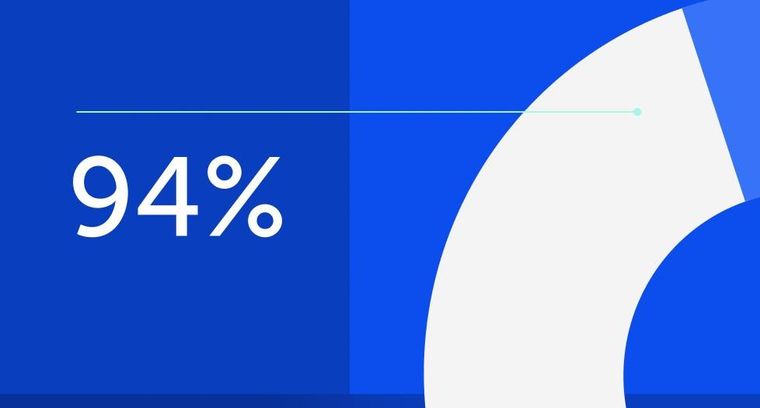
94% of researchers rate our articles as excellent or good
Learn more about the work of our research integrity team to safeguard the quality of each article we publish.
Find out more
MINI REVIEW article
Front. Ecol. Evol., 01 February 2024
Sec. Evolutionary Developmental Biology
Volume 12 - 2024 | https://doi.org/10.3389/fevo.2024.1345733
This article is part of the Research TopicEmergence of Dynamic Membranes – Role of Terpenoids in the Evolution of Membrane Organization and RegulationView all 5 articles
Cellular membranes define the physical boundary of life and provide scaffolds for various fundamental metabolic activities, including ATP synthesis, respiration, phototrophy, endocytosis and ion transport. Terpenoids, also known as isoprenoids, are known to play important roles in membrane organization and regulation across the three domains of life through unique interactions with other membrane lipids and membrane proteins. Terpenoids are present in not only the membranes of the three domains, but also viral membranes and extracellular vesicles. The large structural diversity of terpenoids and their ubiquitous distribution in modern organisms make terpenoids distinct from other membrane lipids, such as fatty acyls that are nearly absent in archaea. Addressing the biochemical and biophysical properties that allow terpenoids to play critical roles in membrane organization is important to understand the driving forces that shaped cellular life as we know it. This review summarizes the major classes of terpenoids that are involved in membrane organization and discuss the impact of terpenoid-membrane interactions on the evolutionary trajectory of membrane dynamics and the fitness of host organisms.
Cellular membrane was among critical factors for the emergence of life. Compartmentalization of small molecules in a cellular structure would have been a prerequisite for the onset of pre-biotic chemistry and subsequent biochemistry during the formative period of life (Deamer et al., 2002). In modern biology, cellular membranes are mixtures of a variety of lipid molecules that have a distinct evolutionary origin and history. Among those lipids, terpenoids, also known as isoprenoids, are known for their large structural and functional diversity (Bloch, 1991; Hartmann, 1998; Xu et al., 2004). Terpenoids are present in the cellular membrane of all three domains of life – Archaea, Bacteria and Eukarya – but are utilized distinctly from one another. Archaea use terpenoids as structural components of their membranes (Jain et al., 2014), while bacteria and eukaryotes use terpenoids as membrane regulators (Sezgin et al., 2017; Belin et al., 2018). The establishment of the archaeal and eukaryotic domains is in fact built upon the presence of certain terpenoids. Hence, the structural and functional divergence of terpenoids was an important driving force for some major evolutionary events of life. This review focuses on the role of terpenoids in the diversification of membrane organization and associated dynamics in cellular life, thereby providing an integrated perspective for the co-evolutionary relationship between terpenoids and biological membranes.
Biological membranes encompass not only cellular membranes, but also ‘non-cellular’ membranes, including endomembranes (intracellular membranes), extracellular vesicles and viral envelopes. These non-cellular membranes are derived from host cells, but not necessarily directly from cellular membranes. Hence, the actual composition may be divergent, depending on individual membranes. In principle, there are only two cellular membrane systems in biology: archaeal-type and bacterial-type membranes (Koga, 2011) (Figure 1). The archaeal membrane lipids have sn-glycerol-1-phosphate (G1P) as the lipid backbone that is linked to linear terpenoid chains via ether bonds, while the bacterial lipids have sn-glycerol-3-phosphate (G3P) that is linked to fatty acyl chains via ester bonds. This structural difference is known as the ‘lipid divide’ and represents one of fundamental differences between Archaea and Bacteria, despite that both domains evolved from LUCA (Koga, 2011; Jain et al., 2014). Eukarya has a bacterial-type membrane, even though the domain is inferred to have evolved from within Archaea (Eme et al., 2017). Some bacteria are known to possess genes to biosynthesize archaeal-type membrane lipids, but the in vivo production of those archaeal-type lipids is yet to be confirmed (Villanueva et al., 2021).
Figure 1 Membrane tree of life. The bifurcation of the archaeal and bacterial membranes represents the ‘lipid divide’. The evolutionary timing of two regulatory terpenoids (steroids and hopanoids) is also shown. The eukaryotic membrane is a variation of the bacterial membrane, which is different from the species relationship of eukaryotes and bacteria. G1P and G3P indicate the stereochemistry of the glycerol backbone. *1: some bacteria likely have sphingolipids, but the bacterial biosynthesis pathway seems to be distinct from the eukaryotic pathway (Stankeviciute et al., 2022). *2: hopanoids are not universal in the bacterial domain. *3: LUCA membrane composition remains hypothetical (Koga, 2011). P in a circle indicates the polar head group. G1P, sn-glycerol-1-phosphate; G3P, sn-glycerol-3-phosphate.
