- 1Department of Microbiology, Miami University, Oxford, OH, United States
- 2Biology Department, University of New Mexico, Albuquerque, NM, United States
High-latitude meromictic lakes such as those in the Antarctic McMurdo Dry Valleys (MDV) harbor aquatic ecosystems dominated by the microbial loop. Within this habitat, which is limited year-round by light and nutrients, protists, or single celled eukaryotes, play outsized roles in the food web as the dominant primary producers and the apex predators. Thus, the MDV lake ecosystem represents an ideal system to study the role of sentinel protist taxa in carbon and nutrient cycling. The perennially ice-covered lakes are part of the McMurdo Long Term Ecological Research (McM LTER; mcmlter.org) established in 1993. In this review we will highlight the diversity and trophic roles of the MDV lake protist community and compare environmental factors driving spatiotemporal patterns in key protist taxa in two lakes within the McM LTER, Lakes Bonney and Fryxell. We will then discuss lessons learned from manipulated experiments on the impact of current and future climate-driven environmental change on sensitive protist taxa. Last, we will integrate knowledge gained from 25 years of lab-controlled experiments on key photosynthetic protists to extend our understanding of the function of these extremophiles within the MDV aquatic food webs. Our research group has studied the distribution and function of the MDV microbial community for nearly two decades, training the next generation of scientists to tackle future problems of these globally significant microbes. This review article will also highlight early career scientists who have contributed to this body of work and represent the future of scientific understanding in the Anthropocene.
Introduction
Protists are a morphologically and taxonomically diverse group of single-celled lifeforms which dominate diversity within the eukaryotic lineage (Keeling and Burki, 2019; Burki et al., 2021). Protists play key functional roles in carbon and nutrient cycling in all ecosystems, in particular freshwater and marine food webs (Caron et al., 2012). Often dominating the primary producer community of aquatic ecosystems (Falkowski et al., 1998; Singer et al., 2021), photosynthetic protists (i.e. eukaryotic algae) fix inorganic carbon for the rest of the aquatic food chain (Carpenter et al., 1991; Field et al., 1998). While often under-described relative to their photosynthetic counterparts, heterotrophic protists appear to be extremely diverse and abundant (Seeleuthner et al., 2018). Predatory protists consume significant quantities of phytoplankton and bacteria, exerting top-down control over their prey populations (Leander, 2020; Laundon et al., 2021), as well as linking carbon fixed within the microbial loop with higher trophic levels (Azam and Malfatti, 2007). The trophic modes of heterotrophic protists are diverse and include predation, symbiosis, parasitism, and saprotrophy (De Vargas et al., 2015; Lima-Mendez et al., 2015; Seeleuthner et al., 2018). Mixotrophic protists are ubiquitous and have complex influences on carbon and nutrient cycling, owing to their ability for combined phototrophic and heterotrophic metabolism (Sanders, 1991; Stoecker et al., 2017). Given the critical roles of these microorganisms, it is important to understand their distribution and function across global aquatic ecosystems. In recent years, the immense taxonomic diversity of protists has been recognized (Santoferrara et al., 2020), making them significant in understanding the eukaryote tree of life (Keeling et al., 2005). The ecological function of some key protists has been characterized, while many others are unknown. Further, the essential ecological roles of protists are likely to shift under anthropogenic climate change conditions. Improving our predictive power of these functional shifts in the Anthropocene epoch would contribute to disentangling the complex ecological response of microbial communities to climate-related environmental disturbances.
High-latitude meromictic lakes such as those in the Antarctic McMurdo Dry Valleys harbor ecosystems dominated by the microbial loop. Within these truncated food webs, protists occupy prominent roles at all trophic levels. Photosynthetic protists dominate the photic zone and represent the major contributors of autochthonous dissolved organic carbon (DOC) to these closed aquatic ecosystems (Priscu et al., 1999; Dolhi et al., 2015). Heterotrophic protists predate on either bacteria or smaller phytoplankton and occupy the top of the food chain. Mixotrophic metabolism appears to be very prevalent and advantageous in this extreme environment: constitutive mixotrophic algae (e.g. cryptophytes) contribute to both DOC production and control of bacterial prey (Laybourn-Parry et al., 2000; Laybourn-Parry, 2009; Kong et al., 2014), while larger nonconstitutive mixotrophic ciliates are important top-down regulators of key phytoplankton (Roberts and Laybourn-Parry, 1999). The distribution, diversity, and trophic activity of protist communities within and between the water columns of the Dry Valley lakes is highly variable and is influenced by abiotic drivers such as light and nutrient availability (Bielewicz et al., 2011; Dolhi et al., 2015; Li and Morgan-Kiss, 2019; Li et al., 2019), as well as other stressors, including salinity gradients (Rojas-Jimenez et al., 2017). The Dry Valley lakes, situated in the largest ice-free expanse of Antarctica, have historically been ice-covered and fed by ephemeral streams (Gooseff et al., 2022). This cold desert environment, and the life that inhabits it, is subject to changes in solar radiation and temperature.
Beginning in the austral summer of 2011, our research group initiated an intensive focus on how major biogeochemical and physical parameters influence the vertical distribution of protists. Additionally, the sensitivity of key protists to climate change has also been probed by the application of bioassays and in situ incubations. Last, the Lake Bonney green alga, Chlamydomonas priscuii (formerly, Chlamydomonas sp. UWO241), represents a an important non-model species for cold adaptation of photosynthetic processes (reviewed recently in: (Cvetkovska et al., 2022; Hüner et al., 2022). Here we present a synthesis of field studies, in situ manipulation experiments and lab-controlled experiments representing three decades of “Team Protist” working on native communities and phytoplankton isolates from the McMurdo Dry Valley lakes.
McMurdo Dry Valley lakes
The McMurdo Dry Valley lakes are part of a network of hundreds of permanently ice-covered lakes and ponds located throughout the valleys in the Transantarctic Mountains, and more specifically within the Taylor Valley (Figure 1). Ice-covers overlay water columns which remain liquid throughout the year and exhibit minimal gas exchange, permanent chemical stratification, and severely limited light availability (Howard-Williams et al., 1998; Fritsen and Priscu, 1999). The MDV lakes represent one of the last sites where perennially ice-covered lakes still exist. Ice-covered lakes in other high latitude ecosystems (e.g. the high Arctic) have become seasonally ice-free during the summer in recent years, disrupting the permanent meromixis (Walsh, 2009). Thus, the MDV lakes as well as other permanent ice-covered Antarctic lakes in areas such as the Vestfold Hills in Princess Elizabeth Land (Figure 1, inset) (Gibson and Burton, 1996; Laybourn-Parry and Bell, 2014), are some of the last representative “natural laboratories” for studying microbial communities isolated in stratified water columns under permanent ice (Gooseff et al., 2017b).
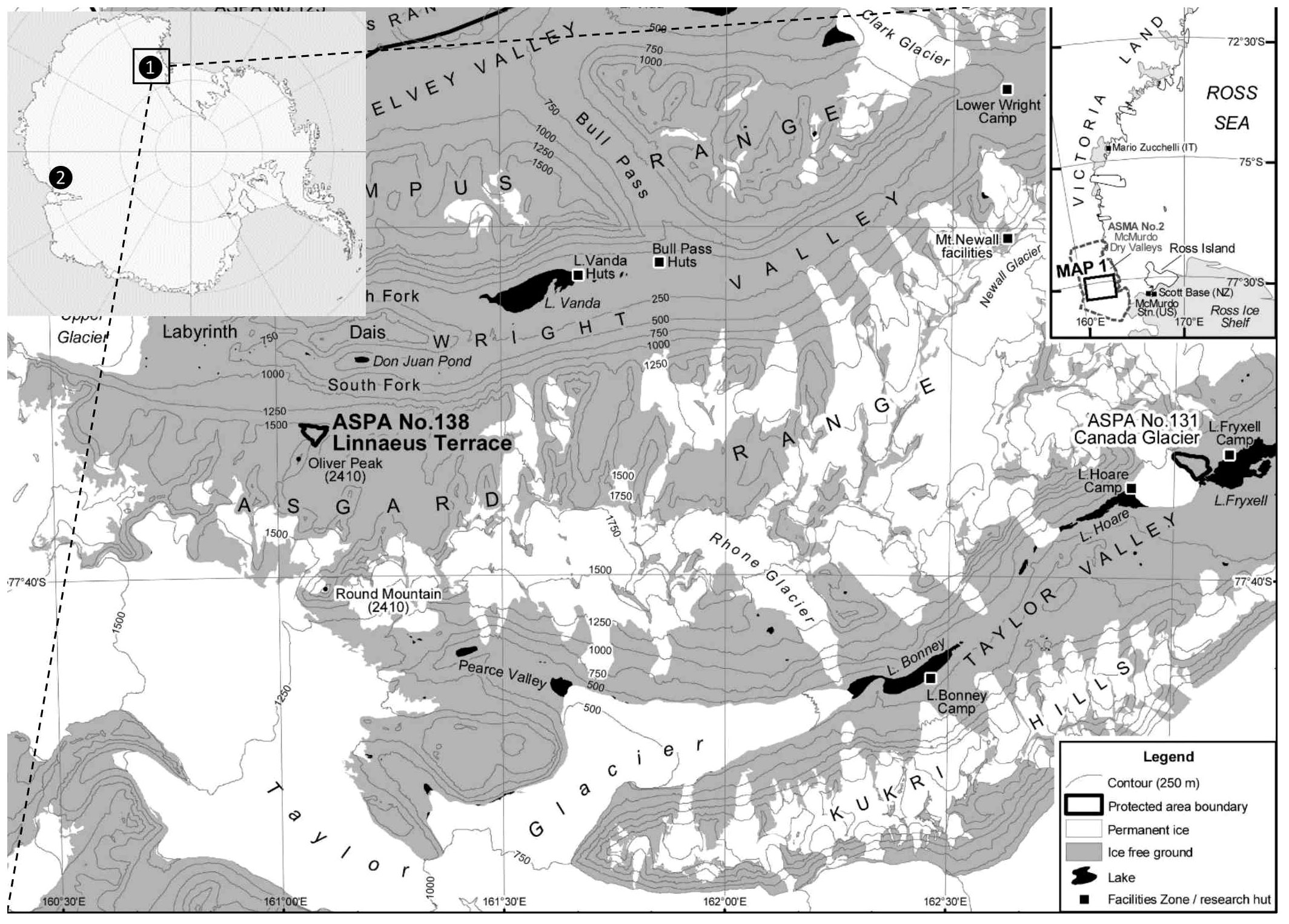
Figure 1 Map of the Taylor and Wright Valleys, Antarctica. Inset: location of the McMurdo Dry Valleys (Victoria Land, site 1) and the Vestfold Hills (Princess Elizabeth Land, site 2); both sites of long-term studies on protist communities residing in meromictic water columns under permanent ice covers. Image was edited from a map from the Antarctic Geospatial Information Center (http://www.agic.umn.edu/maps/Antarctic).
The MDV lake water columns represent a refuge from the harsh conditions of a polar desert for food webs dominated by the microbial loop. Several lakes, including Lakes Fryxell, Bonney, Hoare, Vanda and Joyce have been intensively studied since the early 1990s by the McMurdo Long Term Ecological Program (MCM LTER). The MCM LTER long-term data set (mcmlter.org) represents an invaluable record of climate change in a highly sensitive ecosystem. Recent work on the protist communities has focused on Lakes Fryxell and Bonney, which exhibit distinctions in physicochemistry (Lyons et al., 2000), driving difference in the structure of the microbial communities (Kwon et al., 2017; Li and Morgan-Kiss, 2019).
Lake Fryxell is a brackish, relatively shallow body of water (20 m maximum depth) near the marine influence of the Ross Sea. Light levels in the austral summer are extremely low (PAR < 10 µmol m-2 s-1). Lake Fryxell is fed by numerous glacial streams, providing increased nutrients and organic matter which flow into a wide perimeter moat during the summer months. Higher nutrient levels, especially phosphorus, support a mesotrophic ecology, some of the highest detectable levels of phytoplankton and bacterial biomass of all studied MDV lakes (Priscu, 1995). Microbial productivity peaks within the permanent chemocline, at 9 m.
Lake Bonney is separated into two 40-m deep lobes (west and east; WLB and ELB, respectively) by a 12-m deep bedrock sill which allows exchange of surface waters for a few weeks in the short summer. Above the permanent chemoclines, the water columns are characterized by low light (<10% surface radiation levels), oxygen-saturation, oligotrophy, and low productivity. Both lobes exhibit deep chlorophyll-a maxima near the chemoclines (13 – 15 m). The deep, saline waters of the two basins are ancient (~3000 yrs) and cold (-2°C to -4°C) (Poreda et al., 2004). Mixing times of the water columns are approximately every 50,000 years (Spigel and Priscu, 1996): microbial communities are vertically stratified through the water column and are adapted to the local physical and chemical parameters.
Continental Antarctica harbors numerous inland lakes, many of which share common physicochemical characteristics with the MDV lakes, such as meromixis, extreme shade conditions, and oligotrophy (Green and Lyons, 2009). For example, perennially ice-covered lakes in the Vestfold Hills are a second long-term research site on the ecology of Antarctic lake microbial communities (Figure 1, inset). Numerous permanent stratified bodies of water have been recorded within the Vestfold Hills region; although, only a handful have been sampled (Gibson, 1999). Ace Lake is one of the most intensively studied systems in this region (Laybourn-Parry and Bell, 2014). It is saline and meromictic, and in common with the MDV lakes, harbors a truncated microbial food web of bacteria and protist within the upper aerobic photic zone (Laybourn-Parry and Bell, 2014). In contrast with the isolated lake systems within the Antarctic continent, lakes within the maritime Antarctic region typically experience ice-free summers and higher nutrient status, owing to the proximity to populations of sea animals and birds (Hawes, 1990; Laybourn-Parry et al., 1996). Thus, the permanently ice-covered lakes of the McMurdo Dry Valleys and Vestfold Hills are end members for studying isolated microbial communities which have experienced minimal external impacts. In contrast, the lakes in the maritime and sub-continental regions are closer analogues to other global freshwater systems which experience seasonal ice loss and significant allochthonous inputs.
