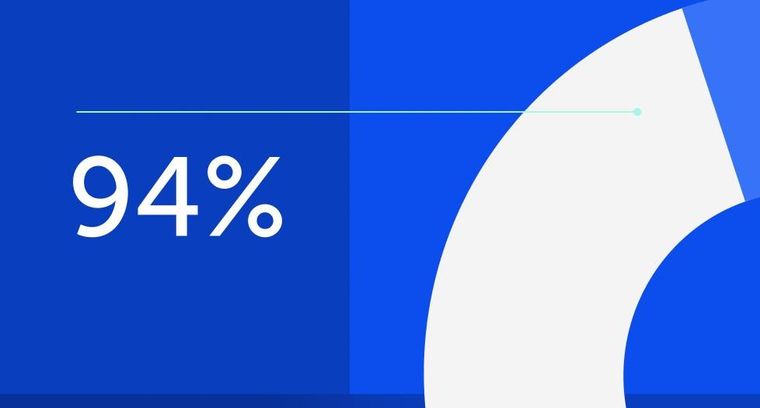
94% of researchers rate our articles as excellent or good
Learn more about the work of our research integrity team to safeguard the quality of each article we publish.
Find out more
ORIGINAL RESEARCH article
Front. Ecol. Evol., 27 February 2024
Sec. Biogeography and Macroecology
Volume 12 - 2024 | https://doi.org/10.3389/fevo.2024.1182021
This article is part of the Research TopicInterpretation and Implications of Variability in Ecological SystemsView all 13 articles
Climate-driven shifts in the natural flow regime can threaten species persistence in stream systems, and anticipating such shifts before they occur is critical for conservation. We can explore how climate change may impact biota by examining natural systems that differ in terms of climate yet are similar in terms of other landscape features such as geology, size, and elevation. Across an established precipitation and hydrologic gradient on the east coast of Hawaii Island, we sampled stream habitat and populations of the endemic migratory mountain shrimp Atyoida bisulcata over three years and examined how habitat as well as population metrics and individual condition respond to differences in stream flow. Along the precipitation gradient, baseflow declined and streams shifted from run/riffle systems with moss cover to those with predominately pools and limited available habitat. Across years, baseflow conditions were relatively consistent within streams while measures of stream flow stability and the duration of high flows were more variable. Streams with high and persistent baseflow had greater atyid biomass density with larger individuals less prone to disease. Within-stream interannual variation in baseflow was low relative to differences across streams, and most A. bisulcata metrics also had low within-stream interannual variability, making average baseflow an appropriate surrogate for differences in suitability. However, extremes in annual rainfall may result in high variability in A. bisulcata metrics within a single stream due to seasonal drying or persistent high flows, highlighting the importance of long-term monitoring to fully understand population responses to climate-mediated stream flow. Our study suggests that changes in rainfall patterns will alter stream flow and may ultimately negatively influence tropical stream organisms.
Aspects of stream flow influence aquatic biota by shaping the availability and quality of habitat (Poff et al., 1997). Many stream organisms are adapted to characteristics of natural flow regimes, and changes in flow outside the existing range of variability can have negative ecological consequences. Reductions in flow magnitude and increases in duration or frequency of low-flow events can, for example, reduce physical habitat and alter other physico-chemical conditions. Such changes can stress organisms and limit growth (Rolls et al., 2012) or result in unsuitable habitat (Canton et al., 1984). High flows can also act as a trigger for spawning or larval hatching (Naesje et al., 1995; Lytle and Poff, 2004), and changes in the magnitude or timing of high flow can negatively impact reproductive success. Conversely, increased frequency of floods can result in a temporary loss of species not adapted to high flows (e.g., Meffe and Minckley, 1987). The rate of change of stream flow can also affect organisms, stranding individuals in suboptimal habitats following quick flow recessions or flushing them downstream during freshets (e.g., Cushman, 1985; Bradford, 1997; Davey et al., 2006). Understanding what aspects of the stream flow regime influence species or populations is critical for their conservation, especially given anticipated impacts of climate change on stream flow via changes in rainfall timing and magnitude.
Alterations to the natural stream flow regime are a primary pathway through which climate change impacts stream species. For example, warmer air temperatures have increased winter and spring discharges and reduced summer flows in snowmelt-driven streams of the western United States (Leppi et al., 2012; Zeigler et al., 2012), limiting habitat for at-risk species like cutthroat trout (Isaak et al., 2012). In the Pacific Northwest, more frequent rainstorms and greater winter flow variability have been linked to reduced Chinook salmon reproduction (Ward et al., 2015). In the Rio Grande, warmer air temperatures have reduced spring discharges and increased interspecific competition among early life stages of stream fishes (Krabbenhoft et al., 2014). Collectively, such studies show clear widespread change that can inform future conservation action, but knowledge is missing for many regions and species. For example, documented effects of climate change on freshwater species are limited in tropical low-latitude ecosystems despite tropical species having potentially lower adaptive capacity (Comte et al., 2013; Myers et al., 2017; Krabbenhoft et al., 2020).
The lack of information on climate change impacts on tropical stream organisms is problematic for several reasons. First, seasonally- and regionally-specific changes in the timing and magnitude of rainfall are projected throughout the tropics (IPCC, 2013), altering flow regimes and threatening endemic species (Leong et al., 2014). Organisms that inhabit tropical island streams may be particularly vulnerable to such changes, as many streams require substantial rainfall to maintain baseflow (Craig, 2003). Additionally, many endemic tropical island stream species complete amphidromous life histories where larvae hatch in streams and drift downstream to the marine environment before migrating back upstream after the larval stage (Keith, 2003; Nishimoto and Fitzsimons, 2006; McDowall, 2007; Bauer, 2013). Reductions in streamflow can reduce or stall larval drift and slow or stop juvenile migration upstream (Gorbach et al., 2012; Walter et al., 2012; Leong et al., 2014). Further, stream flow reductions may reduce individual condition and growth by changing food availability (James et al., 2007). Thus, climate-driven changes in stream flow within tropical island systems could negatively impact populations.
The Hawaiian Islands are home to an endemic assemblage of amphidromous fish, shrimp and snails that are potentially threatened by climate change, including the atyid shrimp Atyoida bisulcata. Atyoida bisulcata can traverse most waterfalls and are found in many headwater streams, but they have been negatively impacted by widespread flow fragmentation and landscape degradation (Brasher, 2003). Climate-driven changes in flow are expected to further threaten these organisms through several mechanistic pathways. Like other amphidromous species, Atyoida bisulcata exist as a series of local populations within a larger metapopulation connected by mixing during the marine larval stage (Chubb et al., 1998; McRae, 2007). Reductions in baseflow may eliminate suitable juvenile and adult stream habitat and lower the likelihood of larval transport to the marine environment, making formally productive streams unsuitable. Sub-lethal effects also have the potential to influence individual condition and ultimately reproductive capacity. For example, lower baseflow and associated reductions in fine particulate organic matter (FPOM) as well as shifts from riffle/run to pool-dominated systems may reduce foraging opportunities for Atyoida bisulcata, which select for high velocity areas to filter feed and graze (Couret, 1976; Brasher, 1997). Diseases associated with less suitable habitats may also become more common, such as the chitinoclastic bacterial infection “brown spot disease” that is hypothesized to be the result of increased abrasion on Atyoida bisulcata exoskeletons in turbid high flow environments (Chan, 1978). Paired with climate change projections, increasing our understanding of the specific impacts of shifts in the stream flow regime on Atyoida bisulcata can improve our ability to project how individual local populations will be impacted in the upcoming decades.
The goal of our study was to examine how changes in the stream flow regime associated with lower rainfall influence Atyoida bisulcata. Using an established hydrologic and rainfall gradient, we tested the hypothesis that lower baseflow reduces Atyoida bisulcata habitat, population size, and individual condition. We predicted that lower baseflow would be associated with limited suitable habitat, lower population size and decreased individual condition. Our results can provide insight into the impact of climate change on runoff-driven stream ecosystems of Hawaii.
