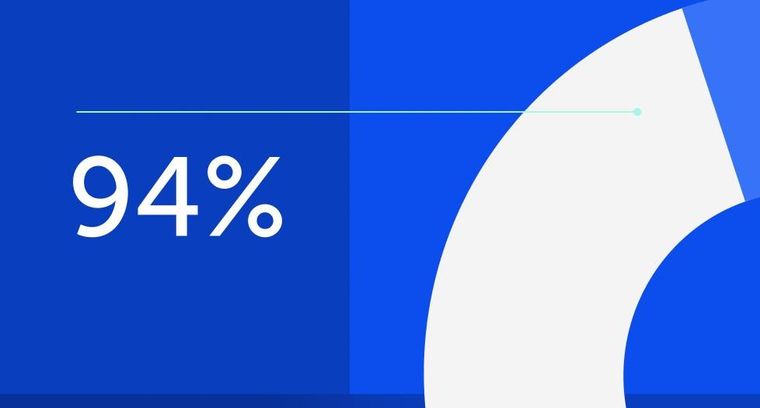
94% of researchers rate our articles as excellent or good
Learn more about the work of our research integrity team to safeguard the quality of each article we publish.
Find out more
ORIGINAL RESEARCH article
Front. Ecol. Evol., 14 March 2024
Sec. Coevolution
Volume 12 - 2024 | https://doi.org/10.3389/fevo.2024.1333028
This article is part of the Research TopicRising Stars in Coevolution 2022/2023View all 5 articles
Introduction: In the upside-down jellyfish, Cassiopea xamachana (Cnidaria: Scyphozoa), the establishment of photosymbiosis with dinoflagellates (family Symbiodiniaceae) is necessary for the sessile polyp to undergo metamorphosis (strobilation) into a free-swimming adult. C. xamachana has the capacity to associate with a wide variety of dinoflagellate species and representatives of divergent genera. While some studies have looked at the successful induction of symbiosis, none to date have examined the lasting effect of diverse symbiont taxa on host survivorship and development, which is needed to assess the fitness costs of such symbioses.
Methods: Our study exposes C. xamachana polyps to 22 different cultured Symbiodinaceae strains representing 13 species from 5 genera. We analyzed the time to strobilation, the number of ephyra (juvenile medusa) produced, and the proportion of ephyra that died prematurely.
Results: Here we show that C. xamachana strobilation can be induced by nearly each symbiodinacean strain we tested, with the exception of free-living species (i.e., unknown to establish symbiosis with any other marine host). Additionally, ephyrae did not display morphological variation or survivorship differences with varying symbionts. However, we observed intraspecific variation in time to induce strobilation with different cultured dinoflagellate strains.
Discussion: This work expands the known symbiont species that can form stable mutualisms with C. xamachana, primarily in the genera Symbiodinium and Breviolum. Additionally, we provide evidence of differences in ability of cultured symbiodiniaceans to establish symbiosis with a host, which suggests population-level differences in dinoflagellate cultures impact their symbiosis success. By utilizing an animal like C. xamachana with flexible symbiont uptake, we are able to explore how symbiont diversity can influence the timing and success of symbiosis-driven development.
Numerous marine animals receive nutritional benefits from mutualistic microbes. The presence of certain microorganisms is also critical for the animal during the early stages of development (McFall-Ngai et al., 2013). It has become increasingly evident that most, if not all, marine organisms rely on the presence of symbionts throughout their life cycles, as reviewed by González-Pech et al. (2024). For example, many invertebrate larvae rely on the presence of a bacterial biofilm to settle and develop into adults and require the presence of specific bacteria for settlement (Freckelton et al., 2017; Cavalcanti et al., 2020). In the Hawaiian bobtail squid Euprymna scolopes (Mollusca : Cephalopoda), symbiosis with diverse bacteria is necessary for the proper development of the female reproductive system (McAnulty et al., 2023). Many cnidarians, including reef-building corals, sea anemones, sea fans, and jellies, depend upon mutualisms with endocellular dinoflagellate photosymbionts (Dinophyceae: Suessialess: Symbiodinaceae) (LaJeunesse, 2020). Newly metamorphosed polyps of these animals will fail to survive without a compatible algal symbiont (McIlroy et al., 2019). Symbiont acquisition is therefore critical for survivorship.
In animal–dinoflagellate mutualisms, most hosts acquire their symbionts horizontally from environmental sources during the early stages of development (Harii et al., 2009; Fransolet et al., 2012; Baird et al., 2021). Initial infections may not be representative of the symbiont species found in adult animals, indicating the potential for ontogenetic flexibility in early symbiont uptake (Thornhill et al., 2006; Mellas et al., 2014). Despite this lack of symbiont specificity in early development, a winnowing process occurs, and the “host-specific,” or homologous, symbiont becomes rapidly prevalent (Coffroth et al., 2001; McIlroy et al., 2019). While this sorting process occurs in nature, little is known about the long-term compatibility of heterologous symbionts and how these mutualisms would perform if the homologous symbiont were absent. This and larger questions about factors governing host–symbiont specificity, such as developmental phenotype trajectories, may be addressed under controlled experimental conditions.
The upside-down jellyfish Cassiopea xamachana (Cnidaria: Scyphozoa) is an established experimental system, useful for investigating animal–dinoflagellate interactions (Lampert, 2016; Ohdera et al., 2018; Medina et al., 2021). In the wild, this jellyfish establishes a nutritional and developmental symbiosis with Symbiodinium microadriaticum, a photosynthetic dinoflagellate in the family Symbiodiniaceae (Hofmann et al., 1978; Thornhill et al., 2006; Mellas et al., 2014; LaJeunesse, 2017; Medina et al., 2021). Unique to Cassiopea spp., the host experiences halted sexual development until symbiosis is established (Jarms and Morandini, 2019; Medina et al., 2021). Soon after, C. xamachana undergoes a metamorphic process termed strobilation, during which the polyp head develops into a pre-adult swimming ephyra. These ephyrae detach from the polyp through lateral fission and develop into adult medusae (Figure 1A). The polyp remains behind and regenerates a new head, undergoing strobilation two to three more times (Hofmann et al., 1978; Hofmann and Brandt, 1987; Hofmann et al., 1996; Medina et al., 2021). Without the presence of a symbiont, C. xamachana remains in the polyp stage indefinitely, though when fed, it will propagate itself through asexual budding (Figure 1A). The ability to maintain these animals aposymbiotically and infect them with individual strains representative of different symbiont species makes this animal ideal for assessing symbiont specificity and the relative compatibility and performance of different algal partner combinations (Medina et al., 2021).