Trophic function, diversity, identification of key dry valley lake protists
Protists play key trophic roles in the MDV food webs as the main primary producers and the top predators. Several recent studies have focused on environmental DNA sequencing of ribosomal and functional genes to describe the diversity and distribution of MDV protist communities (Bielewicz et al., 2011; Vick-Majors et al., 2014; Dolhi et al., 2015; Li and Morgan-Kiss, 2019). Microbial eukaryotes are key producers and consumers of organic matter within the MDV food web (Figure 2). Obligate photoautotrophic as well as constitutive mixotrophic algae produce DOC for heterotrophic bacteria (Figure 2, Boxes 1, 4). A variety of heterotrophic protists predate on bacteria and algae (Figure 2, Boxes 2, 5). In Lake Bonney, pure photoautotrophic chlorophytes (eg: Chlamydomonas sp) contribute significantly to fixing new carbon for the aquatic food web, while mixotrophic populations (Geminigera, Isochrysis sp.) combine photosynthesis and predation and are a potential source of biogenic sulfur compounds, such as dimethylsulfoniopropionate (DMSP) (Lee et al., 2004; Dolhi et al., 2015). A diverse group of small heterotrophic protists graze on bacteria [Figure 2, Box 5; (Li and Morgan-Kiss, 2019)], while a parasitic Stramenopile (Personia) is a predator of Chlamydomonas (Figure 2, Box 3; (Li et al., 2016). Smaller phytoplankton are prey for ciliates which are typically the tertiary predators in these truncated food webs (Figure 2, Box 2).
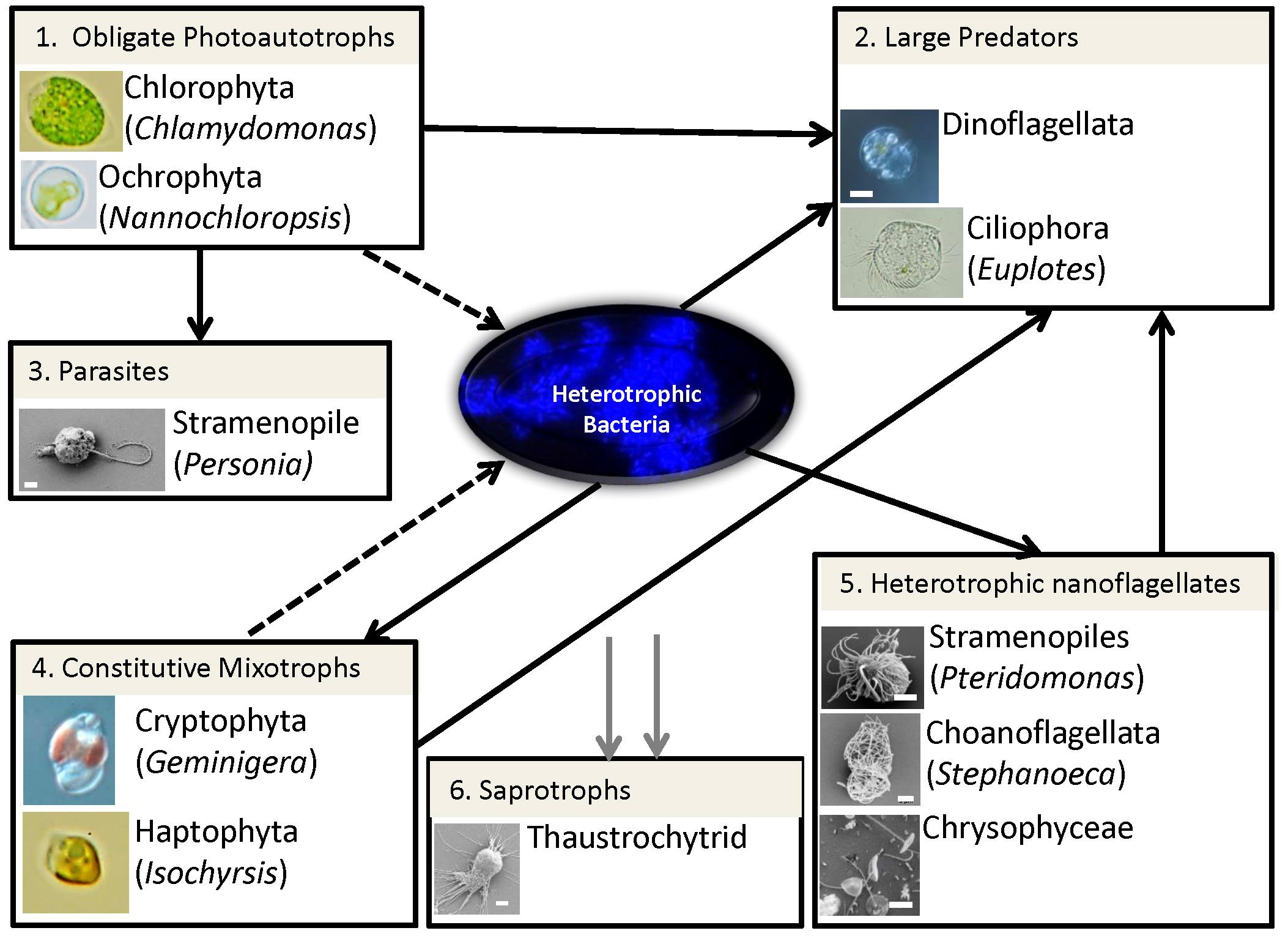
Figure 2 Major trophic roles and microbial interactions of protists in the MDV lake food web. Lines represent the direction of the flow of carbon and/or nutrients: dotted lines, dissolved organic carbon from primary production; solid lines, heterotrophic metabolism (black, predation; grey, saprotrophy). Boxes represent major functional groups with images of examples for each group. Boxes: 1, Obligate photoautotrophic protists fix CO2 and produce DOC which is taken up by heterotrophic bacteria; 2, Large predators represent the top predators of the food web, consuming phytoplankton, flagellates, bacteria and other ciliates; 3, Parasitic nanoflagellates predate upon algal prey; 4, Constitutive mixotrophs contribute to both primary production and consumption of bacterial prey; 5, Heterotrophic nanoflagellates are small predators of bacteria; 6, A poorly characterized saprophytic population of heterotrophic protists, fungi and bacteria mineralizes dead biomass.
We complemented phylogenetic information from past molecular surveys with a morphological description of key MDV protists residing in Lake Bonney (Figure 3). Large, biflagellate chlorophytes are abundant taxa (Bielewicz et al., 2011): our survey identified several members of Chlorophyta, including multiple Chlamydomonas spp. (Figure 3, plates 1-3), Chlorella (Figure 3, plates 4-5), and a lobed cell related to Brachiomonas (Figure 3, plates 6-7). The shallow layers of Lake Bonney also harbor a cryptophyte related to Geminigera cryophila, a marine cryptophyte. This organism is the dominant phytoplankton in Lake Fryxell (Li and Morgan-Kiss, 2019), and is a major prey species for ciliates (Laybourn-Parry et al., 1997). Both Lakes Bonney and Fryxell cryptophyte populations are closely related to a single species, G. cryophila, while the chlorophyte populations are lake-specific. A single haptophyte species related Isochrysis is the third most abundance photosynthetic protist in Lake Bonney (Bielewicz et al., 2011) (Figure 3, plates 11-13). A number of heterotrophic protists reside in the water column. In oligotrophic Lake Bonney, smaller predators, including choanoflagellates and dinoflagellates, are dominant members of the predatory protist populations (Li and Morgan-Kiss, 2019) while large ciliates make up a larger proportion of the predators in the more productive Lake Fryxell (Roberts and Laybourn-Parry, 1999). Both lakes harbor a community of diverse community of Ochrophyta, including a parasitic heterotrophic nanoflagellates related to Personia which preys upon Chlamydmonas cells (Figure 3, plates 19-21) (Li et al., 2016; Li and Morgan-Kiss, 2019). The combined morphological and environmental sequencing survey provides an overall view of a diverse protist population, performing key functional roles in the MDV aquatic ecosystem. Distribution and metabolism of protist communities is significantly influenced by strong gradients in major physicochemical factors, including light, salinity and macronutrients (Li and Morgan-Kiss, 2019; Li et al., 2019).
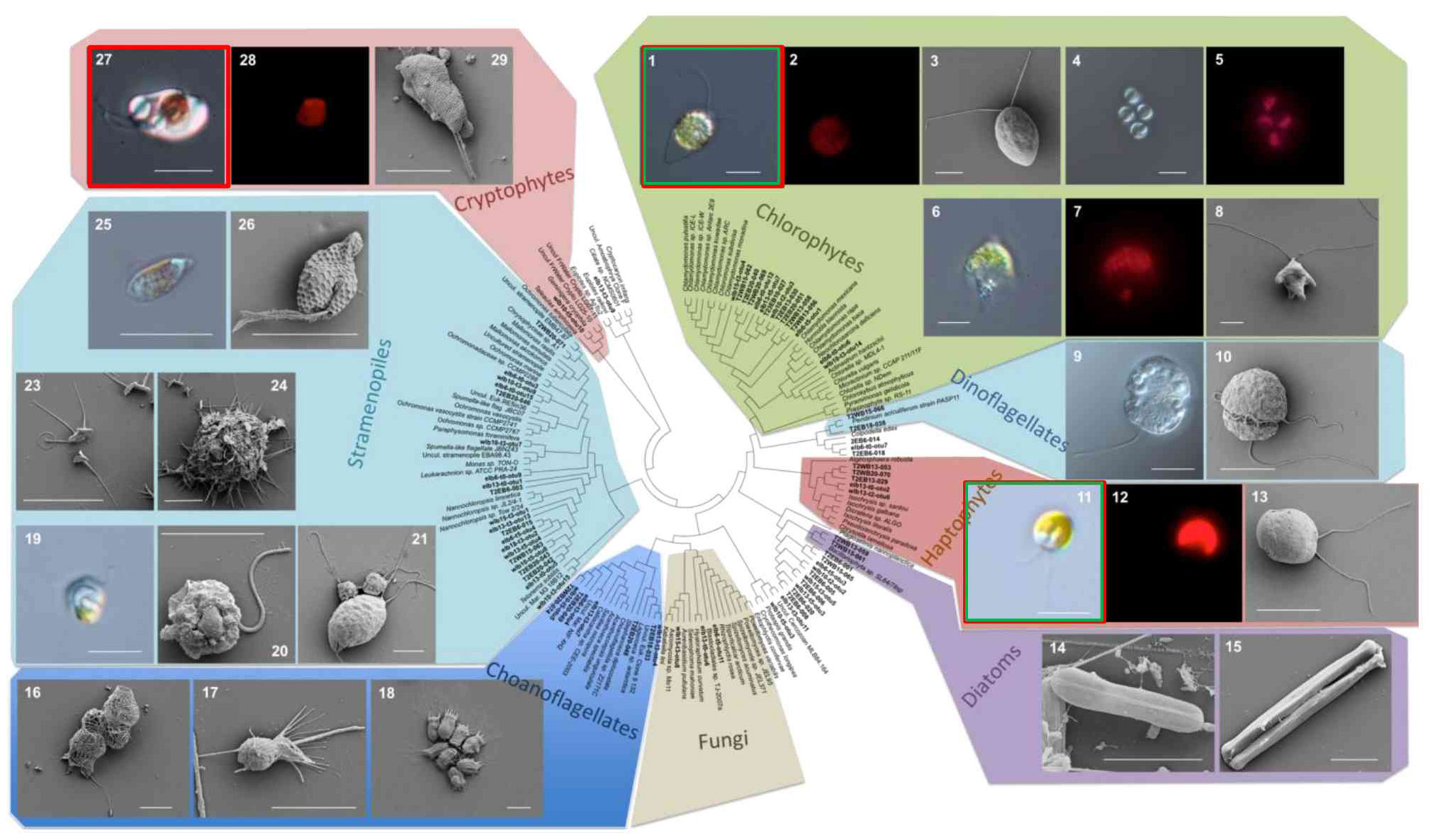
Figure 3 Micrographs of a selection of photosynthetic (Plates 1-15, 24-28) and heterotrophic (Plates 16-23) protists are shown. Plate IDs: 1-8 Chlorophyta: 1-3, Chlamydomonas, 4-5; Chlorella; 6-8, Brachiomonas; red boxes – abundant protist taxa in MDV lakes; green boxes – MDV protist taxa available as isolates. Figure is based on data from: (Bielewicz et al., 2011; Li and Morgan-Kiss, 2019).
Spatiotemporal patterns in key MDV protist taxa
A variety of approaches have been applied to monitor spatial and temporal patterns of key MDV lake protist taxa. Early studies used clone sequence libraries to provide the first view of the vertical distribution patterns of protists in Lake Bonney. Bielewicz et al. (2011) reported under-ice communities living in shallow waters of ELB were dominated by a community of cryptophytes, while a haptophyte population related to Isochrysis resides within the permanent chemocline. A population of chlorophytes was detected in the deep photic zone. Recent applications of next generation sequencing confirmed these findings and identified additional key taxa, including choanoflagellates and numerous heterotrophic nanoflagellates (Li and Morgan-Kiss, 2019); Figure 4B). In Lake Fryxell, a cryptophyte community dominates the protist populations at all depths within the photic zone, while Chlorophytes are present at significantly lower abundance in Lake Fryxell compared to Lake Bonney (Li and Morgan-Kiss, 2019).
