- 1National Institute of Amazônia Research, CODUT- Coordination of Land Use and Climate Change, Manaus, Brazil
- 2Department of Wetland Ecology, Institute of Geography and Geoecology, Karlsruhe Institute of Technology, Rastatt, Germany
- 3Escola Superior de Agricultura “Luiz de Queiroz”- University of São Paulo (USP), Piracicaba, Brazil
- 4Department of Plant Biology, Institute of Biology, Campinas, Brazil
- 5Department of Biogeochemical Processes (BGP), Max Planck Institute of Biogeochemistry, Jena, Germany
Introduction: Trees from flooded forests have to adjust their xylem hydraulic structure to face the annual flooding and the climatic conditions of the atmosphere. Usually, this adjustment of anatomical tissues in the tropics is driven by drought events inducing conservative behavior and can be recorded annually in tree rings. However, how the flood pulse and the climatic conditions influence the xylem hydraulic structure in floodplain trees is unknown.
Methods: To fill this gap, we explore if flooded periods and monthly climate variation affect the annual tree growth and xylem anatomy structure for the tree species Hydrochorea corymbosa (Fabaceae) from the várzea flooded forest in the Central Amazon. We developed a 41-year ring width chronology (1971–2018) and a 30-year time series of xylem anatomy parameters (1988–2018) as mean hydraulic vessel diameter (Dh), vessel frequency (VF), and parenchyma quantity (PQ). We correlated the series with monthly hydrological and climatic data.
Results: The hydrological regime did not correlate with annual tree growth in that species as we previously expected but showed correlations with the xylem anatomical structure. High flood levels during the end of the flooding period induced conservative patterns of the anatomical structure, with a negative correlation with Dh (rho June = −0.40, p < 0.05) and a positive correlation with the PQ (rho September/October = 0.42, p < 0.05). These responses show that these trees are responding to flooding similar to the tree responses to drought. Regarding the climatic variation, the annual tree growth showed a negative correlation with the vapor pressure deficit (VPD), after the second half of the flooded period with the strongest correlation happening during the non-flooded period (rho December = −0.66, p < 0. 01). These conservative patterns in tree behavior also happened when the maximum temperature negatively affected the vessel diameter (rho September = −0.42, p < 0.05).
Discussion: In that case, we recognized two different moments that the environment is inducing conservative patterns in the xylem structure of those trees: 1) increasing the flood levels and 2) the high evaporative demand during the non-flooded period. In this way, the intensification of the hydrological regime, as well as the strong drought conditions during the non-flooded periods, can be a risk for H. corymbosa in the Central Amazonian floodplains.
1 Introduction
The Amazon forests are experiencing hydroclimatic changes over the last decades. The recent environmental changes are showing a tendency to increase mean annual temperature (Marengo et al., 2018) and vapor pressure deficit driven by the last strong droughts (2005, 2010, 2015, and 2023) (Barkhordarian et al., 2019). At the same time, an increase in precipitation in the northwestern region (Gloor et al., 2013) resulted in a significant increase in the frequency and magnitude of the flood level in the Central Amazon region (Barichivich et al., 2018; Schöngart and Junk, 2020). In the Amazon várzea floodplain, the annual flood pulse of 10.2 m on average in the Central Amazon is the principal driving force shaping the forest community structure (Junk et al., 1989; Wittmann et al., 2006, 2010). Consequently, these strong climate and hydrological events are directly affecting the Central Amazon floodplain forests, where the trees are physiologically adapted to the hydrological regime of alternating the flooded (with anoxic soil conditions) and non-flooded phases (Parolin and Wittmann, 2010). As the evaporative demand from the atmosphere regulates the soil–plant–atmosphere continuum, these trees need to adjust physiologically the connections of roots and leaves to the soil and atmosphere during both phases (Parolin et al., 2010a; Olson et al., 2014; Cintra et al., 2019; Lambers and Oliveira, 2020; Park et al., 2021). In this scenario, it is necessary to understand how the Amazon trees are responding to hydrological and climatic changes in this peculiar forest ecosystem.
The Amazon floodplain forests are one of the biggest expanses of flood-pulsed environments worldwide (Junk et al., 2011). These forest ecosystems have an ecology driven by 3–7-month uninterrupted periods of inundation and waterlogging that induce anoxic conditions in the rhizosphere (Junk et al., 1989, 2010; Parolin et al., 2010a). To survive in the inundated period, trees have developed morphological, physiological, and phenological adaptations (Parolin et al., 2004; Ferreira et al., 2009; Piedade et al., 2010). For example, the flood pulse regulates the timing of phenological events (Parolin et al., 2004). During the first half of the inundated phase, many deciduous and evergreen trees induce cambial dormancy and leaf shedding (Schöngart et al., 2002; Parolin et al., 2010b), with leaf flush also occurring while trees are still inundated (Schöngart et al., 2002). This period with new leaves during the second half of the inundated phase stimulates photosynthetic rates and high sap flux (Parolin et al., 2008; Horna et al., 2010). Even though tree growth mainly occurs during the non-inundated phase (Schöngart et al., 2002), it is possible to observe vigorous metabolic activities during flooding (Parolin et al., 2004).
Trees adjust diameter growth and xylem anatomy according to environmental conditions (Mencuccini and Grace, 1995; White et al., 1998; Bryukhanova and Fonti, 2013; Venegas-González et al., 2015; Durgante et al., 2023; Alves et al., 2024). In non-flooded forests, when the climatic conditions are favorable for growth, trees develop more acquisitive xylem anatomy, such as larger vessel diameters and smaller amounts of axial parenchyma (Chave et al., 2009; Poorter et al., 2010; Klein et al., 2018a; Silva da Costa et al., 2020). In extreme situations like severe droughts, xylem anatomy exhibits more conservative features, producing smaller vessels and more parenchyma quantity (Baas et al., 2004; Pfautsch et al., 2016; Lourenço et al., 2022). Anatomical studies on historical series of growth rings have demonstrated that such xylem hydraulic adjustments can occur annually in response to environmental conditions (Fonti et al., 2010; Venegas-González et al., 2015) or can be a climate frequency-dependent signal (Fonti et al., 2009).
Trees face extreme abiotic conditions in floodplains and probably adjust the xylem hydraulic structure to survive the seasonal changes in water availability. In addition, climate extremes in the Amazon have been increasing for some decades, with more intense drought and flood events (Barichivich et al., 2018), creating further constraints for tree growth and survival. To understand how these trees adjust the xylem tissues to extreme conditions, this study evaluates how the growth rings and xylem anatomy (vessels and parenchyma) time series correlate with the variation of local hydrological and climatic factors in the common tree Hydrochorea corymbosa in the Central Amazon. This study aims to answer the following questions: I) Is the tree growth of H. corymbosa driven by hydrological and climatic factors? II) Are the wood anatomical tissues driven every year by the hydrological and climatic variability? We chose to use tree rings and anatomical dendrochronological series to understand the adjustment of the xylem in response to annual environmental factors, such as the flood pulse. Considering the flood pulse is the principal driver of tree growth, we expect the duration of the non-flooded period will drive acquisitive behavior in tree growth and xylem anatomic tissues. However, if the intensification of the hydrological cycle, the air temperature, and the vapor pressure deficit (VPD) from the last decades are affecting these trees, we expect these events to drive conservative behavior in the xylem tissues. In that case, determining how the flooded trees are facing the hydrological and climatic conditions will support knowledge to understand how this ecosystem is vulnerable to the intensification of hydroclimatic change.
2 Material and methods
2.1 Study area
Wood samples were collected from 30 trees located at the Catalão Lake (03°10′04″S and 59°54′45″W) of the municipality Iranduba, Amazonas state, Brazil, which is approximately 10 km from the city of Manaus (Figure 1). The area has a mean annual temperature of 26.7°C, with a slight seasonal variation (25.9°C to 27.7°C), and mean annual rainfall of 2,420 mm, and its driest month is August (Figure 2A). According to Köppen, the climate is classified as Af (Alvares et al., 2013). The lake is located at the confluence of the Negro (black-water) and Solimões (white-water) rivers. It receives water from both rivers but has a physical–chemical predominance of the waters of the Solimões throughout the year (de Brito et al., 2014). This flooded forest is characterized as “várzea” with nutrient-rich sediments from the Andes (Junk et al., 2011).