Figure 1 Cassiopea xamachana life cycle and experimental design. (A) C. xamachana strobilation. Algal ingestion and subsequent symbiosis establishment occurred at day 0. Scale bar for algae in black (1 mm); scale bar for host in white (1 mm). (B) Number of days each trial was run, number of species, and number of algal strains. Symbionts were introduced on day 0. (C) List of symbiont species, algal strains, and average number of days to strobilation. Trial 1’s strains and species shown in orange, while trials 2 and 3 used the same strains and species, outlined in blue and purple, respectively.
Although S. microadriaticum is the homologous symbiont of adult C. xamachana (Thornhill et al., 2006; LaJeunesse, 2017), juvenile jelly polyps have been shown to uptake symbionts across several Symbiodiniaceae genera (Mellas et al., 2014; Newkirk et al., 2020; Medina et al., 2021). While C. xamachana polyps have been shown to associate with phylogenetically diverse symbiodiniaceans up until strobilation (reviewed in LaJeunesse, 2001), this symbiosis has not been monitored ontogenetically through the resulting ephyrae. It is currently unknown whether heterologous symbioses in these cnidarians are maintained after strobilation and how they may affect host phenotype and fitness. To observe how a cnidarian host responds to heterologous algal symbionts, we introduced numerous species and strains from five symbiodiniacean genera to clonal aposymbiotic polyps. We measured developmental phenotypes, such as time to strobilation, growth, and survivorship, of both polyps and ephyra. Here, we report wide phenotypic plasticity in host developmental response to a broad range of algal symbionts up to approximately 3 months after symbiont uptake.
Aposymbiotic C. xamachana polyps were obtained from a clonal line kept by the Medina Lab (Pennsylvania State University). Each line represents one originating aposymbiotic polyp collected from the Florida Keys, USA, that was allowed to bud continuously, forming a clonal colony. Line T1C was used for trials 1 and 3, and T1D was used for trial 2 (Figures 1B, C).
One polyp was placed in each well of a six-well CellStar cell culture plate (Thomas Scientific, Swedesboro, NJ, USA) with approximately 7–9 mL of 35 parts per thousand (ppt) Instant Ocean (Spectrum Brands, Inc., Miramar, FL, USA) seawater (n = 6 per algal treatment). Polyps were fed daily with two to fifteen 1-day-old Artemia franciscana hatched from eggs purchased from Brine Shrimp Direct, Inc. (Ogden, UT, USA), and water changes were performed three times a week. Aposymbiotic polyps were kept in an incubator with a 12:12 day/night cycle (27.5°C day, 25.5°C night) for 2 weeks prior to the start of the experiment to acclimate them to the light cycle. Light levels ranged between 70 and 150 μmol·m−2·s−1 depending on proximity to the fluorescent lights. Therefore, plates were rotated daily to ensure even light exposure over the course of the experiment.
Symbiodiniaceae cultures (Supplementary Table 1) cultured and/or maintained by the LaJeunesse lab (Pennsylvania State University) were grown in Asp-8A media (Blank, 1987) for 2–4 months prior to infections. They were grown at ~180 µmol·m−2·s−1 lighting in a 12:12 day:night cycle of 27.5°C during the day and 26°C at night. Prior to the start of the experiment, culture cell density was calculated by counting live algal cells on a hemacytometer. Culture aliquots were pelleted at 0.8 rcf, and the media were removed before resuspension with an equal volume of 35 ppt seawater. Symbionts were added at a concentration of ~150,000 cells/mL per polyp to each 9-mL well of a six-well sterile tissue culture plate along with live A. franciscana to encourage the immediate ingestion of algae. A control plate with aposymbiotic polyps was kept in the same incubator and fed and cleaned on the same schedule as plates with symbiotic polyps.
The identity of algal cultures was confirmed by Sanger sequencing of the 28S (LSU) ribosomal DNA, and a conserved marker was used to identify Symbiodiniaceae genera (forward: 5′-CCCGCTGAATTTAAGCATATAAGTAAGCGG-3′; reverse: 5′-GTTAGACTCCTTGGTCCGTGTTTCAAGA-3′) (LaJeunesse et al., 2018). DNA extraction and polymerase chain reaction followed the methods described in LaJeunesse et al. (2018). Sequencing was performed at the Pennsylvania State Genomic Core Facility. Sequences were imported into Geneious Prime® 2023.0.4 (https://www.geneious.com), and BLAST was performed against the LSU rRNA sequences from LaJeunesse et al. (2018). Geneious Tree Builder was used to generate a neighbor-joining phylogenetic tree using the Tamura–Nei genetic distance model, and the outgroup was set to an LSU sequence of Biecheleria halophila, a dinoflagellate from a different family. Photographs were taken of each culture using a Leica MZ16 F fluorescence stereo microscope. The length (longest dimension) of ~20 cells per culture was measured using ImageJ (Schneider et al., 2012) to calculate average cell size. Only cells not actively dividing were used for this analysis.