Our study area occurs across the North Hilo coast of Hawaii Island where over a linear distance of less than 30 km, mean annual rainfall ranges from approximately 3,000 to 7,000 mm/year (Giambelluca et al., 2013; Figure 1). We evaluated 11 first- and second-order stream reaches between 400 and 510 m in elevation and bounded by waterfalls (Strauch et al., 2015). Study area catchments have minimal urban and agricultural land use, are composed primarily of mixed native and non-native forests (Price et al., 2012) and drain land on porous volcanic geology (Sherrod et al., 2008). Stream reaches at this elevation are inhabited by A. bisulcata and are above barrier waterfalls for most other amphidromous species (Tingley et al., 2019). Previous research in this study system indicates that stream flow is heavily dependent on precipitation, with baseflow positively correlated and flow variability negatively correlated with effective mean annual rainfall (rainfall below catchment elevations of 1,830 m; Strauch et al., 2015; Frauendorf et al., 2020). In turn, lower baseflow has been shown to reduce the quantity of FPOM available and the quality of food resources in a subset of these streams (i.e., shifts to leaf litter dominated systems; Frauendorf et al., 2019, 2020).
Figure 1 North Hilo coast of Hawaii Island and locations of study reaches along a gradient in mean annual rainfall. Study stream names are adjacent to their respective watersheds.
We obtained daily stream flow records for 2012, 2013, and 2014 water years from multiple sources. For a single study reach we downloaded data from the USGS National Water Information System (https://waterdata.usgs.gov/usa/nwis/16717000). For all other reaches, we averaged daily stream flow from 15-min interval instantaneous data collected at gaging stations installed between spring of 2011 and fall of 2012 (Strauch et al., 2015). In three study reaches we supplemented gaps in daily stream flow using a Distributed Hydrology Soil Vegetation Model for the North Hilo coast (DHSVM; Strauch et al., 2016).
We conducted a multi-step process to select a small number of minimally redundant flow metrics that vary across the study region and may influence available habitat and A. bisulcata populations. We first calculated all flow metrics available from the USGS Hydrologic Index Tool (HIT; Henriksen et al., 2006) for the seven-month period (October – April) prior to the sample season (May-September). Next, we selected seven HIT metrics, one to two that were minimally redundant within each aspect of the flow regime (i.e., magnitude, duration, timing, frequency, rate of change; Poff et al., 1997), that are relevant to the flashy streams along the North Hilo coast (Supplementary Material Tables S1, S2). Metrics describing frequency of flow events included measures of low flow (total number of flow events below 5% of mean flow) and high flow (total number of flow events greater than the 25th percentile exceedance). Duration metrics included the mean length in days of high flows and the minimum 7-day mean flow normalized by effective catchment area. Timing was represented by the constancy of flow, or the stability in day-to-day stream flows over the study period, while rate of change was represented by the average rise rate of days with increased flow normalized by catchment area. Metrics describing flow magnitude included the coefficient of variability in daily flow and an additional metric calculated independently of the HIT tool; the 70th percentile exceedance value. Oki et al. (2010) found that the 70th percentile exceedance value is broadly representative of baseflow conditions in Hawaiian streams and we normalized it by effective catchment area to characterize potential differences across our study reaches.
Next, we used principal components analysis (PCA) to determine whether composite variables were useful for describing aspects of the flow regime across streams and years. The 70th percentile exceedance value (hereafter Q70Y) and the minimum 7-day mean flow (hereafter DLY) were log-transformed to improve linearity, and all flow variables were centered and scaled. We performed a varimax rotation to increase interpretability, with variable loadings >0.6 on the rotated axes considered to be heavily weighted and representative of the principal component (PC). The first three PCs accounted for 84% of the total variation in the dataset and were retained for interpretation (Supplementary Material Table S3). The constancy of stream flow was positively associated with the first principal component (PC1; 51% variance explained) while rise rate normalized by catchment area, the frequency low flow events and the coefficient of variation in flow were negatively associated with the axis, suggestive of a gradient in flow stability ranging from stable conditions with few extreme flow events to flashy systems with many low flows events. Log-transformed DLY and Q70Y were strongly associated with PC2 (23% variance explained) while the duration of high flows (hereafter DH) and frequency of high flows were strongly associated with the third PC (10% variation explained). Given the results of the PCA, we chose to include three metrics that broadly reflect differences in the stream flow regime in further analysis: the composite PC representative of flow stability (PC1), Q70Y and DH. We chose to use the flow metrics Q70Y and DH because they contributed little to PC1 but were one of two metrics associated with the second and third PC, respectively. As expected, log-transformed Q70Y had a strong positive correlation with mean annual rainfall in each study season (r= 0.88, 0.93, 0.84), while DH (r = 0.20, 0.38, -0.33) and PC1 (r=-0.04, 0.05, 0.56) were variable in strength and direction of correlation (Supplementary Material Figure S1). PC1 had the lowest values (suggestive of unstable flow regimes) in streams with moderate levels of mean annual rainfall while DH did not show a clear relationship with mean annual rainfall (Supplementary Material Figure S1).
We established study reach lengths equal to 20 times mean stream width (MSW) to capture a representative sample of habitat and biology (Kido, 2008, 2013). Transects lines were established once every MSW over study reaches. In a few instances, we truncated study reaches by up to 2 MSW due to limited length between unsurpassable upstream and downstream waterfalls.
In all sample years we estimated percent substrate, geomorphic units and channel dimensions to understand how habitat quality and quantity changes with stream flow and to infer potential drivers associated with changes in A. bisulcata metrics. Moving upstream, two researchers recorded the percent ( ± 5%) of substrate categories comprising the stream reach including silt, sand, gravel, cobble, boulder and bedrock (following Higashi and Nishimoto, 2007). Mean substrate size was calculated by multiplying the average percent substrate within a reach across transects by geometric mean of particle size range, with bedrock set to 5,656 mm (Kaufmann and Robinson, 1998). Percent of geomorphic units (e.g, pool, riffle, run) within 1 m of the transect line were visually estimated and averaged over the study reach (Higashi and Nishimoto, 2007). Wetted width was measured to the nearest 0.1 m, and maximum water depth along the transect line was measured to the nearest 0.01 m. Average wetted transect area was calculated by multiplying transect wetted width by one-half of maximum depth and averaging across transects (Knighton, 1998). In 2013 and 2014, we visually estimated the average percent (± 5%) substrate covered by aquatic moss and filamentous algae within 1 m of the transect line over the study reach. Aquatic moss is not a food resource for A. bisulcata (Riney, 2015) but it may act as high-quality habitat that traps FPOM and contains high levels of epiphytic microalgae, increasing foraging opportunities for grazers (Maurer and Brusven, 1983; Brusven et al., 1990; Bowden and Stream Bryophyte Group, 1999). Shifts to algal-dominated systems represents a potential reduction in available food for A. bisulcata (Riney, 2015).
We sampled A. bisulcata each year, approaching each transect from downstream with a hand seine net (2 mm mesh; 0.91 m wide; 0.45 m deep) and completing a single pass along the transect line. Individual transects were excluded if they were not able to be sampled effectively (e.g., debris in the transect, dry stream beds due to seasonal drought). The total numbers of A. bisulcata collected from each transect were recorded, and a subsample of all individuals were kept for further analysis (typically 75-125 individuals). In some sampling events, few atyids were captured and entire samples were processed. Given low samples sizes in some study reaches, we also collected individuals from the nearest plunge pool upstream to supplement analyses of individual condition. Plunge pools (>~2 m in depth) occurred below waterfalls and were not sampleable using our standard transect design. We seined 1 m2 areas at 2 m intervals along the diameter of the pool to supplement our collection of individuals from the study reach. We included supplemental individuals from plunge pools only in streams with low rainfall (Supplementary Material Table S4). Based on the near absence of A. bisulcata from the study reach in these low rainfall streams, we assumed individuals collected from these plunge pools were representative of the stream population and individual condition could be compared to atyids subsampled from the entire reach in wetter systems.
