- Integrative Ecophysiology, Alfred Wegener Institute Helmholtz Centre for Polar and Marine Research, Bremerhaven, Germany
Recently, we could show that scallops show limitations of muscular performance like a reduced force under ocean warming and acidification. However, the underlying mechanisms at the cellular level are not completely understood. Metabolomics has become a valuable tool to evaluate the responses of marine organisms to various stressors. In the present study we therefore used a semi-targeted, multi tissue NMR based metabolomic approach to analyze metabolite patterns in the Atlantic king scallop, Pecten maximus, that were long-term acclimated to different end of century conditions of ocean warming (OW), ocean acidification (OA) and their combination (OWA). We investigated tissue specific metabolic profiles and metabolite concentrations in frozen tissues from gills, mantle and phasic and tonic adductor muscle of P. maximus under present conditions using 1H-HR-MAS NMR spectroscopy. A set of 33 metabolites revealed a clear tissue-specific pattern which can be attributed to the individual functions of the respective tissue type. We then evaluated the impact of OW, OA and OWA on the metabolic profiles of the different tissues. OW was the main driver of the changes in metabolites. In particular, energy-related metabolites seem to play an important role in the physiological response of scallops to OW and OWA. In combination with pathway analysis and network exploration we propose a possible correlation between metabolic changes in the adductor muscle and limited swimming performance of P. maximus under future climate. While the metabolic response of the phasic muscle seems to mainly depend on net consumption of energy related metabolites such as ATP and phospho-L-arginine, the tonic muscle seems to rely on metabolizing specific amino acids and beta-oxidation to account for the elevated energetic requirements under ocean warming and acidification.
1 Introduction
Increasing greenhouse gas emissions are not only responsible for the ongoing global warming trend, but as anthropogenic CO2 has been taken up by the oceans (Bindoff et al., 2019; Licker et al., 2019), they also lead to a decrease in ocean pH through the marine carbonate system (Zeebe, 2012). This process known as “ocean acidification” (OA) is a global phenomenon and called the evil twin of warming, since both ocean warming and acidification are occurring in parallel. It is therefore important to investigate the influence of both factors on the physiology of marine organisms in order to be able to make realistic statements about climate-induced changes in marine ecosystems (Pörtner et al., 2014; Gattuso et al., 2018; Landrigan et al., 2020). Attempts to gain an understanding of the combined effects of elevated temperatures and PCO2 levels on marine species in their ecosystem have been underway for some time (Doney et al., 2012; Byrne and Przeslawski, 2013), and the effects of these climate variables turned out to be context and species-dependent (Harvey et al., 2013). The combination of both factors has recently been reviewed (Baag and Mandal, 2022) and literature shows that ocean warming is the main driver affecting an organism’s metabolism while the effects of acidification play more of an additive role (e.g. Pinsky et al., 2019; Matoo et al., 2021).
For water-breathing poikilotherms whose body temperature varies with environmental temperature, the concept of oxygen and capacity limited thermal tolerance highlights that, with increasing temperature, marine ectotherms experience progressive hypoxemia and finally, when reaching the critical temperature, rely on anaerobic ATP production to fuel the rising gap in energy demand, which is no longer supported by solely aerobic mitochondrial ATP production (Pörtner, 2002; Pörtner et al., 2017). Accordingly, since the aerobic power budget (excess of aerobic energy after maintenance costs are covered; Guderley and Pörtner, 2010; Pörtner et al., 2017) is limited and an organism cannot rely long-term on anaerobiosis, there are trade-offs between an organism’s performance parameters (growth, exercise, reproduction) and there are bottlenecks in an organism’s performance capacity under stressful (energy-demanding) environmental conditions such as ocean warming and acidification (OWA).
The impact of ocean acidification on marine life is manyfold (see reviews by e.g. Melzner et al., 2020; Doney et al., 2020; Findlay et al., 2022) and various calcifiers suffer from reduced growth and calcification rates even though the previously high level of concern about the fate of calcifiers in a future ocean no longer holds (see reviews by Zhao et al., 2020; Leung et al., 2022). Bivalves have a limited capacity for acid base regulation and are experiencing a drop in extracellular pH when exposed to OA (Lannig et al., 2010; Heinemann et al., 2012; Gazeau et al., 2014; Zhao et al., 2017) including the scallop Pecten maximus (Schalkhausser et al., 2013; Schalkhausser et al., 2014). Such studies indicate that OA impact and sensitivity differ between wild and aquaculture animals (Richards et al., 2015; Stapp et al., 2018). Among bivalves, scallops are unique due to their swimming (escape) behavior via jet propulsion through adductor muscle contractions (Guderley and Tremblay, 2016). The adductor muscle consists of two types that interact during swimming exercise (Pérez et al., 2008; Tremblay et al., 2012; Guderley and Tremblay, 2016). The striated phasic muscle, which is essentially responsible for swimming through claps, and the smaller smooth tonic muscle, which is mainly responsible for closing the shells and holding them closed (Chantler, 2006; Sun et al., 2018) During swimming, muscle contraction cannot solely be fueled by mitochondrial oxidative phosphorylation and is highly dependent on ATP generation from phosphate reserves like phospho-L-arginine (PLA) and anaerobic glycolysis (Guderley and Tremblay, 2016). In comparison to other bivalves like oysters or mussels that are able to colonize the intertidal zone, their active lifestyle gives scallops less flexibility to cope with changing environmental factors (Guderley and Pörtner, 2010; Ivanina and Sokolova, 2016; Götze et al., 2020).
1.1 Previous observations on scallops swimming performance
In previous studies, we investigated the influence of ocean warming and acidification on swimming performance of the king scallop, Pecten maximus (Schalkhausser et al., 2013, 2014). According to Schalkhausser et al. (2014) P. maximus’ swimming ability was affected by warming more than by acidification (PCO2 0.112 kPa). While the number of claps to exhaustion was similar for P. maximus between 10°C and 20°C, the force of the phasic adductor muscle was significantly reduced under long-term acclimation to 20°C. The time to exhaustion was reduced and the recovery period prolonged in warm-exposed P. maximus. Warming resulted in a significant decrease in hemolymph oxygen levels (extracellular partial pressure of O2: PeO2). In combination with a significant increase in respiration rate at 20°C, the nearly 50% reduction in PeO2 indicated that P. maximus had surpassed its optimal temperature range and was exhibiting a progressive warming-induced mismatch between aerobic energy supply and demand (Pörtner et al., 2017). In a subsequent study on P. maximus exposed long-term to OW (20°C, 0.04 kPa PCO2) and OWA (20°C, 0.112 kPa PCO2; Bock et al., 2019), we investigated the availability of energy-rich phosphates, in particular PLA and inorganic phosphate (Pi) using in vivo 31P NMR spectroscopy and calculated the mitochondrial maximum surplus oxidative flux of the phasic muscle. In both OW- and OWA-exposed scallops, PLA and maximum surplus oxidative flux of phasic muscle were reduced, which was attributed to the lower PeO2 in the haemolymph found in OW- and OWA-exposed scallops described in Schalkhausser et al. (2014).
The aim of the present study was to use state-of-the-art metabolomics and thereby elucidate the mechanisms at the cellular level which underpin the performance limitations on the organism-level in P. maximus. Metabolomics enables the holistic tracking of changing metabolic processes in response to an external stimulus such as environmental changes (Viant, 2008, for a recent review see Wishart, 2019). Metabolomics is believed to unravel the phenotype of an organism from the response to an external stimulus (Fiehn, 2002; Wishart et al., 2022). Using tissue samples from our previous experiments (see Schalkhausser et al., 2014), we applied a semi-untargeted metabolomics approach based on NMR spectroscopy to a multi-tissue dataset for obtaining tissue specific insights into the metabolic response of Pecten maximus. In addition, metabolic responses of the adductor muscle were then related to whole-animal performance and capacity parameters (clapping response and oxygen consumption of P. maximus as described in Schalkhausser et al., 2014) to gain a more integrative understanding. To further support our interpretation of the data, a pathway analysis was complemented by a network exploration. This combination enables a better interpretation of the metabolite changes and the generation of testable experimental hypotheses. In particular, we focused on potential pathway modifications of the intermediary metabolism of the two adductor muscle types (phasic and tonic muscle). We propose a cellular mechanism that might explain the observed limitations in whole-animal performance of P. maximus under future climate change (Schalkhausser et al., 2014; Bock et al., 2019).
2 Materials and methods
2.1 Experimental design and tissue collection
All investigations were performed on tissue samples that were taken from our experiment described in Schalkhausser et al. (2014). Briefly, wild living Atlantic king scallop Pecten maximus with similar size were collected by SCUBA divers at Morlaix Bay (Baie de Morlaix, Les Grandes Fourches, France; 48°42′33.6″N, 3°55′59.30″W) and transported to the Alfred Wegener Institute (Bremerhaven, Germany). Animals were considered as typical from the sampling spot. After recovery from transportation for 2 weeks, scallops were cleaned from epibionts and randomly placed in recirculation aquarium systems and exposed to two different temperatures (10°C; C vs. 20°C; OW) and two different PCO2 values (~0.04 kPa (400 μatm); OA vs. ~0.112 kPa (1,120 μatm); OWA) for at least 50 days. Three times per week scallops were fed live phytoplankton (DT´s Premium Reef Blend). Mortality was 9% for the C group, 0% for OA, 20% for OW and 9% in OWA group at the end of the experiment (Nov. 2011), but not statistically different between groups (see Schalkhausser et al., 2014). Scallops were dissected on ice, tissues (gills, mantle, phasic and tonic muscle of the adductor muscle) were freeze-clamped, shock-frozen in liquid nitrogen and stored at −80°C until present metabolite analyses were performed.
2.2 Metabolite analysis via NMR spectroscopy
Metabolite profiling of the different tissues was conducted using high resolution magic angle spinning nuclear magnetic resonance spectroscopy (1H-HR-MAS NMR spectroscopy) using a wide-bore 400 MHz NMR spectrometer (9.4 T WB with Avance III HD electronics, Bruker Biospin, Germany) equipped with a triple tuned 1H-13C-31P-HR-MAS NMR probe. All measurements were performed on frozen tissue samples similar to Podbielskie et al. (2016). Briefly, a sample was taken of the frozen organ of interest using a biopsy punch needle (3 mm diameter) and immediately placed in a standard zirconium HR-MAS rotor for untargeted metabolite profiling based on 1H-NMR spectroscopy. A drop of D2O (containing 0.05% TSP as standard) was added to the sample for lock and calibration purposes. All samples were measured at 4°C at a spinning rate of 3000 Hz using the Bruker acquisition software TopSpin 3.5pl. NMR parameters were as follows (see also Schmidt et al., 2017; Rebelein et al., 2018): pulse program: 1D-Carr-Purcell-Meiboom-Gill (CPMG) pulse train including f1 presaturation (Bruker protocol cpmgpr1d), 90° bp pulse length 8.4 μs, time domain 70,656, sweep width of 8802 Hz (22 ppm), acquisition time 4.01 s, relaxation delay 4 s, four dummy scans and 64–256 number of scans depending on the signal-to-noise ratio.