Terpenoids are present in all types of biological membranes and also in all forms of cellular life on Earth (Figure 1). The involvement of terpenoids in membrane organization seems to have a deep root and the possible presence of terpenoids in the hypothetical membrane of the last universal common ancestor (LUCA) has been debated (Coleman et al., 2019; Villanueva et al., 2021; Hoshino and Villanueva, 2023). Terpenoid biosynthesis starts from the oligomerization of C5 isoprene units, but subsequent modifications, including cyclization and prenylation, produce the large structural diversity of terpenoids (Figure 2).
Figure 2 Biosynthesis flow and taxonomic distribution of terpenoids that are present in biological membranes. Carbon numbers at the upper left or right corner of grey boxes indicate the typical molecular sizes of produced terpenoids or terpenoid moieties of larger molecules (exceptions exist). Excluding downstream modifications, terpenoids always have a carbon number of multiples of five, due to the isoprene units. Arrows do not necessarily indicate a single enzymatic step. Terpenoids in the dashed boxes are intermediates, while those in the solid grey boxes are final products (only examples are shown). *1: polyprenoids include any functionalized forms, but the abundance of polyprenoids as final products is not well understood. The dephosphorylation step is similarly not well understood. *2: In vitro production of archaeal lipids by bacterial enzymes is not included in the figure (Villanueva et al., 2021). Also, the carbon numbers at the upper right corner correspond to the terpenoid single chains. DMAPP, dimethylallyl diphosphate; IPP, isopentenyl diphosphate; PP, diphosphate.
Terpenoids are directly or indirectly involved in membrane organization. The ability of terpenoids to modulate membrane properties is based on their hydrophobic structure. C<25 linear terpenoids either constitute membrane lipids (e.g. archaeol) (Jain et al., 2014), or are used as membrane anchors for certain biomolecules such as chlorophylls, hemes and proteins (Hederstedt, 2012; Jiang et al., 2018; Proctor et al., 2022). Other C<25 small terpenoids, such as 1,8-cineole and cedrol, may function as transient membrane modulators in bacteria and eukaryotes, through permeating membranes due to their size and hydrophobic nature (Pham et al., 2015) (Figure 2). In contrast, C>25 polycyclic terpenoids – most notably hopanoids and steroids – are permanent membrane regulators in bacteria and eukaryotes (Sezgin et al., 2017; Belin et al., 2018). These terpenoids are produced by the so-called class II terpene cyclase (TC) family (Christianson, 2017). In turn, carotenoids, quinones and polyprenoids primarily engage in photosynthesis, respiration and cell envelope biogenesis, respectively (Jones et al., 2009; Hashimoto et al., 2016; Franza and Gaudu, 2022), but can also alter the property of surrounding membrane environments. While carotenoids are mainly found in phototrophic bacteria and eukaryotes, quinones and polyprenoids (particularly polyprenyl diphosphates) are universally distributed in the three domains.
Bacterial and eukaryotic membranes contain terpenoids mainly as regulatory components. While hopanoids and steroids are the two major membrane terpenoids, several specific lineages, including anaerobic protists and ciliates, alternatively produce a unique structural homolog called tetrahymanol (Takishita et al., 2012; Banta et al., 2015). In some species, the abundance of regulatory terpenoids is comparable to that of fatty acyl phospholipids (van Meer et al., 2008; Belin et al., 2018).
Steroids in eukaryotes are the most extensively studied terpenoids for their roles in membrane organization. Eukaryotic-specific membrane dynamics is based on the triad of distinct lipid components – fatty acyl phospholipids, sphingolipids and steroids (van Meer et al., 2008; Sezgin et al., 2017). The planar polycyclic structure of steroids, particularly cholesterol, has an ability to decrease the fluidity and the permeability of host membranes and laterally compress membranes, forming a liquid-ordered microdomains (Cheng and Smith, 2019). Further, it has increasingly been suggested that eukaryotic membranes are kept near the critical state (Shaw et al., 2020), which enables eukaryotic cells to respond to external stimuli in a highly dynamic way (Hammond et al., 2005; Levental et al., 2010). Individual steroids have varying effects, due to their subtle structural differences. For instance, C-24 alkylated steroids have elevated interactions with other lipid components, relative to cholesterol, enabling host membranes to have a wider resilience against temperature fluctuations and thus providing a selective advantage for host algae and plants (Beck et al., 2007). Hopanoids have a similar membrane packing ability (Sáenz et al., 2012; Sáenz et al., 2015), but to a lesser degree than cholesterol, because of the presence of additional methyl groups in the rings.
Bacterial membranes have also been suggested to have a eukaryotic-like heterogeneous membranes (functional membrane microdomains; FMMs) (Bramkamp and Lopez, 2015; Lopez and Koch, 2017), although the critical status of FMMs is currently unclear. It is suggested that FMMs are induced by terpenoids, including hopanoids, carotenoids and farnesol (Feng et al., 2014; Bramkamp and Lopez, 2015; García-Fernández et al., 2017; Cheng and Smith, 2019). Also, sphingolipids are distributed in some bacteria (Stankeviciute et al., 2022) and hence those bacteria might have eukaryotic-like membrane regulations based on the combination of sphingolipids and hopanoids. The presence of membrane heterogeneity has also been suggested in the archaeal membrane that is entirely composed of linear terpenoids, but its dynamics remains underexplored (Bagatolli et al., 2000; Salvador-Castell et al., 2020; Tourte et al., 2020).