We measured the post-orbital carapace (POC) length of atyids (i.e., the linear distance from the posterior of the ocular cavity to the posterior of the carapace) to the nearest 0.1 mm using an ocular micrometer and recorded individual wet weights to the nearest 0.001 g. Sex was determined based on shape of the endopod of the first pleopod and the presence of the appendix masculina on males (Couret, 1976; Chace, 1983). We recorded small specimens as juveniles if they did not show characteristics associated with either sex. In 2013 and 2014, we also inspected individuals for brown spot disease. We dried specimens at 60-105°C for at least 24 hours, reweighed, then combusted them at 500°C in a muffle furnace for at least 2.5 hours to obtain ash-free dry mass (AFDM; Benke et al., 1999). In a single stream in 2013, AFDM measurements were not available and were derived from dry weights. We calculated the average relative mass from length-mass relationships for each reach each year using the linear form of the basic length-mass model:
where M is mass (AFDM; g), L is length (POC length; mm) and a and b are constants (Benke et al., 1999). Geometric means of a and b (am and bm, respectively) were calculated from length-mass regressions from each reach each year (Froese, 2006). We calculated the relative mass (Mrm) of each individual as:
We calculated average biomass (g/m2) by finding the proportion of individuals in 0.5 mm POC length-classes in each reach each year. Abundance counts were multiplied by the size-class proportions to estimate the number of individuals within each 0.5 mm size-class at each transect (Cross et al., 2008). Length-mass regressions were used to predict an average AFDM for each size-class, which was multiplied by the size-class counts and summed then divided by wetted width to estimate biomass density at each transect.
We included three metrics of individual condition: POC length, presence of brown spot disease and percent relative mass (Froese, 2006). Females were excluded in calculation of POC length due to their low representation in samples and evidence of size stratification by sex. Females and juveniles were also excluded during analysis of brown spot disease presence and percent relative mass. Disease detection bias can result from post-egg release molting in females or high molting rates in juveniles (Couret, 1976; Chan, 1978; Nishimoto and Kuamo’o, 1996) while percent relative mass may be biased by differences in body shape among life stages and sexes as well as post-egg release declines (Froese, 2006). In two sampling events, low sample sizes restricted the calculation of average relative mass.
We tested for the effects of Q70Y on the percent pools, percent riffles and wetted area averaged across each transect using the linear model:
where DA is effective drainage area. We chose to use an average value of Q70Y across years for each stream, as interannual variation in baseflow was relatively low within streams across our model system. Mean differences across years were tested using the difflsmeans function in R package lmerTest (Kuznetsova et al., 2017). For mean substrate size, percent algal cover and percent moss cover, we used the linear model:
which included the composite metric PC1 as flow stability likely influences the growth and retention of moss and algae and the movement of substrate within the stream channel.
We developed candidate models and used Akaike’s Information Criterion (AIC) to determine which flow metrics, or interactions among them, explain the most variation in A. bisulcata populations and individual condition metrics (A). For biomass density, POC length and percent relative mass, we developed 6 models (Equations 1–6):
with Model 1 as the null model. We included Year as a fixed term instead of a fully crossed random design because the number of factor levels (years) was less than five (Harrison et al., 2018). For models of atyid biomass density, we altered the random intercept term to allow transect estimates of biomass to be nested within streams (1|Stream/Transect). For percent relative mass we included 6 additional models (Equations 7–11):
where we let the Year term interact with flow metrics after visually assessing differences in trends across sample years. We modeled atyid biomass, POC length and percent relative mass using a linear mixed model (LMM; lmer function, R package lme4; Bates et al., 2015) and used a generalized linear mixed model (GLMM; glmer function, R package lme4) assuming a binomial error distribution (logit link) for brown spot disease. Prior to analysis, we added a small amount (0.0001 g/m2) to biomass density estimates and log-transformed to improve normality. We examined LMM residuals for deviations from normality prior to model interpretation. We considered all models<2 ΔAIC as “top-ranked” models with equivalent empirical support and interpreted predictors from the simplest top-ranked model for each A. bisulcata metric. We calculated intraclass correlation coefficients (ICC) to quantify the proportion of variation explained by grouping variables (stream, transect) and marginal and conditional R2 to assess the relative contribution of fixed and random effects to variance explained (tab_model function, R package sjPlot; Nakagawa and Schielzeth, 2013).
Associations between log-transformed Q70Y and habitat metrics indicate that habitat quality was highest in streams with the greatest mean annual rainfall and generally increased with baseflow (Supplementary Material Table S5). Wetted area (p=0.02), percent riffle habitat (p<0.01) and percent moss cover (p = 0.01) were positively associated with Q70Y, while the percent of pool habitat decreased with greater values of Q70Y (p<0.01; Table 1). Wetted area was greater in 2012 than in 2013 (p=0.03) and 2014 (p=0.03), while the percent of pool habitat was lower in 2013 (p<0.01) and 2014 (p=0.01). The initial model of percent algal cover was singular and after removal of the grouping term there were no significant associations with predictor variables. Mean substrate size was significantly positively associated drainage area (p<0.01) and was not significantly associated with changes in stream flow stability or baseflow, but the interaction term was significant (p=0.02).
Table 1 Parameter estimates, mariginal/conditional R2 values, and intraclass correlation coefficients (ICC) for habitat metrics averaged across transects for each sampling event modeled by differences in the natural log of the 70th percentile exceedance of annual flow (Q70Y) normalized by effective drainage area (DA).
Mean biomass density (range 0.000 – 0.824 g/m2), POC length (3.3 – 7.4 mm) and relative mass (68.5 – 120.1%) estimates were generally higher in streams with greater mean annual rainfall across years while the prevalence of brown spot disease was lower (0.0 – 50.0%; Supplementary Material Table S4). Baseflow, as represented by log-transformed Q70Y, was a predictor in all top-ranked models, and the null model ΔAIC was >2 for all A. bisulcata metrics (Supplementary Material Table S6). In all cases, the simplest top-ranked model had the lowest AIC value. Models 2 (ΔAIC = 0) and 5 (ΔAIC = 1.5) were the top-ranked models for A. bisulcata biomass density. Fixed effects in Model 2 accounted for ~66% of the variation explained by the overall model (Marginal R2/Conditional R2 = 0.570/0.874; Table 2), with biomass density positively associated with baseflow and drainage area (Figure 2A). Model 11 was the only top-ranked model for percent relative mass, with fixed effects accounting for 63% of the total variation explained (Marginal R2/Conditional R2 = 0.211/0.332). Percent relative mass was positively associated with baseflow and DH, but the relationship with baseflow was influenced by year (Figure 2B). In 2014, average relative mass within streams did not increase with baseflow and was higher across streams (107.99 ± 2.02 standard error; SE) than in 2012 and 2013 (94.5 ± 3.62 SE and 96.2 ± 2.81 SE respectively; Supplementary Material Table S4). Model 6 was the sole top-ranked model for post-orbital carapace length with A. bisulcata length positively associated with baseflow (Figure 2C). The interaction of baseflow with DH indicates the duration of high flows has a negative effect on average length when baseflow is low but has limited impact on average length when baseflow is high (Supplementary Material Figure S2). Models 2 (ΔAIC = 0) and 6 (ΔAIC = 1.9) were the top-ranked models for predicting brown spot disease infection but fixed effects explained only a small amount of variation (Model 2 Marginal R2 = 0.117). The probability of brown spot disease was lower in streams with high baseflow and disease was most common in drier systems (Table 2, Figure 2D). Metrics of individual condition were less similar within groups than biomass density, which had ICC values at the stream and transect level >0.5.
