- 1Department of Geography and the Environment, University of North Texas, Denton, TX, United States
- 2Department of City and Metropolitan Planning, Natural History Museum of Utah, University of Utah, Salt Lake City, UT, United States
- 3Department of Geography, University of Utah, Salt Lake City, UT, United States
- 4Department of Biological Sciences, University of North Texas, Denton, TX, United States
- 5Wolf Creek Operating Foundation, Wolf, WY, United States
Feather quality in birds is considered an honest signal of individual health as feather appearance and function depend on an individual’s ability to maintain them. In addition to flight and insulation, feathers are essential for social interactions and sexual selection in the form of visual signals. Airborne particulate matter (PM) can accumulate on feather surfaces and alter feather appearance. We quantified PM accumulation on Rock Pigeon (Columba livia) feathers and analyzed the spectral properties of extracted particulates. Feathers were sampled from two pigeon populations, one rural and one urban, in the Dallas-Fort Worth area, with 47 and 93% developed land cover, respectively, within 2 km of the populations. We determined accumulated PM gravimetrically after rinsing feathers and then measured the visible-near-infrared diffuse reflectance and color properties of extracted particulates. The rate of PM accumulation on rural birds was higher than on urban birds. However, feather particulates collected from urban pigeons had significantly lower total reflectance, reflectance in the visible portion of the spectrum, lightness, and hue angle compared to those of rural pigeons. The hue angle of rural feather particulates displayed a negative relationship with PM accumulation while total reflectance, reflectance in the visible range, and lightness of urban feather particulates were negatively related to PM accumulation. Our findings suggest that wild birds could incur an urban pollution penalty as PM accumulation has the potential to alter feather properties. Further research is needed to better understand the influence of external PM accumulation on the physiological and behavioral health of birds.
1. Introduction
Many bird species rely on visual signals for sexual selection and social interactions, in part relayed by the vast array of feather colors that are produced when light reflects off pigments deposited in the feather or interacts with nano-scale feather structures (Hill and McGraw, 2006). Melanins and carotenoids are the most abundant feather pigments and produce color by reflecting and absorbing different wavelengths of visible light. Common to all birds, melanins produce black, brown, gray, and rufous colors. Carotenoid pigments, produced by plants and available only through diet, generate yellow, orange, and red hues. Structural colors, like those found in iridescent feathers, result from differences in barbule structure at the nanoscale and the physical interplay of light, reflecting most strongly in shortwave ultraviolet (UV) wavelengths. Feather appearance depends on complex interactions among these processes resulting in reflectance and color characteristics unique to each species (Hill and McGraw, 2006).
Age, sex, diet, and environmental stressors such as disease and pollutants influence body condition, which in turn affects feather health and appearance (Leclaire et al., 2014; Leveau, 2021). Because feather spectral properties are condition-dependent, they communicate different aspects of individual quality and thus are considered honest signals (D’Alba et al., 2011; Cantarero et al., 2016; reviewed in White, 2020). Maintaining these honest signals may be challenging in increasingly polluted atmospheres.
Particulate matter (PM) is an air pollutant of concern in urban areas worldwide (Apte et al., 2021). Industrial processes, power generation, and vehicle-related emissions (fuel combustion, road dust, brake- and tire-wear) are the main sources of urban PM pollution (National Research Council, 2010; World Health Organization (WHO) European Centre for Environment and Health, 2013; United States Environmental Protection Agency, 2021). Wildfires also contribute to regional spikes in urban PM (Buysse et al., 2019), a problem exacerbated by increasing wildfire frequency and severity. Particulate matter can also be an air quality issue in rural areas, where agriculture, unpaved roads, biomass burning, and diesel-powered farm equipment represent major PM sources (Sun et al., 2017). Inhalation of PM adversely affects bird health (North et al., 2017; Sanderfoot and Holloway, 2017; Sanderfoot et al., 2022), however inhalation is not the only route of exposure to PM.
Substantial amounts of PM can accumulate on feather surfaces (Borghesi et al., 2016; DuBay and Fuldner, 2017; Pitre et al., 2021) due to their unique structural properties (e.g., barbs, barbules). Feathers collected from Greater Flamingo (Phoenicopterus roseus) chicks, for example, revealed densely packed and diverse particulates concentrated near barbs (Borghesi et al., 2016), and examination of feather vanes under a scanning electron microscope showed accumulation of black carbon particles on museum specimens (DuBay and Fuldner, 2017). Because of their light-scattering and light-absorbing properties, particulate pollutants can affect feather appearance.
Many studies show complex and species-specific changes in feather reflectance and color due to exposure to and accumulation of various anthropogenic pollutants (Dauwe and Eens, 2008; Griggio et al., 2011; Chatelain et al., 2017; Grunst et al., 2020). External PM accumulation may alter visual signals, with physiological and behavioral implications for wild bird populations. Thus, in this preliminary study, we had two objectives: (1) quantify total external PM accumulation on Rock Pigeon (Columba livia) feathers at one urban and one rural site, and (2) analyze differences in the spectral properties of extracted particulates and their relationship to PM accumulation.
2. Materials and methods
2.1. Study area and sites
This study was conducted in Denton County, Texas, in the Dallas-Fort Worth (DFW) area. Denton is a rapidly urbanizing county, with most of the human population and development concentrated in the south. The northern part has a lower population density and is dominated by agricultural land. Prevailing southerly winds (Supplementary Figure 1) transport pollutants from the cities of Dallas and Fort Worth toward Denton (Ponette-González et al., 2022). These transported pollutants, and emissions from major county roads, contribute to elevated air pollution in Denton County (Luce et al., 2020; United States Census Bureau, 2020).
We selected two sites 13.9 km apart––one rural and one urban––with resident pigeon populations (Supplementary Figure 1). We characterized land cover within a 2-km radius buffer of the sites using the 2016 National Land Cover Database (NLCD; Yang et al., 2018; Jin et al., 2019; Homer et al., 2020; Figure 1). We used this buffer size because most pigeons forage within 2 km of their home roost (Rose et al., 2006). Total developed land was calculated as the sum of four NLCD categories: open space, low-intensity, medium-intensity, and high-intensity developed land.