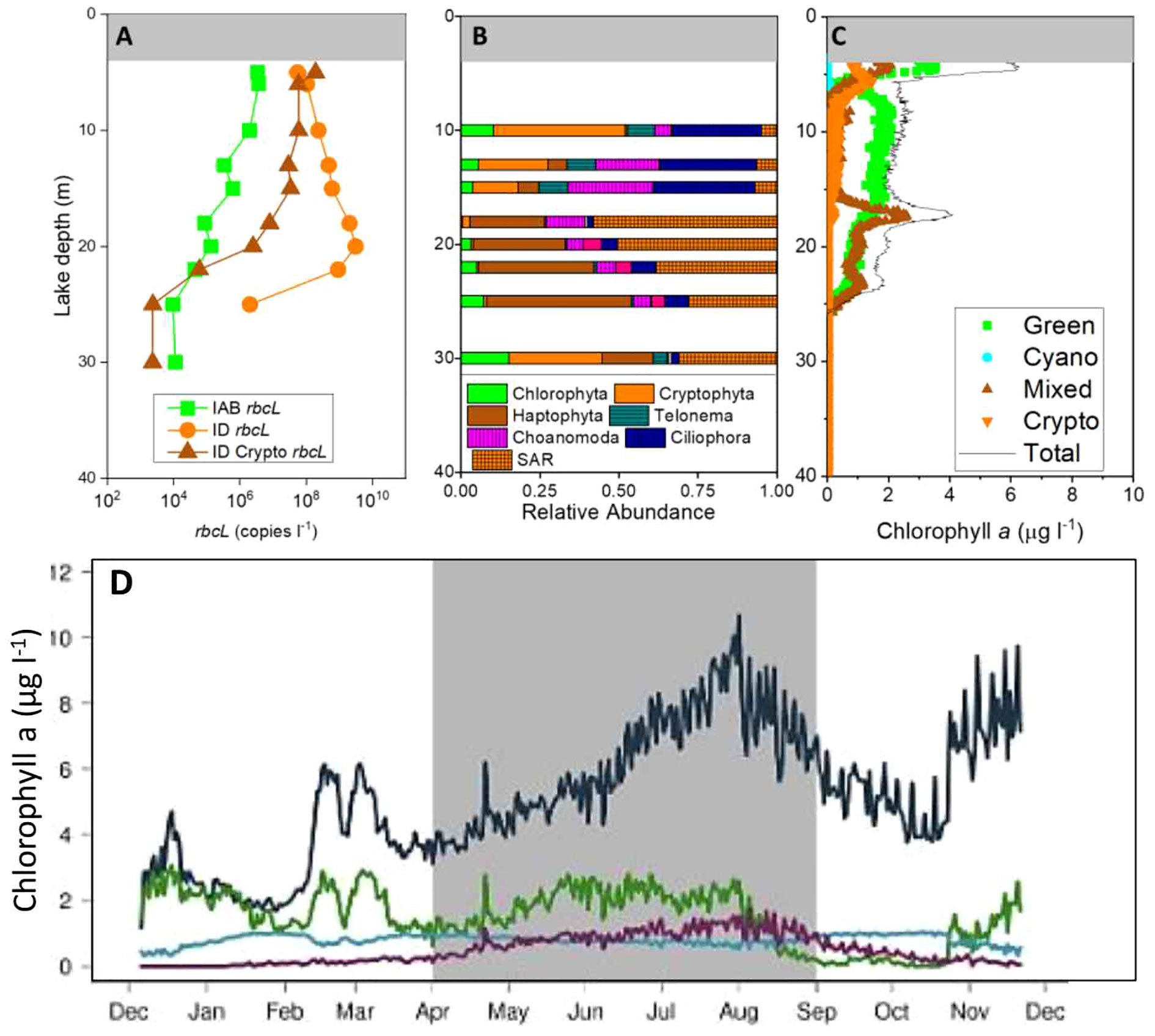
Figure 4 Spatial trends in Lake Bonney protists using different detection tools. (A). Group-targeted RubisCO rbcL qPCR, (B). Vertical trends in major eukaryal groups. (C, D). Spectral algal classes, chlorophyll a fluorescence, on spatial (C) and temporal (D) scales. Figure based on data from refs: (Dolhi et al., 2015; Li and Morgan-Kiss, 2019; Patriarche et al., 2021).
In addition to sequencing efforts, probes for several functional genes, particularly related to carbon fixation (rbcL, cbbM, nifJ) and photochemistry (psbB), have been used to describe both the spatial and temporal patterns of major primary producers in the MDV lakes. Using several primers sets specific for isoforms of the RubisCO large subunit (encoded by the gene, rbcL), Kong et al. (Kong et al., 2012a, b) confirmed that chlorophytes (harboring rbcL A/B) and Haptophytes (harboring rbcL ID) exhibit distinct spatial patterns in gene copy abundance (using environmental DNA; Figure 4A). Dolhi et al. (2015) also confirmed that cryptophyte rbcL is significantly more abundant in Lake Fryxell. Last, Kong and colleagues showed that temporal patterns in rbcL IA/B and ID gene copy during the transition from fall to winter were different (Kong et al., 2012b): rbcL IA/B expression exhibited a linear relationship with light availability, while levels of rbcL ID transcripts exhibited no significant trends with light.
Submersible instruments which measure chlorophyll a fluorescence provide real-time data on phytoplankton biomass. When deployed in well-defined aquatic environments such as the MDV lakes, the use of a spectral fluorometer reports additional information on relative abundance of spectral classes of phytoplankton, as defined by their diagnostic pigments. The bbe FluoroProbe has been deployed in the MDV lakes for more than a decade and has provided a long-term record of spatial variation in phytoplankton abundance. The FluoroProbe relies on differential excitation of major algal spectral class pigments to delineate between four major algal classes: chlorophytes, cryptophytes, cyanobacteria, and a ‘mixed’ group (Beutler et al., 2002). While there have been debates among the literature over the limitations and accuracy of spectral fluorometers (Kring et al., 2014), in the simple, defined systems of the dry valley lakes, the FluoroProbe has been a valuable tool to complement other long-term datasets (Li et al., 2019; Patriarche et al., 2021; Sherwell et al., 2022).
The bbe FluoroProbe has been deployed annually during the austral summer in the MDV lakes since 2005. Long-term trends in phytoplankton biomass, estimated as total chlorophyll a, indicate that phytoplankton levels have increased in Lake Bonney over the past two decades. This trend is particularly significant for carbon cycling in the MDV lakes as most of the organic carbon is autochthonous in origin, owing to minimal external inputs from glacial-fed streams. Patriarche et al. (2021) reported that the rise in phytoplankton biomass was due to a rising trend in Chlorophytes. While this paper did not verify the specific chlorophyte taxa, previous studies have documented that the chlorophyte community is dominated by the large biflagellate, Chlamydomonas (Bielewicz et al., 2011; Li and Morgan-Kiss, 2019).
More recently, the bbe FluoroProbe was deployed in Lake Bonney as part of a suite of instruments and samples to provide one of the first year-round views of seasonal succession in the algal communities (Patriarche et al., 2021). A surprising discovery was that the total chlorophyll a levels increased during early winter, owing to a rise in biomass of the mixed spectral group (Figure 4D). Previously, the mixotrophic haptophyte, Isochrysis sp., was identified as a major algal component of the mixed group (Dolhi et al., 2015). Thus, in addition to contributing to primary production during the summer, Isochrysis sp. is also active during the winter, mostly likely relying on predatory consumption of bacterial prey (Li et al., 2016).
Molecular surveys during recent years have significantly contributed to a fuller understanding of the dominant members of the MDV lake protist communities. Vertical stratification of the protist communities within the water column is a reflection of strong chemical gradients, redox transitions, and availability of solar light. While the functional role of some of the dominant taxa is understood, advances in understanding the activity and function of taxa such as the diverse group of heterotrophic nanoflagellates as well as the full diversity of saprotrophic protists.
Signs and impacts of the Anthropocene in the dry valleys
The Western Antarctic continent, in particular the northern Antarctic Peninsula has shown obvious signs and impacts of climate-related change. Since the 1950’s, upper ocean temperatures have risen almost 3°C (Turner et al., 2005) causing the collapse of ice shelves, resulting in major shifts in macrofauna life cycles and introduction of nonnative species. Although not fully understood, warm summers, including increases in the number of annual above freezing days, will have multiple positive and negative impacts on biology, as ecosystems on the Antarctic continent are restricted to ice-free areas comprising <0.5% of the continent (Brooks et al., 2019), and are largely colonized by low temperature-adapted microscopic lifeforms. The impact of discrete climate events, traditionally termed pulses, has been particularly notable in dry environments, such as the polar desert conditions of the MDVs.
The MDVs are located in the larger eastern region of Antarctica: in the past, East Antarctica has been largely spared from extreme weather events, in part due to ozone depletion over this region (Doran et al., 2002). In fact, conditions in the MDVs have been metastable for thousands of years (Green and Lyons, 2009); however, evidence of extreme weather events have appeared in the past three decades. Long-term data records from the McMurdo LTER have been critical in witnessing the stochastic shifts in major climate conditions in the MDVs (Figure 5). One of the most notable climate events was an unprecedented flooding from December 2001 to January 2002, caused by a discrete warming event which drove increased glacial melt and record stream discharge (Foreman et al., 2004). While this climate event was short, it caused both immediate and long-term shifts in the MDV landscape (Gooseff et al., 2017a). During the flood, high stream discharge caused increased water column turbidity, while increased nutrient loading occurred over the first year after the warm summer (Foreman et al., 2004).
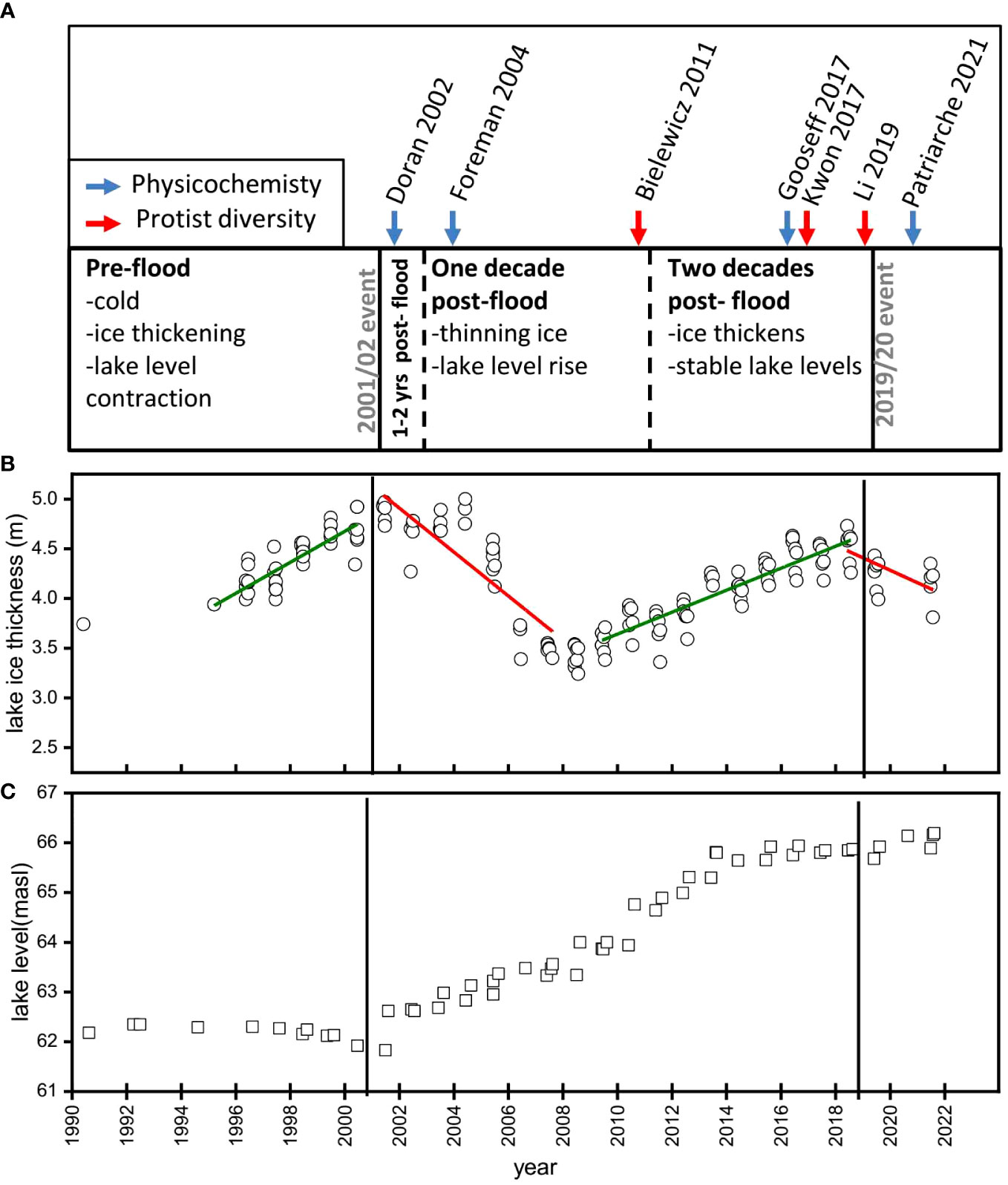
Figure 5 Impact of episodic climate events on McMurdo dry valley lakes. (A) Key papers documenting long-term trends in lake physicochemistry (blue arrows) and protist diversity (red arrows) in relation to two warm summer events occurring in 2001/02 and 2019/20. (B, C). Long-term physicochemistry from east lobe Lake Bonney. Figure is based on data from refs: (Doran and Gooseff, 2023; Priscu, 2023).