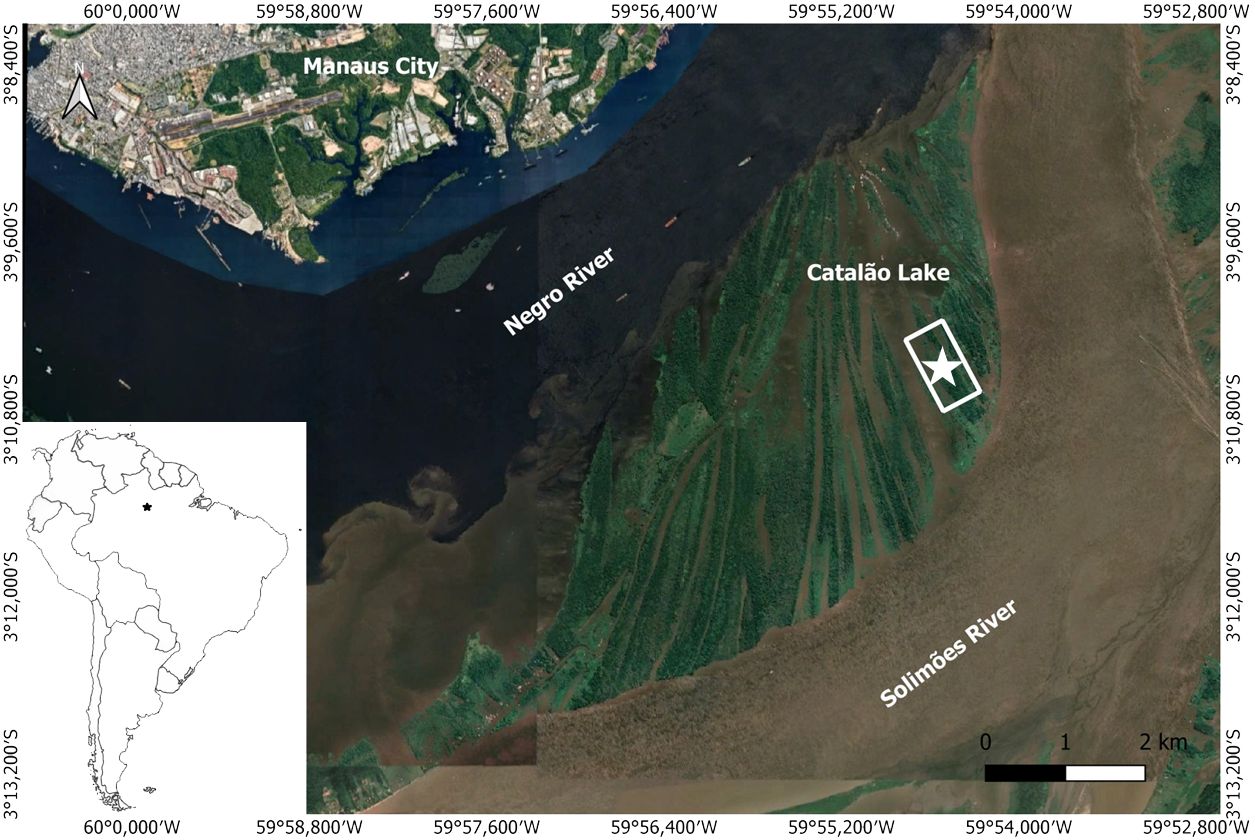
Figure 1 Research site map from Catalão Lake (Amazonas state), close to Manaus City in Brazil (Google Earth). The white rectangle and star represent the area where trees were sampled.
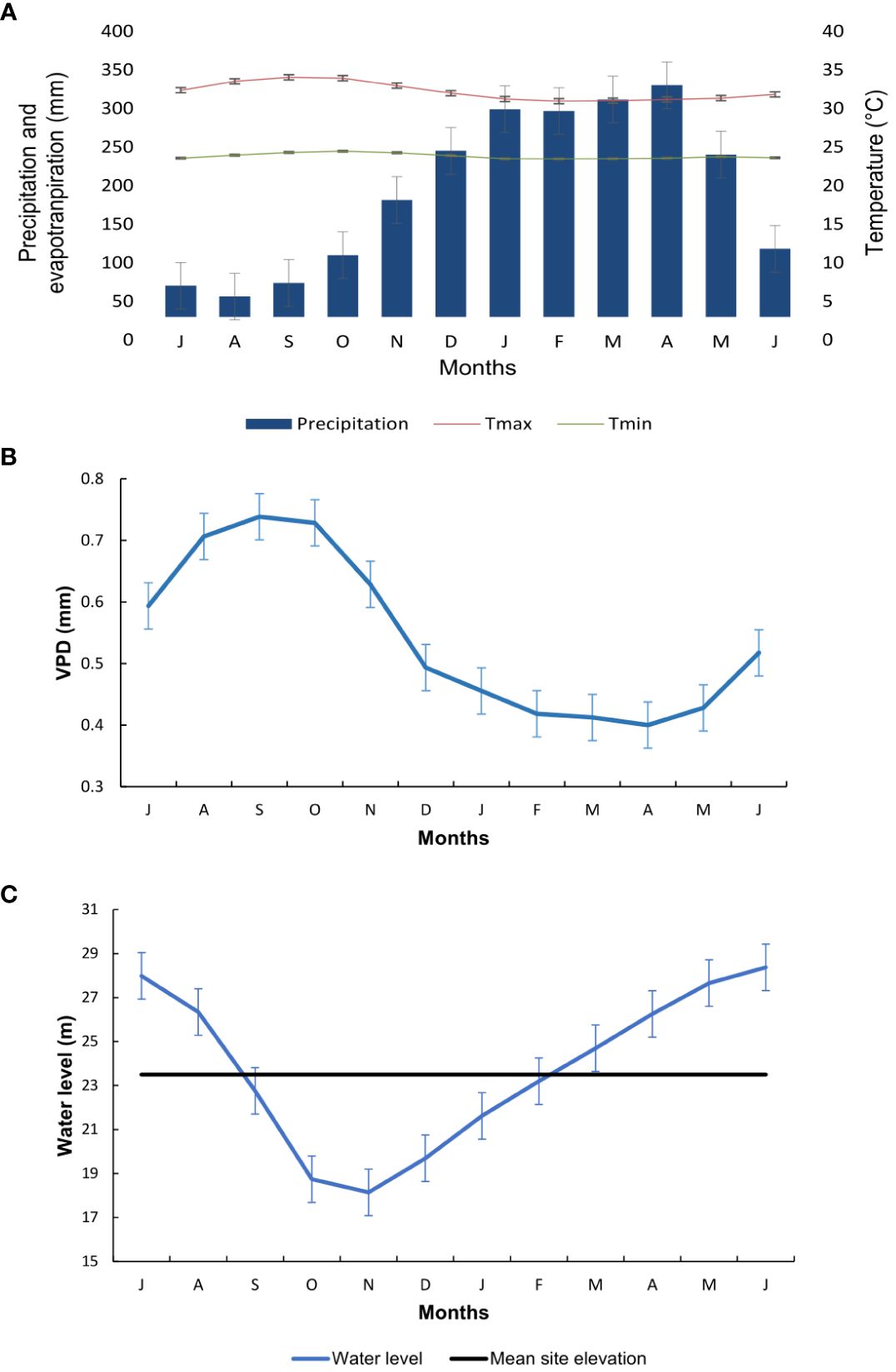
Figure 2 Average monthly climatic and hydrological conditions for the study region during the period 1988–2018, considering the hydrological year from July to June. (A) Precipitation (mm), potential evapotranspiration (mm), and maximum and minimum temperature (°C) from the Climatic Research Unit gridded (CRU-TS) (Harris et al., 2020) from Catalão Lake coordinates (−60.025780 W, −3.10719 S). (B) The mean annual vapor pressure deficit (kPa). (C) Mean monthly water level from National Water Resources Information System (SNIRH) and the mean topography of the collected trees at Catalão Lake. Maximum values were found in June (28.37 m) and minimum (18.14 m) in November.
The hydrological regime of the rivers is controlled by the monomodal and predictable flood pulse in Catalão Lake, as shown by the daily recorded water level of the Negro River at the Port of Manaus (Figure 2B). The average flood amplitude is 10.2 m, with the peak of the high waters in June (Schöngart and Junk, 2007). Várzea floodplain forests are subject to flooding up to an average of 230 days per year (Wittmann et al., 2002; Junk et al., 2011), but the number of flood days depends on the topographic location of each tree (Worbes et al., 1992).
2.2 The species H. corymbosa (Rich.) Barneby and J.W. Grimes
This species from the Fabaceae family is native to South America, occurring in Amazonian white-sand ecosystems (campinarana), savannas (cerrado), and episodically flooded riparian or gallery forests and the large Amazonian river floodplains (Koponen et al., 2003; Soares, 2020). Its wood has a cream color with no characteristic smell and a density of ≈0.65 g/cm³ (Horna et al., 2010; Parolin et al., 2010a; De Souza, 2012). The wood of H. corymbosa has an aliform paratracheal axial parenchyma of rhomboid aspect that forms small confluences. Fibers with calcium oxalate crystals border the axial parenchyma (Evans et al., 2006). Its vessel elements feature solitary vessels and, less frequently, two to four vessels forming multiple radials. Rays are uniseriate and unstoried (Evans et al., 2006). The naked eye quickly sees the paratracheal axial parenchyma, though the radial parenchyma is distinct only under magnification.
The leaf phenology described by Horna et al. (2010) and Schöngart et al. (2002) indicates that H. corymbosa is a deciduous species, with leaf fall at the beginning of the inundated phase in February (Supplementary Figure 1). The cambial phenology presents dormancy from March to June and a resumption of diametric growth in August after the peak of the high waters, lagging by approximately 1 month the flush of new leaves that starts in May (Schöngart et al., 2002). After the flushing of new leaves, there is an increase in cambial sap flow that reaches its maximum in the period July–August, when the diametric growth begins in August (Horna et al., 2010). This period is characterized by high air temperature and VPD, low precipitation, and a declining water level (Figure 2). During the non-flooded phase, radial increment rates and sap flux remain high, so the year of the growth ring was considered from August of the previous year until March of the following year (Schöngart et al., 2002) going to a dormancy period when the new growth ring begins. In the studies of Schöngart et al. (2002) and Horna et al. (2010), this species was identified as Albizia multiflora. Still, the correct identification of the species has now been updated (Schöngart, personal communication).