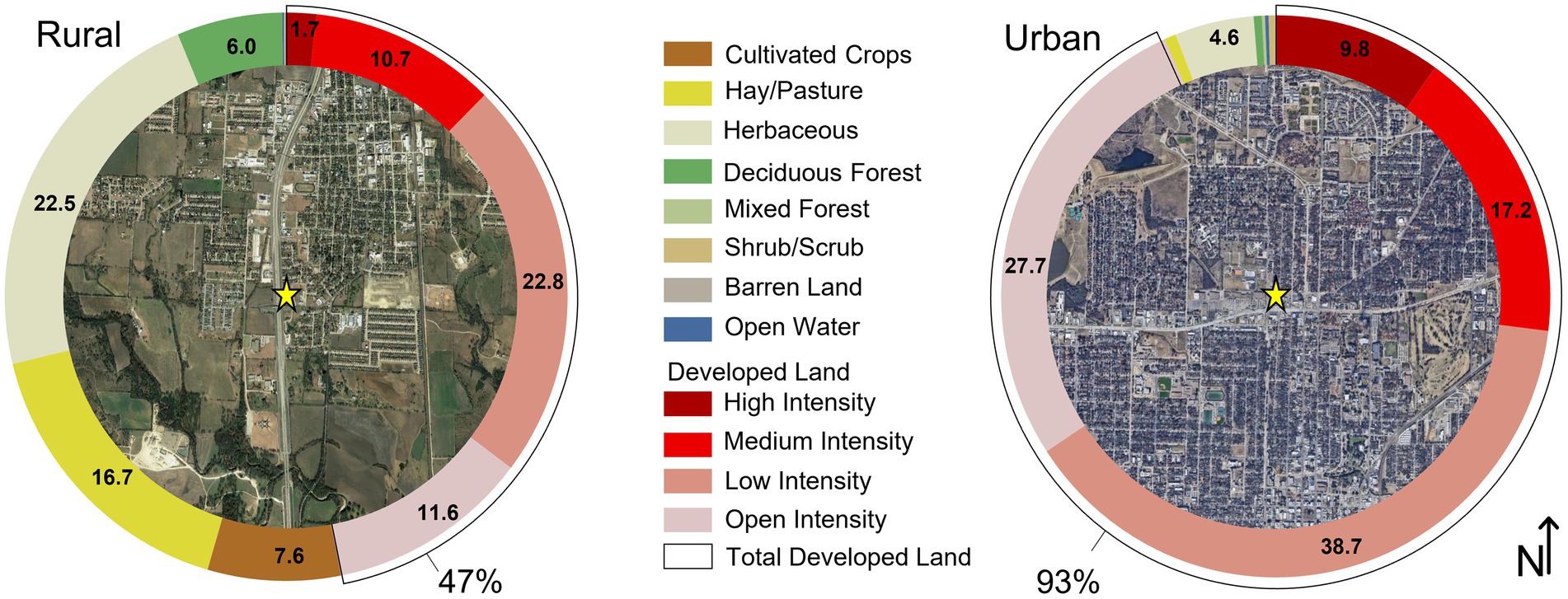
Figure 1. 2016 Land cover (Yang et al., 2018; Jin et al., 2019; Homer et al., 2020) within 2 km of sampled pigeon populations in Denton County. Image sources: “Rural, Sanger, Texas.” 33°21′05.05”N, 97°10′51.58”W. November 26, 2018; “Urban, Denton, Texas.” 33°13′54.80”N, 97°08′10.66”W. November 15, 2020. Google Earth. October 4, 2021.
At the rural site, 47% of the land is developed, with 12% classified as medium- and high-intensity development. Here, pigeons were observed nesting under the eaves of a restaurant building with a paved/gravel surface parking lot 100 m east of Interstate-35 (Figure 2A). At the urban site, developed land comprises 93% of total land cover, of which 27% is medium- and high-intensity development. Pigeons were observed at a carwash located <120 m from a major road, characterized by regular traffic, and surrounded by several building structures (Figure 2B).
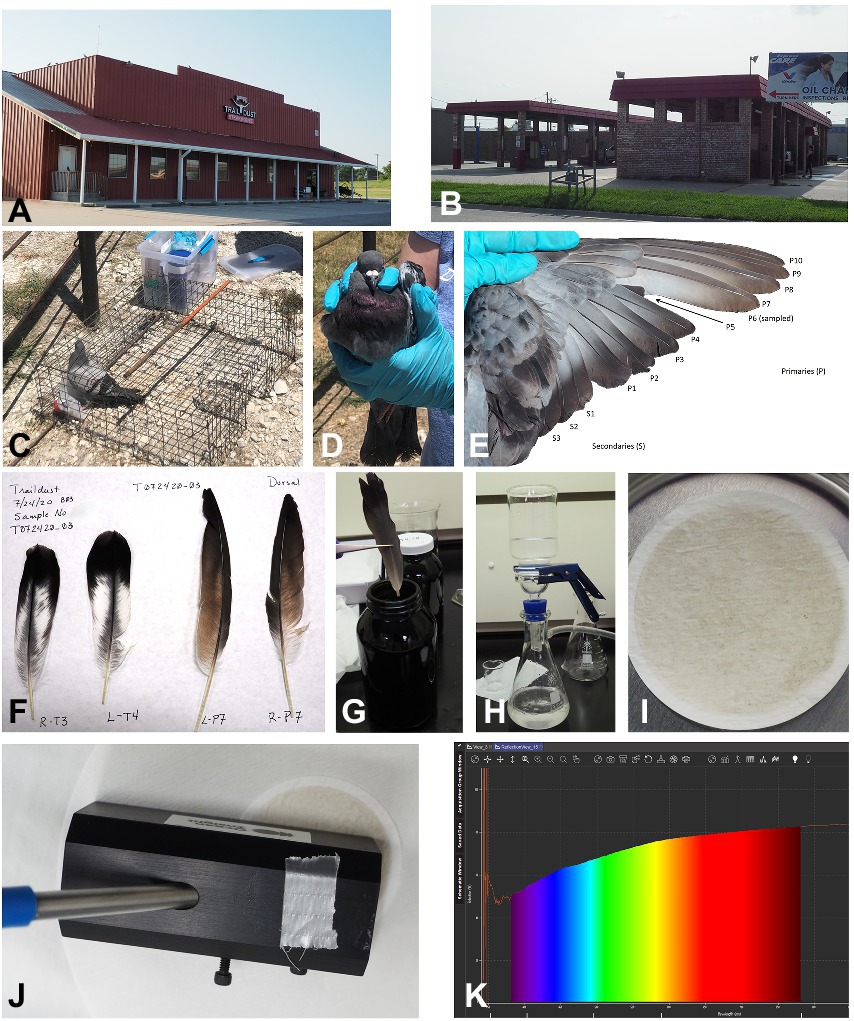
Figure 2. Field sampling and laboratory methods used to investigate the spectral properties of PM collected from Rock Pigeon feathers in Denton County, Texas. (A) Rural site, (B) Urban site, (C) A pigeon in a trap at the rural location, (D) Rural pigeon, (E) Molting pattern in the right wing of a Rock Pigeon, (F) Pooled feather sample labeled and ready for surface area determination, (G) Sonication of a feather sample, (H) Sample filtration, (I) Dried filter with collected particulates, (J) Measurement of diffuse reflectance, and (K) Sample spectral curve.