Table 2 Parameter estimates, mariginal/conditional R2 values, and intraclass correlation coefficients (ICC) for Atyoida bisulcata population and individual condition metrics modeled by differences in the natural log of the 70th percentile exceedance of annual flow (Q70Y) normalized by drainage area (DA) and the duration of high flows (days; DH) from 2012-2014.
Figure 2 Atyoida bisulcata biomass density (A), percent relative mass (B), post orbital carapace length (C) and prevalence of brown spot disease (D) versus baseflow as represented by the natural log of the 70th percentile exceedance value (Q70Y) of flow normalized by catchment area. The gradient in color represents differences in mean annual rainfall across study streams from lowest (dark red; Kaawalii stream) to highest (dark blue; Kolekole stream). Differences in drainage area size are represented by point size for biomass density only. Error bars are one standard error of the mean for transect values (biomass density) or individuals (percent relative mass, post orbital carapace length). Note that the sample size for the prevalance of brown spot disease in Kaawalii Stream in 2014 was only 2, while all other sample sizes were >10. See Supplementary Material Table S4 for additional information on sample size.
Our results demonstrate that tropical island stream organisms are vulnerable to climate change, especially in systems where stream baseflow is primarily controlled by rainfall. Across the study system, baseflow magnitude as well as the amount and quality of habitat declined with mean annual rainfall, while the driest streams had prolonged periods of baseflow close to zero. Our hypothesis, that declines in stream flow negatively influence A. bisulcata habitat, population size and individual condition, was supported. Low A. bisulcata biomass densities in streams with low baseflow and limited physical habitat suggests that seasonal habitat loss limits population establishment in drier systems. However, populations in larger catchments may be less vulnerable to the impacts of prolonged low baseflow. Individual size and condition also generally decline with baseflow and may be driven by changes in foraging opportunities as well as cyclical population loss and recolonization. Brown spot disease prevalence was lowest in streams with high baseflow and diverse habitats, and increased infection rates may indirectly be the result of higher turbidity and bacteria loads during high flow events in drier systems.
Changes in baseflow magnitude were associated with differences in the amount and type of physical stream habitat. This is not surprising, as baseflow changes stream habitat and reduces hydraulic habitat complexity (Rolls et al., 2012). In headwater streams of West Virginia, for example, low baseflow temporarily reduced riffle habitat within seven stream reaches by half but had no effect on total area of pools (Hakala and Hartman, 2004). The homogenization of habitat types in drier systems is apparent across our study area, with declines in baseflow coinciding with an increased prevalence of pools and the absence of riffles and runs. We also observed increases in the percent moss cover in streams with more stable and higher baseflow, which aligns with current understanding of flow controls on aquatic moss (Bowden and Stream Bryophyte Group, 1999). While our study design did not allow for hierarchical tests of stream flow on A. bisulcata through shifts in habitat, strong and consistent relationships between baseflow and habitat metrics suggests that A. bisulcata may be influenced in tandem by changes in the flow regime and habitat. Further research across a larger number of systems could provide more nuanced understanding of the hierarchical relationships between stream flow, habitat, and endemic fauna of Hawaiian streams.
Temporary loss of habitat may prevent the establishment of large A. bisulcata populations in streams with low rainfall. Prolonged periods of low flow can lead to temporary habitat desiccation, resulting in substantial loss of biomass within streams (Rolls et al., 2012). Stream macroinvertebrate communities can show trait-dependent threshold responses to increasing drought intensity, eventually resulting in population loss (Aspin et al., 2019). The driest (i.e., Kaawalii, Pahale) streams in our study system, which experience temporary cessation of stream flow in all study years, would likely be uninhabited by A. bisulcata if it were not for the presence of plunge pools below waterfalls (depth >~2 m). While these habitats encompass only a small amount of the entire channel in streams along the North Hilo Coast, they can act as semi-contained systems during low flows (McRae, 2007). Low flow river systems may therefore act as ecological “sinks” for the metapopulation of A. bisulcata and other migratory stream species, where larvae recruit during high flow events but have limited habitat capable of supporting juveniles and adults (McRae, 2007). While A. bisulcata in larger catchments appear to be more resilient to low baseflow due to an increase amount of instream habitat independent of plunge pools (i.e., Waikaumalo), these streams may still act as ecological sinks for the metapopulation. Larger catchment areas produce larger freshwater plumes, which attract more recruits from the nearshore environment (Nishimoto and Kuamoo, 1991). Large streams that are subject to prolonged low flows may attract high numbers of migratory organisms during high flow events. Cessation of flow could lead to population reductions or other limitations on productivity (i.e., fewer flow events that allow larval transport downstream, lower growth due to limited forage), which may result in an overall lower reproductive contribution to the metapopulation than smaller streams with sustained flow (McDowall, 2010). Quantifying the number of larvae transported to the marine environment across small and large catchments with differences in baseflow magnitudes could further test this hypothesis.
Variation in A. bisulcata biomass density estimates within individual sampling events was high, and standard errors often overlapped in streams with similar baseflows. This is not surprising, as macroinvertebrate density estimates are often highly variable and dependent on microhabitat selection (Rabeni, 1985; DiStefano et al., 2003). Because we saw such drastic variation in biomass density across the study region, we are still confident in observed differences in mean biomass resulting from reductions in suitable habitat. However, direct statistical comparisons of A. bisulcata biomass density between two streams with contrasting baseflow would be limited except for cases where differences in flow are extreme. Controlling for habitat in sample design could improve confidence in biomass estimates, but this proved difficult across our study system because of the drastic shifts in habitat (i.e., riffle-dominated to pool-dominated) and uncertainty in preferential habitat selection under different flow conditions. Increasing the number of transects sampled may be a more feasible approach when sampling across large gradients in stream flow. We conducted a power analysis (α=0.05, power=0.8) for biomass density comparisons between two streams, Umauma (moderate baseflow, large catchment) and Honolii (high baseflow, large catchment), for each year. Adequate sample sizes ranged from 15 – 35 transects, suggesting that doubling sampling effort could improve confidence in statistical comparisons across streams in future studies.
The positive relationship between baseflow and relative mass may be the result of higher food quantity and quality in systems with higher flow and more suitable habitat. Frauendorf et al. (2019) found that in four of the reaches included in this study, net-primary production was greater in high flow streams, that lower quality food resources (i.e., leaf litter) were predominant in low flow systems, and that suspended matter was 40x higher in streams with high baseflow compared to low flow systems. Atyoida bisulcata are opportunistic filterers/grazers assumed to filter FPOM in high velocity areas as well as graze on benthic resources (Couret, 1976). Reductions in high-velocity habitats (i.e., riffles and runs) in drier streams likely limits the amount of available habitat suitable for filtering FPOM. Simultaneously, residence time of coarse particular organic matter is expected to increase under lower flow conditions but Hawaiian streams lack a true shredder guild (Nakajima et al., 2006), and an increase in the availability of leaf litter does little for A. bisulcata food resources. However, average relative mass may be sensitive to increases in food availability resulting from temporary but sustained high flow. April runoff yield was consistently higher in 2014 than 2013 and 2012 (Tingley, 2017), coinciding with greater average relative mass across streams systems. Many studies have documented variability in organism condition with short-term or seasonal changes in food availability (e.g., Hakala and Hartman, 2004; Currinder et al., 2014; Miller et al., 2015), which may indicate differences in individual growth rates (Bentley and Schindler, 2013).