On longer time scales, lake levels which had been stable or declining for the previous decade, increased over the first decade after the flood (Figure 5B). Despite lake level rise, the position of the permanent chemocline has been stable relative to sea level in Lake Bonney, which suggests that the volume of the overlaying oligotrophic surface waters has increased (Patriarche et al., 2021). Indeed, the upper water column of Lake Bonney has become fresher since the warm summer, producing a larger freshwater habitat (Sherwell et al., 2022).
Permanent ice covers also thinned during the decade post-flood (-1.20 and -0.61 m per decade in ELB and WLB, respectively) (Gooseff et al., 2017a). Since 2009 the lake ice has thickened to pre-flood values and lake level rise has been paused since 2015 (Sherwell et al., 2022). It is notable that the ice thickness of ELB did not fully return to pre-flood levels (Figure 5B). Between 2001-2005, ice thickness reached a maximum of 4.79 ± 0.16 m; however, only reached a maximum of 4.42 ± 0.17 m between 2015-2019. Recently, an extreme event in the form of a summer heat wave was documented in Antarctica, with temperatures reaching a high of 20°C in February 2020 (Robinson et al., 2020). The two years of data collected since this recent warm summer suggest that the dry valley lakes may have been reset for another round of lake ice thinning and lake level rise (Figure 5).
Many of the studies on the impacts of extreme weather events on the biology in the MDV lakes have focused on response of the phytoplankton communities. Immediately following the 2001/02 flood, Foreman observed that photosynthesis in Lake Bonney was temporarily shut down due to turbidity-associated transient reductions in PAR; however, increased nutrient availability fueled a phytoplankton bloom the following year (Foreman et al., 2004). Over longer time scales, Patriarche and colleagues proposed that a decadal-long rise in chlorophyll a in ELB and WLB was largely due to a rise in green algal biomass (Chlorophytes) in the upper water column (Patriarche et al., 2021). In parallel with lake level rise, major chlorophyll a maxima shifted ~3 m deeper in the water column of ELB during the first decade (2003-2013) after the 2001/02 flood (Sherwell et al., 2022). Since 2013, the position of the phytoplankton biomass maxima has been stable and chlorophyll levels have declined (Sherwell et al., 2022), in agreement with the quiescent phase 2 decades post-flood (Figure 5).
The long-term data record of the MCM LTER provides a critical tool for understanding how climate has shaped the physicochemistry of the MDV lakes. On the other hand, the application of widespread use of environmental sequencing is relatively recent, e.g. next generation sequencing applications began more than a decade after the 2001/02 flood (Figure 5A). Thus, our current understanding of how protist populations responded to past climate events and predicting impacts of future climate related events is limited.
Responses of MDV protists to environmental disturbance
The sequence record for the MDV lakes is relatively recent, with the first reported 18S rRNA sequencing of the photic zone of Lake Bonney occurring in 2011 (Figure 5A; Bielewicz et al., 2011). As a proxy, disturbance experiments were conducted to mimic anticipated climate-related events on the MDV lake communities. These lab- and field-based experiments have addressed several climate-relevant impacts on the under-ice microbial communities, including changes in physciochemistry, ice thickness, hydrological connectivity, and lake level rise. In the earliest study, Priscu (1995) used a nutrient bioassay approach to mimic the impact of increased macronutrient input on phytoplankton communities from several MDV lakes. This study concluded that primary production rates in Lake Bonney were stimulated by phosphorus addition. In contrast, additions of both nitrogen and phosphorus were required to observe a comparable response in Lake Fryxell phytoplankton. These findings correlated well with the long-term data record showing that soils and water within the Lake Bonney and Fryxell basins are deficient for phosphorus and nitrogen, respectively (Priscu, 1995).
Two decades after the initial study from Priscu, Teufel and colleagues performed a second nutrient bioassay on Lakes Bonney and Fryxell which incorporated 16S rRNA sequencing (Teufel et al., 2017). Based on results from the bbe FluoroProbe, they identified chlorophytes as the major contributors to the increase in chlorophyll a and rates of light-dependent primary production in nutrient bioassays conducted from the shallow surface waters of both Lake Fryxell and Bonney. In a similar experiment, lake water from both lakes were incubated in the presence or absence of nitrogen/phosphorus/glucose and community structure was compared using 18S rRNA gene sequencing (Figure 6). In contrast with the findings of Teufel et al., sequence data showed negligible changes in the phytoplankton communities from ELB: a cryptophyte population dominated both the control and treatments throughout the experiment (Figure 6A). However, Lake Fryxell showed a clear enrichment in Chlorophyta in response to the nutrient amendment (Figure 6B). Sherwell et al. added the environmental factor of light availability to the bioassay approach. These investigators found that light levels comparable to the high light environment of the open water moat caused inhibition of photochemistry and a loss of photoautotrophic protists, particularly Chlorophyta (Sherwell et al., 2022).
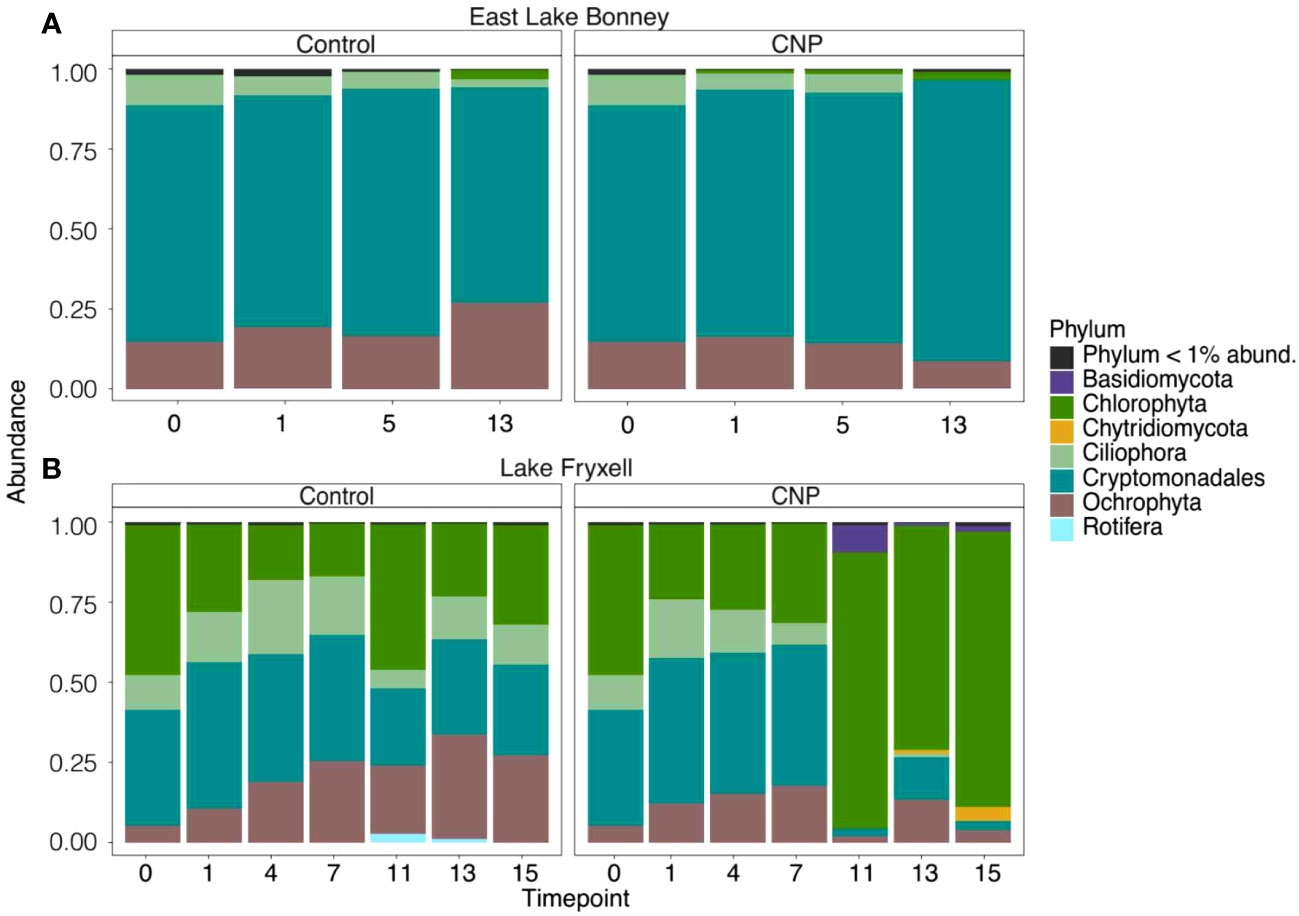
Figure 6 Impact of nutrient addition on protist community structure. Shallow water (5 m sampling depth) was collected from Lakes Bonney, east lobe (A) and Fryxell (B) and incubated in cubitainers in a temperature-controlled photoincubator with or without addition of nutrients. CNP, carbon (glucose)/nitrogen/phosphorus additions. Figure is based on data from: NCBI BioProject PRJNA1066876.
Another experiment was designed to mimic two impacts of warmer summers on phytoplankton communities in Lake Bonney by shifting their location in the water column. Lake water from ELB was captured in dialysis bags and transplanted either to the open water moats or deeper in the water column to mimic either ice-cover loss or one decade of lake level rise, respectively (Figures 5B, C; 7A). Principal coordinate analyses (PcoA) provided evidence of differential impacts on the eukaryotic community in response to the transplants (Figure 7B). Protist communities from the shallow layers of ELB exhibited higher sensitivity to the transplant treatments compared with original communities from deeper in the photic zone: 5 m communities shifted to either the moat or deeper into the water column both exhibited a significant shift in community structure (Figure 7B). Moreover, the transplanted shallow communities more closely resembled the protist communities from their new habitat, indicating ecological homogenization and reduced biodiversity (Figure 7B). Furthermore, specific protist taxa were either positively or negatively impacted by the transplants. In particular, relative abundance of Cryptophyta declined in response to the transplants, and were replaced by Chlorophyta (Figure 7C; Sherwell et al., 2022). The chlorophyte community was dominated by photoautotrophic Chlamydomonas spp., which supports other bioassay experiments as well as the long-term record that these organisms are competitive under climate-driven disturbances (Teufel et al., 2017; Patriarche et al., 2021). Ochrophyta were also stimulated in the moat transplant (Figure 7C): these sequences were related to a diverse group of heterotrophic eukaryotes, including a novel heterotrophic nanoflagellate from Biscosoecida (Sherwell et al., 2022). Last, photosynthetic efficiency of transplanted phytoplankton communities was negatively impacted, particularly in the moat transplant. These results suggest that the phytoplankton were exposed to high excitation pressure and responded by downregulation of their photosynthetic apparatus (Sherwell et al., 2022).
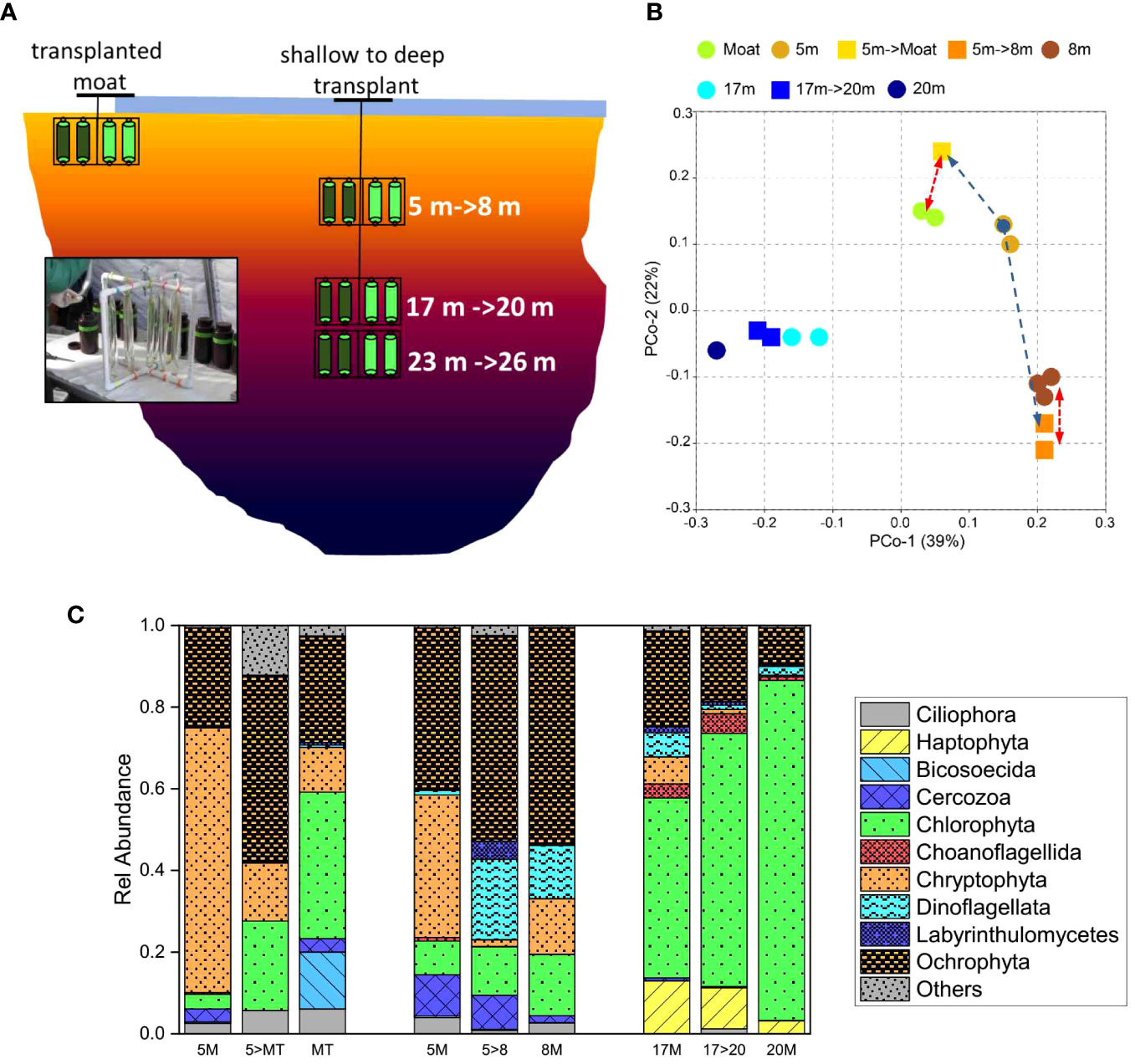
Figure 7 Sensitivity of MDV protists to manipulated environmental stress. (A). Schematic of the transplant experiment. Natural communities residing at various depths in the water column (5 m, 17 m, 23 m) were transferred to dialysis bags, attached to PVC frames, and transplanted to either the open water moat or 3 m deeper in the water column. Frames were retrieved after 14 days incubation. Image: a representative frame containing transplanted microbial communities. (B). PCoA cluster analysis of 18S rRNA from Lake Bonney (east lobe) in response to mimicked moat expansion or lake level rise. Circles, natural communities; squares, transplanted communities (n=1-3). The scatter plots are of principal coordinate 1 (PC1) versus principal coordinate 2 (PC2) from weighted UniFrac results. (Red line, shows homogenization of communities; blue line, represents sensitivity of shifted communities). (C). Changes in relative abundance eukaryal communities. Figure is based on data from ref: (Sherwell et al., 2022).