2.2. Focal species: Rock Pigeon
Rock Pigeons (Columba livia) are an ideal species for investigating PM effects on wild birds. First, as a synanthropic species, their distribution and abundance are closely associated with human activities; pigeons are ubiquitous year-round residents in many urban and rural areas (Lowther and Johnston, 2020). Second, pigeons have small home ranges. Travel distance from the home roost to a foraging site may be <1 km or rarely as far as 25 km, but most birds stay within 2 km of the home roost (Rose et al., 2006). As such, pigeon populations can occupy sites with differing atmospheric environments (level and type of pollution) and minimal overlap. Third, pigeons have large feathers that provide large surface areas for PM accumulation. This means that fewer feathers may be needed to capture and measure detectable quantities of PM (Pitre et al., 2021) and that feather sampling may be less invasive.
2.3. Feather sampling
At each of the sites, resident pigeons were pre-baited for 2 weeks using a wild bird seed mix of milo, millet, and wheat. When birds became accustomed to the seed, an open walk-in funnel trap (funnel removed) made of wire mesh measuring 61cm W x 61cm D x 20.3cm H (Figure 2C) was placed on the ground and baited for an additional 2 weeks. When birds began arriving in numbers, the trap was set for capturing pigeons by inserting the funnel.
Ten individual pigeons were caught at each location (Figure 2D), and feather samples were collected––between 24 and 27 July 2020 at the rural site and between 24 August and 8 September 2020 at the urban site. Young and old feathers were sampled from each population. Approximate feather age was used to estimate relative PM accumulation per individual to help control for differences in timing of sampling among individuals and between sites (see below). Four “older” feathers (one primary feather from each wing and two tail feathers) were plucked from each bird. Feather age was determined by molting sequence, general wear, frayed edges, and fading color. Two “newer” primary feathers were sampled from the urban population while one newer primary feather was sampled from the rural population. These feathers were identifiable by their crisp colors, smooth edges, and lack of wear (Figure 2E). We aimed to minimize stress to birds by plucking as few of these recently regrown feathers as possible.
At both locations, birds were actively molting flight feathers allowing us to identify primary feathers for sampling based on approximate age (i.e., old vs. new) and appearance relative to their replacement sequence. Because molting of individual primaries in Rock Pigeons often occurs in sequence from P1 to P10, the feathers’ appearance at timing of molt and regrowth can be used to identify the relative point of each primary within that sequence in terms of approximate age. In a study of captive Rock Pigeons, primary feathers had mean lifetimes ranging from 10.5 to 12.2 months before their replacement (Mallet-Rodrigues, 2012). The “older” feathers sampled for this study were identified as next in sequence to molt (Figure 2E) and considered approximately 1 year in age, whereas each “newer” primary feather was estimated to be at least 45 days old depending on its position relative to molting sequence. This estimate is based on the growth rate and pattern of replacement observed by Mallet-Rodrigues (2012) who found that an older primary feather in Rock Pigeons dropped once the immediately preceding feather within the molt sequence had grown three-fourths of its length or about 2 weeks. Complete feather growth of each primary took between 19 and 39 days depending on its final feather size (Mallet-Rodrigues, 2012).
We note that these ages are a rough approximation; many factors influence molting patterns in feral pigeons including breeding status and nutritional deficiencies (Johnston and Janiga, 1995). For this study, we sampled pigeons only where molting sequence was easily discernable allowing for a consistent strategy in terms of sampling both old and new primary feathers among individuals and between sites. After collection, feathers were placed in paper envelopes, labeled, and transported to the lab, where they were stored at room temperature until extractions were performed.
2.4. Feather PM extraction and filtration
The four “older” feathers from each bird were pooled into one sample for extraction (Figure 2F). “Newer” feathers were extracted individually so that the protocol remained consistent for both populations. Before use, glassware was soaked overnight in double deionized (DDI) water, washed in a 10% nitric acid bath a minimum of 4 h, and baked in a muffle furnace at 450°C for 5 h to remove all PM.
Feather samples were extracted three times using water and acetone. Feathers were sonicated tip-down in a capped 500 ml amber bottle filled with 300 ml DDI water for 5 min (water rinse). Feathers were then flipped and sonicated an additional 5 min (Figure 2G). After sonication, feathers were rinsed three times on both sides with acetone until 10 ml of acetone were collected. The acetone rinse was added to the water rinse along with 4.65 g of NH4H2PO4 salt (1.5 g per 100 ml solution) to improve recovery of fine and ultrafine particles (Torres et al., 2016). Samples were sonicated an additional 10 min before they were stored in the refrigerator overnight. Glassware was cleaned after each sample following the protocol outlined above.
Following extractions, feathers were laid on a clean lab bench, wrapped lightly in a Kimwipe, and allowed to air dry. Once dry, a digital image of each feather was obtained on a computer scanner and the surface area was calculated using the open-source software ImageJ (Ferreira and Rasband, 2012). Surface area was determined by averaging the dorsal and ventral surface areas.
Samples were filtered through Whatman 934-AH Ready-to-use (RTU) 47-mm glass microfiber filters to collect all PM >1.5 μm in diameter (Figure 2H). Filters were packaged in labeled aluminum pans and pre-conditioned and pre-weighed according to Standard Method 2,540 Part D (Standard Methods Committee of the American Public Health Association et al., 2018). Filters were (1) pre-rinsed under vacuum with three successive lots of 20 ml reagent grade water; (2) dried at 103–105°C for 1 h; (3) cooled in a desiccator to ambient temperature and weighed; and (4) dried and weighed until weight measurements differed by less than 4%. After filtration, filters were air-dried on wire mesh in a desiccator for 2 h.
For quality assurance/quality control, one method blank was included after every five samples (two per population) and the “newer” feather extractions. We performed the same extraction process in the absence of feathers to determine all sources of potential contamination while processing the filters in the lab.
2.5. Gravimetric analysis
To establish a constant weight, sample filters were returned to aluminum pans and dried for 1 h in a drying oven at 103°C, cooled in a desiccator for 1 h to room temperature (Figure 2I), and then weighed on an internally calibrated Mettler Toledo MS105DU Semi-Micro balance to the nearest 0.01 mg.
Pre- and post-weights were conducted under similar conditions of temperature and relative humidity to control for hygroscopic growth of filters. Ideal conditions are 20–23°C and 30–40% relative humidity and do not vary by more than ±2°C and ±5% relative humidity over a 24-h period (Papp and United States, 2016). Temperature and relative humidity were recorded every hour using a Kestrel 4500 Pocket Weather Tracker. The drying-weighing process was repeated until the weight difference between the measurements did not exceed 0.07 mg.