2.3 Sample collection and processing
In January 2019, two wood samples were collected from 30 individuals of H. corymbosa with 12-mm-diameter mechanized increment augers. In total, 62 samples were taken (two or more samples per tree) in the radial direction, preferably at an angle of 180° between them. The average collection height was 0.95 m above the ground. The trees were chosen according to the external health of the trunk, within the diametric class of 22.9 to 37.0 cm, and their diameter at breast height (DBH) was measured at a height of 1.30 m above the ground. Three readings were taken per tree of the size of the last flood, measuring the height of the watermarks on the trunks using a digital hypsometer (Haglof Vertex VL5). Among the individuals sampled, the flood ranged from 4 to 6 m above the soil during the highest water level before the year of collection, resulting in a mean of 219.6 ± 19.79 days of flooding.
The samples were fixed on wooden support using polyvinyl acetate (PVA) glue and adhesive tapes with the transverse face directed upward. These were labeled with a code and transported to the Dendroecology Laboratory of the MAUA Research Group (Ecology, Monitoring and Sustainable Use of Wetlands) at the Institute of Amazonian (INPA), where they were dried at room temperature. The transverse face of the samples was polished with an orbital sander using sandpaper of different grits (40 to 1,200) until it was possible to see their growth rings (Schöngart et al., 2004). With the aid of a magnifying glass (Leica MS5), the macroscopic description of the wood was performed according to Botosso (2011), and to access the annual information on xylem anatomy and growth, the tree rings were marked (Supplementary Figure 2).
2.4 Dendrochronological analysis
The growth rings of the 62 samples were identified as diffuse-porous, with their edges formed by increased wood density, as seen in Supplementary Figure 2. The growth-ring borders are made apparent by their difference in density, which, microscopically, is expressed by the difference in the width of the fiber walls (Supplementary Figure 3). At the beginning of the growth period, the fiber walls are thinner, and, at the end, they become thicker.
The ring width count and measurement, from the core to the bark, were made with a measuring device (LINTAB 5, Rinntech, Alemanha, Germany). The growth-ring curves were first visually cross-dated with the software TSAP-Win (Time Series Analyses and Presentation, Rinntech, Alemanha, Germany). The statistical cross-dating was performed using the software R, with the dplR package (Bunn, 2010). The cross-dated series were detrended using the exponential distribution model (“ModNegExp”), with a 15-year window, and the Rbar and EPS were calculated to evaluate the samples’ common variance and signal strength (Wigley et al., 1984; Cook et al., 2000). Only the samples with significant correlation (p < 0.05) were used for the final chronology.
2.5 Microscopic analysis of the xylem hydraulic architecture
From the growth-ring chronology, five exactly dated samples were selected (CH13, CH15, CH25, CH26, and CH28) for microscopic examination of the anatomical tissues in the Laboratory of Anatomy and Identification of Woods (ESALQ-USP, Piracicaba, SP, Brazil). This selection considered the cores to have a high correlation with the TRW master chronology and the structural quality of the material. Due to the analysis of microanatomy of each growth ring being slow and laborious, five individuals from this species were used as suggested in protocols (Klimešová et al., 2019) and used in previous studies (Bryukhanova and Fonti, 2013 and von Arx et al., 2013). The semi-permanent slides were prepared according to the methodology described by Pérez-de-Lis et al. (2018). The slides were digitally photographed with the aid of the microscope (Axio Scope A1), fitted with a camera (MRC AxioCam), 4× zoom, and processed using AxioVision software version 4.9.1 (Zeiss, Oberkochen, Germany), with the scale in micrometers to join frame by frame. The microscopic description of the transverse wood measurements was performed according to IAWA recommendations (IAWA Committee, 1989). The manual vectorization of xylem vessels and automatic vectorization of paratracheal axial parenchyma tissues were performed in the GIMP software (GIMP Development Team, Berkeley, CA, USA). The automatic measurement of the quantitative characteristics of the transverse wood was conducted in the ImageJ software (Pulido-Rodríguez et al., 2020). The following parameters were obtained: size (mm) and area of tree rings (mm2), number of vessels per specific area of the ring [vessel frequency (VF)], area of vessels per ring (mm2), the mean hydraulically diameter (mm) (Dh) (Equation 1) (Pandey et al., 2018), vessel area (mm2) (VA), and number of paratracheal axial parenchyma cells (mm2) [parenchyma quantity (PQ)]. These wood anatomy features were chosen, as they are important to trees’ hydraulic function (Lourenço et al., 2022). From this information, it was possible to determine xylem hydraulic architecture, which means the mean vessel diameter (mm), vessel frequency (number of vessel/mm2), and amount of paratracheal axial parenchyma cells (number of parenchyma cells/mm2). The mean of these variables was calculated for each sample and tree ring. Afterward, its anatomical character was detrended with a spline equation to avoid ontogenetic tendency (Carrer et al., 2015; Pandey et al., 2018).
where D is the vessel diameter (µm).
The species showed some significant morphology correlations. A weak correlation was observed between the Dh and DBH (rho = 0.29, p ≤ 0.01); PQ, VF, and VA showed no significant correlation with growth (Supplementary Figure 4). Strong correlations between the xylem variables Dh × PQ (rho = −0.78, p ≤ 0.01), Dh × VF (rho = −0.83, p ≤ 0.01), VF × VA (rho = −0.59, p ≤ 0.01), VF × PQ (rho = 0.81, p ≤ 0.01), and VA × PQ (rho = −0.80, p ≤ 0.01). Dh was used for analysis, as Dh and VA were strongly positively correlated.
2.6 Climatic and hydrological data
From 1988 to 2018, climatic and hydrological data were compiled from different sources. The climatic data, such as monthly precipitation (P) and minimum, maximum, and average monthly temperatures, were obtained from the Climatic Research Unit gridded (CRU-TS) (Harris et al., 2020) from Catalão Lake coordinates (−60.025780 W, −3.10719 S). The VPD (mm) was calculated using the equation described in the study of Sadler and Evans (1989) derived from the Penman–Monteith equation: saturation vapor pressure minus the actual vapor pressure equation (Equation 2). The data of the annual hydrological regime were calculated using the data of the daily water level at the Port of Manaus [National Water Resources Information System (SNIRH)]. The individual topography of each tree was calculated by the maximum watermark measured in the field and then subtracted from the top water level of that year. The duration of the non-inundated and inundated phases (days), the maximum flood height (m), the depth of the water table (m) defined by the average of the topography, and the minimum water level and the annual amplitude of the river (m) were determined annually for 1988–2018 period according to the daily water level records of the Manaus river gauge (SNIRH). The period considered the tree-ring year for calculating climatic variables was from July of the previous year to June of the following year (growth year), as defined for the ring by the cambium phenology of this species (Schöngart et al., 2002).
where VPD is vapor pressure deficit (kPa), MT is the mean temperature (°C), and RH is the relative humidity/100.
2.7 Statistical analysis
The historical series of Dh, VF, and PQ anatomical parameters for the five individuals (chosen within the dendrochronology samples) and the tree growth were correlated with the monthly climatic variables. We used the software R to conduct the Shapiro–Wilk test to evaluate a normal distribution of the data and the visual observation of the linearity between the variables. As most values do not present a parametric pattern, we used a non-parametric Spearman’s correlation to evaluate the relation between the variables (significance of p ≤ 0.05).
3 Results
3.1 Chronology
The chronology constructed for H. corymbosa was built on 17 trees and 21 radii and covered 41 years from 1977 to 2018 (Figure 3A). The inter-series correlation of this chronology was 0.38, Rbar was 0.35, and EPS was 0.90. The mean annual radial increment for this species was 3.83 ± 1.05 mm/year. The chronology had monthly negative correlations with VPD mainly during the peak of the aquatic phase (May–July) when xylogenesis initiates, being the strongest in December toward the end of the dry season (rho = −0.66, p = 0.0003) (Figures 3B, C). However, it showed no significant correlation with the other tested climatic and hydrological factors (flood phase, non-flood phase, flood height, minimum and maximum temperature, and precipitation).