Out of 22 Symbiodiniaceae cultures sequenced, three LSU sequences did not match their recorded species; RT362 and RT370 were reported as S. microadriaticum but matched to Symbiodinium tridacnidorum, and MAC1163 was reported as Breviolum minutum and matched to Symbiodinium necroappetens (Supplementary Table 1, bolded and underlined). While these new identifications do not affect our results, we note this information for anyone looking for a particular culture used in this study. Unique labels termed “Species ID” were created for each algal culture and were used herein for figures and discussion (Figure 1C, Supplementary Table 1). Species IDs use the first letter of the genus and the first three letters of the species, followed by a number if it is a different culture of the same microalgal species. For example, two cultures of S. microadriaticum, the homologous symbiont, were used in this experiment, labeled as KB8 and CCMP2464 by initial cultivists. KB8 received the Species ID “Smic”, while CCMP2464 was labeled “Smic2.” All results were compared between algal species and algal cultures.
An initial experiment, run from January to March 2020 (50 days, Figure 1B), introduced six algal species to aposymbiotic C. xamachana polyps (clonal line T1C, Supplementary Table 1). The rate of strobilation and morphological variation of the host was recorded visually. Pictures were taken of the ephyrae once a day to measure the growth rate by calculating the change in host diameter over time. Anesthesia was not used for this; instead, photo bursts were used to ensure a photo was taken of the ephyra at maximum diameter while pulsing. Ephyra diameter was measured using ImageJ. Some ephyrae perished within 1 day of detachment and were only measured once. These deaths made it difficult to accurately compare growth rates, as there was no measurement of ephyra decline in size leading to death in these samples. The algal species that had colonized these ephyra included the homologous symbiont S. microadriaticum (Thornhill et al., 2006) as well as symbiont species historically used in Medina Lab experiments (see Supplementary Table 1).
A second experiment, run from January to February 2021 (28 days, Figure 1B), introduced 22 algal strains (complete list in Supplementary Table 1) to polyps (clonal line T1D). Clonal polyp lines T1C and T1D, with different genotypes, were previously colonized with various symbionts without noticeable differences in symbiosis establishment. Polyps were imaged three times a week to measure growth, time to strobilation, and to record potential morphological differences (i.e., tentacle numbers, strobilation progress, and mortality). When ephyrae detached, they were kept in the same well as the polyp they originated from and experienced the same conditions. Ephyrae were imaged one to two times a day to measure growth and mortality. As ephyra growth measurements were taken twice a day, the approximate number of hours since the time of infection was recorded for each time point and used to calculate the average ephyra growth rate. Ephyra diameter across the bell margin was measured using ImageJ for each time point to calculate the average change in diameter.
A third experiment, run from July to August 2021 (52 days, Figure 1B), introduced the same 22 algal strains to the polyp clonal line T1C. Data collection was identical to the second experimental run. All three experiments were pooled to compare host response to algal species and days to strobilation (see trial summary in Figure 1C). Analyses were performed on pooled results and separated by experiment to account for any experimental effects.
A Zero-Inflated Poisson Regression was chosen to analyze the number of days to strobilation, as it accounts for excess zeros in a dataset, e.g., polyps that were visibly symbiotic but did not strobilate in trial 2. Data analysis and figure generation were performed using the ggplot2, dplyr, emmeans, and ggfortify packages in R version 4.1.2 (Searle et al., 1980; Tang et al., 2016; Wickham, 2016; R Core Team, 2018; Wickham et al., 2022). The cladogram was generated using FigTree (http://tree.bio.ed.ac.uk/software/Figtree/) and edited using Adobe Illustrator (Adobe Inc.). A Bonferroni p-value correction was applied to all tests that had multiple comparisons by dividing the p-value cutoff (p < 0.05) by the number of comparisons (n) (Tables 1, 2).
Table 1 Results of zero-inflated Poisson regressions on significance of factors on days to strobilation.
Sixteen algal strains from eight species induced strobilation in at least one trial (Figure 2). Six algal strains representing four species did not induce strobilation in all three experiments (Spil, Snat, Evor, Evor2, Mlee, and Fkaw; Figure 2, Supplementary Table 1). These six strains did not visibly colonize polyps, as determined by the absence of visible symbiont cells in the translucent head of the polyp (Figure 3B). In another study conducted by several of the authors, symbionts were visible inside the polyp to the human eye and were verified by fluorescent microscopy, supporting the unofficial metric of symbiosis (Mammone et al., 2023). Visible symbiont cells were observed in the polyp head of all strains that induced strobilation (Figure 3B). Ten out of the 22 strains failed to induce strobilation during trial 2, despite confirmation of the successful acquisition of the symbiont cells by the polyps (Smic2, Stri, Stri2, Stri3, Stri4, Snec, Snec2, Snec3, Ssp, and Baen2). All 10 strains induced strobilation during trial 3. These strains were binned by “Fast” (0–20 days), “Medium” (20–30 days), and “Slow” (30 or more days) and mapped onto a phylogenetic tree of our LSU sequences (Figure 4, outgroup taken from LaJeunesse et al., 2018, dataset). There was no pattern observed for phylogenetic distance and bin, but strains that did not induce strobilation clustered together (Figure 4).
Figure 2 Number of days to strobilation pooled across three trials. Y-axis group Species_ID by genera and color-coded by species. Each point represents one polyp inoculated with that Species_ID. Column on right side of figure lists number of polyps that did not strobilate out of the total inoculated across all three trials (column 1) and average algal cell size (column 2). Point size corresponds to average algal cell size, as shown in column 2. Species_ID of those that never induced strobilation or showed any signs of symbiosis in any trial is listed in “No strobilation in any trials” box at bottom.
Figure 3 Representative images of Cassiopea xamachana morphology when associating with different symbionts. (A) Polyps (column 1) and their produced ephyra (column 2) along with final appearance of ephyrae (column 3) and algal cultures (right). Black scale bars represent 10 mm. Each row represents a different algal culture. Rows 2 and 4 show ephyrae that were deceased by the final day (day 52 during trial 3). (B) Aposymbiotic polyp (top) and symbiotic polyp (bottom) with an arrow indicating the visible symbionts inside the polyp head. Images approximately 50 mm in width.