2.6. Reflectance spectroscopy
Reflectance spectra of sample filters were recorded using an Ocean Insight FLAME-S-VIS-NIR-ES spectrometer using a 400 μm fiberoptic probe connected to a 20-watt halogen light source. The spectrometer had a wavelength range of 350–1,000 nm, a 1.33 nm optical resolution, and a slit size of 25 μm. The probe was secured in a holder at a 45° angle and 4–6 mm from the surface of the filter to measure diffuse reflectance (Figure 2J). A blank filter placed below the sample filter ensured that its surface was opaque (Arimoto et al., 2002). A reflectance spectrum (e.g., Figure 2K) was acquired for each sample filter at an integration time of 6.24 ms, 25 scans to average, and a boxcar width of 4. Electric dark and nonlinearity correction were activated. The LabSphere WS-1-SL Spectralon diffuse reflectance standard was used as the white reference. Color measurements were acquired using the D65 illuminant standard (average daylight, including ultraviolet wavelengths) at a 10° observer angle (Morozzi et al., 2021). Ten measurements were taken across the surface of each sample filter and method blank to obtain reflectance values and five color parameters: lightness (L*), a*, b*, chroma (Cab*), and hue angle (hab°).
The colorimetric space known as CIE L*a*b* (CIELAB) defines color using Cartesian coordinates for lightness (L*), redness-greenness (a*), and yellowness-blueness (b*). Lightness is the visual perception of luminance and values vary from 0 (black) to 100 (white). Positive values of a* tend toward red and negative toward green. Positive values of b* tend toward yellow and negative toward blue. The colorimetric space known as CIE L*Cab*hab° (CIELCh) uses polar coordinates to define chroma (Cab*) and hue angle (hab°). Chroma is the purity of the color, or its freedom from white or gray. Higher chroma values are purer. Hue angle, given in degrees (0–360), corresponds to an angular position around a central or neutral point. Hue is the color, representing the relative amounts of red, green, and blue.
3. Calculations and statistical analysis
Absolute PM mass (mg) was determined as the difference between filter weights before and after filtration. For the older feathers (PM Old), two method blanks were run for each population and averaged whereas for the newer feathers (PM New) one method blank was run. To obtain blank-corrected total PM accumulation, method blank weights were subtracted from sample filter weights for each population. For each sample, absolute PM mass was divided by total feather surface area (cm2) to calculate PM accumulation per unit feather surface area. One sample from the rural population was excluded from the gravimetric (but not reflectance) analysis as filter media stuck to the weighing pan after drying. Median PM accumulation values are reported in μg/cm2.
The relative accumulation factor (RAF) was calculated to compare differences in time-dependent accumulation: (1) between the populations that were sampled 1 month apart, and (2) within each population by relating PM accumulation on older feathers exposed for approximately 1 year to a newer feather exposed for approximately 45 days (Martínez-Carrillo et al., 2010). RAF was calculated as:
where PMOld is PM accumulated on the year-old sample and PMNew is PM accumulated on the 45-day old sample.
Reflectance measurements for each filter were averaged by wavelength and then divided into ranges: Ultraviolet = 350–400 nm, Visible = 401–779 nm, and Near Infrared = 780–1,000 nm. Total reflectance across the full spectrum, reflectance within each range, and color measurements were averaged for comparison among samples and between populations.
A Shapiro–Wilk test was used to determine if absolute PM, the RAF, reflectance, and color variables were normally distributed. A Wilcoxon rank-sum test was used to test for median differences in absolute PM accumulation, RAF, reflectance, and color measurements between the two sampling locations as these were not normally distributed. Linear regressions between reflectance and color variables and PM accumulation were conducted. All analyses were performed using JMP v16.2 software (SAS Institute Inc.). Significance was set to p < 0.1 due to the small number of populations and individuals sampled and the high within-population variability in feather PM accumulation and particulate reflectance. In addition, due to the exploratory nature of this work, we sought to avoid Type II errors. Given the numerous critiques of p-values in biological studies (Halsey, 2019), including the arbitrary nature of the p < 0.05 level (Murtaugh, 2014), we also report estimated effect sizes.
4. Results
4.1. Particulate matter accumulation
Particulate matter accumulation on feathers ranged from 5.34 to 110.07 μg/cm2 for the rural population (n = 9; median = 11.92 μg/cm2) and from 6.96 to 60.44 μg/cm2 for the urban population (n = 10; median = 11.44 μg/cm2). Median absolute PM accumulation did not differ between the populations (p = 0.775). However, one sample in both populations had very high amounts of PM. Excluding the two outliers did not change the results (p = 0.665). Absolute PM accumulation on the new feathers was 2.74 μg/cm2 for the rural population and 11.26 μg/cm2 for the urban population.
Median RAF for the rural population (3.35) was significantly higher compared to that of the urban population (0.02, p = 0.003). When the two outliers were excluded, the relative PM accumulation between populations remained significantly different (p = 0.002, Figure 3).