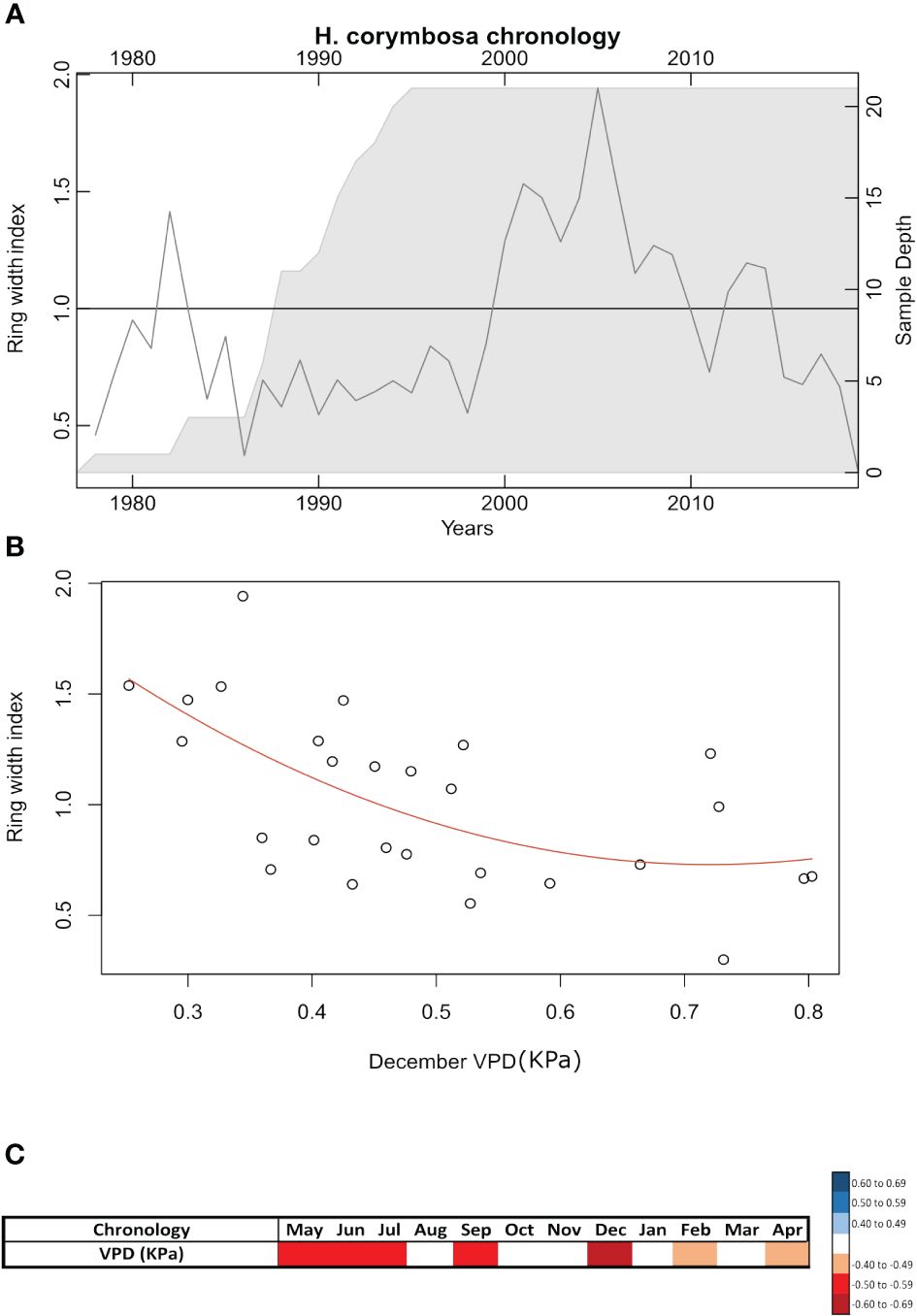
Figure 3 Correlation with climate parameters and the tree-ring chronology of Hydrochorea corymbosa at Catalão Lake site and (p < 0.05). (A) Ring-width index of the chronology based on 21 radii and 17 analyzed trees (1978–2018) with Rbar of 0.35 and EPS of 0.90. (B) Correlations (Spearman’s) between the annual ring-width index and corresponding vapor pressure deficit (VPD) (kPa) at the study site in December. (C) The monthly Spearman’s correlations between the ring-width index and the site vapor pressure deficit (VPD) (kPa).
3.2 Time series of xylem anatomy
The five trees analyzed showed a mean Dh of 174.02 ± 90.77 µm, a mean VF of 7.01 ± 6.48 vessels/mm2, and a mean PQ of 852.03 ± 1,107.65 cells/mm2. The detrended xylem anatomy data of H. corymbosa were correlated with monthly region climatic variables and showed weak correspondence. The vessel diameter (Dh) had a significant negative response to maximum temperature in March and September (rho = −0.41, p = 0.03, and rho = −0.42, p = 0.02) (Figures 4A, B) at the beginning and end of the inundation period, respectively. During the peak of flooding, the correlation was positive with precipitation in July (rho = 0.46, p = 0.01) (Figures 4A, B) and negative with the flood height (m) in June (r = −0.40, p = 0.03) (Figures 4A, B). The PQ showed negative responses to maximum temperature (°C) in July (r = −0.40, p = 0.03) (Figures 5A, B) and minimum temperature (°C) in May, July, August, and November, with the most significant in July (r = −0.47, p = 0.009) (Figures 5A, B). The PQ correlates negatively with VPD in August (dry season) (rho = −0.50, p = 0.009) (Figures 5A, B) and positively with the water level during the low-water period in September and October (rho = 0.42, p = 0.02; rho = 0.42, p = 0.02). The VF did not show a correlation with any of the tested climatic variables.
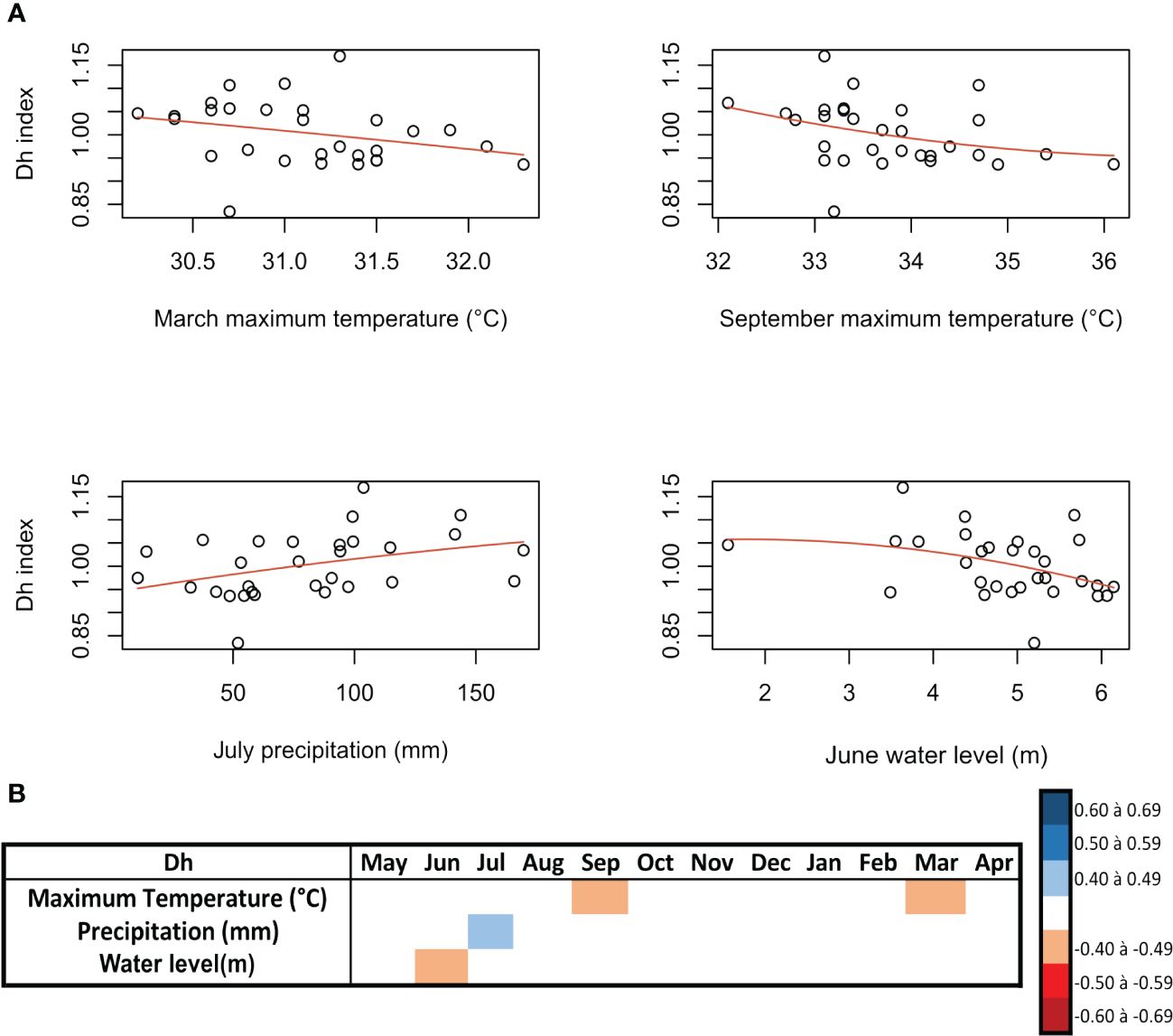
Figure 4 Spearman’s correlations between the indexed vessel diameter (Dh) series of the tree species Hydrochorea corymbosa from Central Amazonian floodplains and hydrological and climatic data (rho > 0.40, p < 0.05). (A) Correlation between the parenchyma quantity (PQ) index and the climatic data. (B) The monthly correlations between the PQ index and the site climatic data.