Figure 4 Phylogeny of the 28S (LSU) ribosomal RNA gene sequenced from algal cultures used. Genetic distance scale bar at bottom. Outgroup in blue font. Each culture is color-coded by the average number of days it took to induce strobilation (see Supplementary Table 1).
No statistically significant differences in time to strobilation (measured in number of days post-dinoflagellate introduction) were observed between algal strains when the three experimental results were grouped (zero-inflated Poisson regression; p = 0.73203) (Table 1). When the results were grouped by species, we found that Breviolum pseudominutum decreased the number of days it took to strobilate compared to the averages of other species (average of 20 days, zero-inflated Poisson regression, p = 0.0475), while S. tridacnidorum increased the number of days it took to strobilate (average of 28 days, zero-inflated Poisson regression, p = 0.0318) (Supplementary Table 1). Bonferroni corrections for multiple comparisons did not change any p-value significance except when algal species, strain, and trial were compared against the number of days to strobilation, which resulted in only the trial achieving significance after the p-value correction (Table 1).
The average algal cell size is recorded in Supplementary Table 1. The strain sizes ranged from 7.6 to 12.01 µm. There were five species with multiple strains, where Breviolum aenigmatum had the lowest standard deviation in average algal cell size between strains (7.6–7.63 µm, SD = 0.011). Effrenium voratum (11.28–12.01 µm, SD = 0.334), S. microadriaticum (8.97–9.64 µm, SD = 0.335), and S. necroappetens (9.39–10 µm, SD = 0.25) had similar standard deviations. S. tridacnidorum had the largest range in algal cell size between strains (7.78–9.9 µm, SD = 0.85).
Using only the data from species that induced strobilation, a zero-inflated Poisson regression tested the correlation between average dinoflagellate length and time to strobilation. When algal cell size and species or algal cell size and strain were considered, we found no significant effect on time to strobilation (Table 1). When species, algal strains, trial, and average algal cell diameter were considered together, we found significant differences (p = 0.010206) in the number of days to strobilation. Algal strain and size were not associated with time to strobilation in any comparison. Bonferroni corrections for multiple comparisons were conducted on all tests, and the p-value cutoff (p < 0.05) was divided by the number of comparisons (n) to establish a new p-value cutoff for those tests (Table 1).
No quantitative measurements were taken for polyp and ephyra morphology beyond ephyra diameter, only visual assessment. Polyp and ephyra shape, tentacles, bell margin, and coloration appeared consistent across association with different symbiont species (Figure 3A). The total number of ephyra produced by algal treatments that induced strobilation was compared against algal species/culture for significance (Table 2). The total number of days the ephyrae survived, the total number of produced ephyrae, and the proportion of deceased ephyrae out of the total produced were compared. Species and algal culture were not significantly associated with these outcomes except for algal strain compared against a proportion of deceased ephyra (one-way ANOVA; Table 2). A post-hoc test found that no strain drove this significance. A chi-squared test was performed to determine if species or algal culture played a significant role in whether or not ephyra lived 14 days or less and the proportion of ephyra that survived at least 14 days. The total number of produced ephyra for this analysis excluded any ephyra produced within 14 days of the end of the experiment. No significant association was found between algal species or algal culture and either comparison (whether ephyrae lived 14 days or less or the proportion that survived 14 days or more) (Figure 5, Table 1). When the ephyra growth rate was calculated, average diameter change was not affected by algal species or algal culture (one-way ANOVA, p = 0.5742 and p = 0.05804, respectively; Table 2). As none of the ANOVA or chi-squared tests achieved significance, no post-hoc tests were performed.
Figure 5 Box plot of the number of days ephyra survived (y-axis) sorted by algal species (x-axis). Boxplots of strains of the same species are clustered and color coded according to the key.
Our results support the flexibility of C. xamachana to form symbiosis with a variety of Symbiodiniaceae species from the Breviolum and Symbiodinium genera. In fact, the homologous S. microadriaticum did not induce strobilation faster on average than other species (Figures 2, 4). This lack of correlation between algal species and host metamorphosis suggests that despite C. xamachana being predominantly associated with S. microadriaticum in the wild (Thornhill et al., 2006), strobilation does not require the acquisition of the homologous symbiont, and the developmental transition can be initiated by a wide range of symbiodiniacean taxa. The species that did not establish symbiosis have been predominantly recorded as non-symbiotic and solely free-living (Jeong et al., 2014; LaJeunesse et al., 2018; Pochon and LaJeunesse, 2021) (Figure 2). While these two symbiotic dinoflagellates cannot associate with C. xamachana, they present interesting subjects for future studies on the specificity of host–symbiont interactions. Otherwise, most symbiodiniaceans capable of forming symbiosis with a marine host appear to be able to associate with C. xamachana. While we did not utilize them in this study, other wild Cassiopea species have been reported to host symbionts in the genera Cladocopium and Durisdinium (Winstead, 2019; Mammone et al., 2021).
C. xamachana has differing abilities to establish symbiosis depending on the symbiodiniacean algal strain, including the time from aposymbiotic host exposure to dinoflagellate cells, to strobilation. While algal strain did not significantly affect time to strobilation across the full dataset, not divided by trial (Table 1), if algal strains within the same species are compared, a noticeable difference in average number of days to strobilation is visible (Figures 2, 4, Supplementary Table 1). Whether these differences are due to the origins of the different cultures (i.e., the algal cultures were isolated from specific hosts), or from the effect of algal generation time, is yet to be determined. The dinoflagellates used in this study have been kept in isolated cultures for multiple years and may have experienced microevolutionary events, possibly dividing monoclonal cultures into subpopulations among culture flasks. As seen in the 2021 study by Gonzalez-Pech et al., Symbiodiniaceae cultures show varying levels of divergence depending on the culture origin. As many cnidarian studies use lab-reared algal cultures passed between research groups (Rahat and Reich, 1986; Abrego et al., 2009; Newkirk et al., 2020), it is crucial to begin to understand whether different cultures of the same algal species function differently to establish the significance for future studies on artificial symbiosis induction.