Other terpenoids may also engage in membrane organization and protein-lipid interactions locally and/or temporarily. Quinones, polyprenoids and C<25 small terpenoids can alter the membrane fluidity and/or permeability, although their physiological significance is not fully understood (Hartley and Imperiali, 2012; Camargos et al., 2014; Sévin and Sauer, 2014; Pham et al., 2015; Mishra et al., 2021). Also, C15 or C20 prenylation of eukaryotic Ras proteins not only anchors the proteins in membranes, but also mediates protein-protein and protein-lipid interactions (Sinensky, 2000).
Endomembrane systems are observed in all three domains of life and often have divergent lipid compositions from outer cellular membranes. In archaea, the hyperthermophilic anaerobe Ignicoccus hospitalis contains two cytoplasmic regions enclosed by outer and inner membranes. These two membranes have different terpenoid profiles and are utilized for different metabolic activities (Flechsler et al., 2021; van Wolferen et al., 2022). The presence of such a double membrane is suggested for several additional archaea, but the overall distribution remains punctate in the domain (Klingl, 2014). In contrast, bacteria are well known to have several unique endomembrane systems, including thylakoids and chromatophores in photosynthetic bacteria (Jürgens et al., 1992; Simonin et al., 1996; Orf and Blankenship, 2013; Mullineaux and Liu, 2020), anammoxosomes in anammox bacteria (van Niftrik et al., 2004) and magnetosomes in magnetotactic bacteria (Barber-Zucker and Zarivach, 2017). Also, eukaryotes are well known to universally possess a variety of membrane-bound organelles, including mitochondria, endoplasmic reticulum, Golgi apparatus and plastids.
However, terpenoids are not necessarily present in those endomembranes, even if terpenoids are present in cellular membranes. This heterogeneity presumably reflects the physiological requirements of individual endomembranes and/or their evolutionary origins. For instance, hopanoids are found in thylakoids in cyanobacteria, but are mostly absent in other bacterial endomembranes (Rattray et al., 2008; Schüler, 2008). Also, steroids are only minor components in mitochondria and endoplasmic reticulum in animals and fungi (van Meer et al., 2008). Mitochondria also lack sphingolipids and this might reflect the membrane composition of ancestral symbiotic alphaproteobacteria. Steroids are similarly absent in eukaryotic thylakoids, possibly reflecting their cyanobacterial origin (Hölzl and Dörmann, 2019).
Photosynthetic endomembranes (thylakoids and chromatophores) contain carotenoids either as part of the photosynthetic machinery, or as non-bound components. Carotenoids span both leaflets of a bilayer and have an ability to vertically compress membranes (Cheng and Smith, 2019). The fluidity of thylakoid membranes is in fact controlled by carotenoids, rather than hopanoids. The imbalance between carotenoids, other lipids and proteins, hampers the proper conformation of thylakoid structures (Bykowski et al., 2021). Carotenoids are also directly involved in photosystem assembly in cyanobacteria (Tóth et al., 2015). Hence, thylakoid membranes seem to be regulated differently from other (endo)membranes of non-photosynthetic organisms.
The presence of lipid membranes is not limited to cellular organisms. Many viruses have lipid membranes either as outer envelopes or as capsid-enclosed inner membranes. Membrane-containing viruses are unevenly distributed among eukaryotic, bacterial and archaeal viruses and viral membranes likely do not share a common ancestry (Poranen et al., 2015; Mäntynen et al., 2019). Viral membranes are thought to have evolved to overcome the surface barriers of host organisms (Poranen et al., 2015; Omasta and Tomaskova, 2022) and/or cope with environmental stress (Baquero et al., 2021). The presence of terpenoids in membranes is known for archaeal and eukaryotic viruses thus far. Archaeal viruses have archaeal-type membrane terpenoids, while eukaryotic viruses have steroids. Viral membranes are commonly acquired by budding of host cellular membranes and this causes a similar lipid composition of viral membranes to that of host membranes (Waheed and Freed, 2010). However, viral membrane formation within host cells is also known (Baquero et al., 2021). In this case, the lipid composition of the viral membrane is highly divergent from that of the host membrane. For instance, positive-strand RNA viruses manipulate the host lipid biosynthesis to optimize the lipid microenvironment for the viral replication (Nagy, 2022). It is currently unknown if viral membrane terpenoids are directly involved in viral entry and replication processes. In contrast, some structural homologs of steroids (e.g. oleanoic acid and betulinic acid) in host membranes are known to interact with viral membrane proteins as antiviral agents and prevent viral-host membrane fusion (Si et al., 2018).
Various forms of membrane-bound extracellular vesicles (EVs) are secreted by cellular organisms, utilizing host membrane lipids (Deatherage and Cookson, 2012; Gill et al., 2019). EVs include plasmid vesicles to virus-like particles and eukaryotic exosomes. EVs facilitate the transfer of genetic materials and other metabolites between cells. EVs share some similarity with enveloped viruses and in fact a shared origin for certain types of archaeal EVs and viruses has been suggested (Nolte-’t Hoen et al., 2016). Further, infectious virus-like plasmids that are surrounded by membrane vesicles were recently discovered from haloarchaea (Erdmann et al., 2017). Terpenoids are present in EVs from all three domains, reflecting host cellular membrane compositions (Berry et al., 1993; Skotland et al., 2020; Liu et al., 2021). However, the role of terpenoids in EVs remains unexplored.