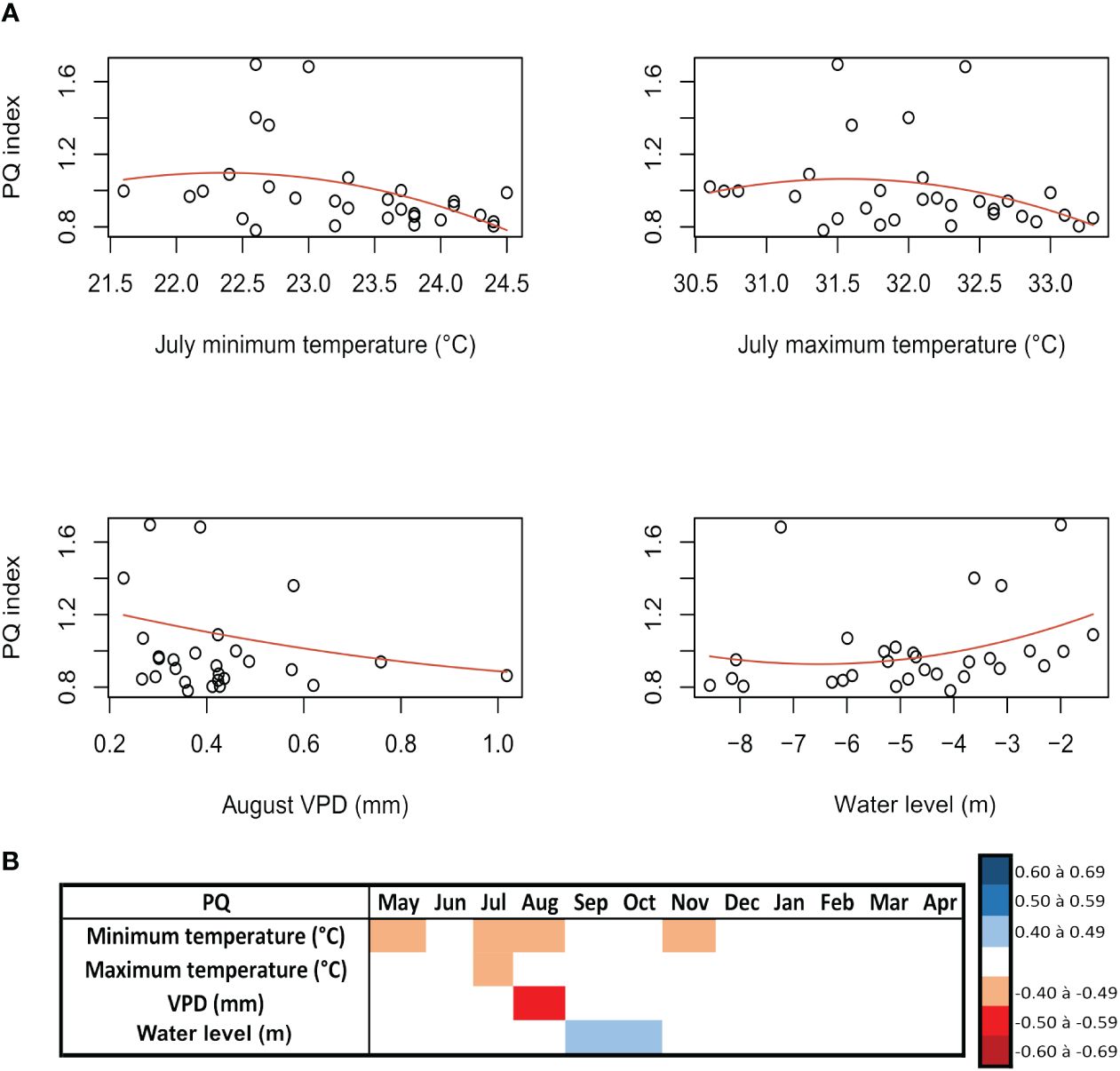
Figure 5 Spearman’s correlations between the indexed parenchyma quantity (PQ) series of Hydrochorea corymbosa at Catalão Lake and hydroclimatic data (rho > 0.40, p < 0.05). (A) Correlation between the PQ index and the climatic data. (B) The monthly correlations between the PQ index and the site climatic data.
4 Discussion
H. corymbosa showed distinct anatomical rings that allowed us to construct a robust chronology for this site of the Central Amazonian floodplain forest with similar parameters to other tropical chronologies (Schöngart et al., 2004, 2005, 2015; Buras, 2017; De Assis Pereira et al., 2018; Albiero-Júnior et al., 2019; Macedo et al., 2020; Gonçalves et al., 2021). The present tree-ring chronology offered a 41-year series of diameter increments and 30 years of wood anatomy that allowed us to recognize the hydrological and climate parameters driving the xylem behavior in these floodplain trees. The high flood level showed a correlation with the anatomical tissue (vessel diameter and parenchyma quantity), while tree growth correlated with VPD mainly during the dry season. Both the intensification of the hydrological regime and climatic drought condition (VPD) induced a conservative behavior in the xylem in these flooded trees, characterized by the Dh and growth reduction. These key results are important to recognize the influence of the climate events affecting the Central Amazon flooded trees during the last three decades.
The flood pulse is the main environmental factor triggering the phenology and diameter increment of trees in the Amazonian floodplain forest (Worbes, 1997; Schöngart et al., 2002, 2005). This factor allowed the recognition of climatic signals in tree-ring chronologies from floodplain forests. The long duration of the non-flooded period proportionate better growth conditions for trees of the species Piranhea trifoliata (Picrodendraceae) as well as other species of the floodplain (Dezzeo et al., 2003; Schöngart et al., 2004, 2005). However, the tree growth of H. corymbosa did not correlate with the hydrological regime, as we previously expected. We found that diameter increment negatively correlated with VPD during the second half of the flooded period and in the middle of the non-flooded period (December). The main growth phase of these trees (non-flooded period) happens between September and February coinciding with the beginning of the rainy season (November to May). In that case, these trees are adapted to precipitation supplying water during this vegetative phase. Nevertheless, if the drought events happen during the non-flooded period when the groundwater level is low, these trees can decrease growth as what happened to H. corymbosa responding to high VPD in December (rho = −0.60). A high VPD often causes stomatal closure (Zhao et al., 2013), and the loss of stomatal conductance implies a lower carbon uptake, reducing tree growth, and may even cause mortality (McDowell and Allen, 2015; McDowell et al., 2018). These results have the same direction that happened with Nectandra amazonum, where the diametric growth had a negative correlation with minimum temperature and evapotranspiration and did not correlate with the hydrological regime. These results add a new limiting factor triggering tree behaviors in Amazon floodplain forests (Gonçalves et al., 2021).
The tree physiology reacts to flooding such as a water limitation at drought events, decreasing tree growth and developing a xylem conservative behavior (Worbes, 1997). Our results from the anatomical tree-ring series corroborate this pattern. The high-water period has a negative correlation with Dh (vessel diameter) in June (apex of the flooded period), while the low-water regime has a positive correlation with PQ (parenchyma quantity) in September and October (end of the flooded period). In that case, the high flood causes hydraulic stress in vessels, decreasing the size, as what happens in hypoxia conditions when the root activity is affected (Haase and Rätsch, 2010; Parolin and Wittmann, 2010). Supporting these behaviors, the axial parenchyma tissue that has the functionality of retaining starch and non-structural carbohydrates (Kawai et al., 2022) is a conservative tissue to support stress conditions. Parenchyma cells could help in water acquisition by acting for osmoregulation (Hartmann and Trumbore, 2016), which could create a hypothesis of hydraulic protection. Both anatomical traits prove that the intensification and the extension of the hydrological regime are inducing the conservative xylem behavior in flooded H. corymbosa trees annually.
The Dh of H. corymbosa was larger than that found for other diffuse-porous species (Zimmermann et al., 2021; Lauriks et al., 2022). However, trees in this species could have other strategies for protecting their hydraulic tissues, like multiple vessels, which provide greater embolism safety, because the increase in hydraulic integration is associated with drier sites (von Arx et al., 2013; Rodríguez-Ramírez et al., 2023). This characteristic allows sap flux to continue even if some vessels are embolized (Zimmerman, 1983; Lens et al., 2011). Also, the axial parathracheal parenchyma could develop an essential role in hydraulic protection, as these cells could provide water and nutrient storage and help embolism recover (Fonti and Jansen, 2012; Klein et al., 2018; Kawai et al., 2022). The VF did not correlate with the climate, although the values found for VF are consistent with those of other studies with diffuse-porous trees.
The seasonal dynamics between terrestrial and aquatic phases that the floodplain forest experiences imply that trees tolerate water excess (anoxic conditions during flooding) and deficit (during the dry season of the non-flooded phase). The physiological dynamics driven by water level show different behaviors in the first half of the flooded period (leaf shedding), the second half of the flooded period (new flush leaves and diurnal sap flow driven by transpiration), and the terrestrial phase (favorable growth conditions). For example, H. corymbosa in the Central Amazon Várzea forest showed a deciduous behavior with leaf shedding between February and May, decreasing the physiological activities in the first half of the flooded period (Supplementary Figure 1). These low physiological activities induce the tree-ring formation limited during the aquatic phase with the cambial dormancy happening from March to May (Schöngart et al., 2002). Between May and June, the species add the new flux leaves. These young leaves activate the diurnal sap flow during the second half of the flooded period, after July (Horna et al., 2010). This sap flow continues during the terrestrial phase when the main growth period for these trees happens (August to February). In that case, the trees respond differently to the climate conditions during the year. The high air temperature and vapor pressure deficit can affect the tree behavior during the second half of the flooded period, as physiological activities such as sap flow driven by transpiration are activated after the flush leaves. At the same time, during the terrestrial phase (non-flooded period), the same climate variables (high air temperature and vapor pressure deficit) can decrease the growth conditions and induce conservative behavior as this is the main growth period for these trees. However, applying the active xylem equation developed by Horna et al. (2010) to our tree ring series (see Supplementary Material 5), we recognized on average five tree rings participating in the water conductance per year. In that case, as the vessel diameter and parenchyma tissue formation respond to specific hydrological and climatic conditions, we can assume that the tree species have high resilience to changing the hydroclimatic conditions, as water conductance is performed by tree rings with different setups of wood anatomical patterns.