When looking further into the number of days to strobilation among different strains of symbiont, this effect was greater when results from individual experiments were separated (Table 1). Due to the different periods of time that each experiment was run (Figures 1B, C), it is clear that the lack of strobilation in trial 2 was due to the shorter time frame (visualized in Supplementary Figure 1) and unlikely due to other differences such as clonal polyp line (T1C in trials 1 and 3 and T1D in trial 2). This is further supported by algal species having a significant association with time to strobilation when all data were grouped, but not when divided by trial (Table 1). Additionally, when species, trial, and average cell size were compared, species achieved significance (Table 1). After running a post-hoc test, this significance was driven by the differences in the algal cell sizes of species that induced strobilation in trial 3, but not trial 2. The post-hoc test (run using the R-function “emmeans”) indicated that it expected that no species with cells larger than 8.77 µm should have induced strobilation. As an example, culture Snec, with an average cell size of 10 µm, did not induce strobilation in trial 2; however, when the length of the experiment was extended for trial 3, this culture induced strobilation. This difference serves to further highlight the differences between algal strains and timelines to induce strobilation, and this range is useful for researchers to keep in mind when observing hosts interacting with different symbiont strains.
Strobilation in C. xamachana can be induced by a chemical stimulant (Cabrales-Arellano et al., 2017), indicating that once polyps receive the signal for strobilation, the metamorphic pathway will continue independent of colonization by a symbiont. Our results support this with natural symbiont infections; the strobilation cascade proceeds along a designated developmental trajectory. Considering the host relies on the presence of the symbiont in the wild to undergo a morphogenetic transition, we hypothesized that different symbiont genotypes may influence host phenotype. However, no morphological differences were observed between polyps and ephyra colonized by different species, nor any visual differences in animal coloration, tentacle/oral arm development or abundance, or shape of the polyps (Figure 3). This reveals that animal morphogenesis during strobilation proceeds independently of the algal species. The average number of days to strobilation for each strain fell within a range of 19–36 days (Supplementary Table 1), and polyps inoculated with the same strain did not always strobilate within the time window (Figure 2). Strobilation timing was highly variable and dependent on symbiont, uncovering broad host phenotypic plasticity despite the single clonal nature of the initial experimental polyps. Plasticity in the initiation of strobilation has been seen in other scyphozoans when temperatures and feeding regimes fluctuated, but this is the first example of this temporal shift involving the presence of other symbionts (Prieto et al., 2010; Treible and Condon, 2019). The monodisc strobilation style of C. xamachana is thought to be an evolutionarily derived strategy (Marques and Collins, 2004), but there appears to be a similar pattern in polydisc strobilating scyphozoans; strobilation timing can change depending on the environment proceeding in a cascade after initiation.
Biquand et al. (2017) speculated that algal cell size would be positively correlated with the time to establish symbiosis with a cnidarian host. However, our data regarding the timing of strobilation, which is triggered after the establishment of symbiosis, do not support this theory. Several symbiont strains led to symbiosis, characterized by visible “spots” in the animals in the upper part of the calyx (Figure 3B), but did not induce strobilation in every polyp. These symbiont strains covered a wide range of cell diameters (Smic2, Stri, Stri2, Stri3, Stri4, Snec, Snec2, Snec3, Ssp, and Baen2, 7.6 to 9.9 μm; Supplementary Table 1), suggesting in this scyphozoan host that symbiont cell size is not correlated with time to symbiosis establishment.
B. pseudominutum induced strobilation the fastest in all three trials (Figure 2). This species is found in sub-tropical environments (Parkinson et al., 2015) and is expected to co-occur in the same water as wild C. xamachana. Conversely, B. aenigmatum, which fell within the medium range of days to induce strobilation (Figure 4), has only been isolated and cultured as a background fraction of symbionts for the Caribbean coral Porites astreoides (Parkinson et al., 2015). B. aenigmatum’s ability to grow and persist despite occurring as a rare member of the photosymbiont community in these hosts may indicate an increased capacity to infiltrate and reproduce once inside a host. This capacity could explain its success in inducing C. xamachana strobilation, which we can further explore in the future. The species S. tridacnidorum, which is naturally found within tridacnid clams (Lee et al., 2015), was associated with a longer time to strobilation independent of cell size, which varied across strains of this species. This result suggests that the successful establishment of symbiosis depends on other factors than cell size. In addition, the free-living symbiont species that did not establish symbiosis with C. xamachana had a variety of cell sizes (Supplementary Table 1).
The survivorship of ephyrae produced by any one photosymbiont strain appears to be highly variable, and we found no significant association between algal species or cultures and the total number of produced ephyrae or their survivorship (Figure 5, Table 2). The exception to this was when the proportion of deceased ephyra was compared against the algal strain (Table 2). However, no individual strain drove this significance in the post-hoc test. Given the small sample size of ephyrae and the variability in numbers between strains (Figure 5), this may be a false positive. Further experiments would need to be conducted with a higher sample size. In our experiments, ephyrae with the homologous symbiont did not have a greater likelihood of survival, so it does not appear that the persistence of ephyrae to adulthood is reliant on initial symbiont association (Table 2, Figure 5). It has been observed in other marine invertebrates that early developmental stages tend to be generalists in symbiont uptake, but as the symbiosis matures and the juvenile individual acquires its homologous (coevolved) symbiont, there is a winnowing of the heterologous symbionts when reaching adult stages (Coffroth et al., 2001; McIlroy et al., 2019). This is also the case of C. xamachana (Thornhill et al., 2006), and because the morphogenetic transition from polyp to ephyra is so critical for lifecycle completion, it may be that this early symbiosis promiscuity aids the survivorship of this host and may be an adaptive trait that is shared among photosymbiotic marine invertebrates.