2.3 NMR processing
All NMR data were processed and analyzed using Chenomx NMR suite 8.4 professional suite (Chenomx Inc., Canada). Data were automatically zero-filled, processed with an exponential multiplication of 0.3 Hz, phase and base-line corrected using the automatic functions within Chenomx. The spectra were automatically shim-corrected and calibrated to the TSP standard. After completion of the processing procedure, all spectra from each individual tissue of the single experimental groups were overlaid within the Chenomx profiler. The superposition of the individual spectra allows a quick overview of the quality of the individual NMR spectra and the assessment of comparability. In this way, differences in the line widths and shifts of individual NMR signals can be specifically identified and different baselines between the spectra can be recognized. These factors are essential for an automatic evaluation of metabolite profiles. Shifts of NMR signals, just like different baselines between individual spectra, would lead to an erroneous determination of metabolite concentrations. Interestingly, there was no need for alignment shifts of NMR signals in individual spectra. Spectra that differed in baseline in comparison to the other NMR spectra were marked and later identified as potential outliers within MetaboAnalyst (MetaboAnalyst 5.0., see below). A standard metabolite profile from scallop gill tissue from our previous study (Götze et al., 2020) was overlaid to an example spectrum of each tissue and checked for consistency of the metabolite specific NMR peaks. A set of 33 metabolites was identified and was used for a targeted binning procedure on batches of spectra from each tissue within Chenomix. The water region (4.7–5.25 ppm) was excluded and removed from the spectra. Integrals of the metabolite specific bins were then automatically calculated in arbitrary units, normalized to integers and exported into a csv file. For further comparative statistical analysis, the csv files of the individual spectra were merged. For this, only those bins from the individual metabolites were used for the additional analysis that were distinct for the identification and quantification of the metabolite profiles, or showed a significant content, to get an unambiguous assignment of the metabolites. This included both the integral and the position of the bins in the spectrum that could be uniquely identified.
2.4 Statistics
All spectral data were analyzed for differences in spectral patterns and individual metabolites using the web-based analysis tool MetaboAnalyst (MetaboAnalyst 5.0). The entire analytic procedure followed the recommendation for metabolomic analysis as described in Chong et al. (2019) and corresponding YouTube tutorials of the same group. In a first step, the csv files of the metabolite specific bins were imported into MetaboAnalyst and normalized using pareto scaling as recommended. The data were checked for outliers using an operator controlled visual inspection of the particular heatmaps in combination with an unsupervised principal component analysis (PCA). Identified outliers were removed from the list before further analysis (4 samples for gill and 1 sample for tonic muscle). The identified outliers matched the spectra that had already been marked, as they had different baselines compared to the other NMR spectra (see above). Dendrograms of the data excluding the outliers were used to observe clusters of specific sample spectra between groups. Differences in patterns and classification discrimination between groups were determined by Partial Least Square-discriminant analysis (PLS-DA). Metabolites of interest were classified using the VIP (variance of importance) score of the PLS-DA. Classification and cross validation by permutation tests based on separation distance were performed in view of possible overfitting and meaning fullness of the PLS-DA. A significance analysis of microarray/metabolites (SAM) analysis was performed to identify significant different metabolites between groups. The delta value to control the false discovery rate (FDR) was set conservatively to the highest possible level (leading to a maximum FDR of 0.1). For the discussion we only considered those metabolites that showed definite significant changes in SAM. In addition, ANOVA combined with a Post-Hoc test (Fisher’s) was used to underpin the results from the SAM analysis where possible. To better understand the interdependencies of the altered metabolites and a possible link to the observed limitations at the whole-animal level, a pathway analysis (PW analysis) was performed in combination with a network (NW) exploration in the muscle samples of control and warming exposed scallops. While the PW analysis establishes a connection to metabolic pathways on the basis of the altered metabolites, the NW exploration looks at the interaction of metabolites and their exchange and thus points to novel interactions. However, the two methods only allow two groups to be compared with each other. Due to its specific role in swimming performance, these analyses were therefore performed on the adductor muscle between the control group and warming. For the network exploration, the metabolite interactions between the control group and the respective experimental groups were also included for comparison. The pathway analysis was performed within MetaboAnalyst, similar to (Götze et al., 2020), to get an idea of the long-term induced changes in metabolic pathways limiting the swimming performance of the adductor muscle. Only those pathways with a significant impact >0.1 were taken into account. For network exploration a metabolite-metabolite interaction network based on STITCH (“search tool for interactions of chemicals”) was used to elucidate potential linkages. STITCH is a searchable database that includes interactions for over 300,000 molecules and 1.6 million proteins. The similarity of chemical structures in combination with text mining are used to make predictions about relationships between molecules. For the network exploration the filter for nodes based on degree and a filter for nodes based on betweenness were adjusted for each exploration individually to reduce the complexity of the network and to identify those metabolites that can act as specific bottlenecks in the metabolic network (see figure legends for exact values). Both analyses were only performed on phasic and tonic muscle tissues to underline the particular interaction of the two tissues in relation to the observed changes in swimming performance of scallops under warming conditions.
3 Results
Figure 1 shows a comparative overview of 1H-HR-MAS NMR spectra of the four tissues examined from Pecten maximus under control incubations. Although signal intensity varied between investigated tissue types, tissue-specific spectral patterns were quite similar. All large and medium sized signals are seen in each spectrum and there were no obvious additional signals that were observed in one or some tissues only. A set of 33 metabolites was automatically aligned to all spectra of tissue type. A closer look at the individual spectra, however, revealed tissue-specific differences between metabolite profiles with respect to signal intensity, and thus metabolite concentration. The spectrum from the mantle tissue biopsy showed the broadest line widths in relation to the standard (TSP). However, the quality was sufficient for a comparative approach between tissues (see Supplementary for example spectra of the different tissues). Figure 1 displays a multivariate comparison between the tissues by an unsupervised principal component analysis (PCA) and a supervised partial least square discriminant analysis (PLS-DA). Both analyses highlight significant differences in the metabolite profiles between the individual tissues, which were also evident in the visual comparison of the individual NMR spectra (see Supplementary), including differences between the two muscle types. The three principal components of the PCA explained 94.3% of the total variance (Figure 1A) and the three components of the PLS-DA produced a clear separation between the four tissue types. Interestingly, more than 70% were explained by the first component (Figure 1B). The clear separation is confirmed by the corresponding scree plot for the PCA. The cross validation as well as the permutation tests for the PLS-DA indicated a good prediction capability and meaningfulness (see Supplementary). The significantly different metabolite concentrations and the corresponding correlations between tissues after Anova analysis and post hoc test are shown in Table 1. Gills and mantle showed the highest concentrations of osmotically relevant metabolites such as imidazole, homarine and glycine, as well as of the two sugars (glucose and UDP glucose). In contrast, the two muscle tissues showed increased concentrations of the membrane-relevant metabolites choline and O-phosphocholine. L-arginine and carnitine were also significantly more concentrated in the two muscle types compared to gill and mantle. Interestingly, the tonic muscle showed metabolite concentrations that were not observed as prominently in the other tissues. These were high concentrations of hypotaurine, tyrosine, methionine, succinate and lactate, whereas ATP concentrations were lowest. The phasic muscle contained the highest concentrations of the amino acids alanine, glutamate and glutamine and of acetate, whereas the gill tissue exhibited the highest trigonelline levels. The mantle showed high concentrations of threonine and valine, but very low betaine concentrations compared to the other tissues.
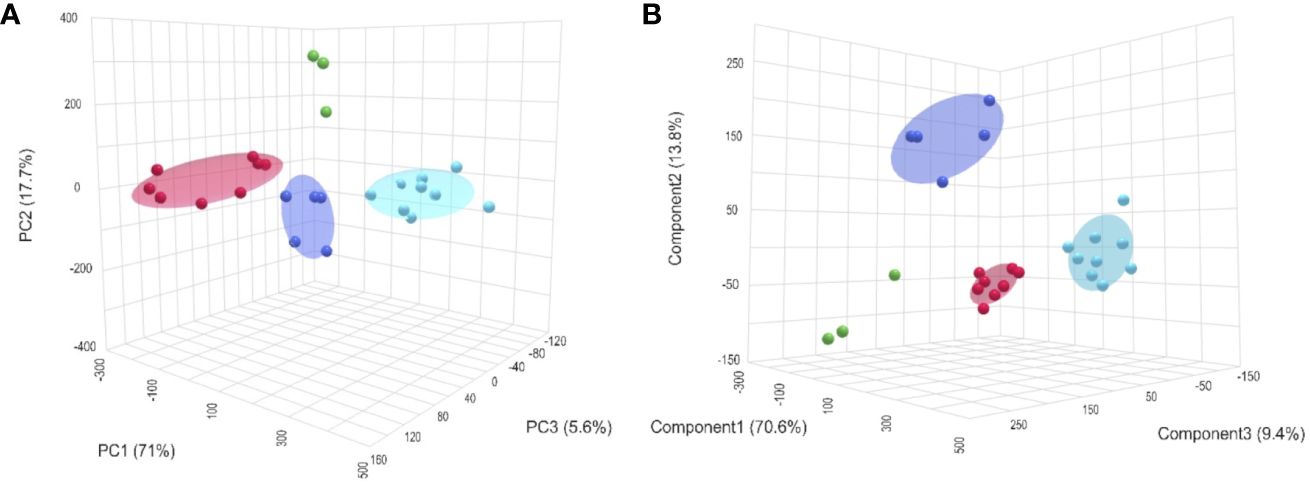
Figure 1 3D views of a PCA (A) and a PLS-DA (B) across the metabolite profiles of all four tissue types (gills = red; mantle = green; phasic muscle = blue and tonic muscle = cyan) of the king scallop, P. maximus exposed to control conditions (normocapnia at 10°C). The unsupervised PCA showed a clear separation of all four tissues and the supervised PLS-DA resulted in a distinct classification between tissues. The ellipsoids indicate the respective confidence areas (not available for mantle n=3).