The disturbance experiments provide critical information regarding the short-term responses of microbial communities to mimicked episodic climate events. Overall, there are protist taxa (e.g. Chlorophyta) which exhibit higher resilience to environmental change and can take advantage of increased resources, such as nutrients. Other important protist taxa (e.g. Cryptophyta) appear to be highly sensitive to environmental perturbation. In future studies, long-term datasets from native communities can be used to validate the findings from these experiments performed under these manipulated conditions.
Physiological and genomic studies on isolated phytoplankton taxa
At the other end of the scale of experimental design, scientists have been studying isolates of key MDV protists under lab-controlled settings for three decades. While ex situ research, using algal isolates, cannot reproduce native environments, these studies provide highly detailed physiological information which is not possible within native communities residing in their complex and changing environment. As the major protist groups and important primary producers within the MDV lakes, physiological studies on the chlorophytes, cryptophytes, and haptophytes can inform how key photosynthetic and mixotrophic protists of these truncated food webs are equipped to survive under the current conditions and predict how resilient they will be to future climate events (Figure 8).
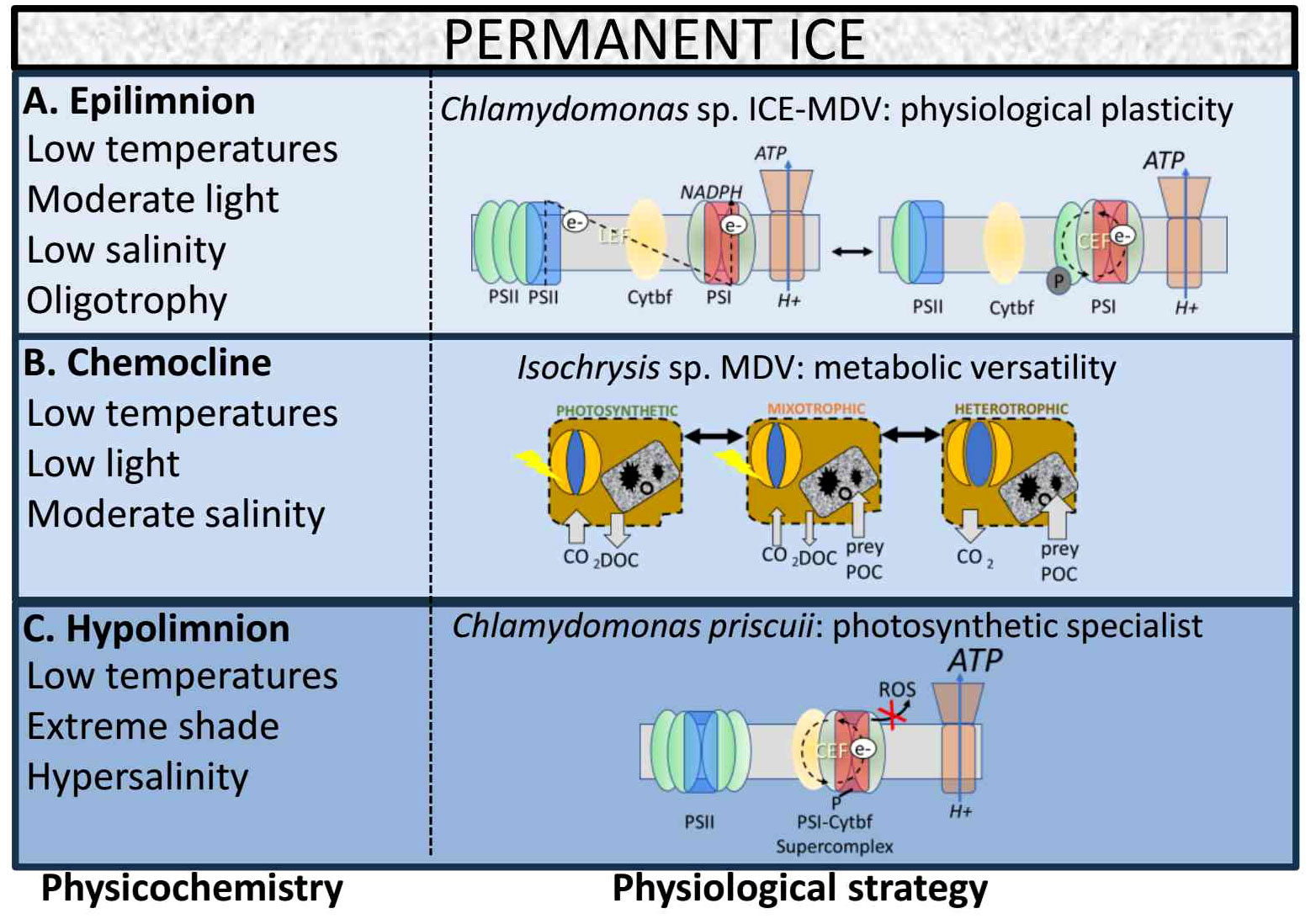
Figure 8 Working model of physiological strategies of key phytoplankton taxa in the Lake Bonney. (A). In the upper photic zone (Epilimnion), obligate photoautotrophic algae exhibit physiological plasticity to respond to environmental variability. The chlorophyte Chlamydomonas sp. ICE-MDV possesses the ability to reorganize its photosynthetic apparatus through the short-term mechanism, state transition. (B). In the chemocline, mixotrophic algae exhibit metabolic versatility. The haptophyte Isochrysis sp. MDV can switch between photosynthesis and phagotrophy, depending on the availability of light, nutrients and bacterial prey. (C). In the deep photic zone (Hypolimnion), phytoplankton are exposed to extreme shade and hypersalinity. The chlorophyte Chlamydomonas priscuii has rewired its photochemical apparatus to be locked in a conformation favoring PSI-driven electron transport.
Chlorophytes
Chlorophyta is a diverse phylum of algae: they are the closest algal relatives of land plants. Many are obligate photoautotrophs while others possess mixotrophic metabolism, i.e. combining light absorption with uptake of dissolved organic compounds. This mode of mixotrophy differs from many other protists who supplement light absorption with phagotrophy. The vast body of research on the model alga Chlamydomonas reinhardtii is conducted under mixotrophic conditions in growth medium containing acetate. Mixotrophic conditions enhance growth rates; however, at a cost of downregulation of photosynthetic capacity (Johnson and Alric, 2012). Chlorophytes are a dominant primary producer in Lake Bonney (Bielewicz et al., 2011; Kong et al., 2012b). Two isolated strains from ELB, Chlamydomonas priscuii and Chlamydomonas sp. ICE-MDV, have been studied under a variety of lab-controlled conditions (Figures 8A, C). C. priscuii was isolated from the deep photic zone (18 m) of ELB in the early 1990s and has emerged as a model of cold-adapted photosynthesis (reviewed in: (Morgan-Kiss et al., 2006; Dolhi et al., 2013; Cvetkovska et al., 2017, 2022). Chlamydomonas sp. ICE-MDV was isolated from the chemocline (15 m) in 2015 (Li et al., 2016; Cook et al., 2019), and it is one of the dominant chlorophyte taxa of the upper water columns of ELB and WLB (Li and Morgan-Kiss, 2019). While the natural habitat of both Chlamydomonas spp. is Lake Bonney, they exhibit distinct physiologies and stress tolerances which are likely due to the stratified layers in which they reside (Figures 8A, C). Isolated studies on these two organisms help to characterize how the chlorophyte populations of Lake Bonney may respond to changes such as temperature, nutrient availability, and light intensity at varying depths.
C. priscuii was isolated from the deep photic zone of ELB where it experiences low light (<50 μmol*m-2*s-1) enriched in blue spectrum (400 – 500 nm), high salinity (80 - 90 mS cm-1; 700 mM NaCl), low temperature (0 - 5 °C) and supersaturated O2 (30 - 40 mg L-1; 200% air saturation levels) (Neale and Priscu, 1995). C. priscuii has been the subject of nearly 30 years of physiological study (reviewed in (Morgan-Kiss et al., 2006; Cvetkovska et al., 2022; Hüner et al., 2023). The genome of C. priscuii has been sequenced and is available in two searchable databases (NCBI and Phytozome) making it a robust model for studying the genetic and physiological underpinnings of stress adaptation (Zhang et al., 2021). C. priscuii is an obligate photoautotroph and a psychrophile, which is defined as an organism obligately adapted to growth at cold temperatures (Morita, 1975). Psychrophily in this organism is at least partly due to highly unsaturated photosynthetic membranes and a high sensitivity of the photosynthetic apparatus to even moderately high temperatures (Morgan-Kiss et al., 2002a). Thus, short- or long-term increases in temperature are expected to significantly impair the growth of this organism.
C. priscuii has lost the rapid response mechanism of state transitions (Morgan-Kiss et al., 2002b); however, it possess a high capacity for photoprotection and oxidative stress detoxification and grows remarkably well under light conditions which are >10-fold higher than native light levels (Stahl-Rommel et al., 2022). Adaptation to the permanently high salinity environment may have contributed to the loss in capacity for state transitions: C. priscuii assembles a pigment-protein super complex which supports sustained rates of an alternative electron transport pathway around photosystem I (Kalra et al., 2020, 2023). Last, Hüner and colleagues reported that C. priscuii forms immobile, multicellular palmelloid structures which are functionally distinct from single cells and exhibit higher photoprotection from short-term high light stress (Szyszka-Mroz et al., 2022; Hüner et al., 2023). A recent study on two field isolates of C. reinhardtii also reported that palmelloids were essential for survival under excessive high light conditions (Suwannachuen et al., 2023). It is unknown whether C. priscuii palmelloids occur in Lake Bonney: it would seem to be a disadvantage in a permanently stratified water column, but could be a photoprotective mechanism in the mixed, open water moats.
Adaptation to a highly stable shade/low temperature/high salinity environment has resulted in a ‘locked’ physiology in C. priscuii which confers adaptive advantages under this extreme environmental regime (Figure 8C). Twenty years after the its isolation, C. priscuii was transplanted back to its native environment: despite long-term isolation in controlled laboratory conditions, the transplanted cultures responded dynamically in response to the transition from summer to the polar night by downregulating expression of major photosynthetic genes and functional downregulation of the photosynthetic apparatus (Morgan-Kiss et al., 2016). This study validated an earlier lab-based model that C. priscuii maintains its photosynthetic apparatus under mimicked winter conditions so that it can rapidly switch on its photochemical apparatus when summer returns (Morgan-Kiss et al., 2005, 2006). On the other hand, specialized adaptations which limit its ability to respond to environmental disturbance may be a disadvantage under future climate scenarios (Figure 8): indeed, C. priscuii is rarely detected in Lake Bonney in recent 18S rRNA surveys (Li and Morgan-Kiss, 2019). Thus, C. priscuii could represent one of the first casualties of climate change in the MDV lakes.
Chlamydomonas sp. ICE-MDV was more recently isolated and is also an obligate photoautotroph and a psychrophile; it displays distinct physiology compared with C. priscuii. Compared to C. priscuii, native communities of Chlamydomonas sp. ICE-MDV have been detected in a variety of locations, representing a broad range of light levels (Li and Morgan-Kiss, 2019), including the high-light environment of the open water moats (Sherwell et al., 2022). Lab-controlled experiments have also shown that Chlamydomonas sp. ICE-MDV has greater metabolic plasticity relative to C. priscuii, including some capacity for state transitions (Figure 8A; Kalra et al., 2023). Chlamydomonas sp. ICE-MDV also responds to variability in the micronutrient, Fe, with typically restructuring of Photosystem I (a high Fe-requiring reaction center), while C. priscuii exhibited minimal PSI restructuring (Cook et al., 2019). Interestingly, 77K fluorescence analyses on native algal communities from the Lake Bonney showed minimal PSI fluorescence emission (Kong et al., 2014). Chlamydomonas sp. ICE-MDV grows faster than C. priscuii under nonstress conditions and is capable of state transitions (Figure 8A), but exhibits significantly lower salinity tolerance (≤ 500 mM NaCl) (Kalra et al., 2023) compared to C. priscuii (up to 1200 mM NaCl; Pocock et al., 2011). Overall, we conclude that Chlamydomonas sp. ICE-MDV has retained physiological plasticity, while C. priscuii has evolved specialized mechanisms to survive additional extreme conditions, particularly high salt (Figures 8A, C). These differing strategies may serve Chlamydomonas sp. ICE-MDV under future climate-driven perturbations, such as thinning ice covers or even seasonal ice-free periods (Obryk et al., 2019) which could significantly increase light availability. As discussed above, mimicked disturbance experiments showed that Chlamydomonas sp. ICE-MDV is competitive under climate-related environmental changes (Teufel et al., 2017; Sherwell et al., 2022), for e.g. during high stream flow years.