We saw no cases of loss of symbiosis, showing that C. xamachana symbiosis is highly stable in laboratory conditions once established. Several species and strains of algae produced more ephyrae over the course of the experiments due to faster strobilation induction (Figures 2, 5), but there was no significant association between any algal species and strain and ephyra survivorship (Table 2). Symbionts in the genus Breviolum did seem to consistently induce strobilation the fastest, but we found no pattern for the genus Symbiodinium and the number of days to strobilation. The two algal strains of the homologous symbiont, S. microadriaticum, also had different average times to strobilation, showing a clear difference in strains of the same species, and failing to support the hypothesis that the homologous symbiont always results in a higher production rate of ephyra (Figure 4). Long-term observation of developing C. xamachana as they grow into medusae with different symbionts would need to be performed to conclusively state that there is no change in host fitness. It is also possible that while we observed large phenotypic plasticity at the morphological level with no apparent fitness costs, there may be distinct molecular processes that can have downstream fitness effects on host survival that could not be captured in our study due to the length of the experiments. The associated algal microbiomes appear to have a potential nutritional role to the assembled holobiont that could also have a key role in fitness and survival (Medina Lab, unpublished).
Overall, we found that life history, animal host origin, and ecological niche do not affect the success of mutualistic Symbiodiniaceae species in forming a symbiosis with this marine host in a lab environment. The results of this study suggest that symbiosis in C. xamachana is highly flexible regardless of symbiont host origin or phylogenetic relatedness to the homologous symbiont, providing valuable information for symbiosis researchers on compatible symbionts in this system, the timing of strobilation, and survivorship of ephyrae. Symbiodiniaceae contains a large number of symbionts to marine hosts, and many of these comprise obligate symbioses with cnidarians (LaJeunesse et al., 2018). For hosts that acquire their photosymbionts from the environment, the timing of the acquisition is crucial for survivorship in the wild. If we project the results for C. xamachana on coral, which is increasingly at risk of bleaching due to climate change, the timing of a few weeks between symbiont introduction and asexual reproduction may influence the survivorship of an animal. Understanding how plastic symbiont acquisition and host development is in a marine host aids predictions of changing ecosystems. The factors involved in the successful establishment of symbiosis with a cnidarian marine host are still unclear, but this study elucidates how the process of establishing symbiosis is highly stable if the host and symbionts are compatible yet how flexible it is in early ontogenetic stages. Other systems that appear to have high host–symbiont specificity in adults will need to be studied to examine patterns of phenotypic plasticity during the establishment of developmental symbiosis. Considering how diverse the response to exposure of different symbiont species is in C. xamachana polyps, it is clear that the early establishment of marine host–symbiont relationships is a complex process. Molecular approaches that examine symbiosis-driven development will shed light on the underlying mechanisms of such vast developmental phenotypic plasticity in marine holobionts.
The original contributions presented in the study are included in the article/Supplementary Material. Further inquiries can be directed to the corresponding author.
The manuscript presents research on animals that do not require ethical approval for their study.
VS: Conceptualization, Data curation, Formal analysis, Investigation, Methodology, Project administration, Validation, Visualization, Writing – original draft. AK: Methodology, Writing – review & editing. MMa: Investigation, Methodology, Writing – review & editing. VA: Methodology, Writing – review & editing. KT: Data curation, Resources, Writing – review & editing. AO: Visualization, Writing – review & editing. TL: Conceptualization, Methodology, Resources, Supervision, Writing – review & editing. MMe: Funding acquisition, Resources, Writing – review & editing.
The author(s) declare financial support was received for the research, authorship, and/or publication of this article. MMe was funded by NSF OCE 1442206. VS was funded by the Alfred P. Sloan foundation.
We thank the participants of the International Cassiopea Workshop for their invaluable feedback on this project as it developed. We would also like to thank Dr. Isaac Wright for his help with statistics questions. MaM thanks the European Marine Research Network for the Individual Fellowship Grant. MMe was funded by NSF IOS 2227068.
The authors declare that the research was conducted in the absence of any commercial or financial relationships that could be construed as a potential conflict of interest.
All claims expressed in this article are solely those of the authors and do not necessarily represent those of their affiliated organizations, or those of the publisher, the editors and the reviewers. Any product that may be evaluated in this article, or claim that may be made by its manufacturer, is not guaranteed or endorsed by the publisher.
The Supplementary Material for this article can be found online at: https://www.frontiersin.org/articles/10.3389/fevo.2024.1333028/full#supplementary-material
Supplementary Figure 1 | Days to strobilation of each strain (y-axis) divided by trial 1 on top, trial 2 in the middle, and trial 3 on the bottom. Diamond points are the average number of days to strobilate for that strain within the trial. Square points represent individual polyps. The orange line indicates where trial 2 stopped, to illustrate how certain strains strobilated when given more time in trial 3.
Supplementary Figure 2 | LSU sequence alignments of the 5 species that had multiple strains. Alignments were done in Geneious.