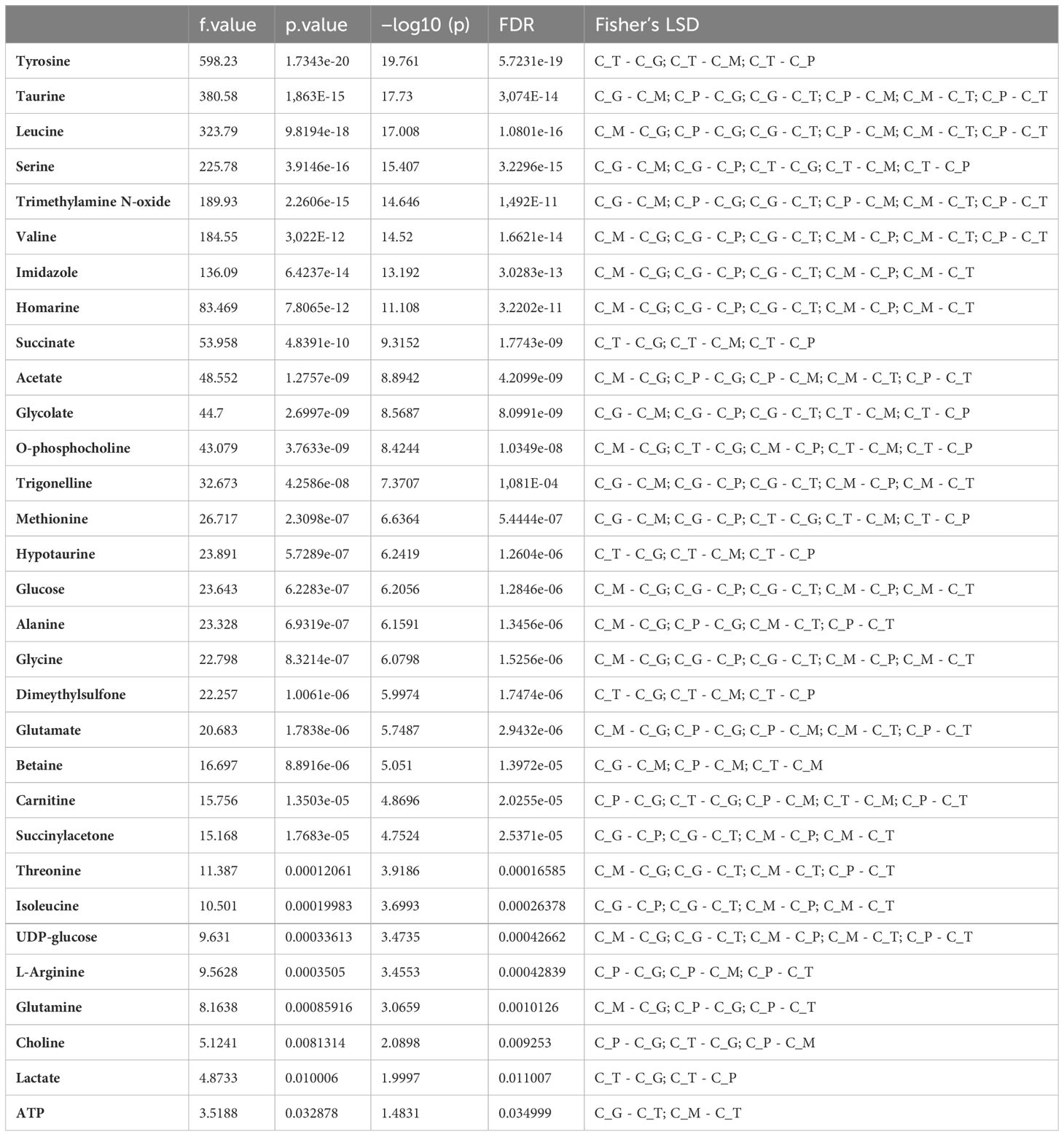
Table 1 Summary of the results of the ANOVA test presenting the significant differences in metabolite concentrations between tissue types (gills (C_G), mantle (C_M), phasic (C_P) and tonic muscle (C_T)) of the king scallop, P. maximus exposed to control conditions (normocapnia at 10°C).
The metabolic response to ocean acidification (OA), warming (OW) and the combination of both (OWA) did partially differ between tissue types. Figure 2 shows a graphical representation of the significant treatment-dependent metabolite changes of gill and phasic muscle tissues. According to the SAM technique, tonic and mantle tissue did not show any significant changes. In gill tissue only the energy related metabolite ATP changed in comparison to control conditions, with ATP levels showing a decrease under OW and OWA (Figure 2A). Most of the changes in concentration were observed in the phasic muscle. Here, eleven metabolites showed concentration changes. These were the essential amino acids leucine and threonine, but also amino acids involved in energy metabolism, such as L-arginine, alanine and glutamine and glutamate, which were lower in the warm groups compared to the control. Acetate and O-phosphocholine, which are utilized in membrane lipid metabolism, were also lower in the warming groups. O-phosphocholine was lowest in the OA group. A similar pattern was observed for lactate. In addition, succinylacetone showed decreased levels in the warming groups. However, glycolate showed increased concentrations under OW and OWA (Figure 2B).
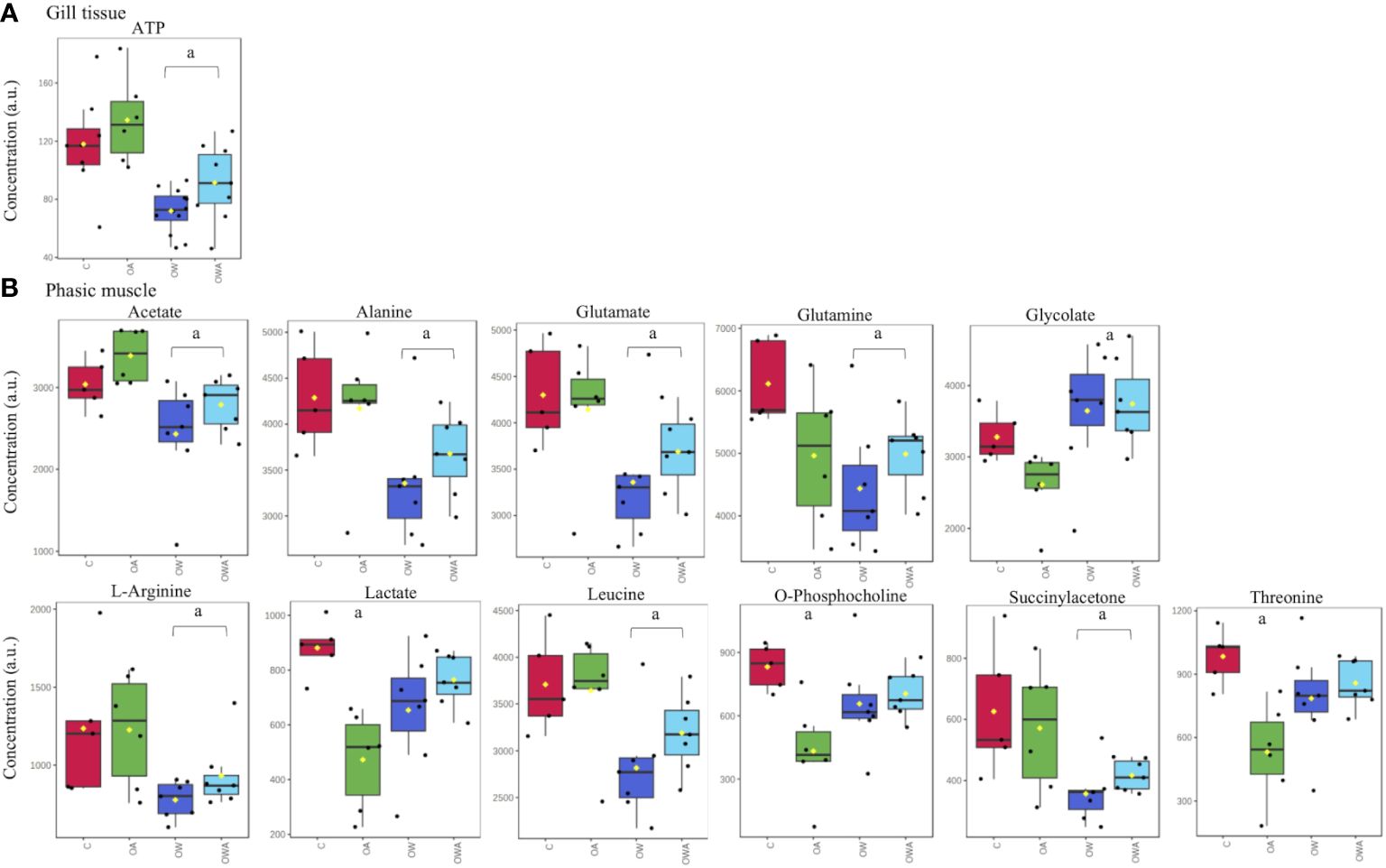
Figure 2 Changes in gill tissue (A) and phasic muscle (B) metabolite concentrations in P. maximus exposed to different treatments, controls (C: 10°C, 0.04 kPa PCO2), ocean acidification (OA: 10°C, 0.112 kPa PCO2), ocean warming (OW: 20°C, 0.04 kPa PCO2) and combination of both (OWA: 20°C, 0.112 kPa PCO2). Significant differences to control are marked with "a”.
3.1 Pathway and network analysis
A combination of pathway analysis and network correlation were performed on the phasic and tonic tissues from the adductor muscle in particular to get more insights of the metabolic mechanisms behind the swimming performance limits observed under ocean warming in comparison to control conditions. The metabolic pathways with the greatest impact identified in the PW analysis are listed in Table 2. The comparison between the metabolic profiles of the adductor muscle of control and OW-exposed scallops identified changes in four important pathways. All of them are directly related to arginine and energy metabolism. A Network (NW) exploration of metabolite–metabolite interactions is presented in Figure 3. The entire network consisted of 647 nodes, 2000 edges and 26 seeds (see insert on the left corner of Figure 3). A reduction of the network to the most important metabolites (nodes) and their connections (betweenness), highlights the central role of L-arginine, in addition to ATP, in the network. L-arginine is directly linked to oxygen, ATP and their degradation products, indicating a central role of L-arginine in energy supply during long-term acclimatization to warming in the adductor muscle. In addition, it is noteworthy that the amino acid L-methionine is in close proximity to L-arginine and ATP and that L-arginine is directly connected to carbon dioxide and sodium. In addition, network explorations from the phasic muscle under OA, OW and OWA were performed for comparison. The reduced networks confirmed the central role of L-arginine under OW and OWA, whereas under OA the metabolite phosphorylcholine was most connected (see Supplementary).

Table 2 Major metabolic pathways identified by PW analysis contributing to the metabolic response of the adductor muscle (phasic and tonic muscle) of P. maximus to long-term exposures to ocean warming (OW: 20°C, 0.112 kPa PCO2).