Resting cells differentiate from vegetative cells to preserve genetic materials and other important metabolites under adverse environmental conditions. Resting cells are observed in multiple lineages of bacteria, including endospores in the phylum Bacillota, exospores in actinobacteria and akinetes in cyanobacteria. Endospores in Bacillota are coated with lipid membranes that contain a unique terpenoid called baciterpenol A that is a structural homolog of hopanoids and steroids (Sato, 2013; Willdigg and Helmann, 2021) (Figure 2). In fact, baciterpenol A is produced by class II TC in aerobic members of the phylum. Baciterpenol A increases the rigidity of the host membrane and thus the resistance against oxidative stress (Bosak et al., 2008). In contrast, polycyclic terpenoids have not been found in exospores, even though they are widespread in hopanoid-producing actinobacteria (e.g. Streptomyces). In turn, hopanoids are widespread in akinetes and even a correlation between a certain type of hopanoids and akinete formation is suggested (Doughty et al., 2009). Exospore formation was recently reported even for haloarchaea (Tang et al., 2023). Haloarchaea are unique because they horizontally acquired a large number of bacterial genes (Gophna and Altman-Price, 2022), including those for carotenoids and even fatty acids that are otherwise bacterial signatures (Dibrova et al., 2014). Hence, the membrane dynamics of haloarchaea may be divergent from that of other archaea.
The diverse chemical structures of terpenoids enable them to involve in membrane organization in various different ways. In fact, the structural diversification of terpenoids is linked to the evolutionary trajectory of biological membranes. A prime example is the evolutionary history of class II TC that produces hopanoids and steroids (Hoshino and Villanueva, 2023). The emergence and the stepwise evolution of class II TC seem to reflect the molecular adaptation of host cellular membranes towards changes in Earth’s environment. The distribution of class II TC is confined to bacteria and eukaryotes, hence to the bacterial-type membrane. Its ultimate origin is likely in bacteria, producing hopanoids (Santana-Molina et al., 2020). Class II TC acts on a special type of terpenoid oligomers that are formed via the so-called head-to-head condensation of isoprene units (Figure 2). This condensation reaction produces highly hydrophobic squalene and phytoene that are embedded in membranes. The influence of these terpenoids on membrane property is well documented (Hauß et al., 2002; LoRicco et al., 2023). Thus, these precursor terpenoids possibly evolved already as membrane regulators before the evolution of class II TC, while the cyclase contributed to the structural diversification of terpenoid regulators.
The modern diversity of class II TC products reflect their adaptation towards the oxygenated Earth. While hopanoids are formed by direct cyclization of squalene, steroids are formed by cyclization of ‘oxygenated’ squalene (oxidosqualene). Accordingly, steroid biosynthesis is performed only by aerobic organisms. Hence, the membrane dynamics of steroid-producing organisms – most notably eukaryotes – owes its emergence to the aerobic adaptation of terpenoids, which possibly occurred shortly after the Great Oxidation Event 2.4 billion years ago (Gold et al., 2017). Further, modern steroids undergo substantial modifications from original cyclization products (protosteroids) (Figure 2). These modifications enhance the membrane modulation ability of steroids and may reflect the evolutionary trajectory of eukaryotes themselves (Hoshino and Gaucher, 2021; Brocks et al., 2023). However, the onset of terpenoid-associated membrane dynamics likely predates the emergence of class II TC and the head-to-head condensation, as one of the simplest and oldest terpenoids, farnesol, already has the ability to induce microdomain formation in the bacterial membrane (Feng et al., 2014).
In contrast, the effects of terpenoids on archaeal-type membranes is largely unknown. In fact, regulatory terpenoids, such as hopanoids, steroids, carotenoids and squalene, are nearly absent in archaea, even though the archaeal membrane itself is made up of terpenoids (Figure 1). Quinones and polyprenoids that are universally distributed in all three domains may function as membrane regulators (Salvador-Castell et al., 2019), but the underlying molecular mechanisms that drive the membrane dynamics in archaea are generally not well understood. Hence, systematic and comprehensive studies about the impact of individual terpenoids on different membrane systems would be critical to elucidate the origin and the evolutionary history of life through the perspective of membrane evolution (King and Wang, 2023). Terpenoids were involved in the evolution of at least two domains – Archaea and Eukarya. The evolution of Archaea from LUCA entailed a fundamental shift in the lipid composition of the cellular membrane: either a terpenoid membrane was newly acquired, or alternatively a hypothetical mixed membrane converged to a terpenoid membrane (Koga, 2011) (Figure 1). Similarly, the evolution of Eukarya from Archaea was achieved by the transformation from a terpenoid membrane to a fatty acyl membrane, followed by the acquisition of a different class of terpenoids – i.e. steroids (Eme et al., 2017; Hoshino and Gaucher, 2021). In parallel, viruses also adapted to these membrane transformations in host cellular organisms. The interaction of viruses with host membranes is a critical factor for viruses’ entry and propagation strategy (Omasta and Tomaskova, 2022). Hence, understanding the evolutionary relationship between host membranes and associated viruses may shed additional light on the driving force behind the evolution of both cellular organisms and viruses.
YH: Conceptualization, Investigation, Writing – original draft, Writing – review & editing.
The author(s) declare financial support was received for the research, authorship, and/or publication of this article. This work was supported by DFG Priority programme 2237 and the Helmholtz Society.
The author declares that the research was conducted in the absence of any commercial or financial relationships that could be construed as a potential conflict of interest.
All claims expressed in this article are solely those of the authors and do not necessarily represent those of their affiliated organizations, or those of the publisher, the editors and the reviewers. Any product that may be evaluated in this article, or claim that may be made by its manufacturer, is not guaranteed or endorsed by the publisher.