Abrego D., Oppen M. J. H. V., Willis B. L. (2009). Onset of algal endosymbiont specificity varies among closely related species of Acropora corals during early ontogeny. Mol. Ecol. 18, 3532–3543. doi: 10.1111/j.1365-294X.2009.04276.x
Baird A. H., Yakoleva I. M., Harii S., Sinniger F., Hidaka M. (2021). Environmental constraints on the mode of symbiont transmission in corals. J. Exp. Mar. Biol. Ecol. 538, 151499. doi: 10.1016/j.jembe.2020.151499
Biquand E., Okubo N., Aihara Y., Rolland V., Hayward D. C., Hatta M., et al. (2017). Acceptable symbiont cell size differs among cnidarian species and may limit symbiont diversity. ISME J. 11, 1702–1712. doi: 10.1038/ismej.2017.17
Blank R. J. (1987). Cell architecture of the dinoflagellate Symbiodinium sp. inhabiting the Hawaiian stony coral Montipora verrucosa. Mar. Biol. 94, 143–155. doi: 10.1007/BF00392906
Cabrales-Arellano P., Islas-Flores T., Thomé P. E., Villanueva M. A. (2017). Indomethacin reproducibly induces metamorphosis in Cassiopea xamachana scyphistomae. PeerJ 5, e2979. doi: 10.7717/peerj.2979
Cavalcanti G. S., Alker A. T., Delherbe N., Malter K. E., Shikuma N. J. (2020). The influence of bacteria on animal metamorphosis. Annu. Rev. Microbiol. 74, 137–158. doi: 10.1146/annurev-micro-011320-012753
Coffroth M. A., Santos S. R., Goulet T. L. (2001). Early ontogenetic expression of specificity in a cnidarian-algal symbiosis. Mar. Ecol. Prog. Ser. 222, 85–96. doi: 10.3354/meps222085
Fransolet D., Roberty S., Plumier J. C. (2012). Establishment of endosymbiosis: The case of cnidarians and Symbiodinium. J. Exp. Mar. Biol. Ecol. 420–421, 1–7. doi: 10.1016/j.jembe.2012.03.015
Freckelton M. L., Nedved B. T., Hadfield M. G. (2017). Induction of invertebrate larval settlement; different bacteria, different mechanisms? Sci. Rep. 7, 42557. doi: 10.1038/srep42557
González-Pech R.A., Li V. Y., Garcia V., Boville E., Mammone M., Kitano H., et al. (2024). The Evolution, Assembly, and Dynamics of Marine Holobionts. Ann Rev Mar Sci. 16 (1), 443–466. doi: 10.1146/annurev-marine-022123-104345
Harii S., Yasuda N., Rodriguez-Lanetty M., Irie T., Hidaka M. (2009). Onset of symbiosis and distribution patterns of symbiotic dinoflagellates in the larvae of scleractinian corals. Mar. Biol. 156, 1203–1212. doi: 10.1007/s00227-009-1162-9
Hofmann D. K., Brandt U. (1987). Induction of metamorphosis in the symbiotic scyphozoan Cassiopea andromeda: role of marine bacteria and of biochemicals. Symbiosis 4, 99–116.
Hofmann D. K., Fitt W. K., Fleck J. (1996). Checkpoints in the life-cycle of Cassiopea spp.: Control of metagenesis and metamorphosis in a tropical jellyfish. Int. J. Dev. Biol. 40, 331–338. doi: 10.1387/ijdb.8735945
Hofmann D. K., Neumann R., Henne K. (1978). Strobilation, budding and initiation of scyphistoma morphogenesis in the rhizostome cassiopea andromeda (Cnidaria: scyphozoa). Mar. Biol. 47, 161–176. doi: 10.1007/BF00395637.
Jarms G., Morandini A. C. (2019). World atlas of jellyfish (Hamburg, Germany: Dölling und Galitz Verlag).
Jeong H. J., Lee S. Y., Kang N. S., Yoo Y. D., Lim A. S., Lee M. J., et al. (2014). Genetics and Morphology Characterize the Dinoflagellate Symbiodinium voratum, n. sp., (Dinophyceae) as the Sole Representative of Symbiodinium Clade E. J. Eukaryotic Microbiol. 61, 75–94. doi: 10.1111/jeu.12088
LaJeunesse T. C. (2001). Investigating the biodiversity, ecology, and phylogeny of endosymbiotic dinoflagellates in the genus symbiodinium using the its region: in search of a “Species” Level marker. J. Phycology 37, 866–880. doi: 10.1046/j.1529-8817.2001.01031.x
LaJeunesse T. C. (2017). Validation and description of Symbiodinium microadriaticum, the type species of Symbiodinium (Dinophyta). J. Phycology 53, 1109–1114. doi: 10.1111/jpy.12570
LaJeunesse T. C., Parkinson J. E., Gabrielson P. W., Jeong H. J., Reimer J. D., Voolstra C. R., et al. (2018). Systematic revision of symbiodiniaceae highlights the antiquity and diversity of coral endosymbionts. Curr. Biol. 28, 2570–2580. doi: 10.1016/j.cub.2018.07.008
Lampert K. P. (2016). “Cassiopea and its zooxanthellae,” in The Cnidaria, Past, Present and Future: The world of Medusa and her sisters. Eds. Goffredo S., Dubinsky Z. (New York, USA: Springer International Publishing). doi: 10.1007/978-3-319-31305-4
Lee S. Y., Jeong H. J., Kang N. S., Jang T. Y., Jang S. H., LaJeunesse T. C. (2015). Symbiodinium tridacnidorum sp. nov., a dinoflagellate common to Indo-Pacific giant clams, and a revised morphological description of Symbiodinium microadriaticum Freudenthal, emended Trench & Blank. Eur. J. Phycology 50, 155–172. doi: 10.1080/09670262.2015.1018336
Mammone M., Ferrier-Pagés C., Lavorano S., Rizzo L., Piraino S., Rossi S., et al. (2021). High photosynthetic plasticity may reinforce invasiveness of upside-down zooxanthellate jellyfish in Mediterranean coastal waters. PloS One 16, e0248814. doi: 10.1371/journal.pone.0248814
Mammone M., Sharp V., Hewitt M., Medina M.. (2023). The influence of photosymbiosis in Cassiopea xamachana regenerative success. Symbiosis 90, 61–70. doi: 10.1007/s13199-023-00920-0
Marques A. C., Collins A. G. (2004). Cladistic analysis of Medusozoa and cnidarian evolution. Invertebrate Biol. 123, 23–42. doi: 10.1111/j.1744-7410.2004.tb00139.x
McAnulty S. J., Kerwin A. H., Koch E., Nuttall B., Suria A. M., Collins A. J., et al. (2023). Failure to launch”: development of a reproductive organ linked to symbiotic bacteria. mBio 14, e02131–e02122. doi: 10.1128/mbio.02131-22
McFall-Ngai M. J., Hadfield M. G., Bosch T. C. G., Carey H. V., Domazet-Loso T., Dougles A. E., et al. (2013). Animals in a bacterial world, a new imperative for the life sciences. Proc. Natl Acad Sci. U S A. 110 (9), 3229–36. doi: 10.1073/pnas.1218525110
McIlroy S. E., Cunning R., Baker A. C., Coffroth M. A. (2019). Competition and succession among coral endosymbionts. Ecol. Evol. 9, 12767–12778. doi: 10.1002/ece3.5749
Medina M., Sharp V., Ohdera A. H., Bellantuono A., Dalrymple J., Gamero-Mora E., et al. (2021). “The upside-down jellyfish cassiopea xamachana as an emerging model system to study cnidarian–algal symbiosis,” in Handbook of Marine Model Organisms in Experimental Biology (Florida, USA: CRC Press).