In the present study, we recognized that the high vapor pressure deficit and the high water level have induced conservative behavior in the xylem structure for H. corymbosa trees during the last 30 years. Considering the climatic change tendency to increase the temperature, increase the vapor pressure deficit (Bauman et al., 2022; IPCC, 2022), and intensify the hydrological regime in the Amazon (Barichivich et al., 2018), our results are alarming regarding the protection of the flooded forest. The conservative behavior driven by climate and hydrological patterns suggests that these trees are stressed in both conditions. Our results indicate that these scenarios are decreasing the carbon absorption capacity in flooded trees. At the same time, as the active xylem is formed on average by five tree rings and these tree rings have been formed during contrasting hydrological extreme events (severe floods and extreme hydrological droughts), the active sapwood consists of a highly variable hydraulic architecture adapted to different hydroclimatic conditions, which increases its resilience against extreme events.
Data availability statement
The raw data supporting the conclusions of this article will be made available by the authors, without undue reservation.
Author contributions
PS: Conceptualization, Investigation, Methodology, Writing – original draft, Writing – review & editing. JS: Conceptualization, Formal analysis, Funding acquisition, Investigation, Methodology, Resources, Supervision, Writing – original draft, Writing – review & editing. FW: Funding acquisition, Investigation, Project administration, Resources, Writing – review & editing. MP: Writing – review & editing. MT-F: Writing – review & editing. RO: Writing – review & editing. VH: Writing – review & editing. PP: Writing – review & editing. FD: Conceptualization, Formal analysis, Investigation, Methodology, Writing – original draft, Writing – review & editing.
Funding
The author(s) declare financial support was received for the research, authorship, and/or publication of this article. A fellowship from CNPq supported this research, and the Botanica Post Graduation Program was supported by Capes (Coordenacao de Aperfeicoamento de pessoal de nível superior). The German–Brazilian project ATTO (Amazon Tall Tower Observatory) was supported by the German Federal Ministry of Education and Research (BMBF contracts 01LK1602A and 01LK1602F) and the Brazilian Ministério da Ciência Tecnologia e Inovação (MCTI/FINEP contract 01.11.01248.00). The Universal FAPEAM 006/2019 was supported by the KIT-Publication Fund from Karlsruhe Institute of Technology. The Brazilian National Council for Scientific and Technological Development (CNPq) was supported by the Long-term Ecological Research Program—PELD (MCTI/CNPq/FAPs; grant number 403792/2012–6), the Technical/Scientific Cooperation between INPA and Max-Planck Society for financial support, and the Wood Anatomy and Tree-Ring Laboratory (LAIM) (FAPESP; grant number 2009/50085–3) and Pire-project (FAPESP; grant number 2017/50085–3). We would also like to thank the CLAMBIO consortium and the BiodivERsA 2019– 2020 Joint COFUND Call on “Biodiversity and Climate Change” (German Federal Ministry of Education and Research - BMBF: 16LC2025A).
Acknowledgments
For field work and technical support, we thank Celso Rabelo Costa, Mario Luiz Picanço, Vitor Hugo Ferreira Andrade, Gildo Feitoza Vieira, Alberto Fialho, Angélica Faria de Resende, Maíra de Oliveira Macedo, Yuri Oliveira Feitosa, Anderson de Araújo Reis, and Luiz Santini Junior.
Conflict of interest
The authors declare that the research was conducted in the absence of any commercial or financial relationships that could be construed as a potential conflict of interest.
Publisher’s note
All claims expressed in this article are solely those of the authors and do not necessarily represent those of their affiliated organizations, or those of the publisher, the editors and the reviewers. Any product that may be evaluated in this article, or claim that may be made by its manufacturer, is not guaranteed or endorsed by the publisher.
Supplementary material
The Supplementary Material for this article can be found online at: https://www.frontiersin.org/articles/10.3389/fevo.2024.1292132/full#supplementary-material
References
Albiero-Júnior A., Venegas-González A., Botosso P. C., Roig F. A., Camargo J. L. C., Tomazello-Filho M. (2019). What is the temporal extension of edge effects on tree growth dynamics? A dendrochronological approach model using Scleronema micranthum (Ducke) Ducke trees of a fragmented forest in the Central Amazon. Ecol. Indic. 101, 133–142. doi: 10.1016/j.ecolind.2018.12.040
Alvares C. A., Stape J. L., Sentelhas P. C., De Moraes Gonçalves J. L., Sparovek G. (2013). Köppen’s climate classification map for Brazil. Meteorol. Z. 22, 711–728. doi: 10.1127/0941–2948/2013/0507
Alves Y. L. A., Durgante F. M., Piedade M. T. F., et al. (2024). Tree growth performance and xylem functional arrangements of Macrolobium Schreb. (Fabaceae) in different wetland forests in the Central Amazon basin. Trees 38, 115–126. doi: 10.1007/s00468-023-02469-3
Baas P., Ewers F. W., Davis S. D., Wheeler E. A. (2004). “Evolution of xylem physiology,” in The evolution of plant physiology. Eds. Hemsley A. R., Poole I.. (Elsevier Ltd), 273–295. doi: 10.1016/B978-012339552-8/50016-0
Barichivich J., Gloor E., Peylin P., Brienen R. J. W., Schöngart J., Espinoza J. C., et al. (2018). Recent intensification of Amazon flooding extremes driven by strengthened Walker circulation. Sci. Adv. 4, 1–7. doi: 10.1126/sciadv.aat8785
Barkhordarian A., Saatchi S. S., Behrangi A., Loikith P. C., Mechoso C. R. (2019). A recent systematic increase in vapor pressure deficit over tropical South America. Sci. Rep. 9, 1–12. doi: 10.1038/s41598-019-51857-8
Bauman D., Fortunel C., Delhaye G., Malhi Y., Cernusak L. A., Bentley L. P., et al. (2022). Tropical tree mortality has increased with rising atmospheric water stress. Nature 608, 528–533. doi: 10.1038/s41586-022-04737-7
Bryukhanova M., Fonti P. (2013). Xylem plasticity allows rapid hydraulic adjustment to annual climatic variability. Trees - Struct. Funct. 27, 485–496. doi: 10.1007/s00468-012-0802-8
Bunn A. G. (2010). Statistical and visual crossdating in R using the dplR library. Dendrochronologia 28, 251–258. doi: 10.1016/j.dendro.2009.12.001
Buras A. (2017). A comment on the expressed population signal. Dendrochronologia 44, 130–132. doi: 10.1016/j.dendro.2017.03.005
Carrer M., Von Arx G., Castagneri D., Petit G. (2015). Distilling allometric and environmental information from time series of conduit size: The standardization issue and its relationship to tree hydraulic architecture. Tree Physiol. 35, 27–33. doi: 10.1093/treephys/tpu108
Chave J., Coomes D., Jansen S., Lewis S. L., Swenson N. G., Zanne A. E. (2009). Towards a worldwide wood economics spectrum. Ecol. Lett. 12, 351–366. doi: 10.1111/j.1461-0248.2009.01285.x
Cintra B. B. L., Gloor M., Boom A., Schöngart J., Locosselli G. M., Brienen R. (2019). Contrasting controls on tree ring isotope variation for Amazon floodplain and terra firme trees. Tree Physiol. 39, 845–860. doi: 10.1093/treephys/tpz009
Cook E. R., Buckley B. M., D’Arrigo R. D., Peterson M. J. (2000). Warm-season temperatures since 1600 BC reconstructed from Tasmanian tree rings and their relationship to large-scale sea surface temperature anomalies. Clim. Dyn. 16, 79–91. doi: 10.1007/s003820050006
De Assis Pereira G., Barbosa A. C. M. C., Torbenson M. C. A., Stahle D. W., Granato-Souza D., Dos Santos R. M., et al. (2018). The climate response of cedrela fissilis annual ring width in the Rio São Francisco Basin, Brazil. Tree-Ring Res. 74, 162–171. doi: 10.3959/1536–1098-74.2.162
de Brito J. G., Alves L. F., Espirito Santo H. M. V. (2014). Seasonal and spatial variations in limnological conditions of a floodplain lake (Lake Catalão) connected to both the Solimões and Negro Rivers, Central Amazonia. Acta Amaz. 44, 121–133. doi: 10.1590/s0044–59672014000100012
Dezzeo N., Worbes M., Ishii I., Herrera R. (2003). Annual tree rings revealed by radiocarbon dating in seasonally flooded forest of the Mapire River, a tributary of the lower Orinoco River, Venezuela. Plant Ecol. 168, 165–175. doi: 10.1023/A:1024417610776
Durgante F. M., Higuchi N., Ohashi S., Householder J. E., Lima A. J. N., Ishizuka M., et al. (2023). Soil fertility and droughr interact to determine large variations in wood production for a hyperdominant Amazonian tree species. Front. For. Glob. Change 5, 1–11. doi: 10.3389/ffgc.2022.1065645
Evans J. A., Gasson P. E., Lewis G. P. (2006). Wood anatomy of the mimosoideae (Leguminosae). Richmond-UK: IAWA Publication. 27 (Suppl. 5), 1–117.