Bagatolli L., Gratton E., Khan T. K., Chong P. L.-G. (2000). Two-photon fluorescence microscopy studies of bipolar tetraether giant liposomes from thermoacidophilic archaebacteria sulfolobus acidocaldarius. Biophys. J. 79, 416–425. doi: 10.1016/S0006-3495(00)76303-X
Banta A. B., Wei J. H., Welander P. V. (2015). A distinct pathway for tetrahymanol synthesis in bacteria. Proc. Natl. Acad. Sci. 112, 13478–13483. doi: 10.1073/pnas.1511482112
Baquero D. P., Gazi A. D., Sachse M., Liu J., Schmitt C., Moya-Nilges M., et al. (2021). A filamentous archaeal virus is enveloped inside the cell and released through pyramidal portals. Proc. Natl. Acad. Sci. 118, e2105540118. doi: 10.1073/pnas.2105540118
Barber-Zucker S., Zarivach R. (2017). A look into the biochemistry of magnetosome biosynthesis in magnetotactic bacteria. ACS Chem. Biol. 12, 13–22. doi: 10.1021/acschembio.6b01000
Beck J. G., Mathieu D., Loudet C., Buchoux S., Dufourc E. J. (2007). Plant sterols in “rafts”: a better way to regulate membrane thermal shocks. FASEB J. 21, 1714–1723. doi: 10.1096/fj.06-7809com
Belin B. J., Busset N., Giraud E., Molinaro A., Silipo A., Newman D. K. (2018). Hopanoid lipids: from membranes to plant–bacteria interactions. Nat. Rev. Microbiol. 16, 304–315. doi: 10.1038/nrmicro.2017.173
Berry A., Harriott O., Moreau R., Osman S., Benson D., Jones A. (1993). Hopanoid lipids compose the Frankia vesicle envelope, presumptive barrier of oxygen diffusion to nitrogenase. Proc. Natl. Acad. Sci. United States America 90, 6091–6094. doi: 10.1073/pnas.90.13.6091
Bloch K. (1991). “Chapter 12 Cholesterol: evolution of structure and function,” in New Comprehensive Biochemistry. Eds. Vance D. E., Vance J. E. (Elsevier Amsterdam), 363–381.
Bosak T., Losick R. M., Pearson A. (2008). A polycyclic terpenoid that alleviates oxidative stress. Proc. Natl. Acad. Sci. 105, 6725–6729. doi: 10.1073/pnas.0800199105
Bramkamp M., Lopez D. (2015). Exploring the existence of lipid rafts in bacteria. Microbiol. Mol. Biol. Rev. 79, 81. doi: 10.1128/MMBR.00036-14
Brocks J. J., Nettersheim B. J., Adam P., Schaeffer P., Jarrett A. J. M., Güneli N., et al. (2023). Lost world of complex life and the late rise of the eukaryotic crown. Nature 618, 767–773. doi: 10.1038/s41586-023-06170-w
Bykowski M., Mazur R., Wójtowicz J., Suski S., Garstka M., Mostowska A., et al. (2021). Too rigid to fold: Carotenoid-dependent decrease in thylakoid fluidity hampers the formation of chloroplast grana. Plant Physiol. 185, 210–227. doi: 10.1093/plphys/kiaa009
Camargos H. S., Moreira R. A., Mendanha S. A., Fernandes K. S., Dorta M. L., Alonso A. (2014). Terpenes increase the lipid dynamics in the leishmania plasma membrane at concentrations similar to their IC50 values. PloS One 9, e104429. doi: 10.1371/journal.pone.0104429
Cheng X., Smith J. C. (2019). Biological membrane organization and cellular signaling. Chem. Rev. 119, 5849–5880. doi: 10.1021/acs.chemrev.8b00439
Christianson D. W. (2017). Structural and chemical biology of terpenoid cyclases. Chem. Rev. 117, 11570–11648. doi: 10.1021/acs.chemrev.7b00287
Coleman G. A., Pancost R. D., Williams T. A. (2019). Investigating the origins of membrane phospholipid biosynthesis genes using outgroup-free rooting. Genome Biol. Evol. 11, 883–898. doi: 10.1093/gbe/evz034
Deamer D., Dworkin J. P., Sandford S. A., Bernstein M. P., Allamandola L. J. (2002). The first cell membranes. Astrobiology 2, 371–381. doi: 10.1089/153110702762470482
Deatherage B. L., Cookson B. T. (2012). Membrane vesicle release in bacteria, eukaryotes, and archaea: a conserved yet underappreciated aspect of microbial life. Infect. Immun. 80, 1948–1957. doi: 10.1128/IAI.06014-11
Dibrova D. V., Galperin M. Y., Mulkidjanian A. Y. (2014). Phylogenomic reconstruction of archaeal fatty acid metabolism. Environ. Microbiol. 16, 907–918. doi: 10.1111/1462-2920.12359
Doughty D. M., Hunter R. C., Summons R. E., Newman D. K. (2009). 2-Methylhopanoids are maximally produced in akinetes of Nostoc punctiforme: geobiological implications. Geobiology 7, 524–532. doi: 10.1111/j.1472-4669.2009.00217.x
Eme L., Spang A., Lombard J., Stairs C. W., Ettema T. J. G. (2017). Archaea and the origin of eukaryotes. Nat. Rev. Microbiol. 15, 711–723. doi: 10.1038/nrmicro.2017.133
Erdmann S., Tschitschko B., Zhong L., Raftery M. J., Cavicchioli R. (2017). A plasmid from an Antarctic haloarchaeon uses specialized membrane vesicles to disseminate and infect plasmid-free cells. Nat. Microbiol. 2, 1446–1455. doi: 10.1038/s41564-017-0009-2
Feng X., Hu Y., Zheng Y., Zhu W., Li K., Huang C.-H., et al. (2014). Structural and functional analysis of bacillus subtilis yisP reveals a role of its product in biofilm production. Chem. Biol. 21, 1557–1563. doi: 10.1016/j.chembiol.2014.08.018