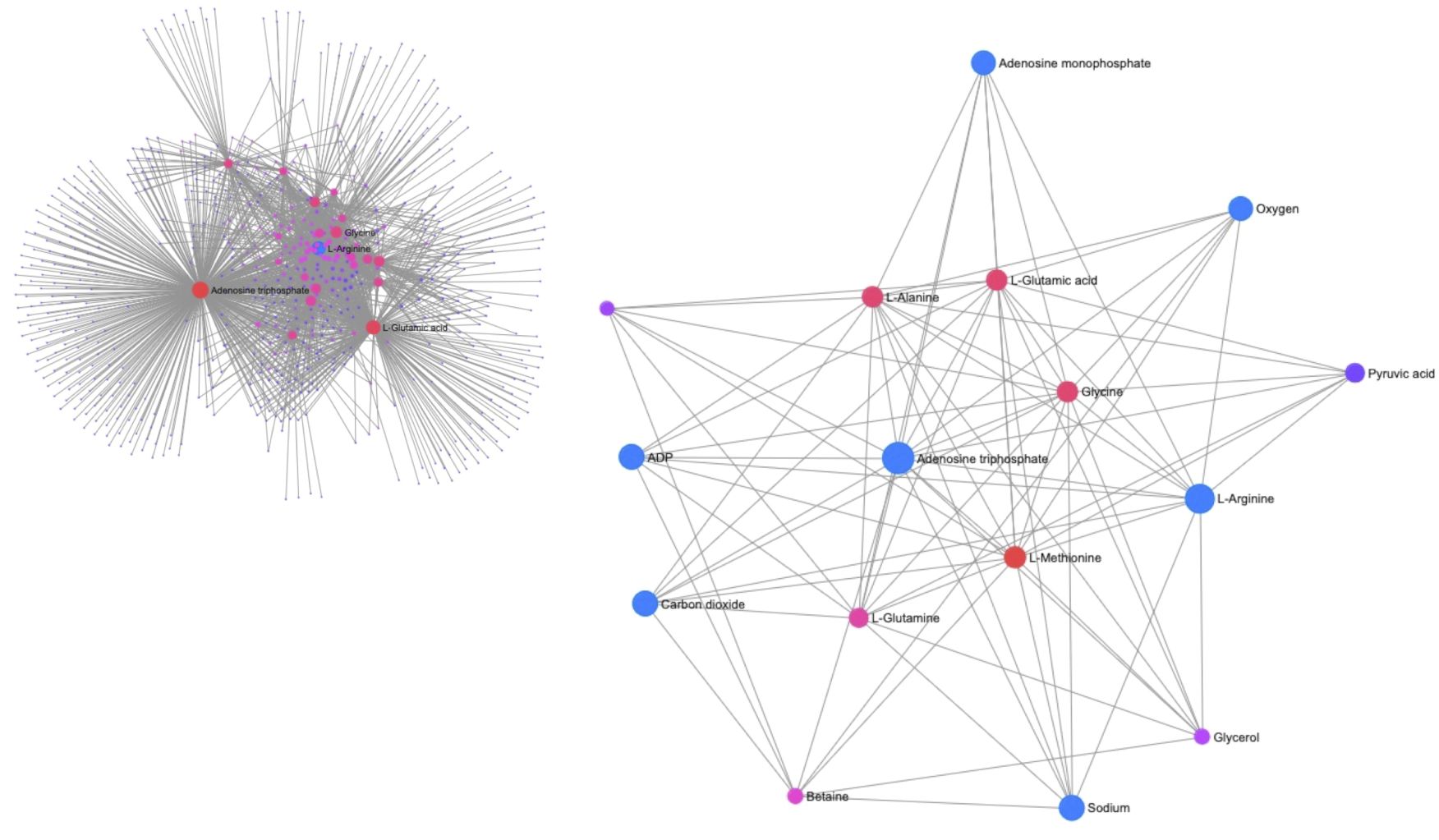
Figure 3 Plot of the metabolite-metabolite interaction network of phasic and tonic muscle tissue between control and long-term OW exposed P. maximus. The insert in the upper left corner shows the entire network, while the center presents a subnetwork reduced to metabolites with strong connections. Both ATP and L-arginine have a central role in the network. The interaction of L-arginine with ATP, ADP and AMP and with oxygen, carbon dioxide and sodium is marked by bigger blue circles. The filter for nodes based on degree were set to 15 and a filter for nodes based on betweenness were set to 5.
4 Discussion
In the present study, a retrospective metabolomic analysis using 1H-HRMAS NMR spectroscopy was conducted using tissue samples of the king scallop, Pecten maximus, from long-term incubation experiments. Our aim was to gain a better understanding of the cellular mechanisms that respond to ocean warming (OW) and acidification (OWA) in scallops, and to relate the observed changes in metabolic pathways to the reduction in swimming performance (Schalkhausser et al., 2013, Schalkhausser et al., 2014; Bock et al., 2019).
4.1 Tissue-specific metabolite concentrations
Following our previously published targeted metabolomics approach we could identify a set of 33 metabolites for all tissues, similar to that defined in gill tissue of P. maximus (Götze et al., 2020). Similarly, Cappello et al. (2018) found only small differences between the metabolic profiles of mussel Mytilus galloprovincialis tissues (gills, phasic muscle and digestive gland). While a main set of metabolites is conserved across all tissue types, metabolite concentrations varied strongly between tissues indicating a differential prioritization of metabolic pathways in each organ.
In gills we found high concentrations of methionine and glycolate. Both metabolites have in common that they have antioxidant properties (methionine: Martínez et al., 2017; glycolate for mitochondria: Diez et al., 2021). The main function of gills is gas exchange making their large surface areas a target for (external and internal) fluctuations of oxygen radicals. In coastal environments, in particular in lagoons or tidal pools, oxygen levels can vary from hyperoxic during day time to hypoxic conditions during night time. Thus, it is not surprising that gills possess a higher concentration of metabolites associated with oxidative stress protection than muscle. Furthermore, gill tissue, which has an important role in osmo- and ion-regulation relies on organic osmolytes, such as compatible solutes like homarine, the sugars glucose and UDP-glucose, but also the amino acid glycine. The high imidazole concentration in gills can be explained by the strong emphasis on effective pH control (Götze et al., 2020). Other amino acids such as isoleucine, serine and valine are highly concentrated in gills due to their importance in protein synthesis and degradation under osmotic stress (Haider et al., 2019). Trigonelline was also found in high concentrations and is known as a plant alkaloid, but has also been found in a variety of marine invertebrates, including bivalves (Cappello et al., 2018; Poulin et al., 2018; Götze et al., 2020; Frizzo et al., 2021; Zhou et al., 2023). The exact function of alkaloids in animals is not yet clear, but trigonelline is supposed to have various biological functions. In crustaceans urinary trigonelline was shown to serve as a chemical sensor for predator–prey interaction (Poulin et al., 2018) but it also to play a role in glucose metabolism and inflammation (see review of Anthoni et al., 1991; Mathur and Kamal, 2012; Moreno et al., 2022). In the hard clam, Mercenaria mercenaria, increased trigonelline levels were found after animals were exposed to hypersaline stress and were discussed as an oxidative stress response (Zhou et al., 2023). Similarly, after acute warming (to 26°C) Götze et al. (2020) found a change in trigonelline levels in gills of P. maximus when additionally exposed to acute hypoxia and to the combination of hypoxia and hypercapnia. Liao et al. (2019) also reported increased levels of trigonelline under oxidative stress in OA-exposed scallop, Patinopecten yessoensis. However, whether the high trigonelline content is related to its putative role in oxidative stress remains speculative and requires further investigation. Interestingly, the tonic muscle shows similar concentrations in “gill-specific” metabolites such as glycolate and methionine, but also a very prominent serine concentration. Since the metabolite matrix of the tonic muscle is only poorly understood we can only assume that such metabolites in the tonic muscle have a function similar to that proposed for gills.
The metabolite spectrum of the mantle tissue is quite comparable to that of the gill tissue, exemplified by high concentrations of osmotically relevant metabolites, which indicate a role in osmo- and ion regulation as expected for a tissue in direct contact to seawater. However, the mantle spectra exhibited a lower quality due to a broader line width of the NMR signals. The generally poorer quality of 1H-NMR spectra from mantle tissue is a phenomenon also observed in other NMR studies on tissue extracts from marine bivalves (e.g. Lannig et al., 2010). This was explained by the functional heterogeneity of the mantle tissue, e.g. between inner and outer mantle (Clark, 2020). However, despite this heterogeneity, small n numbers and lower NMR signal quality, distinct differences in metabolite concentrations were observed to those of the other tissues. The concentrations of the amino acids threonine, glycine and valine were highest in the mantle tissue compared to the other tissues. Other amino acids such as alanine, leucine and isoleucine also showed high concentrations in the mantle tissue, indicating a particular importance of amino acids in the mantle tissue. The main functions of the mantle lie in biomineralization and calcification. A high proportion of free amino acids, provided for biomineralization is therefore not surprising. It has been shown only recently that amino acids like serine and threonine are of particular importance for biomineralization and calcification processes in bivalves such as scallops (Yarra et al., 2016, Yarra et al., 2021).
The spectra of the two muscle types also show a characteristic picture in their metabolite profiles. In particular, both are characterized by prominent concentrations of the amino acid L-arginine. L-arginine is the precursor for PLA, which corresponds to phosphagen in vertebrate muscle. PLA is used as the main rapid energy reserve to provide ATP and for the formation of octopine in the muscle during exercise and recovery (Grieshaber, 1978). It is therefore the essential metabolite for spontaneous locomotion in the muscle of scallops and indeed, it was shown to vary depending on the lifestyle of different scallop species (Tremblay et al., 2012; Tremblay and Guderley, 2014). In addition, fumarate and carnitine could be detected in both muscle types. These metabolites are also important for energy provision in muscle. Fumarate (fumaric acid) is an intermediate in the Krebs cycle for the production of ATP, but is also involved in the biosynthesis of L-arginine. Carnitine is the shuttle for fatty acids into mitochondria where they are subsequently used for energy production through beta-oxidation. The relatively high content of both metabolites may indicate i) a pronounced biosynthesis of L-arginine and ii) a high proportion of beta-oxidation in the adductor muscle. Interestingly, Guderley et al. demonstrated that neither palmitoyl carnitine nor aspartate were oxidized in isolated muscle mitochondria of the tropic scallop, Euvola ziczac (Guderley et al., 1995). Therefore, the question arises why such high carnitine levels were found in the adductor muscle of P. maximus if fatty acids are apparently not used for energy production in muscle mitochondria. Yet, the studies of Guderley et al. were done on a tropical species and the rate of substrate use in mitochondria may differ in tropical and boreal species. Temperature is indeed an important factor for the utilization of a specific substrate in mitochondria in all species (Barbe et al., 2023). In addition to L-arginine, fumarate and carnitine, choline, glucose, homarine and some other amino acids also showed comparable concentrations in the two muscle types. However, there were also some fundamental differences. For example, acetate and the amino acids alanine, glutamine and glutamate were highest in phasic muscle, whereas in tonic muscle they were at similar levels as in gills. In tonic muscle ATP levels were lowest, but succinate, lactate and O-phosphocholine levels were higher than those in other tissues. These differences can be explained by the specific function of each muscle type. The scallops’ tonic muscle, the so-called slow-catch “smooth muscle”, is mainly responsible for shell closure and prolonged contractures with little energy expenditure (Chantler, 2006; Sun et al., 2018). The phasic or glycolytic muscle is a fast twitching, striated muscle and mainly used during swimming. In line with a higher mitochondrial content in invertebrates’ tonic compared to phasic muscle (Nguyen et al., 1997) the observed lower ATP content and elevated succinate levels in tonic muscle appear conceivable. O-phosphocholine is an intermediate for phosphatidylcholines, the key building blocks for cellular membranes, and its observed high levels might indicate that the tonic muscle has a high membrane conversion rate due to its higher mitochondrial content. It is also conceivable that the higher mitochondrial content of tonic muscle needs a higher cell membrane flexibility, as suggested in the theory of membranes as metabolic pacemaker (Hulbert and Else, 1999). The membrane pacemaker theory (MPT) developed for mammals has also been tested in bivalves, although the general applicability to bivalves may differ by species or phenotype and is not always obvious (Pernet et al., 2006, 2007; Sukhotin et al., 2017). However, the differences between individual MPT studies on molluscs could also be due to tissue type, lifestyle or different environmental histories of the species studied (Guderley et al., 2009). Further experiments would be necessary to elucidate the role of MPT for both muscle tissues in bivalves.
Finally, the tonic muscle showed the lowest taurine, TMAO and trigonelline concentrations between tissues. In addition, low concentrations were observed also in other osmotically relevant metabolites such as homarine, glycine and glucose, which were comparable to the phasic muscle. This simply shows that involvement in systemic osmoregulation is only of secondary importance for the tonic and partly also for the phasic muscle. For the amino acids the tonic muscle exhibited highest concentrations of serine and tyrosine, but lowest in alanine, glycine, leucine and threonine concentrations in comparison to the other tissues. The question, however, why these two amino acids were elevated in the tonic muscle and the others were deficient cannot be answered here.