Ferreira C. D. S., Piedade M. T. F., Tiné M. A. S., Rossatto D. R., Parolin P., Buckeridge M. S. (2009). The role of carbohydrates in seed germination and seedling establishment of Himatanthus sucuuba, an Amazonian tree with populations adapted to flooded and non-flooded conditions. Ann. Bot. 104, 1111–1119. doi: 10.1093/aob/mcp212
Fonti P., Jansen S. (2012). Xylem plasticity in response to climate. New Phytol. 195, 734–736. doi: 10.1111/j.1469-8137.2012.04252.x
Fonti P., Treydte K., Osenstetter S., Frank D., Esper J. (2009). Frequency-dependent signals in multi-centennial oak vessel data. Palaeogeogr. Palaeoclimatol. Palaeoecol. 275, 92–99. doi: 10.1016/j.palaeo.2009.02.021
Fonti P., Von Arx G., García-González I., Eilmann B., Sass-Klaassen U., Gärtner H., et al. (2010). Studying global change through investigation of the plastic responses of xylem anatomy in tree rings. New Phytol. 185, 42–53. doi: 10.1111/j.1469-8137.2009.03030.x
Gloor M., Brienen, R. J. W., Galbraith D., Feldpausch T. R., Schöngart J., Guyot J.-L., et al. (2013). Intensification of the Amazon hydrological cycle over the last two decades. Geophys. Res. Lett 40, 1729–1733. doi: 10.1002/grl.50377
Gonçalves J. Q., Durgante F. M., Wittmann F., Piedade M. T. F., Ortega Rodriguez D. R., Tomazello-Filho M., et al. (2021). Minimum temperature and evapotranspiration in Central Amazonian floodplains limit tree growth of Nectandra amazonum (Lauraceae). Trees - Struct. Funct. 35, 1367–1384. doi: 10.1007/s00468-021-02126-7
Haase K., Rätsch G. (2010). “The morphology and anatomy of tree roots and their aeration strategies,” in Amazonian floodplain forests: ecophysiology, biodiversity and sustainable management. Eds. Junk W. J., Piedade M. T. F., Wittmann F., Schöngart J., Parolin P. (Berlin: Springer Verlag), 142–160.
Harris I., Osborn T. J., Jones P., Lister D. (2020). Version 4 of the CRUTS monthly high resolution gridded multivariate climate dataset. Sci. Data., 1–18. doi: 10.1038/s41597-020-0453-3
Hartmann H., Trumbore S. (2016). Understanding the roles of nonstructural carbohydrates in forest trees - from what we can measure to what we want to know. New Phytol. 211, 386–403. doi: 10.1111/nph.13955
Horna V., Zimmermann R., Müller E., Parolin P. (2010). “Sap flow and stem respiration,” in Amazonian floodplain forests: ecophysiology, biodiversity and sustainable management. Eds. Junk W. J., Piedade M. T. F., Wittmann F., Schöngart J., Parolin P. (Berlin: Springer Verlag), 223–242.
IAWA Committee. (1989). IAWA list of microscopic features for hardwood identification. International Association of Wood Anatomists Bulletin. 10, 219–332
IPCC. (2022). “Summary for policymakers,” in Climate change 2022: impacts, adaptation and vulnerability. Contribution of working group II to the sixth assessment report of the intergovernmental panel on climate change. Eds. Pörtner A. O. H.-O., Roberts D. C., Poloczanska E. S., Mintenbeck K., Tignor M., Alegría A., Craig M., Langsdorf S., Löschke S., Möller V. (Cambridge University Press, Cambridge, UK and New York, NY, USA), 3–33. doi: 10.1017/9781009325844.001
Junk W. J., Bayley P. B., Sparks R. E. (1989). Junk. Flood Pulse Concept River-Floodplain Syst. 7, 110–127. doi: 10.1007/BF02564079
Junk W. J., Piedade M. T. F., Schöngart J., Cohn-Haft M., Adeney J. M., Wittmann F. (2011). A classification of major naturally-occurring amazonian lowland wetlands. Wetlands 31, 623–640. doi: 10.1007/s13157-011-0190-7
Junk W. J., Piedade M. T. F., Wittmann F., Schöngart J., Parolin P. (2010). Amazonian floodplain forests: ecophysiology, biodiversity and sustainable management. (Berlin: Springer Verlag). doi: 10.1017/CBO9781107415324.004
Kawai K., Minagi K., Nakamura T., Saiki S. T., Yazaki K., Ishida A. (2022). Parenchyma underlies the interspecific variation of xylem hydraulics and carbon storage across 15 woody species on a subtropical island in Japan. Tree Physiol. 42, 337–350. doi: 10.1093/treephys/tpab100
Klein T., Zeppel M. J. B., Anderegg W. R. L., Bloemen J., De Kauwe M. G., Hudson P., et al. (2018). Xylem embolism refilling and resilience against drought-induced mortality in woody plants: processes and trade-offs. Ecol. Res. 33, 839–855. doi: 10.1007/s11284-018-1588-y
Klimešová J., Martínková J., Pausas J. G., de Moraes M. ,. G., Herben T., Yu F.-H., et al. (2019). Handbook of standardized protocols for collecting plant modularity traits. Perspect. Plant Ecol. Evol. Sys. 40, 125485. doi: 10.1016/j.ppees.2019.125485
Koponen P., Nygren P., Domenach A. M., Le Roux C., Saur E., Roggy J. C. (2003). Nodulation and dinitrogen fixation of legume trees in a tropical freshwater swamp forest in French Guiana. J. Trop. Ecol. 19, 655–666. doi: 10.1017/S0266467403006059
Lambers H., Oliveira R. S. (2020). Plant physiological ecology., 3rd ed. (Cham: Springer Nature Switzerland). doi: 10.2307/j.ctvzsmfh4
Lauriks F., Salomón R. L., de Roo L., Goossens W., Leroux O., Steppe K. (2022). Limited plasticity of anatomical and hydraulic traits in aspen trees under elevated CO2 and seasonal drought. Plant Physiol. 188, 268–284. doi: 10.1093/plphys/kiab497
Lens F., Sperry J. S., Christman M. A., Choat B., Rabaey D., Jansen S. (2011). Testing hypotheses that link wood anatomy to cavitation resistance and hydraulic conductivity in the genus Acer. New Phytol. 190, 709–723. doi: 10.1111/j.1469-8137.2010.03518.x
Lourenço J., Enquist B. J., von Arx G., Sonsin-Oliveira J., Morino K., Thomaz L. D., et al. (2022). Hydraulic tradeoffs underlie local variation in tropical forest functional diversity and sensitivity to drought. New Phytol. 234, 50–63. doi: 10.1111/nph.17944
Macedo T. M., Barros C. F., de Lima H. C., Brandes A. F., das N., da Costa W. S., et al. (2020). Climate signals in tree rings of Paubrasilia eChinata (Leguminosae-Caesalpinioidea) from the Atlantic Forest of Brazil. Trees - Struct. Funct. 34, 337–347. doi: 10.1007/s00468-019-01919-1
Marengo J. A., Alves L. M., Avala R. C. S., Cunha A. P., Brito S., Moraes O. L. L. (2018). Climatic characteristics of 2010-2016 drought in the semi-arid Northeast Brazil region. Ann. Brazilian Acad. Sci. 90 (2 Suppl. 1), 1973–1985. doi: 10.1590/0001-3765201720170206
McDowell N. G., Allen C. D. (2015). Darcy’s law predicts widespread forest mortality under climate warming. Nat. Clim. Change 5, 669–672. doi: 10.1038/nclimate2641
McDowell N., Allen C. D., Anderson-Teixeira K., Brando P., Brienen R., Chambers J., et al. (2018). Drivers and mechanisms of tree mortality in moist tropical forests. New Phytol. 219, 851–869. doi: 10.1111/nph.15027
Mencuccini M., Grace J. (1995). Climate influences the leaf area/sapwood area ratio in Scots pine. Tree Physiol. 15, 1–10. doi: 10.1093/treephys/15.1.1
Olson M. E., Anfodillo T., Rosell J. A., Petit G., Crivellaro A., Isnard S., et al. (2014). Universal hydraulics of the flowering plants: Vessel diameter scales with stem length across angiosperm lineages, habits and climates. Ecol. Lett. 17, 988–997. doi: 10.1111/ele.12302
Pandey S., Carrer M., Castagneri D., Petit G. (2018). Xylem anatomical responses to climate variability in Himalayan birch trees at one of the world’s highest forest limit. Perspect. Plant Ecol. Evol. Syst. 33, 34–41. doi: 10.1016/j.ppees.2018.05.004
Park J., Cho S., Moon M., Ryu D., Kim H. S. (2021). Developing stand transpiration model relating canopy conductance to stand sapwood area in a korean pine plantation. IForest 14, 186–194. doi: 10.3832/ifor3291-014
Parolin P., De Simone O., Haase K., Waldhoff D., Rottenberger S., Kuhn U., et al. (2004). Central amazonian floodplain forests: tree adaptations in a pulsing system. Bot. Rev. 70, 357–380. doi: 10.1663/0006-8101(2004)070[0357:CAFFTA]2.0.CO;2
Parolin P., Lucas C., Piedade M. T. F., Wittmann F. (2010b). Drought responses of flood-tolerant trees in Amazonian floodplains. Ann. Bot. 105, 129–139. doi: 10.1093/aob/mcp258
Parolin P., Müller E., Junk W. J. (2008). Sapwood area in seven common tree species of central amazon floodplains. Pesqui. Botânica 59, 277–286.