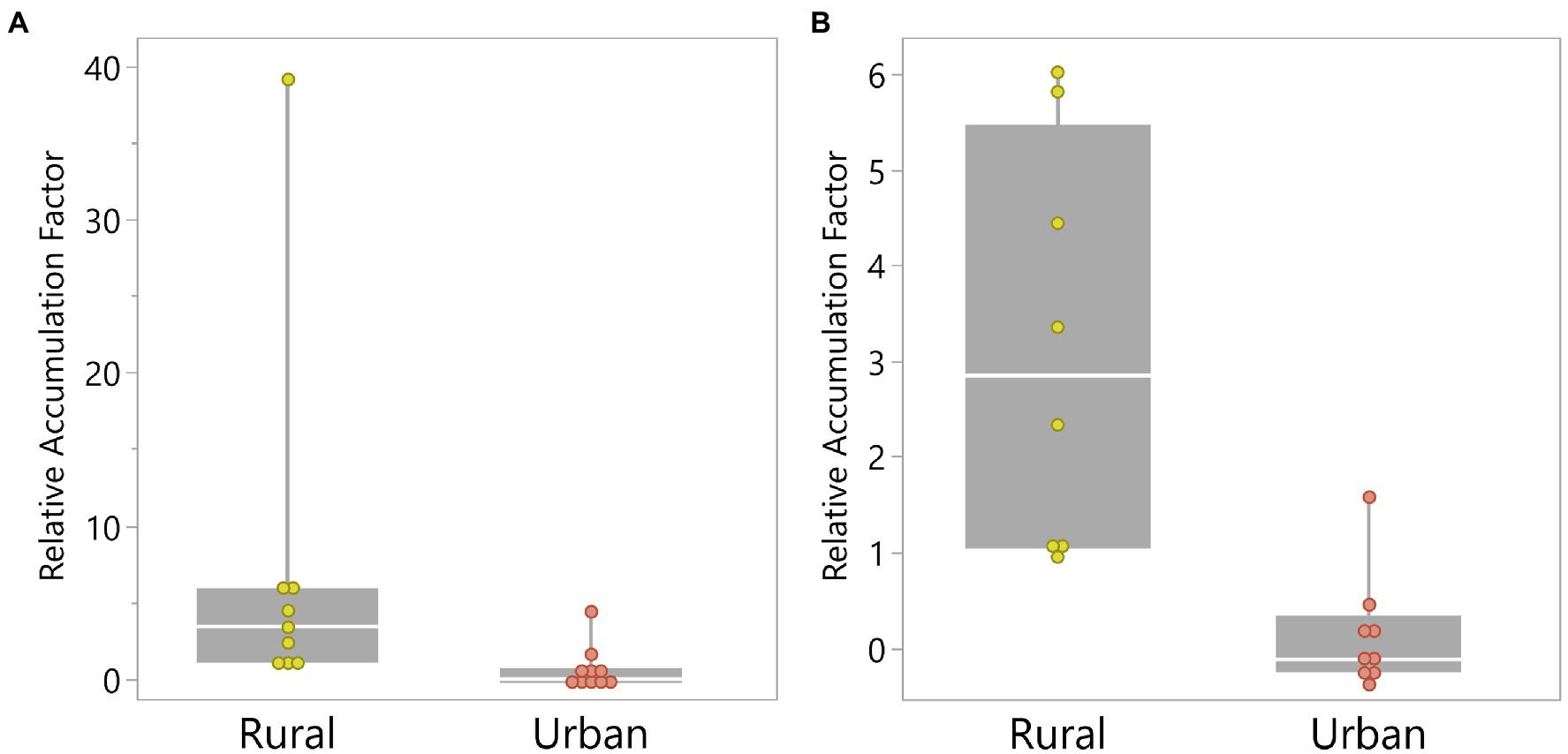
Figure 3. Boxplots of relative accumulation factors (A) with (p = 0.003) and (B) without outliers (p = 0.002) for rural and urban Rock Pigeon populations sampled in Denton County, Texas, in Summer 2020.
4.2. Reflectance and color
Particulates extracted from urban pigeon feathers had lower total reflectance (Z = −1.85, p = 0.064, r = −0.41), lower reflectance in the visible range (Z = −1.85, p = 0.064, r = −0.41), reduced lightness (Z = −1.93, p = 0.054, r = −0.43), and a lower hue angle (Z = −1.78, p = 0.076, r = −0.40) compared to particulates collected from rural pigeon feathers. Differences in chroma were not significant between populations (Z = 0.94, p = 0.345, r = 0.21, Figures 4A,B). The urban population showed moderate and significant negative relationships between PM accumulation and total reflectance (R2 = 0.57, p = 0.011), visible reflectance (R2 = 0.64, p = 0.006), and lightness (R2 = 0.68, p = 0.003). For the rural population, there was a significant but weak negative relationship between hue angle and PM accumulation (R2 = 0.37, p = 0.082). Chroma was positively related to PM accumulation for the urban (R2 = 0.59, p = 0.009) and rural (R2 = 0.37, p = 0.080) populations (Figure 4C).
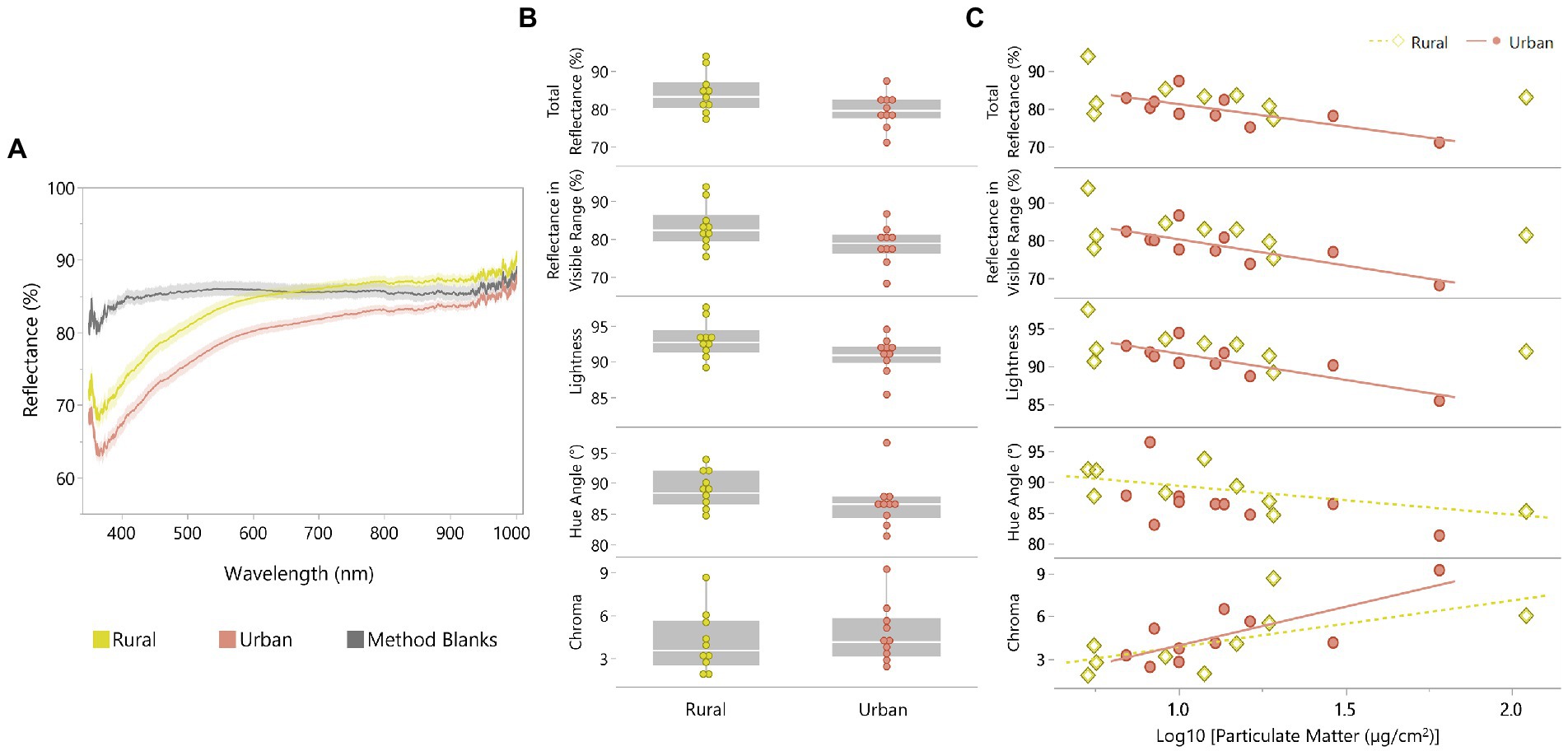
Figure 4. (A) Reflectance spectra of particulate matter (PM) accumulated on glass microfiber filters for rural and urban pigeon populations compared to spectra of control filters. Spectra are smoothed using a Savitzky–Golay filter with a 3rd order polynomial fit. Shaded areas represent a 90% CI. (B) Box plots of spectral properties - total reflectance (350–1,000 nm), reflectance in the visible range (400–779 nm), lightness, hue angle, and chroma for PM from feathers of rural and urban pigeon populations. (C) Relationships between PM accumulation and total reflectance (350–1,000 nm), reflectance in the visible range (400–779 nm), lightness, hue angle, and chroma.