4.2 Tissue-specific OA, OW and OWA related metabolite changes
Metabolite profiles after long-term acclimation to ocean acidification (OA), ocean warming (OW) and the combination of ocean warming and acidification (OWA) are tissue-specific. In gills, ATP levels decreased in the two warming groups indicating increased ATP demand in the warmth. Indeed, Schalkhausser observed an increased oxygen consumption after long-term acclimation (Schalkhausser et al., 2014) which may be mirrored in the lowering of ATP levels. Compared to other tissues, gills are an aerobic-active and mitochondria-rich tissue with a nearly 2-fold higher metabolism compared to, for example, mantle tissue (Stapp et al., 2017). In line with increased gill respiration rates determined in the blue mussel after long-term exposure to intermediate and high OA scenarios, the increased gill energy demand can be explained by increased cilia movements involved in water and food transport (Stapp et al., 2017).
The phasic muscle showed only a few changes in metabolite concentrations under OA but more prominent changes in both warming groups, OW and OWA. Glycolate, lactate, O-phosphocholine and threonine were all decreased in the phasic muscle of the OA group. The amino acids alanine, glutamine, glutamate, arginine and leucine, as well as acetate and succinylacetone also displayed lower concentrations in the OW and OWA groups. The observed decrease in O-phosphocholine under OA can be explained by its specific function as an essential constituent for membranes and their turnover. An increased turnover of cell membranes as visible in concentration changes of e.g. phosphocholine and its derivatives (also proposed by Rebelein et al., 2018 and Götze et al., 2020) could be induced by warming. In addition, bivalves are osmoconformers and OA as well as warming, similar to salinity fluctuations, were shown to induce osmotic changes in bivalve tissues (e.g. Morabito et al., 2013 for OA; Jiang et al., 2020; Georgoulis et al., 2022 for warming). Since muscle tissues have a lower content of organic osmolytes than gills (see above), the observed changes of phosphocholine in phasic muscle might indicate a remodeling of the cell membrane to compensate for cell volume changes and ensure cellular homeostasis. This is also indicated by the network exploration of phasic muscle under OA, in which the cell membrane-specific metabolite phosphorylcholine is at the center of the network together with lactate (see Supplementary). At the same temperature, a decrease in lactate levels might indicate metabolic depression under OA. A connection between lactate and metabolic depression was already suggested by Pörtner et al. (2014) in 1994. A depressive effect by the OA induced reduction in extracellular pH has been intensively discussed for different bivalve species (e.g. Thomsen and Melzner, 2010; Lesser, 2016 for blue mussels; Lannig et al., 2010 for oyster; Liu and He, 2012 for mussels, oysters and scallops). On the one hand, Liu and He (2012) reported decreased MO2 levels under OA in the scallop Chlamys nobilis suggesting metabolic depression for scallops. On the other, one of our earlier organismal studies did not observe decreased MO2 levels in P. maximus under OA, which argues against metabolic depression under the selected OA conditions for this species (Schalkhausser et al., 2014). The degree of metabolic depression will clearly be influenced by warming. In addition, lactate may be used as an extra energy source via the pyruvate shuttle under routine metabolism under OA conditions, when PeO2 levels are reduced in the hemolymph (Schalkhausser et al., 2014). However, it must be noted that the prominent NMR signals of lactate and threonine are close to each other in the NMR spectrum. In our semi-targeted binning analysis approach, it cannot be ruled out that nearby NMR signals may influence each other due to overlap, resulting in a misleading profile. The similar pattern of the two metabolites between the four groups could indicate this, so the results for lactate and threonine should be interpreted with caution. Indeed, threonine changes are not as expected for a proteinogenic amino acid, where one would expect that it changes in parallel to other proteinogenic amino acids between treatments, which is not the case.
The changes in amino acid levels under OW and OWA can all be explained by the increasing energy demand due to the higher temperatures, as all changing amino acids are involved to a greater or lesser extent in energy metabolism. In the particular case of L-arginine, the decrease in phasic muscle can be explained by PLA reduction and octopine formation. As shown above PLA is the main energy source for the fast-twitching phasic muscle to refuel ATP during swimming and escape responses. In our previous study, using in vivo 31P-NMR spectroscopy we revealed a PLA decrease in resting phasic muscle of P. maximus following long term exposure to warming and OWA (Bock et al., 2019). In the present study, we obtained metabolic profiles via 1H-HRMAS NMR spectroscopy. As in the case of lactate and threonine, the 1H-NMR signals of L-arginine and PLA are very close to each other in the 1H-NMR spectrum, therefore it was not possible to distinguish between PLA and L-arginine. The decreased L-arginine content under OW and OWA conditions may reflect the warming-induced increase in energy demand and dephosphorylation of PLA to fuel ATP, if octopine formation absorbed L-arginine. Unfortunately, we have only rudimentary information on the possible 1H-NMR signals of octopine and have not found any data on octopine in the literature and databases. Therefore, an increase of octopine from L-arginine decomposition could not be verified in the NMR spectra from the phasic muscle of the OW and OWA groups. The decrease in acetate in the two warming groups could indicate that acetate is metabolized to fatty acids in order to obtain the required energy through beta-oxidation or to synthesize phospholipids (Zhukova, 1986) for the warming- induced rebuilding of cell membranes.
Finally, succinylacetone (SA) decreased in the phasic muscle under OW and OWA. This is a finding that to our knowledge has not been reported in the literature. SA is a metabolite in tyrosine metabolism and has signaling effects (Bechara et al., 2007). In vertebrates SA can inhibit the biosynthesis pathway of heme groups in mitochondria and it also can release iron in the liver and brain (Bechara et al., 2007). More importantly, SA can activate the biosynthesis of glutathione peroxidase and SOD (Rocha et al., 2000; Bechara et al., 2007). The production of reactive oxygen species is a well-known phenomenon in isolated mitochondria under heat stress, also in bivalves (e.g. Heise et al., 2003; Sokolova, 2023) and its importance was recently shown for the yesso scallop under OA (Liao et al., 2019). The observed increase of glycolate in phasic muscle of both warming groups would be in line with a strengthened antioxidative function of glycolate, compensating for the decrease in SA under these conditions. As described for gills the function of glycolate in marine animals is unclear and needs further investigations.
4.3 Link to organismal performance
The review of Guderley and Pörtner which focuses on scallops and fish, highlights the crucial role of energy availability under climate change for the aerobic power budget in marine ectotherms (Guderley and Pörtner, 2010). Energy availability depends on energy resources via food and/or stored energy reserves such as carbohydrates and lipids which are processed via aerobic and anaerobic metabolic pathways. Thereby, thermally induced hypoxemia which results from the mismatch between rising oxygen demand and increasingly limited supply via uptake (ventilatory capacity) and transport (cardiac circulatory capacity) is a main driver for temperature induced limitation in ectotherm performance (Pörtner, 2002, Pörtner and Lannig, 2009; Sokolova et al., 2012; Pörtner et al., 2017). Present observations in tissue-specific responses may indicate coordinated tissue changes balancing functional constraints for the sake of organism survival (Lannig et al., 2010; Tripp-Valdez et al., 2017; Götze et al., 2020).
In the following, we aim to interpret our previous results of P. maximus’ swimming performance and oxidative capacity under ocean warming and acidification (Schalkhausser et al., 2014; Bock et al., 2019) together with the present metabolomics findings in tissue samples. The increased energy demand in ocean warming treatments indicated by elevated resting metabolic rates of P. maximus at 20°C compared to 10°C (Schalkhausser et al., 2014) is accompanied by and correlates with the observed decrease of ATP levels in gill tissue (see above). The decreased levels of L-arginine in phasic muscle of OW- and OWA exposed scallops may have followed the lowered levels of PLA (Bock et al., 2019) including absorption of L-arginine in octopine formation (Grieshaber, 1978). In addition, we showed a mainly OW-induced decrease in the phasic muscle’s maximum oxidative flux of OW and OWA exposed P. maximus as well as the delayed replenishing of the phasic muscle’s PLA pool in the warmth (Bock et al., 2019). All of this can be linked to the warming induced strong reduction in haemolymph PeO2 levels of OW- and OWA-exposed P. maximus (Schalkhausser et al., 2014) indicating that the warming induced hypoxemia does not allow for maximal and fast recovery of PLA levels, since PLA replenishment occurs upon aerobic recovery (Grieshaber, 1978; Kamp, 1993; Sokolova et al., 2000). Most metabolite changes in the warming groups were observed in the phasic muscle, which were mainly energy related, as confirmed by the pathway analysis and network exploration. A change in energy demand via the TCA cycle was also reported for the adductor muscle of Argopecten irradians under thermal stress (Song et al., 2022). In line with our conclusion, the authors suggested that stored energy reserves were mobilized under thermal stress. In addition, Song et al. reported the degradation of valine, leucine and isoleucine for A. irradians under thermal stress (Song et al., 2022), similar to our observations. According to the pathway analysis and network exploration, L-arginine plays a central role in the metabolite network for energy provision under long term acclimation to warming in the adductor muscle, which is decoupled from the impact of OA. In terms of energy metabolism, this is not surprising, as L-arginine-phosphate is the basis for providing phosphate groups to ADP for building ATP. However, its direct connection to oxygen as determined from the network analysis also shows its dependence on oxygen and supports our hypothesis that PLA cannot be sufficiently rebuilt due to the reduced oxygen content in the hemolymph. This in turn leads to a reduced support for the increased ATP demand under OW. Interestingly, the network exploration for the warming groups also displayed a direct connection of L-arginine and ATP to methionine, sodium and CO2. Furthermore, both muscle types exhibited significantly higher concentrations of carnitine in comparison to gills and mantle (see Table 1).
We therefore propose the following model of metabolic connectivity between the two muscle types, in accordance with the work by Pérez et al. (2008), who demonstrated the recuperation of the phasic muscle by the tonic muscle during swimming in scallops. Compared to the phasic muscle, for which energy must be provided immediately during swimming, the tonic muscle uses energy rather slowly and for a prolonged period. To accomplish their different tasks the phasic muscle requires fast ATP supply under performance and essentially uses PLA for ATP generation. Only during prolonged exercise at low speeds does it switch to aerobic utilization of carbohydrates and fatty acids to supply ATP, as is generally the case for contracting muscles (Hargreaves and Spriet, 2020). The tonic muscle provides ATP for a prolonged period of performance and therefore preferably utilizes beta-oxidation to generate ATP. This is reasonable, as carbohydrate oxidation has a higher rate of ATP synthesis than fatty acid oxidation; the latter, however, can obtain more energy per molecule in the long term (overall ATP generation of 130 ATP vs 36 ATP per molecule; Hargreaves and Spriet, 2020). Fatty acids are used as the predominant substrate, for example during fasting and hypoglycemia, to provide energy via beta-oxidation in the muscle (Longo et al., 2016; Talley and Mohiuddin, 2023). It is therefore quite conceivable that P. maximus make increased use of beta-oxidation for energy supply in times of increased energy requirements such as under OW and exercise in the tonic muscle. This view is supported by the increase of carnitine in the phasic and tonic muscle in comparison to control (see Figure 4), albeit not significant, and the high concentration of methionine in the tonic muscle (Table 1). The higher carnitine concentration may allow a higher shuttle of fatty acids into tonic muscle mitochondria and thus an increased beta-oxidation (see above). The connection to sodium and methionine in the network of L-arginine might confirm this, since sodium is essential for the transport of carnitine across membranes (Talley and Mohiuddin, 2023) and methionine, from which carnitine can be synthesized endogenously (Longo et al., 2016). The link to CO2 might indicate the importance of beta-oxidation for energy provision in muscles under unfavorable conditions (Longo et al., 2016), such as OA, OW and the combination of both.