Cryptophytes
Cryptophyceae is a group nanophytoplankton which harbors phycobilin-type chloroplasts derived from secondary endosymbiosis. Many are constitutive mixotrophs, or phago-mixotrophs, representing significant bacterivores in fresh water ecosystems (Grujcic et al., 2018). A recent paper also reported aplastic cryptophytes as dominant members of the heterotrophic nanoflagellate populations of freshwater environments (Šimek et al., 2023). Cryptophytes reside in the shallow layers of Lake Bonney but are rare in the deeper photic zone. In contrast, they are the dominant phototroph throughout the photic zone of Lake Fryxell (Bielewicz et al., 2011; Dolhi et al., 2015). Currently, it is unknown what drives the differential distribution of the cryptophyte populations in Lake Bonney versus Lake Fryxell, but higher cryptophyte abundance in the latter is likely supported by higher abundance of bacterial prey. Li and colleagues reported a negative correlation between salinity and cryptophytes (Li et al., 2019), which could also explain why cryptophyte populations are restricted to the upper water column of saline Lake Bonney Similarly, cryptophytes occupy lower salinity regions along the coast of the Antarctic peninsula (Mendes et al., 2013; Mendes et al., 2023).
Cryptomonad isolates from the MDV lakes are currently unavailable and so detailed knowledge of their physiology and response to varying environmental conditions is limited. A closely related isolate Geminigera cryophila, has been studied for several years and has yielded significant information regarding its ability to acclimate to a number of environmental variables (Trimborn et al., 2019; Camoying and Trimborn, 2023). These studies have revealed that the marine cryptophyte exhibits physiological plasticity to respond to variations in temperature and light. Trimborn and colleagues reported that G. cryophila was sensitive to increasing irradiance, with complete growth inhibition under high light (Trimborn et al., 2019). However, in the presence of high CO2 (a condition associated with ocean acidification), this light sensitivity phenotype was alleviated. Growth of G. cryophila was also stimulated under warming temperatures (Camoying and Trimborn, 2023). The conditions of higher and variable light combined with warmer temperatures and high CO2 match climate-driven changes in the WAP. Indeed, a recent paper from Mendes and colleagues reported that cryptophytes are gradually outgrowing diatoms in the Antarctic coastal marine ecosystem as oceanic pCO2 increases and conditions switch to prolonged summer stratification (Mendes et al., 2023). It is unknown whether the MDV cryptophytes will respond in a comparable manner as their WAP counterparts; however, as discussed above, results from the disturbance experiments conducted thus far suggest that the MDV cryptophyte communities are highly sensitive to environmental perturbation and are less competitive than the chlorophytes (Teufel et al., 2017; Sherwell et al., 2022).
Haptophytes
The order Isochrysidales (Phylum Haptophyta) is a second abundant mixotrophic nanophytoplankton taxa in the MDV lakes. Haptophytes have major environmental importance in aquatic habitats: mixotrophic haptophytes are major grazers of bacteria in marine environments (Unrein et al., 2014; Penot et al., 2022). A single haptophyte species belonging to the genus Isochrysis, appears to be highly abundant within the chemoclines in both lobes of Lake Bonney (Figure 8B) (Bielewicz et al., 2011; Dolhi et al., 2015). Kong et al. (Kong et al., 2012b) detected relatively high levels of haptophyte rbcL ID transcripts in the chemocline of Lake Bonney, suggesting that Isochrysis contributes significantly to primary production. There is no evidence that this mixotroph is present in other MDV lakes (Li et al., 2019).
There is evidence that the haptophyte community in Lake Bonney may be a major contributor to biogenic sulfur cycling in Lake Bonney. ELB has some of the highest levels of DMSP recorded (Lee et al., 2004). DMSP is an algal osmolyte in marine environments which is almost exclusively produced by photosynthetic eukaryotes (Sunda et al., 2002). Since Lake Bonney is a hypersaline lake, it is highly likely that one of the dominant phytoplankton is responsible for its production. While the identity of the DMSP-producing algae is currently not known, peaks in DMSP were reported at depths of maximal expression of the rbcL ID gene of Isochrysis sp., suggesting that this organism might be the major producer of DMSP (Kong et al., 2012a).
Details on the physiology of the MDV Isochrysis sp. are minimal; however, a nonaxenic Isochrysis (named Isochrysis sp. MDV) culture from Lake Bonney was isolated in 2016 (Li et al., 2016). Isochrysis sp. MDV is most closely related to a mesophilic species, I. galbana (Bielewicz et al., 2011). One master’s thesis reported some preliminary analyses of the physiology of the MDV strain, including a comparison between Chlamydomonas spp. and Isochrysis sp. MDV under mimicked polar night (Cariani, 2019). This study found that Isochrysis sp. MDV strain is psychrophilic and can grow under variable light levels of up to 250 μmol photons m-2 s-1. Growth rates of Isochrysis sp. MDV did not change significantly under a range of light intensities, and photochemical activity was generally lower compared with MDV Chlamydomonas spp. isolates (Cariani, 2019). Last, Isochrysis sp. MDV exhibited a two-fold increase in growth rate and cell biomass yield under mixotrophic growth conditions relative to photoautotrophic conditions, and Li et al. reported the presence of an acidic food vacuole (Li et al., 2016).
In response to mimicked winter conditions in a lab-controlled experiment, Chlamydomonas sp. ICE-MDV and C. priscuii rapidly downregulated photochemistry activity upon the onset of 24-hour darkness, while Isochrysis sp. MDV maintained functional photochemical activity throughout the dark incubation of up to 4 weeks. These findings from lab-controlled experiments fit with findings from Kong et al. (Kong et al., 2012b) who reported that expression of haptophyte rbcL ID is not correlated with light availability during the transition from summer to polar winter, while chlorophyte rbcL IAB declined with linearly with light over the same time period. This suggests that Isochrysis sp. MDV is a facultative phototroph that relies on heterotrophy under low light or nutrients (Figure 8B). We conclude that unlike the Chlamydomonas spp. of Lake Bonney which are reliant the physiological strategy of a tunable photochemical apparatus, Isochrysis relies on the physiological strategy of metabolic versatility to be competitive under low light (summer) and dark (winter) conditions (Figure 8B).
Future directions and training the next generation
Protists clearly play outsized roles in the truncated food webs of the McMurdo Dry Valley lakes. While three decades of research on the native communities in concert with focused investigations on several isolates, we are really at the beginning of the journey to fully understand the functional contributions of protists to polar aquatic ecosystems. In the MDV lakes, the current literature represents an overemphasis on photosynthetic representatives: there is a need for improved understanding of diversity and functional roles of heterotrophic protists, particularly poorly understood trophic modes including parasitic, saprotrophic, mutualistic, and symbiotic lifestyles. Our understanding of the contributions of parasitic and saprotrophic fungi to the MDV lake biogeochemical cycles is also in its infancy. The long-term data record of the McMurdo LTER is invaluable for understanding the physical and chemical trends in response to climate-driven events: the temporal record of MDV protist communities is catching up.
Last, on a broader scale, a place for MDV protists within the context of global protist diversity should be addressed. A recent paper reported on the biogeography of protists among marine, freshwater and soil communities (Singer et al., 2021). Their results confirm earlier findings that salinity is an environmental barrier between marine and freshwater ecosystems, preventing marine-freshwater transitions between microbial communities (Logares et al., 2009). Unlike marine and freshwater systems which are geographically separated, salinity is a major physicochemical factor with strong gradients within the MDV lakes. As studies on MDV algal isolates have shown, there are protist lineages within the same lake which possess different salinity tolerances and physiology (Kalra et al., 2020, Kalra et al., 2023). Identifying additional protist lineages with varying salt sensitivity could make them valuable sentinels of climate change.
Antarctic field research provides one of the most unique training opportunities in the world. The McMurdo LTER has been training and sending early career scientists, particularly graduate students and postdoctoral fellows, to work in the dry valleys for three decades. Many of these scientists have gone on to continue to conduct research in polar environments, but others have pursued diverse careers in government agencies (e.g. EPA and NASA) and private industry. Laboratory-based inquiries provide additional opportunities for many more early career researchers to get involved in Antarctic research than would not be possible from field-based studies. Specifically, laboratory-based inquiries exploiting isolates of MDV lake phytoplankton have recruited a large number of high school and undergraduate students to work on independent research projects. Last field- and laboratory-based research activities on the MDV protists have engaged a large number of early career female scientists (Figure 9). These remarkable women represent role models, inspiring the next generation of polar scientists!
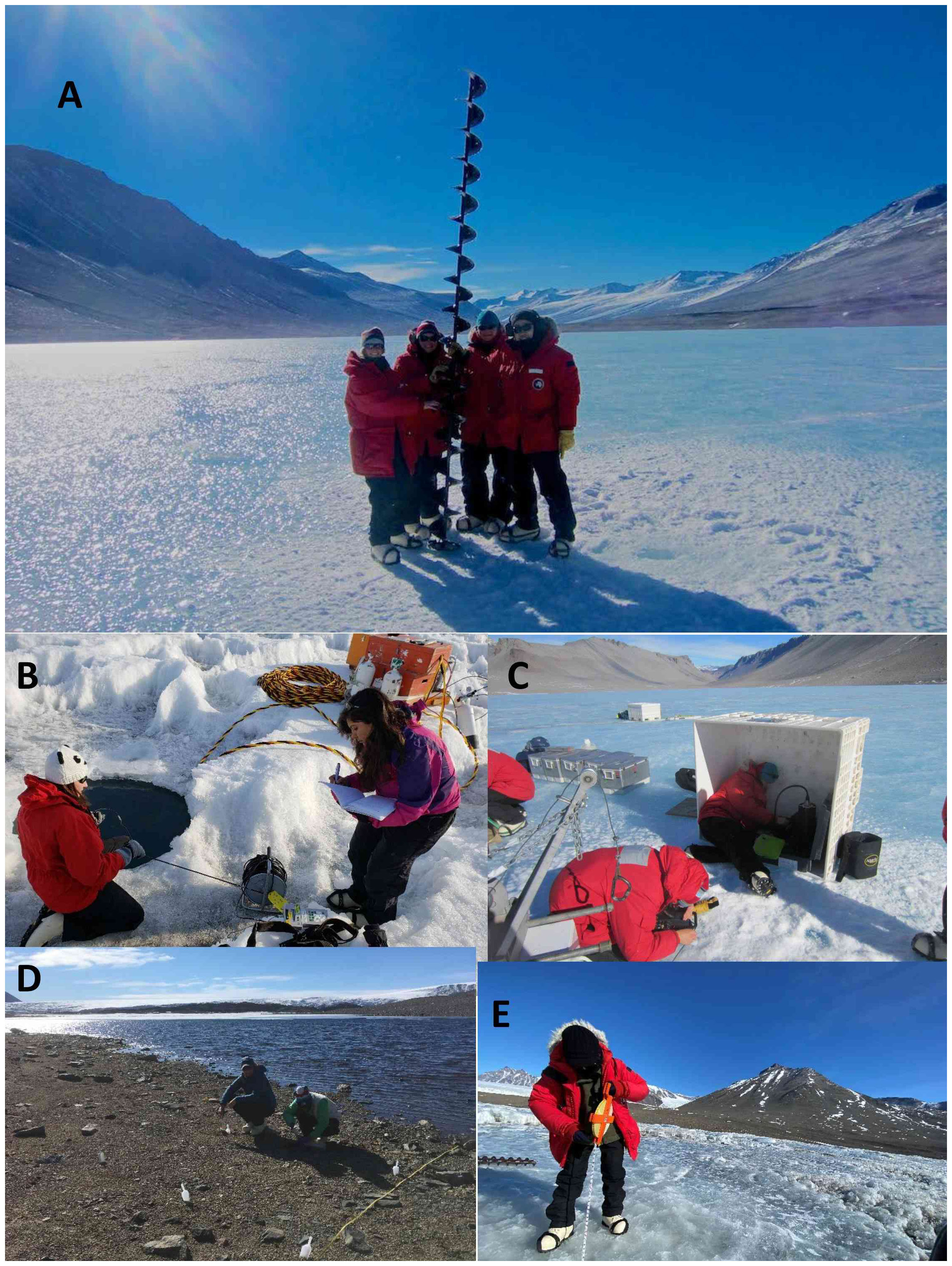
Figure 9 Early career polar scientists working in the field in McMurdo Dry Valleys, Antarctica (A-D) and growing Antarctic algae in the lab (E). (A) “Team Protist” – J Dolhi-Binder (PhD 2015), (A) Teufel (PhD 2016), R. Morgan-Kiss, W. Li (PhD 2016), Lake Bonney, Nov 2012. (B) S. Sherwell (MSc 2018) & (I) Kalra (PhD 2020) measuring PAR, Lake Bonney, Jan 2019. (C) A. Teufel & R. Morgan-Kiss deploying the bbe FluoroProbe, Lake Vanda, Nov 2017. (D) C. Takacs-Vesbach and (E) Rynebeau (current PhD student) collecting samples at Lake Brownworth, Jan 2020 (E) R. Pereira (current PhD student) measuring ice thickness, Lake Fryxell, December 2022.
Author contributions
RM-K: Conceptualization, Funding acquisition, Writing – original draft, Writing – review and editing. DP: Writing – original draft, Writing – review and editing. RP: Writing – original draft, Writing – review and editing. JD-B: Writing – review and editing. AT: Writing – review and editing. WL: Writing – review and editing. IK: Writing – review and editing. SS: Writing – review and editing. ER: Writing – review and editing. CT-V: Writing – review and editing.