Average POC was highest in stable systems with high baseflow and, like observed differences in relative mass, may be due to higher growth in streams with higher food quantity and quality. Higher stream temperatures may also contribute to smaller A. bisulcata in streams with lower baseflow as increases in rearing temperature of ectotherms results in lower maximum adult size (as reviewed by Atkinson, 1994). Across several of our study reaches, Strauch et al. (2017) demonstrated that mean dry season water temperature increased as baseflow decreased. Given observed declines in adult POC length with declines in stream baseflow, higher average stream temperature may have contributed to lower maximum adult size. It is also likely that lower average sizes in streams with the lowest baseflows are the result of intermittent drying and iterative reductions in the standing stock of A. bisulcata, where few adults survive through dry periods, and streams may be recolonized by recruits moving upstream. This is partially supported by the observed interaction between baseflow and the duration of high flows; prolonged periods of high flow may attract new recruits to even low baseflow systems, resulting in populations dominated by small individuals. Future research could include the quantification of upstream migrant densities in addition to population demographics (length at age, maximum age) to fully explore this hypothesis. While it was beyond the scope of our study, direct age estimation techniques for some crustaceans are possible (e.g., Leland et al., 2015).
Brown spot disease was least prevalent in stream systems with high baseflow. Gagne and Blum (2016) had a similar finding of decreased parasite infection of a native amphidromous goby (Awaous stamineus) in streams with greater mean annual rainfall along the North Hilo coast. The exact mechanism causing increased prevalence of brown spot disease in drier streams is not clear, but previous research indicates that carapace surface abrasions increase susceptibility to chitinoclastic bacterial infection (Chan, 1978), and abrasive forces acting upon macroinvertebrates in streams increase during periods of high suspended sediment loads (Jones et al., 2012). Measures of turbidity from 2012-2014 in three reaches along the North Hilo coast did show higher sediment loads during flood events in streams receiving less annual precipitation (Strauch et al., 2018). Combined with higher bacterial loading during high flow events in drier streams (Strauch et al., 2014), it appears infection with chitinoclastic bacteria is more likely in systems with low baseflow and prolonged high flow events. While the strength of evidence from our study was relatively weak (null model ΔAIC = 3.1; Supplementary Material Table S6), the exact mechanism resulting in higher infection rates could be further explored by testing for differences in the effects of stream flow, turbidity and bacterial load in a controlled environment.
In Hawaii, precipitation is projected to increase in wet regions and decline in dry regions, following a general pattern observed in climate change projections (Elison Timm et al., 2015; Zhang et al., 2016). On windward facing slopes of Hawaii Island and Maui, rainfall is projected to increase significantly in the wet season, with total rainfall during the dry season changing little (Xue et al., 2020). However, the magnitude and timing of rainfall and its delivery to stream systems may ultimately impact stream ecosystems as rainfall intensity is also projected to increase (Fandrich et al., 2022). If streams dependent on persistent rainfall to maintain baseflow receive a higher proportion of rainfall during storm events, average baseflow conditions that result in lower numbers of atyids that are smaller, in poorer condition, and more susceptible to disease may still become more prominent even if mean annual rainfall does not change. Finer resolution projections of annual and daily rainfall paired with our results and additional studies on species-specific sensitivity to flow regime changes would improve the ability to predict climate change impacts on Hawaiian stream ecosystems.
The sustainable management of scarce resources necessitates a holistic perspective that incorporates future climate projections to protect the structure and function of ecosystems. Our results provide an initial understanding of the potential ramifications of climate change for tropical freshwater island streams as shifts in flow regimes alter such ecological processes as mortality, recruitment, and growth. Our results could also be used to understand climate change impacts in regions with the same ecological stream types, such as northeastern Maui (Tingley et al., 2019). Inference beyond similar stream types is not fully supported as broad-scale landscape controls (i.e., groundwater delivery, elevation) influence the relationship between rainfall and flow and the species assemblage present. However, understanding of how such fundamental ecological characteristics will shift across changing flow regimes is limited (Franklin and Gee, 2019), and our results may also have value in assessing how water management may promote critical life-history stages (Way et al., 1998) or how native communities will respond to introduced species (Brasher et al., 2006) in understudied tropical streams ecosystems.
Use of a space-for-time model allowed for the examination of how shifting flow regimes affect A. bisulcata populations but required careful consideration of interannual variation in flow within and across streams. Baseflow (Q70Y) followed a predictable pattern with mean annual rainfall, and variation across streams was greater than within-stream interannual variation. Biotic metrics responded to differences in baseflow across streams as hypothesized, yet when we initially used year-specific baseflow as a predictor, slope estimates appeared erroneous and were sometimes opposite visual patterns observed across the study streams (e.g., POC length). Estimating slopes across years with only small variation in baseflow within streams (a grouping variable in our mixed effects models) seemingly captured spurious relationships between our primary predictor of interest and A. bisulcata populations. The presence of sign-reversal in parameter estimation is an observed phenomena resulting from confounding variables within groups (i.e., Simpson’s paradox; Simpson, 1951), but aggregating Q70Y across years was the most appropriate approach for our study. An important assumption with the aggregation approach is that baseflow is a surrogate for differences in suitability that likely influence our biotic metrics over a timescale longer than a single year. We acknowledge that this assumption is dependent on yearly variation in rainfall. If we had sampled during an extremely dry year, we might have observed lower POC lengths and near-zero biomass density estimates in streams with moderate mean annual rainfall due to habitat loss and population die-off events. Continued sampling over many years would likely provide a greater range in baseflow conditions associated with climate extremes, making the use of yearly estimates of Q70Y more appropriate than an aggregation approach.
The raw data supporting the conclusions of this article will be made available by the authors, without undue reservation.
The manuscript presents research on animals that do not require ethical approval for their study.
RM conceptualized the model system; RM and DI developed the study. RM, DI and RT developed the survey design. RT, AS, PF, RM and DI conducted field sampling. RT, PF and AS processed data in the field and lab. RT, AS, RM, DI and BR contributed to data summary and analysis. RT led on development of figures, tables and initial drafts of the manuscript. RM, DI, AS, RT and BR contributed to critical review and finalization of the manuscript.
The author(s) declare financial support was received for the research, authorship, and/or publication of this article. We are thankful for financial support from the United States Department of Agriculture Forest Service (FS 12-JV-11272138-039), the US Fish and Wildlife Service via the Hawaii Fish Habitat Partnership (F12AC00708), and Michigan State University. Travel to present this research was also partially supported by the National Climate Change and Wildlife Science Center (now the US Geological Survey National Climate Adaptation Science Center) (G10AC00129).
We wish to thank Therese Frauendorf, Michael Riney, James Akau and Kristina Black for assistance in field sampling and laboratory analysis as well as Kevin McDonnell, Andrew Honsey, Robin Debruyne, Robert Klinger and the three individuals who provided initial reviews for edits that improved the manuscript.
The authors declare that the research was conducted in the absence of any commercial or financial relationships that could be construed as a potential conflict of interest.
All claims expressed in this article are solely those of the authors and do not necessarily represent those of their affiliated organizations, or those of the publisher, the editors and the reviewers. Any product that may be evaluated in this article, or claim that may be made by its manufacturer, is not guaranteed or endorsed by the publisher.