5. Discussion
Findings from this preliminary study suggest that wild birds may incur a pollution penalty for inhabiting urban environments. Urban Rock Pigeon feathers accumulated particulates that were less reflective, darker, and more orange (decreased hue angle) compared to those extracted from rural birds. Although few studies investigate the effects of external particulate accumulation on plumage characteristics, changes in feather properties in response to environmental pollution gradients are well documented. For example, the hue angle of Great Tit (Parus major) feathers decreased along a gradient of increasing metal pollution in Belgium (Dauwe and Eens, 2008). Grunst et al. (2020) found that proximity to roads was negatively related to feather hue: birds near roads had feathers with lower hue angles than birds distant from roads. In contrast to our results, feathers of urban birds have also been shown to be less chromatic than those of their rural counterparts (Dauwe and Eens, 2008; Biard et al., 2017; Grunst et al., 2020). Interestingly, none of these studies detected changes in surface reflectance with increasing environmental pollution.
Numerous factors not measured here are known to affect feather health and, therefore, reflectance and color. In the case of urban pigeons, male and female birds utilize habitat differently, both spatially and temporally (Rose et al., 2006), which may alter their exposure to atmospheric pollutants. Poor nutrition can also degrade bird feathers. For instance, pigeons whose diets were supplemented with lead had reduced iridescent feather brightness while melanic feathers showed reduced reflectance in green wavelengths (Chatelain et al., 2017). High external bacterial loads on pigeons have been found to increase total reflectance of iridescent neck feathers (Leclaire et al., 2014). We also acknowledge that particulates deposited on filters may exhibit spectral properties that are distinctive from those deposited on feather surfaces. The characteristics of feather surfaces with accumulated PM would likely be influenced by the highly variable nature of the feathers themselves, which differ in pigmentation, thickness, nanostructure, and the amount and composition of preen oil. Furthermore, spectral properties may vary under field conditions due to changes in ambient light. Finally, pigeons have varied phenotypes that can occur within a population, making it difficult to control for color. Even feathers collected from one individual can display a range of colors (Figure 2F).
While we cannot rule out the influence of these factors on feathers sampled in this study, our results suggest that PM accumulation on urban bird feathers may contribute to reduced total and visible feather reflectance and lightness as indicated by moderate to strong relationships between these variables and low p-values (Supplementary Table 1). Previous studies support these findings. DuBay and Fuldner (2017) found that bird specimens from the first decade of the 20th century were darker in color whereas modern specimens (1960–2015) were significantly lighter due to lower levels of soot accumulation. Iridescent feathers of European Starlings (Sturnus vulgaris) experimentally exposed to open air in a heavily polluted region of Italy showed decreased reflectance in UV and visible wavelengths. This effect became stronger as duration of exposure increased and was especially pronounced for UV wavelengths, indicating a greater impact on shortwave structural colors (Griggio et al., 2011). Our data also show a strong negative relationship between PM accumulation and reflectance in the visible portion of the spectrum for urban feather particulates. We did not observe a similar relationship in the rural population even though the rate of PM accumulation was higher, suggesting that urban bird feathers collected a higher proportion of light-absorbing particles. In a previous study in the same urban area, high accumulations of black carbon were measured on Domestic Chicken (Gallus domesticus) feathers experimentally exposed to ambient air near a highway (Pitre et al., 2021).
The unique characteristics of the sites could help to further explain the differences in feather PM accumulation rate and particulate reflectance and color we observed between populations (Supplementary Table 2). The rural site is surrounded by agriculture, pasture, and herbaceous cover, has low building density, and is more exposed to wind. At this site, pigeons were observed foraging in a parking lot with paved and gravel dust surfaces. The urban site has a higher density of built structures that shelter the area from wind but has a higher prevalence of idling vehicles. Thus, we speculate that rural pigeons accumulated more wind-driven dust whereas urban pigeons accumulated more traffic-related pollutants. Because larger (dust) particles weigh more than fine (combustion) particles, this conjecture is consistent with the higher rate of PM accumulation on rural pigeon feathers. It is worth noting that the presence of major roads near both sites may have muted the urban–rural difference between the two groups of pigeon feathers.
In our preliminary study, we find that p-values <0.1 are suggestive of a significant effect of PM on feather reflectance and color. However, we encourage further research to demonstrate unequivocally the relationship between urban-rural differences in PM accumulation and feather spectral properties. A larger study with more paired urban-rural sites and a more rigorous statistical approach are both needed to confirm the pattern revealed by our preliminary findings.
Additional research is warranted given the potential for PM-induced changes in reflectance and color to affect bird health. Differences among conspecifics in reflectance and color are indicators of individual quality and fitness as their expression is a result of body condition (D’Alba et al., 2011; Cantarero et al., 2016; reviewed in White, 2020). Maintaining these honest signals of fitness is costly. Birds invest time and energy in preening and preen oil production (Leclaire et al., 2014), an effort which may necessarily increase if birds inhabit environments with heavy air pollution (Griggio et al., 2011). While external PM accumulation may directly darken feathers (Griggio et al., 2011; DuBay and Fuldner, 2017), inhalation of PM affects feather condition as well by adversely affecting bird health via respiratory distress, illness, immunosuppression, and impaired reproductivity (Sanderfoot and Holloway, 2017). Consequently, the cumulative effects of PM exposure may be severe in terms of impact on fitness and population viability.
We have shown that particulates collected from the feathers of urban pigeons exhibit reduced lightness, reflectance, and hue which may affect feather spectral properties. This, in turn, may alter honest signals of individual fitness, thereby affecting mate selection, camouflage, and communication as feather spectral properties are key components in these behaviors (Griggio et al., 2011). As urban areas grow, particle pollution will continue to pose a threat, and pollution penalty, to the physiological and behavioral health of birds.