Funding
The author(s) declare that financial support was received for the research, authorship, and/or publication of this article. The National Science Foundation provided support for all field-based studies. The Department of Energy provided support for recent studies on Chlamydomonas spp. RM-K, JD-B, SS, AT, WL, RP, DP were supported by the National Science Foundation (OPP-1056396 and -1637708) and the Department of Energy (DE-SC0019138). ER and CT-V were supported by the National Science Foundation (OPP-1637708). WL is partially supported by Lawrence Livermore National Laboratory operated by Lawrence Livermore National Security, LLC, for the U.S. Department of Energy, National Nuclear Security Administration under Contract DE-AC52-07NA27344.
Acknowledgments
The authors thank McMurdo LTER students and technicians for field and lab assistance. We also thank Antarctic Support Contractors and Petroleum Helicopters International for logistical support.
Conflict of interest
Author AT was employed by the company Procter & Gamble.
The remaining authors declare that the research was conducted in the absence of any commercial or financial relationships that could be construed as a potential conflict of interest.
Publisher’s note
All claims expressed in this article are solely those of the authors and do not necessarily represent those of their affiliated organizations, or those of the publisher, the editors and the reviewers. Any product that may be evaluated in this article, or claim that may be made by its manufacturer, is not guaranteed or endorsed by the publisher.
References
Azam F., Malfatti F. (2007). Microbial structuring of marine ecosystems. Nat. Rev. Microbiol. 5, 782–791. doi: 10.1038/nrmicro1747
Beutler M., Wiltshire K. H., Meyer B., Moldaenke C., Lüring C., Meyerhöfer M., et al. (2002). A fluorometric method for the differentiation of algal populations in vivo and in situ. Photosyn. Res. 72, 39–53. doi: 10.1023/A:1016026607048
Bielewicz S., Bell E. M., Kong W., Friedberg I., Priscu J. C., Morgan-Kiss R. M. (2011). Protist diversity in a permanently ice-covered Antarctic lake during the polar night transition. ISME J. 5, 1559–1564. doi: 10.1038/ismej.2011.23
Brooks S. T., Jabour J., Van Den Hoff J., Bergstrom D. M. (2019). Our footprint on Antarctica competes with nature for rare ice-free land. Nat. Sustainabil. 2, 185–190. doi: 10.1038/s41893-019-0237-y
Burki F., Sandin M. M., Jamy M. (2021). Diversity and ecology of protists revealed by metabarcoding. Curr. Biol. 31, R1267–R1280. doi: 10.1016/j.cub.2021.07.066
Camoying M. G., Trimborn S. (2023). Physiological response of an Antarctic cryptophyte to increasing temperature, CO2, and irradiance. Limnol. Oceanogr. 68, 1880–1894. doi: 10.1002/lno.12392
Cariani Z. (2019). Impact of simulated polar night on Antarctic mixotrophic and strict photoautotrophic phytoplankton (Oxford, OH: Miami University).
Caron D. A., Countway P. D., Jones A. C., Kim D. Y., Schnetzer A. (2012). Marine protistan diversity. Annu. Rev. Mar. Sci. 4, 467–493. doi: 10.1146/annurev-marine-120709-142802
Carpenter S. R., Frost T. M., Kitchell J. F., Kratz T. K., Schindler D. W., Shearer J., et al. (1991). “Patterns of primary production and herbivory in 25 North American lake ecosystems,” in Comparative analyses of ecosystems: Patterns, mechanisms, and theories (Springer), 67–96.
Cook G., Teufel A., Kalra I., Li W., Wang X., Priscu J., et al. (2019). The Antarctic psychrophiles Chlamydomonas spp. UWO241 and ICE-MDV exhibit differential restructuring of photosystem I in response to iron. Photosyn. Res. 141, 209–228. doi: 10.1007/s11120-019-00621-0
Cvetkovska M., Hüner N. P., Smith D. R. (2017). Chilling out: the evolution and diversification of psychrophilic algae with a focus on Chlamydomonadales. Polar Biol. 40, 1169–1184. doi: 10.1007/s00300-016-2045-4
Cvetkovska M., Vakulenko G., Smith D. R., Zhang X., Hüner N. P. (2022). Temperature stress in psychrophilic green microalgae: Minireview. Physiol. Plant. 174, e13811. doi: 10.1111/ppl.13811
De Vargas C., Audic S., Henry N., Decelle J., Mahé F., Logares R., et al. (2015). Eukaryotic plankton diversity in the sunlit ocean. Science 348, 1261605. doi: 10.1126/science.1261605
Dolhi J. M., Maxwell D. P., Morgan-Kiss R. M. (2013). The Antarctic Chlamydomonas raudensis: an emerging model for cold adaptation of photosynthesis. Extremophiles 17, 711–722. doi: 10.1007/s00792-013-0571-3
Dolhi J. M., Teufel A. G., Kong W., Morgan-Kiss R. M. (2015). Diversity and spatial distribution of autotrophic communities within and between ice-covered Antarctic lakes (McMurdo Dry Valleys). Limnol. Oceanogr. 60, 977–991. doi: 10.1002/lno.10071
Doran P. T., Gooseff M. N. (2023). Lake level surveys in the McMurdo Dry Valleys, Antarctica, (1991-2023, ongoing). E.D. Initiative.
Doran P. T., Priscu J. C., Lyons W. B., Walsh J. E., Fountain A. G., Mcknight D. M., et al. (2002). Antarctic climate cooling and terrestrial ecosystem response. Nature 415, 517–520. doi: 10.1038/nature710
Falkowski P. G., Barber R. T., Smetacek V. (1998). Biogeochemical controls and feedbacks on ocean primary production. science 281, 200–206. doi: 10.1126/science.281.5374.200
Field C. B., Behrenfeld M. J., Randerson J. T., Falkowski P. (1998). Primary production of the biosphere: integrating terrestrial and oceanic components. science 281, 237–240. doi: 10.1126/science.281.5374.237
Foreman C. M., Wolf C. F., Priscu J. C. (2004). Impact of episodic warming events. Aquat. Geochem. 10, 239–268. doi: 10.1007/s10498-004-2261-3
Fritsen C. H., Priscu J. C. (1999). Seasonal change in the optical properties of the permanent ice cover on Lake Bonney, Antarctica: consequences for lake productivity and phytoplankton dynamics. Limnol. Oceanogr. 44, 447–454. doi: 10.4319/lo.1999.44.2.0447
Gibson J. A. (1999). The meromictic lakes and stratified marine basins of the Vestfold Hills, East Antarctica. Antarctic Sci. 11, 175–192. doi: 10.1017/S0954102099000243
Gibson J. A., Burton H. R. (1996). Meromictic Antarctic lakes as recorders of climate change: The structures of Ace and Organic Lakes, Vestfold Hills, Antarctica. Papers Proc. R. Soc. 130, 73–78. doi: 10.26749/rstpp.130.2.73
Gooseff M. N., Barrett J. E., Adams B. J., Doran P. T., Fountain A. G., Lyons W. B., et al. (2017a). Decadal ecosystem response to an anomalous melt season in a polar desert in Antarctica. Nat. Ecol. Evol. 1, 1334–1338. doi: 10.1038/s41559-017-0253-0
Gooseff M. N., Mcknight D. M., Doran P. T., Fountain A. (2022). Long-term stream hydrology and meteorology of a Polar Desert, the McMurdo Dry Valleys, Antarctica. Hydrol. Process. 36, e14623. doi: 10.1002/hyp.14623
Gooseff M. N., Wlostowski A., Mcknight D. M., Jaros C. (2017b). Hydrologic connectivity and implications for ecosystem processes-Lessons from naked watersheds. Geomorphology 277, 63–71. doi: 10.1016/j.geomorph.2016.04.024
Green W. J., Lyons W. B. (2009). The saline lakes of the mcmurdo dry valleys, Antarctica. Aquat. Geochem. 15, 321–348. doi: 10.1007/s10498-008-9052-1
Grujcic V., Nuy J. K., Salcher M. M., Shabarova T., Kasalicky V., Boenigk J., et al. (2018). Cryptophyta as major bacterivores in freshwater summer plankton. ISME J. 12, 1668–1681. doi: 10.1038/s41396-018-0057-5
Hawes I. (1990). “Eutrophication and vegetation development in maritime Antarctic lakes,” in Antarctic ecosystems: ecological change and conservation (Heidelberg, Germany: Springer Berlin), 83–90.
Howard-Williams C., Schwarz A. M., Hawes I., Priscu J. C. (1998). Optical properties of the McMurdo dry valley lakes, Antarctica. Ecosys. dynam. polar desert: McMurdo Dry Valleys Antarctica 72, 189–203. doi: 10.1029/AR072p0189
Hüner N. P., Smith D. R., Cvetkovska M., Zhang X., Ivanov A. G., Szyszka-Mroz B., et al. (2022). Photosynthetic adaptation to polar life: Energy balance, photoprotection and genetic redundancy. J. Plant Physiol. 268, 153557. doi: 10.1016/j.jplph.2021.153557
Hüner N. P., Szyszka-Mroz B., Ivanov A. G., Kata V., Lye H., Smith D. R. (2023). Photosynthetic adaptation and multicellularity in the Antarctic psychrophile, Chlamydomonas priscuii. Algal Res. 103220. doi: 10.1016/j.algal.2023.103220
Johnson X., Alric J. (2012). Interaction between starch breakdown, acetate assimilation, and photosynthetic cyclic electron flow in Chlamydomonas reinhardtii. J. Biol. Chem. 287, 26445–26452. doi: 10.1074/jbc.M112.370205
Kalra I., Wang X., Cvetkovska M., Jeong J., Mchargue W., Zhang R., et al. (2020). Chlamydomonas sp. UWO 241 exhibits high cyclic electron flow and rewired metabolism under high salinity. Plant Physiol. 183, 588–601. doi: 10.1104/pp.19.01280
Kalra I., Wang X., Zhang R., Morgan-Kiss R. (2023). High salt-induced PSI-supercomplex is associated with high CEF and attenuation of state transitions. Photosyn. Res. 157, 65–84. doi: 10.1007/s11120-023-01032-y
Keeling P. J., Burger G., Durnford D. G., Lang B. F., Lee R. W., Pearlman R. E., et al. (2005). The tree of eukaryotes. Trends Ecol. Evol. 20, 670–676. doi: 10.1016/j.tree.2005.09.005
Keeling P. J., Burki F. (2019). Progress towards the tree of eukaryotes. Curr. Biol. 29, R808–R817. doi: 10.1016/j.cub.2019.07.031
Kong W., Dolhi J. M., Chiuchiolo A., Priscu J., Morgan-Kiss R. M. (2012a). Evidence of form II RubisCO (cbbM) in a perennially ice-covered Antarctic lake. FEMS Microbiol. Ecol. 82, 491–500. doi: 10.1111/fem.2012.82.issue-2
Kong W., Li W., Romancova I., Prášil O., Morgan-Kiss R. M. (2014). An integrated study of photochemical function and expression of a key photochemical gene (psbA) in photosynthetic communities of Lake Bonney (McMurdo Dry Valleys, Antarctica). FEMS Microbiol. Ecol. 89, 293–302. doi: 10.1111/fem.2014.89.issue-2
Kong W., Ream D. C., Priscu J. C., Morgan-Kiss R. M. (2012b). Diversity and expression of RubisCO genes in a perennially ice-covered Antarctic lake during the polar night transition. Appl. Environ. Microbiol. 78, 4358–4366. doi: 10.1128/AEM.00029-12
Kring S. A., Figary S. E., Boyer G. L., Watson S. B., Twiss M. R. (2014). Rapid in situ measures of phytoplankton communities using the bbe FluoroProbe: evaluation of spectral calibration, instrument intercompatibility, and performance range. Can. J. Fish. Aquat. Sci. 71, 1087–1095. doi: 10.1139/cjfas-2013-0599
Kwon M., Kim M., Takacs-Vesbach C., Lee J., Hong S. G., Kim S. J., et al. (2017). Niche specialization of bacteria in permanently ice-covered lakes of the M cMurdo Dry Valleys, A ntarctica. Environ. Microbiol. 19, 2258–2271. doi: 10.1111/1462-2920.13721
Laundon D., Mock T., Wheeler G., Cunliffe M. (2021). Healthy herds in the phytoplankton: the benefit of selective parasitism. ISME J. 15, 2163–2166. doi: 10.1038/s41396-021-00936-8
Laybourn-Parry J., Bell E. M. (2014). Ace Lake: three decades of research on a meromictic, Antarctic lake. Polar Biol. 37, 1685–1699. doi: 10.1007/s00300-014-1553-3
Laybourn-Parry J., Ellis-Evans J. C., Butler H. (1996). Microbial dynamics during the summer ice-loss phase in maritime Antarctic lakes. J. Plankton Res. 18, 495–511. doi: 10.1093/plankt/18.4.495
Laybourn-Parry J., James M.R., McKnight D. M., Priscu J., Spaulding S. A, Shiel R. (1997). The microbial plankton of Lake Fryxell, southern Victoria Land, Antarctica during the summers of 1992 and 1994. Polar Biol. 17, 54–61.
Laybourn-Parry J., Roberts E. C., Bell E. M. (2000). “Mixotrophy as a survival strategy in Antarctic lakes,” in Antarctic Ecosystems: Models for wider Ecological Understanding. Eds. Davidson W., Howard-Williams C., Broady P. A. (The Caxon Press, Christchurch, NZ), 33–40.