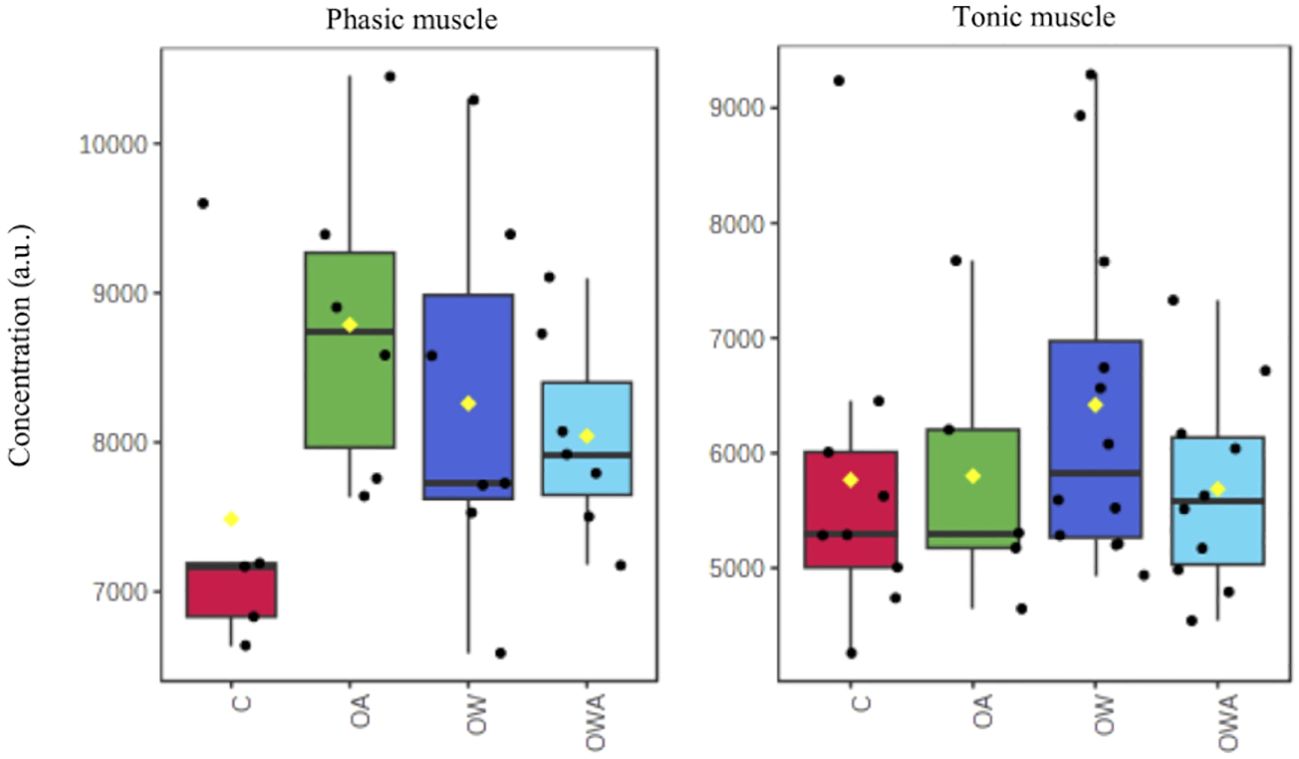
Figure 4 Changes in carnitine concentrations in phasic (left) and tonic muscle (right) tissue between treatments. Note, the differences are not significant.
The decreased levels of proteinogenic amino acids in both warming groups in the adductor muscle (Figure 2) might indicate a switch to the use of proteins for energy production as also suggested by (Götze et al., 2020), since carbohydrates are limited. An ongoing depletion of carbohydrates during prolonged exercise together with lowered oxygen levels in the haemolymph would lead to a decrease in power output (Hargreaves and Spriet, 2020). Indeed, a decreased clapping force was observed in the warming groups, while the number of claps remained the same compared to control (Schalkhausser et al., 2014). Since the carbohydrate reserves in the phasic muscle of the warming groups (OW & OWA), just like the PLA level (Bock et al., 2019), are already reduced due to the higher energetic costs in the warmth, there is an earlier reliance on the tonic muscle during swimming (Pérez et al., 2008). Beta-oxidation, with its higher capacity but lower power output, can thus maintain the number of claps, but at the expense of a reduced clapping force. In addition, beta-oxidation can provide the energy during recovery from exercise in mammals (Hargreaves and Spriet, 2020), but the processes and pathways that metabolize fatty acids to provide ATP are much slower than carbohydrate pathways. If one assumes that due to the lower PeO2 levels in the haemolymph, the energy is rather provided by beta-oxidation and octopine is formed from L-arginine (Grieshaber, 1978), it becomes understandable why recovery after fatigue was prolonged in the scallops of the warming groups (OW & OWA) compared to control. To summarize, if the tonic muscle compensates for the reduced performance of the phasic muscle and if beta-oxidation is favored for energy production under systemic hypoxemia during OW and OWA, the observed organismic limitations of the scallops from the previous studies can be explained. However, additional studies on the transport and flow of substrates fueling ATP production in the two muscle types will be needed to test this. Future experiments must show to what extent the energy is distributed in the body of the scallops under changing environmental conditions and whether additional energy requirements such as gonadal development will lead to a reduction in the energy budget and/or changes in energy distribution. This could have further consequences for the performance level of scallops from coastal and intertidal areas (Feidantsis et al., 2020).
5 Conclusions
The presented study of metabolite profiles of individual tissues showed distinct metabolite profiles, which are adjusted to the specific tasks of the organs. After long-term incubation under OA, OW and their combination OWA, metabolic profiles were largely different within the adductor muscle, between phasic and tonic muscles. These changes in metabolite concentrations mainly involved metabolic adjustments essential for the increased energy demand induced by warming and the associated hypoxemia. The proposed model for the interaction of the two muscle types of the adductor muscle can explain the observed performance limitations of scallops under OW and OWA. The tonic muscle apparently pursues a different strategy for energy provision than the phasic muscle, which for strong clapping essentially relies on PLA hydrolysis. This interplay is shifted to lower performance rates by organismic changes such as oxygen reduction in the hemolymph, especially under warming, and thus leads to the observed limitations in the swimming behavior of P. maximus.
Data availability statement
The datasets presented in this study can be found in online repositories. The names of the repository/repositories and accession number(s) can be found below: https://doi.pangaea.de/10.1594/PANGAEA.843533.
Ethics statement
Ethical approval was not required for the study involving animals in accordance with the local legislation and institutional requirements because an ethical approval by the German Council for Animal Care was not required for this study with bivalves.
Author contributions
CB: Conceptualization, Data curation, Formal analysis, Methodology, Validation, Visualization, Writing – original draft, Writing – review & editing. SG: Writing – review & editing. HP: Writing – review & editing. GL: Data curation, Validation, Visualization, Writing – review & editing.
Funding
The author(s) declare financial support was received for the research, authorship, and/or publication of this article. The project was supported by the research program “Changing Earth—Sustaining our Future” in the program-oriented funding periods (PoF IV subtopic 6.2—Adaptation of marine life: from genes to ecosystems) of the Helmholtz Association and is a contribution to the BMBF-funded project “Biological Impacts of Ocean Acidification” (BIOACID, FKZ 03F0608B) and the DFG funded project “TERSANE2”.
Acknowledgments
We appreciate the technical assistance of Burgel Schalkhausser, F. Véliz Moraleda, Annette Tillman, Felicitas Wermter and Rolf Wittig. Furthermore, we would like to thank the biological technical assistant trainees from the AWI: Lara Holst, Caroline Otten, Imke Lüdecke, Sean Segert and Anna Klitsch for assisting during sample processing.
Conflict of interest
The authors declare that the research was conducted in the absence of any commercial or financial relationships that could be construed as a potential conflict of interest.
Publisher’s note
All claims expressed in this article are solely those of the authors and do not necessarily represent those of their affiliated organizations, or those of the publisher, the editors and the reviewers. Any product that may be evaluated in this article, or claim that may be made by its manufacturer, is not guaranteed or endorsed by the publisher.