Data availability statement
The dataset related to this article is hosted on the Knowledge Network for Biocomplexity, https://doi.org/10.5063/F14B2ZRB.
Ethics statement
This animal study was reviewed and approved by the University of North Texas Institutional Animal Care and Use Committee (IACUC).
Author contributions
All authors contributed to conception of the study. JE, AP-G, and JJ developed the study methodology. JE and AP-G performed statistical analysis and wrote the first draft of the manuscript. All authors contributed to the article and approved the submitted version.
Funding
This work was supported by the National Science Foundation (CAREER #1552410), the University of North Texas Toulouse Graduate School and the University of North Texas Department of Geography and the Environment.
Acknowledgments
We thank Bethel Steele for invaluable help in developing the methodology and Shane DuBay for constructive feedback. For their generosity in allowing us access to their properties to spend hours observing and trapping pigeons, we would like to thank the citizens and representatives of the City of Denton and Denton County.
Conflict of interest
The authors declare that the research was conducted in the absence of any commercial or financial relationships that could be construed as a potential conflict of interest.
Publisher’s note
All claims expressed in this article are solely those of the authors and do not necessarily represent those of their affiliated organizations, or those of the publisher, the editors and the reviewers. Any product that may be evaluated in this article, or claim that may be made by its manufacturer, is not guaranteed or endorsed by the publisher.
Supplementary material
The Supplementary material for this article can be found online at: https://www.frontiersin.org/articles/10.3389/fevo.2023.946624/full#supplementary-material
References
Apte, J., Seraj, S., Chambliss, S., Hammer, M., Southerland, V., Anenberg, S., et al. (2021). Air inequality: global divergence in urban fine particulate matter trends. ChemRxiv. Preprint. doi: 10.26434/chemrxiv.14671908.v1.
Arimoto, R., Balsam, W., and Schloesslin, C. (2002). Visible spectroscopy of aerosol particles collected on filters: iron-oxide minerals. Atmos. Environ. 36, 89–96. doi: 10.1016/S1352-2310(01)00465-4
Biard, C., Brischoux, F., Meillere, A., Michaud, B., Niviere, M., Ruault, S., et al. (2017). Growing in cities: an urban penalty for wild birds? A study of phenotypic differences between urban and rural great tit chicks (Parus major). Front. Ecol. Evol. 5:79. doi: 10.3389/fevo.2017.00079
Borghesi, F., Dinelli, E., Migani, F., Béchet, A., Rendón-Martos, M., Amat, J. A., et al. (2016). Assessing environmental pollution in birds: a new methodological approach for interpreting bioaccumulation of trace elements in feather shafts using geochemical sediment data. Methods Ecol. Evol. 8, 96–108. doi: 10.1111/2041-210X.12644
Buysse, C. E., Kaulfus, A., Nair, U., and Jaffe, D. A. (2019). Relationships between particulate matter, ozone, and nitrogen oxides during urban smoke events in the western US. Environ. Sci. Technol. 53, 12519–12528. doi: 10.1021/acs.est.9b05241
Cantarero, A., Laaksonen, T., Järvistö, P. E., López-Arrabé, J., Gil, D., and Moreno, J. (2016). Testosterone levels in relation to size and UV reflectance of achromatic plumage traits of female pied flycatchers. J. Avian Biol. 48, 243–254. doi: 10.1111/jav.01032
Chatelain, M., Pessato, A., Frantz, A., Gasparini, J., and Leclaire, S. (2017). Do trace metals influence visual signals? Effects of trace metals on iridescent and melanic feather colouration in the feral pigeon. Oikos 126, 1542–1553. doi: 10.1111/oik.04262
D’Alba, L., van Hemert, C., Handel, C. M., and Shawkey, M. D. (2011). A natural experiment on the condition-dependence of achromatic plumage reflectance in black-capped chickadees. PLoS One 6:e25877. doi: 10.1371/journal.pone.0025877.g004
Dauwe, T., and Eens, M. (2008). Melanin- and carotenoid-dependent signals of great tits (Parus major) relate differently to metal pollution. Naturwissenschaften 95, 969–973. doi: 10.1007/s00114-008-0400-1
DuBay, S. G., and Fuldner, C. C. (2017). Bird specimens track 135 years of atmospheric black carbon and environmental policy. Proc. Natl. Acad. Sci. U. S. A. 114, 11321–11326. doi: 10.1073/pnas.1710239114
Ferreira, T., and Rasband, W. (2012). The ImageJ user guide — version 1.46r. Available at: http://imagej.nih.gov/ij/docs/guide/index.html (Accessed May 21, 2021).
Griggio, M., Serra, L., and Pilastro, A. (2011). The possible effect of dirtiness on structurally based ultraviolet plumage. Ital. J. Zool. 78, 90–95. doi: 10.1080/11250003.2010.504238
Grunst, M. L., Grunst, A. S., Pinxten, R., Bervoets, L., and Eens, M. (2020). Carotenoid- but not melanin-based plumage coloration is negatively related to metal exposure and proximity to the road in an urban songbird. Environ. Pollut. 256:113473. doi: 10.1016/j.envpol.2019.113473
Halsey, L. G. (2019). The reign of the p-value is over: what alternative analyses could we employ to fill the power vacuum? Biol. Lett. 15:20190174. doi: 10.1098/rsbl.2019.0174
Hill, G. E., and McGraw, K. J. (2006). Bird Coloration. Mechanisms and Measurements. Vol. 1. Cambridge: Harvard University Press.
Homer, C., Dewitz, J., Jin, S., Xian, G., Costello, C., Danielson, P., et al. (2020). Conterminous United States land cover change patterns 2001–2016 from the 2016 National Land Cover Database. ISPRS J. Photogramm. Remote Sens. 162, 184–199. doi: 10.1016/j.isprsjprs.2020.02.019
Jin, S., Homer, C., Yang, L., Danielson, P., Dewitz, J., Li, C., et al. (2019). Overall methodology design for the United States National Land Cover Database 2016 products. Remote Sens. 11:2971. doi: 10.3390/rs11242971
Leclaire, S., Pierret, P., Chatelain, M., and Gasparini, J. (2014). Feather bacterial load affects plumage condition, iridescent color, and investment in preening in pigeons. Behav. Ecol. 25, 1192–1198. doi: 10.1093/beheco/aru109
Leveau, L. M. (2021). United colours of the city: a review about urbanization impact on animal colours. Austral Ecol. 46, 670–679. doi: 10.1111/aec.13005
Lowther, P. E., and Johnston, R. F. (2020). “Rock Pigeon (Columba livia), version 1.0” in Birds of the World. ed. S. M. Billerman (Ithaca, NY: Cornell Lab of Ornithology).