Flechsler J., Heimerl T., Huber H., Rachel R., Berg I. A. (2021). Functional compartmentalization and metabolic separation in a prokaryotic cell. Proc. Natl. Acad. Sci. 118, e2022114118. doi: 10.1073/pnas.2022114118
Franza T., Gaudu P. (2022). Quinones: more than electron shuttles. Res. Microbiol., 173 103953. doi: 10.1016/j.resmic.2022.103953
García-Fernández E., Koch G., Wagner R. M., Fekete A., Stengel S. T., Schneider J., et al. (2017). Membrane microdomain disassembly inhibits MRSA antibiotic resistance. Cell 171, 1354–1367.e1320. doi: 10.1016/j.cell.2017.10.012
Gill S., Catchpole R., Forterre P. (2019). Extracellular membrane vesicles in the three domains of life and beyond. FEMS Microbiol. Rev. 43, 273–303. doi: 10.1093/femsre/fuy042
Gold D. A., Caron A., Fournier G. P., Summons R. E. (2017). Paleoproterozoic sterol biosynthesis and the rise of oxygen. Nature 543, 420. doi: 10.1038/nature21412
Gophna U., Altman-Price N. (2022). Horizontal gene transfer in archaea—From mechanisms to genome evolution. Annu. Rev. Microbiol. 76, 481–502. doi: 10.1146/annurev-micro-040820-124627
Hammond A. T., Heberle F. A., Baumgart T., Holowka D., Baird B., Feigenson G. W. (2005). Crosslinking a lipid raft component triggers liquid ordered-liquid disordered phase separation in model plasma membranes. Proc. Natl. Acad. Sci. United States America 102, 6320. doi: 10.1073/pnas.0405654102
Hartley M. D., Imperiali B. (2012). At the membrane frontier: A prospectus on the remarkable evolutionary conservation of polyprenols and polyprenyl-phosphates. Arch. Biochem. Biophys. 517, 83–97. doi: 10.1016/j.abb.2011.10.018
Hartmann M.-A. (1998). Plant sterols and the membrane environment. Trends Plant Sci. 3, 170–175. doi: 10.1016/S1360-1385(98)01233-3
Hashimoto H., Uragami C., Cogdell R. J. (2016). “Carotenoids and Photosynthesis,” in Carotenoids in Nature: Biosynthesis, Regulation and Function. Ed. Stange C. (Cham: Springer International Publishing), 111–139.
Hauß T., Dante S., Dencher N. A., Haines T. H. (2002). Squalane is in the midplane of the lipid bilayer: implications for its function as a proton permeability barrier. Biochim. Biophys. Acta (BBA) - Bioenergetics 1556, 149–154. doi: 10.1016/S0005-2728(02)00346-8
Hederstedt L. (2012). Heme A biosynthesis. Biochim. Biophys. Acta (BBA) - Bioenergetics 1817, 920–927. doi: 10.1016/j.bbabio.2012.03.025
Hölzl G., Dörmann P. (2019). Chloroplast lipids and their biosynthesis. Annu. Rev. Plant Biol. 70, 51–81. doi: 10.1146/annurev-arplant-050718-100202
Hoshino Y., Gaucher E. A. (2021). Evolution of bacterial steroid biosynthesis and its impact on eukaryogenesis. Proc. Natl. Acad. Sci. 118, e2101276118. doi: 10.1073/pnas.2101276118
Hoshino Y., Villanueva L. (2023). Four billion years of microbial terpenome evolution. FEMS Microbiol. Rev. 47, fuad008. doi: 10.1093/femsre/fuad008
Jain S., Caforio A., Driessen A. J. M. (2014). Biosynthesis of archaeal membrane ether lipids. Front. Microbiol. 5, 641. doi: 10.3389/fmicb.2014.00641
Jiang H., Zhang X., Chen X., Aramsangtienchai P., Tong Z., Lin H. (2018). Protein lipidation: occurrence, mechanisms, biological functions, and enabling technologies. Chem. Rev. 118, 919–988. doi: 10.1021/acs.chemrev.6b00750
Jones M. B., Rosenberg J. N., Betenbaugh M. J., Krag S. S. (2009). Structure and synthesis of polyisoprenoids used in N-glycosylation across the three domains of life. Biochim. Biophys. Acta (BBA) - Gen. Subj. 1790, 485–494. doi: 10.1016/j.bbagen.2009.03.030
Jürgens U. J., Simonin P., Rohmer M. (1992). Localization and distribution of hopanoids in membrane systems of the cyanobacterium Synechocystis PCC 6714. FEMS Microbiol. Lett. 92, 285–288. doi: 10.1111/j.1574-6968.1992.tb05275.x
King J. P., Wang A. (2023). Putative roles of terpenoids in primitive membranes. Front. Ecol. Evol. 11. doi: 10.3389/fevo.2023.1272163
Klingl A. (2014). S-layer and cytoplasmic membrane – exceptions from the typical archaeal cell wall with a focus on double membranes. Front. Microbiol. 5. doi: 10.3389/fmicb.2014.00624
Koga Y. (2011). Early evolution of membrane lipids: how did the lipid divide occur? J. Mol. Evol. 72, 274–282. doi: 10.1007/s00239-011-9428-5
Levental I., Lingwood D., Grzybek M., Coskun Ü., Simons K. (2010). Palmitoylation regulates raft affinity for the majority of integral raft proteins. Proc. Natl. Acad. Sci. 107, 22050. doi: 10.1073/pnas.1016184107
Liu J., Soler N., Gorlas A., Cvirkaite-Krupovic V., Krupovic M., Forterre P. (2021). Extracellular membrane vesicles and nanotubes in Archaea. microLife 2, uqab007. doi: 10.1093/femsml/uqab007