Supplementary material
The Supplementary Material for this article can be found online at: https://www.frontiersin.org/articles/10.3389/fevo.2024.1347160/full#supplementary-material
References
Anthoni U., Christophersen C., Hougaard L., Nielsen P. H. (1991). Quaternary ammonium compounds in the biosphere—An example of a versatile adaptive strategy. Comp. Biochem. Physiol. B. Comp. Biochem. 99, 1–18. doi: 10.1016/0305-0491(91)90002-U
Baag S., Mandal S. (2022). Combined effects of ocean warming and acidification on marine fish and shellfish: A molecule to ecosystem perspective. Sci. Total Environ. 802, 149807. doi: 10.1016/j.scitotenv.2021.149807
Barbe J., Roussel D., Voituron Y. (2023). Effect of physiological hyperthermia on mitochondrial fuel selection in skeletal muscle of birds and mammals. J. Therm. Biol. 117, 103719. doi: 10.1016/j.jtherbio.2023.103719
Bechara E. J. H., Dutra F., Cardoso V. E. S., Sartori A., Olympio K. P. K., Penatti C. A. A., et al. (2007). The dual face of endogenous alpha-aminoketones: pro-oxidizing metabolic weapons. Comp. Biochem. Physiol. C Toxicol. Pharmacol. 146, 88–110. doi: 10.1016/j.cbpc.2006.07.004
Bindoff N. L., Cheung W. W. L., Kairo J. G., Arístegui J., Guinder V. A., Hallberg R., et al. (2019). “Changing ocean, marine ecosystems, and dependent communities,” in IPCC Special Report on the Ocean and Cryosphere in a Changing Climate. Eds. Pörtner H.-O., Roberts D. C., Masson-Delmotte V., Zhai P., Tignor M., Poloczanska E., et al (Cambridge University Press, Cambridge, UK and New York, NY, USA), 447–587. doi: 10.1017/9781009157964.007
Bock C., Wermter F. C., Schalkhausser B., Blicher M. E., Pörtner H. O., Lannig G., et al. (2019). In vivo31P-MRS of muscle bioenergetics in marine invertebrates: Future ocean limits scallops’ performance. Magn. Reson. Imag. 61, 239–246. doi: 10.1016/j.mri.2019.06.003
Byrne M., Przeslawski R. (2013). Multistressor impacts of warming and acidification of the ocean on marine invertebrates’ life histories. Integr. Comp. Biol. 53, 582–596. doi: 10.1093/icb/ict049
Cappello T., Giannetto A., Parrino V., Maisano M., Oliva S., De Marco G., et al. (2018). Baseline levels of metabolites in different tissues of mussel Mytilus galloprovincialis (Bivalvia: Mytilidae). Comp. Biochem. Physiol. Part D Genomics Proteom. 26, 32–39. doi: 10.1016/j.cbd.2018.03.005
Chantler P. D. (2006). Scallop adductor muscles: structure and function. Dev. Aquac. Fish Sci. 35, 229–316. doi: 10.1016/S0167-9309(06)80031-1
Chong J., Wishart D. S., Xia J. (2019). Using MetaboAnalyst 4.0 for comprehensive and integrative metabolomics data analysis. Curr. Protoc. Bioinf. 68, e86. doi: 10.1002/cpbi.86
Clark M. S. (2020). Molecular mechanisms of biomineralization in marine invertebrates. J. Exp. Biol. 223, jeb206961. doi: 10.1242/jeb.206961
Diez V., Traikov S., Schmeisser K., Adhikari A. K. D., Kurzchalia T. V. (2021). Glycolate combats massive oxidative stress by restoring redox potential in Caenorhabditis elegans. Commun. Biol. 4, 151. doi: 10.1038/s42003-021-01669-2
Doney S. C., Ruckelshaus M., Duffy J. E., Barry J. P., Chan F., English C. A., et al. (2012). Climate change impacts on marine ecosystems. Ann. Rev. Mar. Sci. 4, 11–37. doi: 10.1146/annurev-marine-041911-111611
Feidantsis K., Giantsis I. A., Vratsistas A., Makri S., Pappa A. Z., Drosopoulou E., et al. (2020). Correlation between intermediary metabolism, Hsp gene expression and oxidative stress related proteins in long-term thermal stressed Mytilus galloprovincialis. Am. J. Physiol.: Regul. Integr. Comp. Physiol. 319, R264–R281. doi: 10.1152/ajpregu.00066.2020
Fiehn O. (2002). Metabolomics–the link between genotypes and phenotypes. Plant Mol. Biol. 48, 155–171. doi: 10.1023/A:1013713905833
Findlay H. S., Artoli Y., Birchenough S. N. R., Hartman S., León P., Stiasny M. (2022). Climate change impacts on ocean acidification relevant to the UK and Ireland. MCCIP Sci. Rev. 24. doi: 10.14465/2022.reu03.oac
Frizzo R., Bortoletto E., Riello T., Leanza L., Schievano E., Venier P., et al. (2021). NMR Metabolite Profiles of the Bivalve Mollusc Mytilus galloprovincialis Before and After Immune Stimulation With Vibrio splendidus. Front. Mol. Biosci. 8. doi: 10.3389/fmolb.2021.686770
Gattuso J. P., Magnan A. K., Bopp L., Cheung W. W. L., Duarte C. M., Hinkel J., et al. (2018). Ocean Solutions to Address Climate Change and Its Effects on Marine Ecosystems. Front. Mar. Sci. 5. doi: 10.3389/fmars.2018.00337
Gazeau F., Alliouane S., Bock C., Bramanti L., López Correa M., Gentile M., et al. (2014). Impact of ocean acidification and warming on the Mediterranean mussel (Mytilus galloprovincialis). Front. Mar. Sci. 1. doi: 10.3389/fmars.2014.00062
Georgoulis I., Bock C., Lannig G., Pörtner H. O., Feidantsis K., Giantsis I. A., et al. (2022). Metabolic remodeling caused by heat hardening in the Mediterranean mussel Mytilus galloprovincialis. J. Exp. Biol. 225, jeb244795. doi: 10.1242/jeb.244795
Götze S., Bock C., Eymann C., Lannig G., Steffen J. B. M., Pörtner H. O. (2020). Single and combined effects of the “Deadly trio” hypoxia, hypercapnia and warming on the cellular metabolism of the great scallop Pecten maximus. Comp. Biochem. Physiol. B Biochem. Mol. Biol. 243-244, 110438. doi: 10.1016/j.cbpb.2020.110438
Grieshaber M. (1978). Breakdown and formation of high-energy phosphates and octopine in the adductor muscle of the scallop, Chlamys opercularis (L.), during escape swimming and recovery. J. Comp. Physiol. B 126, 269–276. doi: 10.1007/BF00688937
Guderley H., Pörtner H. O. (2010). Metabolic power budgeting and adaptive strategies in zoology: examples from scallops and fish. Can. J. Zool. 88, 753–763. doi: 10.1139/Z10-039
Guderley H. E., Rojas F. M., Nusetti O. A. (1995). Metabolic specialization of mitochondria from scallop phasic muscles. Mar. Biol. 122, 409–416. doi: 10.1007/BF00350873
Guderley H. E., Tremblay I. (2016). “Swimming in scallops,” in Developments in Aquaculture and Fisheries Science, vol. Vol. 40. (Elsevier, Amsterdam), 535–566. doi: 10.1016/B978-0-444-62710-0.00012-2
Guderley H., Labbé-Giguere S., Janssoone X., Bourgeois M., Pérez H. M., Tremblay I. (2009). Thermal sensitivity of escape response performance by the scallop Placopecten magellanicus: Impact of environmental history. J. Exp. Mar. Biol. Ecol. 377, 113–119. doi: 10.1016/j.jembe.2009.07.024
Haider F., Sokolov E. P., Timm S., Hagemann M., Blanco Rayón E., Marigómez I., et al. (2019). Interactive effects of osmotic stress and burrowing activity on protein metabolism and muscle capacity in the soft shell clam Mya arenaria. Comp. Biochem. Physiol. A Mol. Integr. Physiol. 228, 81–93. doi: 10.1016/j.cbpa.2018.10.022
Hargreaves M., Spriet L. L. (2020). Skeletal muscle energy metabolism during exercise. Nat. Metabol. 2, 817–828. doi: 10.1038/s42255-020-0251-4
Harvey B. P., Gwynn-Jones D., Moore P. J. (2013). Meta-analysis reveals complex marine biological responses to the interactive effects of ocean acidification and warming. Ecol. Evol. 3, 1016–1030. doi: 10.1002/ece3.516
Heinemann A., Fietzke J., Melzner F., Böhm F., Thomsen J., Garbe-Schönberg D., et al. (2012). Conditions of Mytilus edulis extracellular body fluids and shell composition in a pH-treatment experiment: Acid-base status, trace elements and δ11B. Geochem. Geophys. Geosys. 13 (1). doi: 10.1029/2011GC003790
Heise K., Puntarulo S., Pörtner H. O., Abele D. (2003). Production of reactive oxygen species by isolated mitochondria of the Antarctic bivalve Laternula elliptica (King and Broderip) under heat stress. Comp. Biochem. Physiol. C 134, 79–90. doi: 10.1016/S1532-0456(02)00212-0
Hulbert A. J., Else P. L. (1999). Membranes as possible pacemakers of metabolism. J. Theor. Biol. 199, 257–274. doi: 10.1006/jtbi.1999.0955
Ivanina A. V., Sokolova I. M. (2016). Effects of intermittent hypoxia on oxidative stress and protein degradation in molluscan mitochondria. J. Exp. Biol. 219, 3794–3802. doi: 10.1242/jeb.146209
Jiang Y., Jiao H., Sun P., Yin F., Tang B. (2020). Metabolic response of Scapharca subcrenata to heat stress using GC/MS-based metabolomics. PeerJ 8, e8445. doi: 10.7717/peerj.8445
Kamp G. (1993). “Intracellular reactions controlling environmental anaerobiosis in the marine annelid Arenicola marina, a fresh look at old pathways,” in Surviving hypoxia: mechanisms of control and adaptation. Eds. Hochachka P. W., Lutz P. L., Sick T., Rosental M., van den Thillart G. (CRC, Boca Raton), pp 5 ± 17.
Landrigan P. J., Stegeman J. J., Fleming L. E., Allemand D., Anderson D. M., Backer L. C., et al. (2020). Human health and ocean pollution. Ann. Glob. Health 86, 151. doi: 10.5334/aogh.2831
Lannig G., Eilers S., Pörtner H. O., Sokolova I. M., Bock C. (2010). Impact of Ocean Acidification on Energy Metabolism of Oyster, Crassostrea gigas—Changes in Metabolic Pathways and Thermal Response. Mar. Drugs 8 (8), 2318–2339. doi: 10.3390/md8082318
Lesser M. (2016). Climate change stressors cause metabolic depression in the blue mussel, Mytilus edulis, from the Gulf of Maine. Limnol. Ocean. 61, 1705–1717. doi: 10.1002/lno.10326
Leung J. Y. S., Zhang S., Connell S. D. (2022). Is Ocean Acidification Really a Threat to Marine Calcifiers?A Systematic Review and Meta-Analysis of 980+ Studies Spanning Two Decades. Small 18 (35), 2107407. doi: 10.1002/smll.202107407
Liao H., Yang Z., Dou Z., Sun F., Kou S., Zhang Z., et al. (2019). Impact of ocean acidification on the energy metabolism and antioxidant responses of the Yesso scallop (Patinopecten yessoensis). Front. Physiol. 9, 1967. doi: 10.3389/fphys.2018.01967
Licker R., Ekwurzel B., Doney S. C., Cooley S. R., Lima I. D., Heede R., et al. (2019). Attributing ocean acidification to major carbon producers. Environ. Res. Lett. 14, 124060. doi: 10.1088/1748-9326/ab5abc
Liu W., He M. (2012). Effects of ocean acidification on the metabolic rates of three species of bivalve from southern coast of China. Chin. J. Ocean. Limnol. 30, 206–211. doi: 10.1007/s00343-012-1067-1
Longo N., Frigeni M., Pasquali M. (2016). Carnitine transport and fatty acid oxidation. Biochim. Biophys. Acta 1863, 2422–2435. doi: 10.1016/j.bbamcr.2016.01.023
Martínez Y., Li X., Liu G., Bin P., Yan W., Más D., et al. (2017). The role of methionine on metabolism, oxidative stress, and diseases. Amino Acids 49, 2091–2098. doi: 10.1007/s00726-017-2494-2
Mathur M., Kamal R. (2012). Studies on trigonelline from Moringa oleifera and its in vitro regulation by feeding precursor in cell cultures. Rev. Bras. Farmacogn. 22, 994–100. doi: 10.1590/S0102-695X2012005000041
Matoo O. B., Lannig G., Bock C., Sokolova I. M. (2021). Temperature but not ocean acidification affects energy metabolism and enzyme activities in the blue mussel, Mytilus edulis. Ecol. Evol. 00, 1–14. doi: 10.1002/ece3.7289
Melzner F., Mark F. C., Seibel B. A., Tomanek L. (2020). Ocean acidification and coastal marine invertebrates: tracking CO2 effects from seawater to the cell. Ann. Rev. Mar. Sci. 12, 499–523. doi: 10.1146/annurev-marine-010419-010658
Morabito R., Marino A., Lauf P. K., Adragna N. C., La Spada G. (2013). Sea water acidification affects osmotic swelling, regulatory volume decrease and discharge in nematocytes of the jellyfish Pelagia noctiluca. Cell Physiol. Biochem. 32, 77–85. doi: 10.1159/000356629
Moreno R. I., Zambelli V. O., Picolo G., Cury Y., Morandini A. C., Marques A. C., et al. (2022). Caspase-1 and Cathepsin B Inhibitors from Marine Invertebrates, Aiming at a Reduction in Neuroinflammation. Mar. Drugs 20, 614. doi: 10.3390/md20100614
Nguyen P. V., Marin L., Atwood H. L. (1997). Synaptic physiology and mitochondrial function in crayfish tonic and phasic motor neurons. J. Neurophysiol. 78, 281–294. doi: 10.1152/jn.1997.78.1.281
Pérez H. M., Janssoone X., Guderley H. (2008). Tonic contractions allow metabolic recuperation of the adductor muscle during escape responses of giant scallop Placopecten magellanicus. J. Exp. Mar. Biol. Ecol. 360, 78–84. doi: 10.1016/j.jembe.2008.04.006
Pernet F., Tremblay R., Comeau L., Guderley H. (2007). Temperature adaptation in two bivalve species from different thermal habitats: energetics and remodelling of membrane lipids. J. Exp. Biol. 210, 2999–3014. doi: 10.1242/jeb.006007
Pernet F., Tremblay R., Gionet C., Landry T. (2006). Lipid remodeling in wild and selectively bred hard clams at low temperatures in relation to genetic and physiological parameters. J. Exp. Biol. 209, 4663–4675. doi: 10.1242/jeb.02581
Pinsky M. L., Eikeset A. M., McCauley D. J., Payne J. L., Sunday J. M. (2019). Greater vulnerability to warming of marine versus terrestrial ectotherms. Nature 569, 108–111. doi: 10.1038/s41586-019-1132-4
Podbielski I., Bock C., Lenz M., Melzner F. (2016). Using the Critical Salinity (Scrit) Concept to Predict Invasion Potential of the Anemone Diadumene Lineata in the Baltic Sea. Mar. Biol. 163, 227. doi: 10.1007/s00227-016-2989-5
Pörtner H. O. (2002). Climate variations and the physiological basis of temperature dependent biogeography: systemic to molecular hierarchy of thermal tolerance in animals. Comp. Biochem. Physiol. A. Mol. Int. Physiol. 132, 739–761. doi: 10.1016/s1095-6433(02)00045-4
Pörtner H. O., Bock C., Mark F. C. (2017). Oxygen-and capacity-limited thermal tolerance: bridging ecology and physiology. J. Exp. Biol. 220, 2685–2696. doi: 10.1242/jeb.134585
Pörtner H.-O., Karl D., Boyd P., Cheung W., Lluch-Cota S. E., Nojiri Y., et al. (2014). “Ocean systems,” in Climate Change 2014: Impacts, Adaptation, and Vulnerability. Part A: Global and Sectoral Aspects. Contribution of Working Group II to the Fifth Assessment Report of the Intergovernmental Panel on Climate Change. Eds. Field C. B., Barros V. R., Dokken D. J., Mach K. J., Mastrandrea M. D., Bilir T. F., et al (Cambridge University Press, New York, NY), 411–484.