Lee P. A., Priscu J. C., Ditullio G. R., Riseman S. F., Tursich N., Demora S. J. (2004). Elevated levels of dimethylated-sulfur compounds in Lake Bonney, a poorly ventilated Antarctic lake. Limnol. Oceanogr. 49, 1044–1055. doi: 10.4319/lo.2004.49.4.1044
Li W., Dolhi-Binder J., Cariani Z. E., Morgan-Kiss R. M. (2019). Drivers of protistan community autotrophy and heterotrophy in chemically stratified Antarctic lakes. Aquat. Microbial. Ecol. 82, 225–239. doi: 10.3354/ame01891
Li W., Morgan-Kiss R. M. (2019). Influence of environmental drivers and potential interactions on the distribution of microbial communities from three permanently stratified Antarctic lakes. Front. Microbiol. 10, 1067. doi: 10.3389/fmicb.2019.01067
Li W., Podar M., Morgan-Kiss R. M. (2016). Ultrastructural and single-cell-level characterization reveals metabolic versatility in a microbial eukaryote community from an ice-covered Antarctic lake. Appl. Environ. Microbiol. 82, 3659–3670. doi: 10.1128/AEM.00478-16
Lima-Mendez G., Faust K., Henry N., Decelle J., Colin S., Carcillo F., et al. (2015). Determinants of community structure in the global plankton interactome. Science 348, 1262073. doi: 10.1126/science.1262073
Logares R., Bråte J., Bertilsson S., Clasen J. L., Shalchian-Tabrizi K., Rengefors K. (2009). Infrequent marine–freshwater transitions in the microbial world. Trends Microbiol. 17, 414–422. doi: 10.1016/j.tim.2009.05.010
Lyons W., Fountain R., Priscu J. C., Neumann K., Welch K. A. (2000). Importance of landscape position and legacy: the evolution of the lakes in Taylor Valley, Antarctica. Freshw. Biol. 43, 355–367. doi: 10.1046/j.1365-2427.2000.00513.x
Mendes C. R. B., Costa R. R., Ferreira A., Jesus B., Tavano V. M., Dotto T. S., et al. (2023). Cryptophytes: An emerging algal group in the rapidly changing Antarctic Peninsula marine environments. Global Change Biol. 29, 1791–1808. doi: 10.1111/gcb.16602
Mendes C. R. B., Tavano V. M., Leal M. C., De Souza M. S., Brotas V., Garcia C. (2013). Shifts in the dominance between diatoms and cryptophytes during three late summers in the Bransfield Strait (Antarctic Peninsula). Polar Biol. 36, 537–547. doi: 10.1007/s00300-012-1282-4
Morgan-Kiss R. M., Ivanov A. G., Huner N. P. (2002b). The Antarctic psychrophile, Chlamydomonas subcaudata, is deficient in state I–state II transitions. Planta 214, 435–445. doi: 10.1007/s004250100635
Morgan-Kiss R. M., Ivanov A. G., Pocock T., Król M., Gudynaite-Savitch L., Hüner N. P. (2005). The Antarctic psychrophile, Chlamydomonas raudensis ettl (UWO241)(Chlorophyceae, Chlorophyta), exhibits a limited capacity to photoacclimate to red light. J. Phycol. 41, 791–800. doi: 10.1111/j.1529-8817.2005.04174.x
Morgan-Kiss R., Ivanov A. G., Williams J., Khan M., Huner N. P. (2002a). Differential thermal effects on the energy distribution between photosystem II and photosystem I in thylakoid membranes of a psychrophilic and a mesophilic alga. Biochim. Biophys. Acta (BBA)-Biomembranes 1561, 251–265. doi: 10.1016/S0005-2736(02)00352-8
Morgan-Kiss R., Lizotte M., Kong W., Priscu J. (2016). Photoadaptation to the polar night by phytoplankton in a permanently ice-covered Antarctic lake. Limnol. Oceanogr. 61, 3–13. doi: 10.1002/lno.10107
Morgan-Kiss R. M., Priscu J. C., Pocock T., Gudynaite-Savitch L., Huner N. P. (2006). Adaptation and acclimation of photosynthetic microorganisms to permanently cold environments. Microbiol. Mol. Biol. Rev. 70, 222–252. doi: 10.1128/MMBR.70.1.222-252.2006
Morita R. Y. (1975). Psychrophilic bacteria. Bacteriol. Rev. 39, 144–167. doi: 10.1128/br.39.2.144-167.1975
Neale P. J., Priscu J. C. (1995). The photosynthetic apparatus of phytoplankton from a perennially ice-covered Antarctic lake: acclimation to an extreme shade environment. Plant Cell Physiol. 36, 253–263. doi: 10.1093/oxfordjournals.pcp.a078757
Obryk M. K., Doran P. T., Priscu J. C. (2019). Prediction of ice-free conditions for a perennially ice-covered Antarctic lake. J. Geophys. Res.: Earth Surf. 124, 686–694. doi: 10.1029/2018JF004756
Patriarche J., Priscu J., Takacs-Vesbach C., Winslow L., Myers K., Buelow H., et al. (2021). Year-round and long-term phytoplankton dynamics in Lake Bonney, a permanently ice-covered Antarctic lake. J. Geophys. Res.: Biogeosci. 126, e2020JG005925.
Penot M., Dacks J. B., Read B., Dorrell R. G. (2022). Genomic and meta-genomic insights into the functions, diversity and global distribution of haptophyte algae. Appl. Phycol. 3, 340–359. doi: 10.1080/26388081.2022.2103732
Pocock T., Vetterli A., Falk S. (2011). Evidence for phenotypic plasticity in the Antarctic extremophile Chlamydomonas raudensis Ettl. UWO 241. J. Exp. Bot. 62, 1169–1177. doi: 10.1093/jxb/erq347
Poreda R. J., Hunt A. G., Lyons W. B., Welch K. A. (2004). The helium isotopic chemistry of Lake Bonney, Taylor Valley, Antarctica: Timing of late Holocene climate change in Antarctica. Aquat. Geochem. 10, 353–371. doi: 10.1007/s10498-004-2265-z
Priscu J. C. (1995). Phytoplankton nutrient deficiency in lakes of the McMurdo Dry Valleys, Antarctica. Freshw. Biol. 34, 215–227. doi: 10.1111/j.1365-2427.1995.tb00882.x
Priscu J. (2023). Lake ice thickness and density measurments, McMurdo Dry Valleys, Antarctica, (1989-2023, ongoing). E.D. Initiative.
Priscu J. C., Wolf C. F., Takacs C. D., Fritsen C. H., Laybourn-Parry J., Roberts J. K. M., et al. (1999). Carbon transformations in the water column of a perennially ice-covered Antarctic Lake. Biosci 49, 997–1008. doi: 10.2307/1313733
Roberts E. C., Laybourn-Parry J. (1999). Mixotrophic cryptophytes and their predators in the Dry Valley lakes of Antarctica. Freshw. Biol. 41, 737–746. doi: 10.1046/j.1365-2427.1999.00401.x
Robinson S. A., Klekociuk A. R., King D. H., Pizarro Rojas M., Zúñiga G. E., Bergstrom D. M. (2020). The 2019/2020 summer of Antarctic heatwaves. Global Change Biol. 26, 3178–3180. doi: 10.1111/gcb.15083
Rojas-Jimenez K., Wurzbacher C., Bourne E. C., Chiuchiolo A., Priscu J. C., Grossart H.-P. (2017). Early diverging lineages within Cryptomycota and Chytridiomycota dominate the fungal communities in ice-covered lakes of the McMurdo Dry Valleys, Antarctica. Sci. Rep. 7, 15348. doi: 10.1038/s41598-017-15598-w
Sanders R. W. (1991). Mixotrophic protists in marine and freshwater ecosystems. J. protozool. 38, 76–81. doi: 10.1111/j.1550-7408.1991.tb04805.x
Santoferrara L., Burki F., Filker S., Logares R., Dunthorn M., Mcmanus G. B. (2020). Perspectives from ten years of protist studies by high-throughput metabarcoding. J. Eukaryotic Microbiol. 67, 612–622. doi: 10.1111/jeu.12813
Seeleuthner Y., Mondy S., Lombard V., Carradec Q., Pelletier E., Wessner M., et al. (2018). Single-cell genomics of multiple uncultured stramenopiles reveals underestimated functional diversity across oceans. Nat. Commun. 9, 310. doi: 10.1038/s41467-017-02235-3
Sherwell S., Kalra I., Li W., Mcknight D. M., Priscu J. C., Morgan-Kiss R. M. (2022). Antarctic lake phytoplankton and bacteria from near-surface waters exhibit high sensitivity to climate-driven disturbance. Environ. Microbiol. 24, 6017–6032. doi: 10.1111/1462-2920.16113
Šimek K., Mukherjee I., Szöke-Nagy T., Haber M., Salcher M. M., Ghai R. (2023). Cryptic and ubiquitous aplastidic cryptophytes are key freshwater flagellated bacterivores. ISME J. 17, 84–94. doi: 10.1038/s41396-022-01326-4
Singer D., Seppey C. V., Lentendu G., Dunthorn M., Bass D., Belbahri L., et al. (2021). Protist taxonomic and functional diversity in soil, freshwater and marine ecosystems. Environ. Int. 146, 106262. doi: 10.1016/j.envint.2020.106262
Spigel R. H., Priscu J. C. (1996). Evolution of temperature and salt structure of Lake Bonney, a chemically stratified Antarctic lake. Hydrobiologia 321, 177–190. doi: 10.1007/BF00143749
Stahl-Rommel S., Kalra I., D’silva S., Hahn M. M., Popson D., Cvetkovska M., et al. (2022). Cyclic electron flow (CEF) and ascorbate pathway activity provide constitutive photoprotection for the photopsychrophile, Chlamydomonas sp. UWO 241 (renamed Chlamydomonas priscuii). Photosyn. Res. 151, 235–250. doi: 10.1007/s11120-021-00877-5
Stoecker D. K., Hansen P. J., Caron D. A., Mitra A. (2017). Mixotrophy in the marine plankton. Annu. Rev. Mar. Sci. 9, 311–335. doi: 10.1146/annurev-marine-010816-060617
Sunda W., Kieber D., Kiene R., Huntsman S. (2002). An antioxidant function for DMSP and DMS in marine algae. Nature 418, 317–320. doi: 10.1038/nature00851
Suwannachuen N., Leetanasaksakul K., Roytrakul S., Phaonakrop N., Thaisakun S., Roongsattham P., et al. (2023). Palmelloid formation and cell aggregation are essential mechanisms for high light tolerance in a natural strain of Chlamydomonas reinhardtii. Int. J. Mol. Sci. 24, 8374. doi: 10.3390/ijms24098374
Szyszka-Mroz B., Ivanov A. G., Trick C. G., Hüner N. (2022). Palmelloid formation in the Antarctic psychrophile, Chlamydomonas priscuii, is photoprotective. Front. Plant Sci. 13, 911035. doi: 10.3389/fpls.2022.911035
Teufel A. G., Li W., Kiss A. J., Morgan-Kiss R. M. (2017). Impact of nitrogen and phosphorus on phytoplankton production and bacterial community structure in two stratified Antarctic lakes: a bioassay approach. Polar Biol. 40, 1007–1022. doi: 10.1007/s00300-016-2025-8
Trimborn S., Thoms S., Karitter P., Bischof K. (2019). Ocean acidification and high irradiance stimulate the photo-physiological fitness, growth and carbon production of the Antarctic cryptophyte Geminigera cryophila. Biogeosciences 16, 2997–3008. doi: 10.5194/bg-16-2997-2019
Turner J., Colwell S. R., Marshall G. J., Lachlan-Cope T. A., Carleton A. M., Jones P. D., et al. (2005). Antarctic climate change during the last 50 years. Int. J. Climatol. 25, 279–294. doi: 10.1002/(ISSN)1097-0088
Unrein F., Gasol J. M., Not F., Forn I., Massana R. (2014). Mixotrophic haptophytes are key bacterial grazers in oligotrophic coastal waters. ISME J. 8, 164–176. doi: 10.1038/ismej.2013.132
Vick-Majors T. J., Priscu J. C., Amaral-Zettler L. A. (2014). Modular community structure suggests metabolic plasticity during the transition to polar night in ice-covered Antarctic lakes. ISME J. 8, 778–789. doi: 10.1038/ismej.2013.190
Walsh J. E. (2009). A comparison of Arctic and Antarctic climate change, present and future. Antarctic Sci. 21, 179–188. doi: 10.1017/S0954102009001874
Keywords: protist, Antarctica, McMurdo Dry Valley lakes, phytoplankton, disturbance
Citation: Morgan-Kiss RM, Popson D, Pereira R, Dolhi-Binder J, Teufel A, Li W, Kalra I, Sherwell S, Reynebeau E and Takacs-Vesbach C (2024) Sentinel protist taxa of the McMurdo Dry Valley lakes, Antarctica: a review. Front. Ecol. Evol. 12:1323472. doi: 10.3389/fevo.2024.1323472
Received: 17 October 2023; Accepted: 19 February 2024;
Published: 05 March 2024.
Edited by:
Tássio Brito de Oliveira, Federal University of Paraíba, BrazilReviewed by:
Kazuhiro Yoshida, Saga University, JapanGenoveva Esteban, Bournemouth University, United Kingdom
Copyright © 2024 Morgan-Kiss, Popson, Pereira, Dolhi-Binder, Teufel, Li, Kalra, Sherwell, Reynebeau and Takacs-Vesbach. This is an open-access article distributed under the terms of the Creative Commons Attribution License (CC BY). The use, distribution or reproduction in other forums is permitted, provided the original author(s) and the copyright owner(s) are credited and that the original publication in this journal is cited, in accordance with accepted academic practice. No use, distribution or reproduction is permitted which does not comply with these terms.
*Correspondence: Rachael M. Morgan-Kiss, bW9yZ2FucjJAbWlhbWlvaC5lZHU=
†Present address: Amber Teufel, Procter & Gamble, Cincinnati, OH, United States Wei Li, Lawrence Livermore National Laboratory, Livermore, CA, United States Isha Kalra, Department of Biology, University of Southern California, Los Angeles, CA, United States