Lopez D., Koch G. (2017). Exploring functional membrane microdomains in bacteria: an overview. Curr. Opin. Microbiol. 36, 76–84. doi: 10.1016/j.mib.2017.02.001
LoRicco J. G., Hoffmann I., Caliò A., Peters J. (2023). The membrane regulator squalane increases membrane rigidity under high hydrostatic pressure in archaeal membrane mimics. Soft Matter 19, 6280–6286. doi: 10.1039/D3SM00352C
Mäntynen S., Sundberg L.-R., Oksanen H. M., Poranen M. M. (2019). Half a century of research on membrane-containing bacteriophages: bringing new concepts to modern virology. Viruses 11, 76. doi: 10.3390/v11010076
Mishra S. K., Bae Y. S., Lee Y.-M., Kim J.-S., Oh S. H., Kim H. M. (2021). Sesquiterpene alcohol cedrol chemosensitizes human cancer cells and suppresses cell proliferation by destabilizing plasma membrane lipid rafts. Front. Cell Dev. Biol. 8. doi: 10.3389/fcell.2020.571676
Mullineaux C. W., Liu L.-N. (2020). Membrane dynamics in phototrophic bacteria. Annu. Rev. Microbiol. 74, 633–654. doi: 10.1146/annurev-micro-020518-120134
Nagy P. D. (2022). Co-opted membranes, lipids, and host proteins: what have we learned from tombusviruses? Curr. Opin. Virol. 56, 101258. doi: 10.1016/j.coviro.2022.101258
Nolte-’t Hoen E., Cremer T., Gallo R. C., Margolis L. B. (2016). Extracellular vesicles and viruses: Are they close relatives? Proc. Natl. Acad. Sci. 113, 9155–9161. doi: 10.1073/pnas.1605146113
Omasta B., Tomaskova J. (2022). Cellular lipids - hijacked victims of viruses. Viruses 14, 1896. doi: 10.3390/v14091896
Orf G. S., Blankenship R. E. (2013). Chlorosome antenna complexes from green photosynthetic bacteria. Photosyn. Res. 116, 315–331. doi: 10.1007/s11120-013-9869-3
Pham Q. D., Topgaard D., Sparr E. (2015). Cyclic and linear monoterpenes in phospholipid membranes: phase behavior, bilayer structure, and molecular dynamics. Langmuir 31, 11067–11077. doi: 10.1021/acs.langmuir.5b00856
Poranen M. M., Bamford D. H., Oksanen H. M. (2015). Membrane-containing bacteriophages. eLS, 1–11. doi: 10.1002/9780470015902.a0000779.pub3
Proctor M. S., Sutherland G. A., Canniffe D. P., Hitchcock A. (2022). The terminal enzymes of (bacterio)chlorophyll biosynthesis. R. Soc. Open Sci. 9, 211903. doi: 10.1098/rsos.211903
Rattray J. E., van de Vossenberg J., Hopmans E. C., Kartal B., van Niftrik L., Rijpstra W. I. C., et al. (2008). Ladderane lipid distribution in four genera of anammox bacteria. Arch. Microbiol. 190, 51–66. doi: 10.1007/s00203-008-0364-8
Sáenz J. P., Grosser D., Bradley A. S., Lagny T. J., Lavrynenko O., Broda M., et al. (2015). Hopanoids as functional analogues of cholesterol in bacterial membranes. Proc. Natl. Acad. Sci. United States America 112, 11971–11976. doi: 10.1073/pnas.1515607112
Sáenz J., Sezgin E., Schwille P., Simons K. (2012). Functional convergence of hopanoids and sterols in membrane ordering. Proc. Natl. Acad. Sci. United States America 109, 14236–14240. doi: 10.1073/pnas.1212141109
Salvador-Castell M., Brooks N. J., Peters J., Oger P. (2020). Induction of non-lamellar phases in archaeal lipids at high temperature and high hydrostatic pressure by apolar polyisoprenoids. Biochim. Biophys. Acta (BBA) - Biomembranes 1862, 183130. doi: 10.1016/j.bbamem.2019.183130
Salvador-Castell M., Tourte M., Oger P. M. (2019). In search for the membrane regulators of archaea. Int. J. Mol. Sci 20, 4434. doi: 10.3390/ijms20184434
Santana-Molina C., Rivas-Marin E., Rojas A. M., Devos D. P. (2020). Origin and evolution of polycyclic triterpene synthesis. Mol. Biol. Evol. 37, 1925–1941. doi: 10.1093/molbev/msaa054
Sato T. (2013). Unique biosynthesis of sesquarterpenes (C35 terpenes). Biosci. Biotechnol. Biochem. 77, 1155–1159. doi: 10.1271/bbb.130180
Schüler D. (2008). Genetics and cell biology of magnetosome formation in magnetotactic bacteria. FEMS Microbiol. Rev. 32, 654–672. doi: 10.1111/j.1574-6976.2008.00116.x
Sévin D. C., Sauer U. (2014). Ubiquinone accumulation improves osmotic-stress tolerance in Escherichia coli. Nat. Chem. Biol. 10, 266–272. doi: 10.1038/nchembio.1437
Sezgin E., Levental I., Mayor S., Eggeling C. (2017). The mystery of membrane organization: composition, regulation and roles of lipid rafts. Nat. Rev. Mol. Cell Biol. 18, 361. doi: 10.1038/nrm.2017.16
Shaw T. R., Ghosh S., Veatch S. L. (2020). Critical phenomena in plasma membrane organization and function. Annu. Rev. Phys. Chem 72, 51–72. doi: 10.1146/annurev-physchem-090419-115951
Si L., Meng K., Tian Z., Sun J., Li H., Zhang Z., et al. (2018). Triterpenoids manipulate a broad range of virus-host fusion via wrapping the HR2 domain prevalent in viral envelopes. Sci. Adv. 4, eaau8408. doi: 10.1126/sciadv.aau8408