Pörtner H. O., Lannig G. (2009). “Oxygen and capacity limited thermal tolerance,” in Fish Physiology: Hypoxia, vol. Vol 27 . Eds. Richards J. G., Farrell A. P., Brauner C. J. (Burlington, MA: Academic Press, Elsevier Inc.), 143–191.
Poulin R. X., Lavoie S., Siegel K., Gaul D. A., Weissburg M. J., Kubanek J. (2018). Chemical encoding of risk perception and predator detection among estuarine invertebrates. Proc. Natl. Acad. Sci. U. S. A. 115, 662–667. doi: 10.1073/pnas.1713901115
Rebelein A., Pörtner H. O., Bock C. (2018). Untargeted metabolic profiling reveals distinct patterns of thermal sensitivity in two related notothenioids. Comp. Biochem. Physiol. A 217, 43–54. doi: 10.1016/j.cbpa.2017.12.012
Richards R. G., Davidson A. T., Meynecke J. O., Beattie K., Hernaman V., Lynam T., et al. (2015). Effects and mitigations of ocean acidification on wild and aquaculture scallop and prawn fisheries in Queensland. Aus. Fish. Res. 161, 42–56. doi: 10.1016/j.fishres.2014.06.013
Rocha M. E., Bandy B., Costa C. A., de Barros M. P., Pinto A. M., Bechara E. J. (2000). Iron mobilization by succinylacetone methyl ester in rats. A model study for hereditary tyrosinemia and porphyrias characterized by 5-aminolevulinic acid overload. Free Radic. Res. 32, 343–353. doi: 10.1080/10715760000300341
Schalkhausser B., Bock C., Pörtner H.-O., Lannig G. (2014). Escape performance of temperate king scallop, Pecten maximus under ocean warming and acidification. Mar. Biol. 161, 2819–2829. doi: 10.1007/s00227-014-2548-x
Schalkhausser B., Bock C., Stemmer K., Brey T., Pörtner H.-O., Lannig G. (2013). Impact of ocean acidification on escape performance of the king scallop, Pecten maximus, from Norway. Mar. Biol. 160, 1995–2006. doi: 10.1007/s00227-012-2057-8
Schmidt M., Windisch H. S., Ludwichowski K. U., Seegert S. L. L., Pörtner H. O., Storch D., et al. (2017). Differences in neurochemical profiles of two gadid species under ocean warming and acidification. Front. Zool. 14. doi: 10.1186/s12983-017-0238-5
Sokolova I. M. (2023). Ectotherm mitochondrial economy and responses to global warming. Acta Physiol. (Oxf). 237, e13950. doi: 10.1111/apha.13950
Sokolova I. M., Bock C., Pörtner H. O. (2000). Resistance to freshwater exposure in White Sea Littorina spp. I: Anaerobic metabolism and energetics. J. Comp. Physiol. B. 170, 91–103. doi: 10.1007/s003600050264
Sokolova I. M., Frederich M., Bagwe R., Lannig G., Sukhotin A. A. (2012). Energy homeostasis as an integrative tool for assessing limits of environmental stress tolerance in aquatic invertebrates. Mar. Environ. Res. 79, 1–15. doi: 10.1016/j.marenvres.2012.04.003
Song J., Liu B., Wang C. (2022). Metabolomic and Transcriptomic Responses of Argopecten irradians concentricus to Thermal Stresses. Front. Mar. Sci. 9. doi: 10.3389/fmars.2022.818083
Stapp L., Parker L., O'Connor W. A., Bock C., Ross P. M., Pörtner H. O., et al. (2018). Sensitivity to ocean acidification differs between populations of the Sydney rock oyster: role of filtration and ion-regulatory capacities. Mar. Environ. Res. 135, 103–113. doi: 10.1016/j.marenvres.2017.12.017
Stapp L., Thomsen J., Schade H., Bock C., Melzner F., et al. (2017). Intra-population variability of ocean acidification impacts on the physiology of Baltic blue mussels (Mytilus edulis): integrating tissue and organism response. J. Comp. Physiol. B Biochem. Syst. Environ. Physiol. 187, 529–543. doi: 10.1007/s00360-016-1053-6
Sukhotin A., Fokina N., Ruokolainen T., Bock C., Pörtner H. O., Lannig G. (2017). Does the membrane pacemaker theory of metabolism explain the size dependence of metabolic rate in marine mussels? J. Exp. Biol. 220, 1423–1434. doi: 10.1242/jeb.147108
Sun X., Liu Z., Wu B., Zhou L., Wang Q., Wu W., et al. (2018). Differences between fast and slow muscles in scallops revealed through proteomics and transcriptomics. BMC Genomics 19, 377. doi: 10.1186/s12864-018-4770-2
Talley J. T., Mohiuddin S. S. (2023). “Biochemistry, fatty acid oxidation,” in StatPearls (StatPearls Publishing, Treasure Island (FL). Available at: https://www.ncbi.nlm.nih.gov/books/NBK556002/.
Thomsen J., Melzner F. (2010). Moderate seawater acidification does not elicit long-term metabolic depression in the blue mussel Mytilus edulis. Mar. Biol. 157, 2667–2676. doi: 10.1007/s00227-010-1527-0
Tripp-Valdez M. A., Bock C., Lucassen M., Lluch-Cota S. E., Sicard M. T., Lannig G., et al. (2017). Metabolic response and thermal tolerance of green abalone ju- veniles (Haliotis fulgens: Gastropoda) under acute hypoxia and hypercapnia. J. Exp. Mar. Biol. Ecol. 497, 11–18. doi: 10.1016/j.jembe.2017.09.002
Tremblay I., Guderley H. E. (2014). Scallops show that muscle metabolic capacities reflect locomotor style and morphology. Physiol. Biochem. Zool. 87, 231–244. doi: 10.1086/674107
Tremblay I., Guderley H. E., Himmelman J. H. (2012). Swimming away or clamming up: the use of phasic and tonic adductor muscles during escape responses varies with shell morphology in scallops. J. Exp. Biol. 215, 4131–4143. doi: 10.1242/jeb.075986
Viant M. R. (2008). Environmental metabolomics using 1H -NMR spectroscopy. Methods Mol. Biol. 410, 137–150. doi: 10.1007/978-1-59745-548-0_9
Wishart D. S. (2019). Metabolomics for investigating physiological and pathophysiological processes. Physiol. Rev. 99, 1819–1875. doi: 10.1152/physrev.00035.2018
Wishart D. S., Cheng L. L., Copié V., Edison A. S., Eghbalnia H. R., Hoch J. C., et al. (2022). NMR and metabolomics—A roadmap for the future. Metabolites 12, 678. doi: 10.3390/metabo12080678
Yarra T., Gharbi K., Blaxter M., Peck L. S., Clark M. S. (2016). Characterization of the mantle transcriptome in bivalves: Pecten maximus, Mytilus edulis and Crassostrea gigas. Mar. Genomics 27, 9–15. doi: 10.1016/j.margen.2016.04.003
Yarra T., Ramesh K., Blaxter M., Hüning A., Melzner F., Clark M. S. (2021). Transcriptomic analysis of shell repair and biomineralization in the blue mussel, Mytilus edulis. BMC Genomics 22, 437. doi: 10.1186/s12864-021-07751-7
Zhao X., Guo C., Han Y., Che Z., Wang Y., Wang X., et al. (2017). Ocean acidification decreases mussel byssal attachment strength and induces molecular byssal responses. Mar. Ecol. Prog. Ser. 565, 67–77. doi: 10.3354/meps11992
Zhao L., Shirai K., Tanaka K., Milano S., Higuchi T., Murakami-Sugihara N., et al. (2020). A review of transgenerational effects of ocean acidification on marine bivalves and their implications for sclerochronology. Estuar. Coast. Shelf. Sci. 235, 106620. doi: 10.1016/j.ecss.2020.106620
Zeebe R. E. (2012). History of seawater carbonate chemistry, atmospheric CO2, and ocean acidification. Annu. Rev. Earth Planet. Sci. 40, 141–165. doi: 10.1146/annurev-earth-042711-105521
Zhou C., Xu L., Song H., Feng J., Hu Z., Yang M., et al. (2023). Examination of the regulation of energy metabolism, antioxidant response, and ammonia detoxification in hard clam, Mercenaria mercenaria, under hypersalinity stress. Aquaculture 563, 738916. doi: 10.1016/j.aquaculture.2022.738916
Keywords: bivalves, climate change, NMR spectroscopy, metabolite profiling, pathway and network analysis
Citation: Bock C, Götze S, Pörtner HO and Lannig G (2024) Exploring the mechanisms behind swimming performance limits to ocean warming and acidification in the Atlantic king scallop, Pecten maximus. Front. Ecol. Evol. 12:1347160. doi: 10.3389/fevo.2024.1347160
Received: 30 November 2023; Accepted: 21 March 2024;
Published: 09 April 2024.
Edited by:
Piero Calosi, Université du Québec à Rimouski, CanadaReviewed by:
Athanasios Exadactylos, University of Thessaly, GreeceLouis V. Plough, University of Maryland, United States
Copyright © 2024 Bock, Götze, Pörtner and Lannig. This is an open-access article distributed under the terms of the Creative Commons Attribution License (CC BY). The use, distribution or reproduction in other forums is permitted, provided the original author(s) and the copyright owner(s) are credited and that the original publication in this journal is cited, in accordance with accepted academic practice. No use, distribution or reproduction is permitted which does not comply with these terms.
*Correspondence: Christian Bock, Q2hyaXN0aWFuLkJvY2tAYXdpLmRl