The Supplementary Material for this article can be found online at: https://www.frontiersin.org/articles/10.3389/fevo.2024.1182021/full#supplementary-material
Aspin T. W., Khamis K., Matthews T. J., Milner A. M., O’Callaghan M. J., Trimmer M., et al. (2019). Extreme drought pushes stream invertebrate communities over functional thresholds. Glob Chang Biol. 25, 230–244. doi: 10.1111/gcb.14495
Atkinson D. (1994). Temperature and organism size: A biological law for ectotherms? Adv. Ecol. Res. 25, 1–58. doi: 10.1016/S0065-2504(08)60212-3
Bates D., Maechler M., Bolker B., Walker S. (2015). Fitting linear mixed-effects models using lme4. J. Stat. Software 67, 1–48. doi: 10.48550/arXiv.1406.5823
Bauer R. T. (2013). Amphidromy in shrimps: a life cycle between rivers and the sea. Lat. Am. J. Aquat. Res. 41, 633–650. doi: 10.3856/vol41-issue4-fulltext-2
Benke A. C., Huryn A. D., Smock L. A., Wallace J. B. (1999). Length-mass relationships for freshwater macroinvertebrates in North America with particular reference to the southeastern United States. J. N. Am. Benthol. Soc 18, 308–343. doi: 10.2307/1468447
Bentley K. T., Schindler D. E. (2013). Body condition correlates with instantaneous growth in stream-dwelling Rainbow Trout and Arctic Grayling. T. Am. Fish. Soc 142, 747–755. doi: 10.1080/00028487.2013.769899
Bowden W. B., Stream Bryophyte Group (1999). Roles of bryophytes in stream ecosystems. J. N. Am. Benthol. Soc 18, 151–184. doi: 10.2307/1468459
Bradford M. J. (1997). An experimental study of stranding of juvenile salmonids on gravel ears and in side channels during rapid flow decreases. Regul. River. 13, 395–401. doi: 10.1002/(SICI)1099-1646(199709/10)13:5<395::AID-RRR464>3.0.CO;2-L
Brasher A. M. (1997). Habitat use by fish (o’opu), snails (hihiwai), shrimp (‘opae) and prawns in two streams on the island of Moloka’i, University of Hawaii Cooperative National Park Resources Studies Unit Technical Report, 116 (Hawaii: Department of Biology).
Brasher A. M. D. (2003). Impacts of human disturbances on biotic communities in Hawaiian streams. BioScience 11, 1052–1060. doi: 10.1641/0006-3568(2003)053[1052:IOHDOB]2.0.CO;2
Brasher A. M. D., Luton C. D., Goodbred S. L., Wolff R. H. (2006). Invasion patterns along elevation and urbanization gradients in Hawaiian streams. T. Am. Fish. Soc 135, 1109–1129. doi: 10.1577/T05-083.1
Brusven M. A., Meehan W. R., Biggam R. C. (1990). The role of aquatic moss on community composition and drift of fish-food organisms. Hydrobiologia 196, 39–50. doi: 10.1007/BF00008891
Canton S. P., Cline L. D., Short R. A., Ward J. V. (1984). The macroinvertebrates and fish of a Colorado stream during a period of fluctuating discharge. Freshw. Biol. 14, 311–316. doi: 10.1111/j.1365-2427.1984.tb00043.x
Chace J. F. A. (1983). The atya-like shrimps of the Indo-Pacific Region (Decapoda: Atyidae). Smithson. Contrib. Zool. 384, 1–54. doi: 10.5479/SI.00810282.384
Chan J. G. (1978). “Some aspects of a shell disease in the Hawaiian freshwater shrimp, Atya bisulcata (Randall),” in Proceedings of the Second Conference in Natural Sciences Hawaii Volcanoes National Park. Ed. Smith C. W. (Cooperative National Park Resources Study Unit, Hawaii), 42–50.
Chubb A. L., Zink R. M., Fitzsimons J. M. (1998). Patterns of mtDNA variation in Hawaiian freshwater fishes: the phylogeographic consequences of amphidromy. J. Hered. 89, 8–16. doi: 10.1093/jhered/89.1.8
Comte L., Buisson L., Daufresne M., Grenouillet G. (2013). Climate-induced changes in the distribution of freshwater fish: observed and predicted trends. Freshw. Biol. 58, 625–639. doi: 10.1111/fwb.12081
Couret J. C. L. (1976). The biology and taxonomy of a freshwater shrimp, Atyoida bisulcata randall, endemic to the Hawaiian islands. University of Hawai’i at Hilo, Hilo, HI.
Craig D. A. (2003). Geomorphology, development of running water habitats, and evolution of black flies on Polynesian islands. Bioscience 53, 1079–1093. doi: 10.1641/0006-3568(2003)053[1079:GDORWH]2.0.CO;2
Cross W. F., Covich A. P., Crowl T. A., Benstead J. P., Ramirez A. (2008). Secondary production, longevity and resource consumption rates of freshwater shrimps in two tropical streams with contrasting geomorphology and food web structure. Freshw. Biol. 53, 2504–2519. doi: 10.1111/j.1365-2427.2008.02078.x
Currinder B., Cecala K. K., Northington R. M., Dorcas M. E. (2014). Response of stream salamanders to experimental drought in the southern Appalachian Mountains, USA. J. Freshw. Ecol. 29, 579–587. doi: 10.1080/02705060.2014.938135
Cushman R. M. (1985). Review of ecological effects of rapidly varying flows downstream from hydroelectric facilities. N. Am. J. Fish. Manage 5, 330–339. doi: 10.1577/1548-8659(1985)5%3C330:ROEEOR%3E2.0.CO;2
Davey A. J. H., Kelly D. J., Biggs B. J. F. (2006). Refuge-use strategies of stream fishes in response to extreme low flows. J. Fish Biol. 69, 1047–1059. doi: 10.1111/j.1095-8649.2006.01180.x
DiStefano R. J., Gale C. M., Wagner B. A., Zweifel R. D. (2003). A sampling method to assess lotic crayfish communities. J. Crustacean Biol. 23, 678–690. doi: 10.1651/C-2364
Elison Timm O., Giambelluca T. W., Diaz H. F. (2015). Statistical downscaling of rainfall changes in Hawai’i based on the CMIP5 global model projections. J. Geophys. Res-Atmos. 120, 92–112. doi: 10.1002/2014JD022059
Fandrich K. M., Ellison Timm O., Zhang C., Giambelluca T. W. (2022). Dynamical downscaling of near-term, (2026–2035) climate variability and change for the main Hawaiian islands. J. Geophys. Res-Atmos. 127, 1–18. doi: 10.1029/2021JD035684
Franklin P., Gee E. (2019). Living in an amphidromous world: Perspectives on the management of fish passage from an island nation. Aquat. Conserv.: Mar. Freshw. Ecosys. 29, 1424–1437. doi: 10.1002/aqc.3049
Frauendorf T. C., MacKenzie R. A., Tingley R.W. III, Frazier A. G., Riney M. H., El-Sabaawi R. W. (2019). Evaluating ecosystem effects of climate change on tropical island streams using high spatial and temporal resolution sampling regimes. Glob. Change Biol. 25, 1344–1357. doi: 10.1111/gcb.14584
Frauendorf T. C., MacKenzie R. A., Tingley R.W. III, Infante D. M., El-Sabaawi R. W. (2020). Using a space-for-time substitution approach to predict the effects of climate change on nutrient cycling in tropical island stream ecosystems. Limnol. Oceanogr. 65, 3114–3127. doi: 10.1002/lno.11577
Froese R. (2006). Cube law, condition factor and weight-length relationships: history, meta-analysis and recommendations. J. Appl. Ichthyol. 22, 241–253. doi: 10.1111/j.1439-0426.2006.00805.x
Gagne R. B., Blum M. J. (2016). Parasitism of a native Hawaiian stream fish by an introduced nematode increases with declining precipitation across a natural rainfall gradient. Ecol. Freshw. Fish. 25, 476–486. doi: 10.1111/eff.12228
Giambelluca T. W., Chen Q., Frazier A. G., Price J. P., Chen Y. L., Chu P. S., et al. (2013). Online rainfall atlas of Hawai’i. B. Am. Meteorol. Soc 94, 313–316. doi: 10.1175/BAMS-D-11-00228.1
Gorbach K. R., Benbow M. E., McIntosh M. D., Burky A. J. (2012). Dispersal and upstream migration of an amphidromous neritid snail: implications for restoring migratory pathways in tropical streams. Freshw. Biol. 57, 1643–1657. doi: 10.1111/j.1365-2427.2012.02826.x
Hakala J. P., Hartman K. J. (2004). Drought effect on stream morphology and brook trout (Salvelinus fontinalis) populations in forested headwater streams. Hydrobiologia 515, 203–213. doi: 10.1023/B:HYDR.0000027330.12182.24
Harrison X. A., Donaldson L., Correa-Cano M. E., Evans J., Fisher D. N., Goodwin C. E., et al. (2018). A brief introduction to mixed effects modelling and multi-model inference in ecology. PeerJ 6, e4794. doi: 10.7717/peerj.4794
Henriksen J. A., Heasley J., Kennen J. G., Nieswand S. (2006). Users’ Manual for the Hydroecological Integrity Assessment Process Software (including the New Jersey Assessment Tools), U.S. Geological Survey, Biological Resource Discipline, Open File Report 2006-1093 (Washington DC: U.S.: Department of the Interior).