Luce, B. W., Barrett, T. E., and Ponette-González, A. G. (2020). Student cyclists experience PM2.5 pollution hotspots around and urban university campus. Geograph. Bull. 61, 105–113.
Mallet-Rodrigues, F. (2012). Replacement and growth of primary feathers in captive rock pigeons, Columba livia (Aves: Columbidae). Zoologia 29, 121–125. doi: 10.1590/S1984-46702012000200004
Martínez-Carrillo, M. A., Solís, C., Andrade, E., Isaac-Olivé, K., Rocha, M., Murrillo, G., et al. (2010). PIXE analysis of Tillandsia usneoides for air pollution studies at an industrial zone in Central Mexico. Microchem. J. 96, 386–390. doi: 10.1016/j.microc.2010.06.014
Morozzi, P., Ballarin, B., Arcozzi, S., Brattich, E., Lucarelli, F., Nava, S., et al. (2021). Ultraviolet-visible diffuse reflectance spectroscopy (UV-DRS), a rapid and non-destructive analytical tool for the identification of Saharan dust events in particulate matter filters. Atmos. Environ. 252:118297. doi: 10.1016/j.atmosenv.2021.118297
National Research Council (2010). Global Sources of Local Pollution: An Assessment of Long-Range Transport of Key Air Pollutants to and from the United States. Washington, DC: The National Academies Press.
North, M. A., Kinniburgh, D. W., and Smits, J. E. G. (2017). European starlings (Sturnus vulgaris) as sentinels of urban air pollution: a comprehensive approach from noninvasive to post mortem investigation. Environ. Sci. Technol. 51, 8746–8756. doi: 10.1021/acs.est.7b01861
Papp, M., United States (2016). Quality assurance guidance document 2.12: Monitoring PM2.5 in ambient air using designated reference or Class I equivalent methods. Available at: https://permanent.fdlp.gov/gpo69921/m212.pdf (Accessed June 6, 2021).
Pitre, C., Ponette-González, A. G., Rindy, J. E., Lee, A., Doherty, D., Fry, M., et al. (2021). Bird feathers are potential biomonitors for airborne elemental carbon. Environ. Monit. Assess. 193:35. doi: 10.1007/s10661-020-08804-2
Ponette-González, A. G., Chen, D., Elderbrock, E., Ko, Y., Lee, J., Rindy, J. E., et al. (2022). Urban edge trees: urban form and meteorology drive elemental carbon deposition to canopies and soils. Environ. Pollut. 314:120197. doi: 10.1016/j.envpol.2022.120197
Rose, E., Nagel, P., and Haag-Wackernagel, D. (2006). Spatio-temporal use of the urban habitat by feral pigeons (Columba livia). Behav. Ecol. Sociobiol. 60, 242–254. doi: 10.1007/s00265-006-0162-8
Sanderfoot, O. V., Bassing, S. B., Brusa, J. L., Emmet, R. L., Gillman, S. J., Swift, K., et al. (2022). A review of the effects of wildfire smoke on the health and behavior of wildlife. Environ. Res. Lett. 16:123003. doi: 10.1088/1748-9326/ac30f6
Sanderfoot, O. V., and Holloway, T. (2017). Air pollution impacts on avian species via inhalation exposure and associated outcomes. Environ. Res. Lett. 12:16. doi: 10.1088/1748-9326/aa8051
Standard Methods Committee of the American Public Health Association, American Water Works Association, and Water Environment Federation (2018). “2540 solids” in Standard Methods for the Examination of Water and Wastewater. eds. W. C. Lipps, T. E. Baxter, and E. Braun-Howland (Washington, DC: APHA Press)
Sun, F., Dai, Y., and Yu, X. (2017). Air pollution, food production and food security: a review from the perspective of food system. J. Integr. Agric. 16, 2945–2962. doi: 10.1016/S2095-3119(17)61814-8
Torres, A., Bond, T. C., Lehmann, C. M. B., Subramanian, R., and Hadley, O. L. (2016). Measuring Organic Carbon and Black Carbon in Rainwater: Evaluation of Methods (Version 1). Taylor & Francis.
United States Census Bureau (2020). QuickFacts Denton County, Texas; Denton city, Texas. Available at: https://www.census.gov/quickfacts/fact/table/dentoncountytexas,dentoncitytexas/LND110210 (Accessed March 2020).
United States Environmental Protection Agency (2021). Particulate Matter (PM) Basics. Available at: https://www.epa.gov/pm-pollution/particulate-matter-pm-basics (Accessed May 26, 2021).
White, T. E. (2020). Structural colours reflect individual quality: a meta-analysis. Biol. Lett. 16:20200001. doi: 10.1098/rsbl.2020.0001
World Health Organization (WHO) European Centre for Environment and Health (2013). Review of Evidence on Health Aspects of Air Pollution – REVIHAAP Project Technical Report. Copenhagen, Denmark: WHO Regional Office for Europe.
Yang, L., Jin, S., Danielson, P., Homer, C., Gass, L., Bender, S. M., et al. (2018). A new generation of the United States National Land Cover Database: requirements, research priorities, design, and implementation strategies. ISPRS J. Photogramm. Remote Sens. 146, 108–123. doi: 10.1016/j.isprsjprs.2018.09.006
Keywords: air quality, birds, cities, feathers, pollution penalty, spectrometry, urban-rural
Citation: Ellis JL, Ponette-González AG, Fry M and Johnson JA (2023) Reduced reflectance and altered color: The potential cost of external particulate matter accumulation on urban Rock Pigeon (Columba livia) feathers. Front. Ecol. Evol. 11:946624. doi: 10.3389/fevo.2023.946624
Edited by:
Federico Morelli, Czech University of Life Sciences Prague, CzechiaReviewed by:
Heather Proctor, University of Alberta, CanadaFranco Leandro de Souza, Federal University of Mato Grosso do Sul, Brazil
Copyright © 2023 Ellis, Ponette-González, Fry and Johnson. This is an open-access article distributed under the terms of the Creative Commons Attribution License (CC BY). The use, distribution or reproduction in other forums is permitted, provided the original author(s) and the copyright owner(s) are credited and that the original publication in this journal is cited, in accordance with accepted academic practice. No use, distribution or reproduction is permitted which does not comply with these terms.
*Correspondence: Jennifer L. Ellis, ✉ amVuZWxsaXNAdHV0YW5vdGEuY29t
†Present address: Alexandra G. Ponette-González, Department of City and Metropolitan Planning & Natural History Museum of Utah, University of Utah, Salt Lake City, UT, United States; Matthew Fry, Department of Geography, University of Utah, Salt Lake City, UT, United States