Simonin P., Jürgens U., Rohmer M. (1996). Bacterial triterpenoids of the hopane series from the prochlorophyte Prochlorothrix hollandica and their intracellular localization. Eur. J. biochemistry/FEBS 241, 865–871. doi: 10.1111/j.1432-1033.1996.00865.x
Sinensky M. (2000). Functional aspects of polyisoprenoid protein substituents: roles in protein–protein interaction and trafficking. Biochim. Biophys. Acta (BBA) - Mol. Cell Biol. Lipids 1529, 203–209. doi: 10.1016/S1388-1981(00)00149-9
Skotland T., Sagini K., Sandvig K., Llorente A. (2020). An emerging focus on lipids in extracellular vesicles. Adv. Drug Delivery Rev. 159, 308–321. doi: 10.1016/j.addr.2020.03.002
Stankeviciute G., Tang P., Ashley B., Chamberlain J. D., Hansen M. E. B., Coleman A., et al. (2022). Convergent evolution of bacterial ceramide synthesis. Nat. Chem. Biol. 18, 305–312. doi: 10.1038/s41589-021-00948-7
Takishita K., Chikaraishi Y., Leger M. M., Kim E., Yabuki A., Ohkouchi N., et al. (2012). Lateral transfer of tetrahymanol-synthesizing genes has allowed multiple diverse eukaryote lineages to independently adapt to environments without oxygen. Biol. Direct 7, 5. doi: 10.1186/1745-6150-7-5
Tang S.-K., Zhi X.-Y., Zhang Y., Makarova K. S., Liu B.-B., Zheng G.-S., et al. (2023). Cellular differentiation into hyphae and spores in halophilic archaea. Nat. Commun. 14, 1827. doi: 10.1038/s41467-023-37389-w
Tóth T. N., Chukhutsina V., Domonkos I., Knoppová J., Komenda J., Kis M., et al. (2015). Carotenoids are essential for the assembly of cyanobacterial photosynthetic complexes. Biochim. Biophys. Acta (BBA) - Bioenergetics 1847, 1153–1165. doi: 10.1016/j.bbabio.2015.05.020
Tourte M., Schaeffer P., Grossi V., Oger P. M. (2020). Functionalized membrane domains: an ancestral feature of archaea? Front. Microbiol. 11. doi: 10.3389/fmicb.2020.00526
van Meer G., Voelker D., Feigenson G. (2008). Membrane lipids: where they are and how they behave. Nat. Rev. Mol. Cell Biol. 9, 112–124. doi: 10.1038/nrm2330
van Niftrik L. A., Fuerst J. A., Damsté J. S. S., Kuenen J. G., Jetten M. S. M., Strous M. (2004). The anammoxosome: an intracytoplasmic compartment in anammox bacteria. FEMS Microbiol. Lett. 233, 7–13. doi: 10.1016/j.femsle.2004.01.044
van Wolferen M., Pulschen A. A., Baum B., Gribaldo S., Albers S.-V. (2022). The cell biology of archaea. Nat. Microbiol. 7, 1744–1755. doi: 10.1038/s41564-022-01215-8
Villanueva L., von Meijenfeldt F. A. B., Westbye A. B., Yadav S., Hopmans E. C., Dutilh B. E., et al. (2021). Bridging the membrane lipid divide: bacteria of the FCB group superphylum have the potential to synthesize archaeal ether lipids. Isme J. 15, 168–182. doi: 10.1038/s41396-020-00772-2
Waheed A. A., Freed E. O. (2010). The role of lipids in retrovirus replication. Viruses 2, 1146–1180. doi: 10.3390/v2051146
Willdigg J. R., Helmann J. D. (2021). Mini review: bacterial membrane composition and its modulation in response to stress. Front. Mol. Biosci. 8. doi: 10.3389/fmolb.2021.634438
Keywords: membrane evolution, membrane dynamics, terpenoids, Archaea, LUCA (the last universal common ancestor), membrane organization
Citation: Hoshino Y (2024) Terpenoids and membrane dynamics evolution. Front. Ecol. Evol. 12:1345733. doi: 10.3389/fevo.2024.1345733
Received: 28 November 2023; Accepted: 18 January 2024;
Published: 01 February 2024.
Edited by:
Michael Schubert, UMR7009 Laboratoire de Biologie du Développement de Villefranche sur Mer, FranceReviewed by:
Diana X. Sahonero-Canavesi, Royal Netherlands Institute for Sea Research (NIOZ), NetherlandsCopyright © 2024 Hoshino. This is an open-access article distributed under the terms of the Creative Commons Attribution License (CC BY). The use, distribution or reproduction in other forums is permitted, provided the original author(s) and the copyright owner(s) are credited and that the original publication in this journal is cited, in accordance with accepted academic practice. No use, distribution or reproduction is permitted which does not comply with these terms.
*Correspondence: Yosuke Hoshino, eWhvc2hpbm9AZ2Z6LXBvdHNkYW0uZGU=; eWhvc2hpbm8wNkBnbWFpbC5jb20=
Disclaimer: All claims expressed in this article are solely those of the authors and do not necessarily represent those of their affiliated organizations, or those of the publisher, the editors and the reviewers. Any product that may be evaluated in this article or claim that may be made by its manufacturer is not guaranteed or endorsed by the publisher.
Research integrity at Frontiers
Learn more about the work of our research integrity team to safeguard the quality of each article we publish.