Higashi G. R., Nishimoto R. T. (2007). “The point quadrant method: rapid assessment of hawaiian streams,” in Biology of Hawaiian Stream and Estuaries, Bishop Museum Bulletin in Cultural and Environmental Studies. Eds. Evenhuis N. L., Fitzsimons J. M., 305–312.
IPCC (2013). “Climate Change 2013: The Physical Science Basis,” in Contribution of Working Group I to the Fifth Assessment Report of the Intergovernmental Panel on Climate Change. Eds. Stocker T. F., Qin D., Plattner G. K., Tignor M., Allen S. K., Boschung J., Nauels A., Xia Y., Bex V., Midgley P. M. (Intergovernmental Panel on Climate Change, United Kingdom and New York).
Isaak D. J., Wollrab S., Horan D., Chandler G. (2012). Climate change effects on stream and river temperatures across the northwest US from 1980-2009 and implications for salmonid fishes. Climatic Change. 113, 499–524. doi: 10.1007/s10584-011-0326-z
James A. B. W., Dewson Z. S., Death R. G. (2007). The effect of experimental flow reductions on macroinvertebrate drift in natural and streamside channels. River Res. Appl. 24, 22–35. doi: 10.1002/rra.1052
Jones J. I., Murphy J. F., Collins A. L., Sear D. A., Naden P. S., Armitage P. D. (2012). The impact of fine sediment on macro-invertebrates. River Res. Appl. 28, 1055–1071. doi: 10.1002/rra.1516
Kaufmann P. R., Robinson E. G. (1998). “Physical Habitat Characterization,” in Environmental Monitoring and Assessment Program - Surface Waters: Field Operations and Methods for Measuring the Ecological Condition of Wadeable Streams. Eds. Lazorchak J. L., Klemm D. J., Peck D. V. (Washington DC, U.S. Environmental Protection Agency), 77–93.
Keith P. (2003). Biology and ecology of amphidromous Gobiidae of the Indo-Pacific and the Caribbean regions. J. Fish Biol. 63, 831–847. doi: 10.1046/j.1095-8649.2003.00197.x
Kido M. H. (2008). A persistent species assemblage structure along a Hawaiian stream from catchment-to-sea. Environ. Biol. Fishes. 82, 223–235. doi: 10.1007/s10641-007-9276-8
Kido M. H. (2013). A native species-based index of biological integrity for Hawaiian stream environments. Environ. Monit. Assess. 185, 4063–4075. doi: 10.1007/s10661-012-2849-9
Krabbenhoft T. J., Myers B. J. E., Wong J. P., Chu C., Tingley R. W. III, Falke J. A., et al. (2020). FiCli, the Fish and Climate Change Database, informs climate adaptation and management for freshwater fishes. Sci. Data. 7, 1–6. doi: 10.1038/s41597-020-0465-z
Krabbenhoft T. J., Platania S. P., Turner T. F. (2014). Interannual variation in reproductive phenology in a riverine fish assemblage: implications for predicting the effects of climate change and altered flow regimes. Freshw. Biol. 59, 1744–1754. doi: 10.1111/fwb.12379
Kuznetsova A., Brockhoff P. B., Christensen R. H. B. (2017). lmerTest package: tests in linear mixed effects models. J. Stat. Software 82, 1–26. doi: 10.18637/jss.v082.i13
Leland J. C., Bucher D. J., Coughran J. (2015). Direct age determination of a subtropical freshwater crayfish (redclaw, Cherax quadricarinatus) using ossicular growth marks. PloS One 10. doi: 10.1371/journal.pone.0134966
Leong J. A., Marra J. J., Finucane M. L., Giambelluca T., Merrifield M., Miller S. E., et al. (2014). “Chapter 23: Hawai’i and U.S. Affiliated Pacific Islands,” in Climate Change Impacts in the United States: The Third National Climate Assessment U.S. Global Change Research Program. Eds. Melillo J. M., Richmond T. C., Yohe G. W. (Government Printing Office, Washington DC: U.S), 537–556.
Leppi J. C., DeLuca T. H., Harrar S. W., Running S. W. (2012). Impacts of climate change on August stream discharge in the Central-Rocky Mountains. Climatic Change. 112, 997–1014. doi: 10.1007/s10584-011-0235-1
Lytle D. A., Poff N. L. (2004). Adaptation to natural flow regimes. Trends. Ecol. Evol. 19, 94–100. doi: 10.1016/j.tree.2003.10.002
Maurer M. A., Brusven M. A. (1983). Insect abundance and colonization rate in Fontinalis-neo-mexicana (bryophyta) in an Idaho batholith stream. U.S.A. Hydrobiol. 98, 9–15. doi: 10.1007/BF00019246
McDowall R. M. (2007). On amphidromy, a distinct form of diadromy in aquatic organisms. Fish. Fish. 8, 1–13. doi: 10.1111/j.1467-2979.2007.00232.x
McDowall R. M. (2010). Why be amphidromous: Expatrial dispersal and the place of source and sink population dynamics? Rev. Fish Biol. Fish. 20, 87–100. doi: 10.1007/s11160-009-9125-2
McRae M. G. (2007). “The Potential for source-sink population dynamics in Hawaii’s amphidromous fishes,” in Biology of Hawaiian Stream and Estuaries, Bishop Museum Bulletin in Cultural and Environmental Studies 3. Eds. Evenhuis N. L., Fitzsimons J. M., 87–98.
Meffe G. K., Minckley W. L. (1987). Persistence and stability of fish and invertebrate assemblages in a repeatedly disturbed Sonoran desert stream. Am. Midl. Nat. 117, 177–191. doi: 10.2307/2425718
Miller S. J., VanGenechten D. T., Cichra C. E. (2015). Length weight relationships and an evaluation of fish size and seasonal effects on relative condition (K-n) of fishes from the Wekiva River, Florida. Fla. Sci. 78, 1–19.
Myers B. J. E., Lynch A. J., Bunnell D. B., Chu C., Falke J. A., Kovach R. P., et al. (2017). Global synthesis of the documented and projected effects of climate change on inland fishes. Rev. Fish Biol. Fish. 27, 339–361. doi: 10.1007/s11160-017-9476-z
Naesje T., Jonsson B., Skurdal J. (1995). Spring flood: A primary cue for hatching of river spawning Coregoninae. Can. J. Fish. Aquat. Sci. 52, 2190–2196. doi: 10.1139/f95-811
Nakagawa S. N., Schielzeth H. (2013). A general and simple method for obtaining R2 from generalized linear mixed effects models. MEE 4, 133–142. doi: 10.1111/j.2041-210x.2012.00261.x
Nakajima T., Asaeda T., Fujino T., Nanda A. (2006). Coarse particulate organic matter distribution in the pools and riffles of a second-order stream. Hydrobiologia 559, 275–283. doi: 10.1007/s10750-005-9127-6
Nishimoto R. T., Fitzsimons J. M. (2006). “Status of native Hawaiian stream fishes, a unique amphidromous biota,” in Status, Distribution, and Conservation of Native Freshwater Fishes in Western North America. Eds. Brouder M. J., Scheurer J. A. (American Fisheries Society, Bethesda, Maryland), 21–27.