Mellas R. E., McIlroy S. E., Fitt W. K., Coffroth M. A. (2014). Variation in symbiont uptake in the early ontogeny of the upside-down jellyfish, Cassiopea spp. J. Exp. Mar. Biol. Ecol. 459, 38–44. doi: 10.1016/j.jembe.2014.04.026
Newkirk C. R., Frazer T. K., Martindale M. Q., Schnitzler C. E. (2020). Adaptation to bleaching: are thermotolerant symbiodiniaceae strains more successful than other strains under elevated temperatures in a model symbiotic cnidarian? Front. Microbiol. 11. doi: 10.3389/fmicb.2020.00822
Ohdera A. H., Abrams M. J., Ames C. L., Baker D. M., Suescún-Bolívar L. P., Collins A. G., et al. (2018). Upside-down but headed in the right direction: Review of the highly versatile Cassiopea xamachana system. Front. Ecol. Evol. 6. doi: 10.3389/fevo.2018.00035
Parkinson J. E., Coffroth M. A., LaJeunesse T. C. (2015). New species of Clade B Symbiodinium (Dinophyceae) from the greater Caribbean belong to different functional guilds: S. aenigmaticum sp. nov., S. antillogorgium sp. nov., S. endomadracis sp. nov. and S. pseudominutum sp. nov. J. Phycology 51, 850–858. doi: 10.1111/jpy.12340
Pochon X., LaJeunesse T. C. (2021). Miliolidium n. gen, a New Symbiodiniacean Genus Whose Members Associate with Soritid Foraminifera or Are Free-Living. J. Eukaryotic Microbiol. 68, e12856. doi: 10.1111/jeu.12856
Prieto L., Astorga D., Navarro G., Ruiz J. (2010). Environmental control of phase transition and polyp survival of a massive-outbreaker jellyfish. PloS One 5, e13793. doi: 10.1371/journal.pone.0013793
R Core Team. (2018). R: A language and environment for statistical computing. R Foundation for Statistical Computing. Available at: https://www.R-project.org/.
Rahat M., Reich V. (1986). Algal endosymbiosis in brown hydra: host/symbiont specificity. J. Cell Sci. 86, 273–286. doi: 10.1242/jcs.86.1.273.
Schneider C. A., Rasband W. S., Eliceiri K. W. (2012). NIH Image to ImageJ: 25 years of image analysis. Nat. Methods 9, 671–675. doi: 10.1038/nmeth.2089
Searle S. R., Speed F. M., Milliken G. A. (1980). Population marginal means in the linear model: an alternative to least squares means. Am. Statistician 34, 216–221. doi: 10.1080/00031305.1980.10483031
Tang Y., Horikoshi M., Li W. (2016). ggfortify: unified interface to visualize statistical result of popular R packages. The R Journal 8, 474–485. doi: 10.32614/RJ-2016-060
Thornhill D. J., Daniel M. W., LaJeunesse T. C., Schmidt G. W., Fitt W. K. (2006). Natural infections of aposymbiotic Cassiopea xamachana scyphistomae from environmental pools of Symbiodinium. J. Exp. Mar. Biol. Ecol. 338, 50–56. doi: 10.1016/j.jembe.2006.06.032.
Treible L. M., Condon R. H. (2019). Temperature-driven asexual reproduction and strobilation in three scyphozoan jellyfish polyps. J. Exp. Mar. Biol. Ecol. 520, 151204. doi: 10.1016/j.jembe.2019.151204
Wickham H. (2016). ggplot2: Elegant Graphics for Data Analysis eBook (New York: Springer-Verlag). doi: 10.1007/978-3-319-24277-4.
Wickham H., Francois R., Muller K. (2022)dplyr: A grammar of data manipulation. Available online at: https://dplyr.tidyverse.org/ (Accessed 1 December 2022).
Keywords: Symbiodiniaceae, symbiosis, development, cnidarian, Cassiopea, strobilation, holobiont
Citation: Sharp V, Kerwin AH, Mammone M, Avila-Magana V, Turnham K, Ohdera A, LaJeunesse T and Medina M (2024) Host–symbiont plasticity in the upside-down jellyfish Cassiopea xamachana: strobilation across symbiont genera. Front. Ecol. Evol. 12:1333028. doi: 10.3389/fevo.2024.1333028
Received: 04 November 2023; Accepted: 06 February 2024;
Published: 14 March 2024.
Edited by:
William Fitt, University of Georgia, United StatesReviewed by:
Elizabeth Heath-Heckman, Michigan State University, United StatesCopyright © 2024 Sharp, Kerwin, Mammone, Avila-Magana, Turnham, Ohdera, LaJeunesse and Medina. This is an open-access article distributed under the terms of the Creative Commons Attribution License (CC BY). The use, distribution or reproduction in other forums is permitted, provided the original author(s) and the copyright owner(s) are credited and that the original publication in this journal is cited, in accordance with accepted academic practice. No use, distribution or reproduction is permitted which does not comply with these terms.
*Correspondence: Mónica Medina, bXVtNTVAcHN1LmVkdQ==
Disclaimer: All claims expressed in this article are solely those of the authors and do not necessarily represent those of their affiliated organizations, or those of the publisher, the editors and the reviewers. Any product that may be evaluated in this article or claim that may be made by its manufacturer is not guaranteed or endorsed by the publisher.
Research integrity at Frontiers
Learn more about the work of our research integrity team to safeguard the quality of each article we publish.