season in wild bighorn sheep. Heredity 83, 526–532. doi: 10.1038/sj.hdy.6885430
Reznick D. (1985). Costs of reproduction: an evaluation of theempirical evidence. Oikos. 44, 257–267. doi: 10.2307/3544698
Ringsby T. H., Sæther B. E., Solberg E. J. (1998). Factors affecting juvenile survival in house sparrow Passer domesticus. J. Avian Biol. 29, 241–247. doi: 10.2307/3677106
Risch T. S., Dobson F. S., Murie J. O. (1995). Is mean littersize the most productive? A test Columbian groundsquirrels. Ecology. 76, 1643–1654. doi: 10.2307/1938165
Risch T. S., Michener G. R., Dobson F. S. (2007). Variation in litter size: a test of hypotheses in Richardson’s ground squirrels. Ecology 88, 306–314. doi: 10.1890/06-0249
Roff D. A., Fairbairn D. J. (2007). The evolution of trade-offs: where are we? J. Evol. Biol. 20, 433–447. doi: 10.1111/j.1420-9101.2006.01255.x
Rojas A. D., Körtner G., Geiser F. (2012). Cool running: locomotor performance at lowbody temperatureinmammals. Biol. Lett. 8, 868–870. doi: 10.1098/rsbl.2012.0269
Rojas A. D., Körtner G., Geiser F. (2014). Torpor in free-ranging antechinus: does it increase fitness? Naturwissenschaften 101, 105–114. doi: 10.1007/s00114-013-1136-0
Royo J., Aujard F., Pifferi F. (2019). Daily torpor and sleep in a non-human primate, the gray mouse lemur (Microcebus murinus). Front. Neuroanat. 13, 87. doi: 10.3389/fnana.2019.00087
Ruf T., Bieber C., Turbill C. (2012). “Survival, aging, and life-history tactics in mammalian hibernators,” in Living in a seasonal world. Eds. Ruf T., Bieber C., Arnold W., Millesi E. (Springer, Berlin, Heidelberg), 123–132.
Ruf T., Geiser F. (2015). Daily torpor and hibernation in birds and mammals. Biol. Rev. 90, 891–926. doi: 10.1111/brv.12137
Sadowska J., Gębczyński A. K., Konarzewski M. (2013). Basal metabolic rate is positively correlated with parental investment in laboratory mice. Proc. R. Soc B. 280, 20122576. doi: 10.1098/rspb.2012.2576
Schai-Braun S. C., Steiger P., Ruf T., Arnold W., Hackländer K. (2021). Maternal effects on reproduction in the precocial European hare (Lepus europaeus). PloS One 16, e0247174. doi: 10.1371/journal.pone.0247174
Schwartz T. S., Bronikowski A. M. (2013). Dissecting molecular stress networks: identifyingnodesofdivergence between life-history phenotypes. Mol. Ecol. 22, 739–756. doi: 10.1111/j.1365-294X.2012.05750.x
Seebacher F., Little A. G. (2017). Plasticity of performance curves can buffer reaction rates from body temperature variation in active endotherms. Front. Physiol. 8, 575. doi: 10.3389/fphys.2017.00575
Speakman J. R., Król E., Johnson M. S. (2004). The functional significance of individual variation in basal metabolic rate. Physiol. Biochem. Zool 77, 900–915. doi: 10.1086/427059
Steyermark A. C. (2002). A high standard metabolic rate constrains juvenile growth. Zoology 105, 147–151. doi: 10.1078/0944-2006-00055
Strijker B. N., Iwińska K., van der Zalm B., Zub K., Boratyński J. S. (2023). Is personality and its association with energetics sex-specific in yellow-necked mice Apodemus flavicollis? Ecol. Evol. 13, e10233. doi: 10.1002/ece3.10233
Symonds M. R. (1999). Life histories of the Insectivora: the role of phylogeny, metabolism and sex differences. J. Zool. 249, 315–337. doi: 10.1111/j.1469-7998.1999.tb00768.x
Thomas S. C., Coltman D. W., Pemberton J. M. (2002). The use of marker-based relationship information to estimate the heritability of body weight in a natural population: a cautionary tale. J. Evol. Biol. 15, 92–99. doi: 10.1046/j.1420-9101.2002.00372.x
Thomas D. W., Geiser F. (1997). Periodic arousals in hibernating mammals: is evaporative water loss involved? Func. Ecol. 11, 585–591. doi: 10.1046/j.1365-2435.1997.00129.x
Tuomi J. (1980). Mammalian reproductive strategies: a generalized relation of litter size to body size. Oecologia 45, 39–44. doi: 10.1007/BF00346705
Turbill C., Bieber C., Ruf T. (2011). Hibernation is associated with increased survival and the evolution of slow life histories among mammals. Proc. R. Soc B. 278, 3355–3363. doi: 10.1098/rspb.2011.0190
Turbill C., McAllan B. M., Prior S. (2019). Thermal energetics and behaviour of a small, insectivorous marsupial in response to the interacting risks of starvation and predation. Oecologia 191, 803–815. doi: 10.1007/s00442-019-04542-6
Turbill C., Stojanovski L. (2018). Torpor reduces predation risk by compensating for the energetic cost of antipredator foraging behaviours. Proc. R. Soc B. 285, 20182370. doi: 10.1098/rspb.2018.2370
Weller A. K., Chapman O. S., Gora S. L., Guralnick R. P., McLean B. S. (2023). New insight into drivers of mammalian litter size from individual-level traits. Ecography, e06928. doi: 10.1111/ecog.06928
White C. R., Schimpf N. G., Cassey P. (2013). The repeatability of metabolic rate declines with time. J. Exp. Biol. 216, 1763–1765. doi: 10.1242/jeb.076562
Williams G. C. (1966). Natural selection, the costs of reproduction, and a refinement of Lack’s principle. Am. Nat. 100, 687–690. doi: 10.1086/282461
Willis C. K. R., Brigham R. M., Geiser F. (2006). Deep, prolonged torpor by pregnant, free-rangingbats. Naturwissenschaften 93, 80–83. doi: 10.1007/s00114-005-0063-0
Keywords: heterothermy, daily torpor, reproduction, fitness, body size, metabolic rate, body temperature
Citation: Boratyński JS, Iwińska K and Zub K (2024) Heterothermy use in winter is associated with reduced litter size during following breeding season. Front. Ecol. Evol. 12:1365549. doi: 10.3389/fevo.2024.1365549
Received: 04 January 2024; Accepted: 16 February 2024;
Published: 04 March 2024.
Edited by:
Daniel Becker, University of Oklahoma, United StatesReviewed by:
Qingsheng Chi, Zunyi Normal College, ChinaCopyright © 2024 Boratyński, Iwińska and Zub. This is an open-access article distributed under the terms of the Creative Commons Attribution License (CC BY). The use, distribution or reproduction in other forums is permitted, provided the original author(s) and the copyright owner(s) are credited and that the original publication in this journal is cited, in accordance with accepted academic practice. No use, distribution or reproduction is permitted which does not comply with these terms.
*Correspondence: Jan S. Boratyński, amFuLmJvcmF0eW5za2lAZ21haWwuY29t
Disclaimer: All claims expressed in this article are solely those of the authors and do not necessarily represent those of their affiliated organizations, or those of the publisher, the editors and the reviewers. Any product that may be evaluated in this article or claim that may be made by its manufacturer is not guaranteed or endorsed by the publisher.
Research integrity at Frontiers
Learn more about the work of our research integrity team to safeguard the quality of each article we publish.