Parolin P., Wittmann F. (2010). Struggle in the flood: tree responses to flooding stress in four tropical floodplain systems. AoB Plants 2010, 1–19. doi: 10.1093/aobpla/plq003
Parolin P., Wittmann F., Schöngart J. (2010a). “Tree phenology in amazonian floodplain forests,” in Amazonian floodplain forests: ecophysiology, biodiversity and sustainable management. Eds. Junk W. J., Piedade M. T. F., Wittmann F., Schöngart J., Parolin P., 105–126.
Pérez-de-Lis G., Rozas V., Vázquez-Ruiz J. D., Gárcia- González I. (2018). Do ring-porous oaks prioritize earlywood vessel efficiency over safety? Environmental effects on vessel diameter and tyloses formation. Agric. For. Meteorol. 248, 205–214. doi: 10.1016/j.agrformet.2017.09.022
Pfautsch S., Harbusch M., Wesolowski A., Smith R., Macfarlane C., Tjoelker M. G., et al. (2016). Climate determines vascular traits in the ecologically diverse genus Eucalyptus. Ecol. Lett. 19, 240–248. doi: 10.1111/ele.12559
Piedade M. T. F., Ferreira C. S., Oliveira Wittmann A., Buckeridge M., Parolin P. (2010). “Biochemistry of amazonian floodplain trees,” in Amazonian floodplain forests: ecophysiology, biodiversity and sustainable management. Eds. Junk W. J., Piedade M. T. F., Wittmann F., Schöngart J., Parolin P. (Springer, Dordrecht), 127–139.
Poorter L., McDonald I., Alarcón A., Fichtler E., Licona J. C., Peña-Claros M., et al. (2010). The importance of wood traits and hydraulic conductance for the performance and life history strategies of 42 rainforest tree species. New Phytol. 185, 481–492. doi: 10.1111/j.1469-8137.2009.03092.x
Pulido-Rodríguez E., López-Camacho R., Tórres J., Velasco E., Salgado-Negret B. (2020). Traits and trade-offs of wood anatomy between trunks and branches in tropical dry forest species. Trees - Struct. Funct. 34, 497–505. doi: 10.1007/s00468-019-01931-5
Rodríguez-Ramírez E. C., Crispin-Delacruz D. B., Morales M. S., Ticse-Otarola G. A., Ames-Martínez F. N., Guerra A., et al. (2023). Exploring monsoon precipitation signal in the Peruvian tropical montane cloud forest: Andean Walnut wood anatomy plasticity. IAWA J. 17, 1–19. doi: 10.1163/22941932-bja10132
Sadler E. J., Evans D. E. (1989). Vapor pressure deficit calculations and their effect on the combination equation. Agric. For. Meteorol. 49, 55–80. doi: 10.1016/0168-1923(89)90062-2
Schöngart J., Gribel R., Ferreira da Fonseca-Junior S., Haugaasen T. (2015). Age and growth patterns of Brazil nut trees (Bertholletia excelsa bonpl.) in amazonia, Brazil. Biotropica 47, 550–558. doi: 10.1111/btp.12243
Schöngart J., Junk W. J. (2007). Forecasting the flood-pulse in central amazonia by ENSO-indices. J. Hydrol. 335, 124–132. doi: 10.1016/j.jhydrol.2006.11.005
Schöngart J., Junk W. J. (2020). “Clima e hidrologia nas várzeas da Amazônia Central,” in Várzeas Amazônicas: Desafios para um Manejo Sustentável. Eds. Junk W. J., Piedade M. T. F., Wittmann F. (INPA, Manaus), 44–65. J. S.
Schöngart J., Junk W. J., Piedade M. T. F., Ayres J. M., Hüttermann A., Worbes M. (2004). Teleconnection between tree growth in the Amazonian floodplains and the El Niño-Southern Oscillation effect. Glob. Change Biol. 10, 683–692. doi: 10.1111/j.1529–8817.2003.00754.x
Schöngart J., Piedade M. T. F., Ludwigshausen S., Horna V., Worbes M. (2002). Phenology and stem-growth periodicity of tree species in Amazonian floodplain forests. J. Trop. Ecol. 18, 581–597. doi: 10.1017/S0266467402002389
Schöngart J., Piedade M. T. F., Wittmann F., Junk W. J., Worbes M. (2005). Wood growth patterns of Macrolobium acaciifolium (Benth.) Benth. (Fabaceae) in Amazonian black-water and white-water floodplain forests. Oecologia 145, 454–461. doi: 10.1007/s00442-005-0147-8
Silva da Costa W., Da Cunha M., José F. Pena Rodrigues P., de Andrade Iguatemy M., Valladares F., Franca Barros C. (2020). Intraspecific variation in functional wood anatomy of tropical trees caused by effects of forest edge. For. Ecol. Manage. 473, 118305. doi: 10.1016/j.foreco.2020.118305
Soares M. V. B. (2020). Hydrochorea in Flora do Brasil 2020. Jardim Botânico do Rio de Janeiro. Available at: https://floradobrasil2020.jbrj.gov.br/FB22970.
Venegas-González A., von Arx G., Chagas M. P., Filho M. T. (2015). Plasticity in xylem anatomical traits of two tropical species in response to intra-seasonal climate variability. Trees - Struct. Funct. 29, 423–435. doi: 10.1007/s00468-014-1121-z
von Arx G., Kueffer C., Fonti P. (2013). Quantifying plasticity in vessel grouping - Added value from the image analysis tool ROXAS. IAWA J. 34, 433–445. doi: 10.1163/22941932–00000035
White D., Beadle C., Worledge D., Honeysett J., Cherry M. (1998). The influence of drought on the relationship between leaf and conducting sapwood area in Eucalyptus globulus and Eucalyptus nitens. Trees - Struct. Funct. 12, 406–414. doi: 10.1007/PL00009724
Wigley T. M. L., Briffa K. R., Jones P. D. (1984). On the average value of correlated time series with applications in dendroclimatology and hydrometeorology. Am. Meteoroligal Soc 23, 201–213. doi: 10.1175/1520-0450
Wittmann F., Anhuf D., Funk W. J. (2002). Tree species distribution and community structure of central Amazonian várzea forests by remote-sensing techniques. J. Trop. Ecol. 18, 805–820. doi: 10.1017/S0266467402002523
Wittmann F., Schöngart J., Junk W. J. (2010). “Phytogeography, species diversity, community structure and dynamics of central amazonian floodplain forests,” in Amazonian floodplain forests. Eds. Junk W. J., Piedade M. T. F., Wittmann F., Schöngart J., Parolin P. (Berlin: Springer Verlag), 61–104.
Wittmann F., Schöngart J., Montero J. C., Motzer T., Junk W. J., Piedade M. T. F., et al. (2006). Tree species composition and diversity gradients in white-water forests across the Amazon Basin. J. Biogeogr. 33, 1334–1347. doi: 10.1111/j.1365-2699.2006.01495.x
Worbes M. (1997). “11 the forest ecosystem of the floodplain,” in The central amazon floodplain: ecology of a pulsing system. Ed. Junk W. J. (Berlin: Springer), 223–265.
Worbes M., Klinge H., Revilla J. D., Martius C. (1992). On the dynamics, floristic subdivision and geographical distribution of várzea forests in Central Amazonia. J. Veg. Sci. 3, 553–564. doi: 10.2307/3235812
Zhao J., Hartmann H., Trumbore S., Ziegler W., Zhang Y. (2013). High temperature causes negative whole-plant carbon balance under mild drought. New Phytol. 200, 330–339. doi: 10.1111/nph.12400
Zimmerman M. H. (1983). Xylem structure and the ascent of sap. Ed. Timell T. E. (Berlin Heidelberg: Springer Verlag).
Zimmermann J., Link R. M., Hauck M., Leuschner C., Schuldt B. (2021). 60-year record of stem xylem anatomy and related hydraulic modification under increased summer drought in ring- and diffuse-porous temperate broad-leaved tree species. Trees - Struct. Funct. 35, 919–937. doi: 10.1007/s00468-021-02090-2
Keywords: dendrochronology, wood anatomy, climatic variations, floodplain forest, xylem plasticity
Citation: de Sá PA, Schöngart J, Wittmann F, Piedade MTF, Tomazello-Filho M, Oliveira RS, Horna V, Parolin P and Durgante FM (2024) Hydrological and climate intensification induces conservative behavior in the Hydrochorea corymbosa xylem production in a Central Amazon floodplain forest. Front. Ecol. Evol. 12:1292132. doi: 10.3389/fevo.2024.1292132
Received: 11 September 2023; Accepted: 13 May 2024;
Published: 03 June 2024.
Edited by:
Weiwei Huang, Nanjing Forestry University, ChinaReviewed by:
Junzhou Zhang, Lanzhou University, ChinaPeter Petrik, Global Change Research Centre (ASCR), Czechia
Copyright © 2024 de Sá, Schöngart, Wittmann, Piedade, Tomazello-Filho, Oliveira, Horna, Parolin and Durgante. This is an open-access article distributed under the terms of the Creative Commons Attribution License (CC BY). The use, distribution or reproduction in other forums is permitted, provided the original author(s) and the copyright owner(s) are credited and that the original publication in this journal is cited, in accordance with accepted academic practice. No use, distribution or reproduction is permitted which does not comply with these terms.
*Correspondence: Priscila Amaral de Sá, priscilaasa@yahoo.com.br; Flavia Machado Durgante, flavia.durgante@kit.edu