Nishimoto R. T., Kuamoo D. G. K. (1991). “The occurrence and distribution of the native goby (Lentipes concolor) in Hawai ‘i Island streams with notes on the distribution of other native fish species,” in New Directions in Research, Management and Conservation of Hawaiian Freshwater Stream Ecosystems: Proceedings of the 1990 Symposium on Freshwater Stream Biology and Fisheries Management, Honolulu, HI. 77–95 (Division of Aquatic Resources).
Nishimoto R. T., Kuamo’o D. G. K. (1996). Monitoring study of the endemic mountain shrimp, Atyoida bisulcata Randall, in Waikaumalo Stream, Hawaii Island. Proc. West. Assoc. Fish Wildl. Agencies. 76, 262–273.
Oki D. S., Wolff R. H., Perreault J. A. (2010). Effects of surface-water diversion on streamflow, recharge, physical habitat, and temperature, Na Wai Eha, Maui, Hawaii, U.S. Geological Survey Scientific Investigations Report 2010–5011 (Virginia: U. S: Geological Survey).
Poff N. L., Allan J. D., Bain M. B., Karr J. R., Prestegaard K. L., Richter B. D., et al. (1997). The natural flow regime. Bioscience 47, 769–784. doi: 10.2307/1313099
Price J. P., Jacobi J. D., Gon S.M. III, Matsuwaki D., Mehrhoff L., Wagner W., et al. (2012). Mapping plant species ranges in the Hawaiian Islands—Developing a methodology and associated GIS layers, U.S. Geological Survey Open-File Report 2012–1192 (Virginia: U.S.: Geological Survey).
Rabeni C. F. (1985). Resource partitioning by stream dwelling crayfish: the influence of body size. Am. Midl. Nat. 113, 20–29. doi: 10.2307/2425344
Riney M. H. (2015). The impacts of stream flow rate on food resource quality and diets of native endemic atyid shrimps (Atyoida bisulcata) in Hawaiian streams. University of Hawaii at Hilo, Honolulu, HI.
Rolls R. J., Leigh C., Sheldon F. (2012). Mechanistic effects of low-flow hydrology on riverine ecosystems: ecological principles and consequences of alteration. Freshw. Sci. 31, 1163–1186. doi: 10.1899/12-002.1
Sherrod D. R., Robinson J. E., Sinton J. M., Watkins S. E., Brunt K. M. (2008) Database to accompany geologic map of the state of Hawaii. Available online at: https://www.sciencebase.gov/catalog/item/60df56d5d34ed15aa3b8a39c (Accessed March 7, 2013).
Simpson E. (1951). The interpretation of interaction in contingency tables. J. R. Stat. Society Ser. B 13, 238–241. doi: 10.1111/j.2517-6161.1951.tb00088.x
Strauch A. M., Giardina C. P., Mackenzie R. A., Heider C., Giambelluca T. W., Salminen E., et al. (2016). Modeled effects of climate change and plant invasion on watershed function across a steep tropical rainfall gradient. Ecosystems 19, 1–18. doi: 10.1007/s10021-016-0038-3
Strauch A. M., Mackenzie R. A., Bruland G. L., Tingley R. W. III, Giardina C. P. (2014). Climate change and land use drivers of fecal bacteria in tropical Hawaiian rivers. J. Environ. Quality. 43, 1475–1483. doi: 10.2134/jeq2014.01.0025
Strauch A. M., MacKenzie R. A., Giardina C. P., Bruland G. L. (2015). Climate driven changes to rainfall and streamflow patterns in a model tropical island hydrological system. J. Hydrol. 523, 160–169. doi: 10.1016/j.jhydrol.2015.01.045
Strauch A. M., MacKenzie R. A., Giardina C. P., Bruland G. L. (2018). Influence of declining mean annual rainfall on the behavior and yield of sediment and particulate organic carbon from tropical watersheds. Geomorphology 306, 2–39. doi: 10.1016/j.geomorph.2017.12.030
Strauch A. M., MacKenzie R. A., Tingley R.W. III (2017). Baseflow driven shifts in tropical stream temperature regimes across a mean annual rainfall gradient. Hydrol. Process. 31, 1678–1689. doi: 10.1002/hyp.11084
Tingley R.W. III (2017). A multi-scaled research framework for conserving streams with changing climate. Michigan State University, East Lansing, Michigan.
Tingley R.W. III, Infante D. M., MacKenzie R. A., Cooper A. R., Tsang Y.-P. (2019). Identifying natural catchment landscape influences on tropical stream organisms: classifying stream reaches of the Hawaiian Islands. Hydrobiologia 826, 67–83. doi: 10.1007/s10750-018-3726-5
Walter R. P., Hogan J. D., Blum M. J., Gagne R. B., Hain E. F., Gilliam J. F., et al. (2012). Climate change and conservation of endemic amphidromous fishes in Hawaiian streams. Endanger Species Rese. 16, 261–272. doi: 10.3354/esr00404
Ward E. J., Anderson J. H., Beechie T. J., Pess G. R., Ford J. M. (2015). Increasing hydrologic variability threatens depleted anadromous fish populations. Glob. Change Biol. 21, 2500–2509. doi: 10.1111/gcb.12847
Way C. M., Burky A. J., Harding J. M., Hau S., Puleloa W. K. L. C. (1998). Reproductive biology of the endemic goby, Lentipes concolor, from Makamaka’ole Stream, Maui and Waikolu Stream, Moloka’i. Environ. Biol. Fish. 51, 53–65. doi: 10.1023/A:1007305917443
Xue L., Wang Y., Newman A. J., Ikeda K., Rasmussen R. M., Giambelluca T. W., et al. (2020). How will rainfall change over Hawai’i in the future? High-resolution regional climate simulation of the Hawaiian islands. BAST 1, 459–490. doi: 10.1007/s42865-020-00022-5
Zeigler M. P., Todd A. S., Caldwell C. A. (2012). Evidence of recent climate change within the historic range of Rio Grande Cutthroat Trout: Implications for management and future persistence. T. Am. Fish. Soc 141, 1045–1059. doi: 10.1080/00028487.2012.676589
Keywords: climate change, Hawaii, Atyoida bisulcata, stream habitat, flow regime, rainfall, variability
Citation: Tingley RW III, Infante DM, MacKenzie RA, Strauch AM, Foulk PB and Roth B (2024) Climate-driven differences in flow regimes alter tropical freshwater ecosystems with consequences for an endemic shrimp. Front. Ecol. Evol. 12:1182021. doi: 10.3389/fevo.2024.1182021
Received: 08 March 2023; Accepted: 01 February 2024;
Published: 27 February 2024.
Edited by:
Robert Klinger, United States Department of the Interior, United StatesReviewed by:
Roderick Gagne, University of Pennsylvania, United StatesCopyright © 2024 Tingley, Infante, MacKenzie, Strauch, Foulk and Roth. This is an open-access article distributed under the terms of the Creative Commons Attribution License (CC BY). The use, distribution or reproduction in other forums is permitted, provided the original author(s) and the copyright owner(s) are credited and that the original publication in this journal is cited, in accordance with accepted academic practice. No use, distribution or reproduction is permitted which does not comply with these terms.
*Correspondence: Ralph W. Tingley III, cnRpbmdsZXlAdXNncy5nb3Y=
†Present address: Ralph W. Tingley III, USGS Great Lakes Science Center, Ann Arbor, MI, United States
‡ORCID: Ralph W. Tingley III, orcid.org/0000-0002-1689-2133
Dana M. Infante, orcid.org/0000-0003-1385-1587
Richard A. MacKenzie, orcid.org/0000-0003-3779-787X
Disclaimer: All claims expressed in this article are solely those of the authors and do not necessarily represent those of their affiliated organizations, or those of the publisher, the editors and the reviewers. Any product that may be evaluated in this article or claim that may be made by its manufacturer is not guaranteed or endorsed by the publisher.
Research integrity at Frontiers
Learn more about the work of our research integrity team to safeguard the quality of each article we publish.