- 1Key Laboratory of Songliao Aquatic Environment, Ministry of Education, Jilin Jianzhu University, Changchun, Jilin, China
- 2School of Geology and Mining Engineering, Xinjiang University, Urumchi, China
- 3Key Laboratory of Wetland Ecology and Environment, Northeast Institute of Geography and Agroecology, Chinese Academy of Sciences, Changchun, Jilin, China
- 4Key Laboratory for Comprehensive Energy Saving of Cold Regions Architecture of Ministry of Education, Jilin Jianzhu University, Changchun, China
Heavy metal dynamics at the sediment-water interface (SWI) has attracted plenty of attention due to their meticulous depiction for metal sorption-release processes. However, little is known about their concentration, migration and release characteristics underneath the ice, especially at the millimeter scale. Here we investigated dynamics of labile As, Cd, Cu, Mn, Pb and Zn by the diffusive gradients in thin-films (DGT) technique during ice-covered and ice-free periods in the Lake Xingkai basin. The concentrations of metals were relatively high at the SWI and ranged for As: 0.001~13.42 μg L−1, Cd: 0.01~0.45 μg L−1, Cu: 0.001~2.75 μg L−1, Mn: 5.31~2958.29 μg L−1, Pb: 0.06~1.43 μg L−1, and Zn: 2.92~112.96 μg L−1. Particularly, concentration of Mn was extremely higher than other studies, possibly due to diagenetic process. Labile concentrations of heavy metals in January were significantly lower than those in May, suggesting that elevated temperatures lead to the release of heavy metals from the sediment to the overlying water. Based on the Fick’s first law, the diffusive fluxes as a source of Mn (413.82-1163.25 mg·m-2·d-1) and As (3.53 -8.12 mg·m-2·d-1) indicated that heavy metals were released from sediments to the overlying water. While the diffusive fluxes as a sink of Zn (-1.80-(-2.36) mg·m-2·d-1) and Pb (-0.02-(-0.46) mg·m-2·d-1) to sediments. Redundancy Analysis (RDA) revealed that the main factors influencing the heavy metal migration were dissolved oxygen (45.6% of total explanation, P=0.01) in January, and water temperature (52.9%, P=0.006) in May. This study extends theoretical scope for understanding metal migration and release process, and provides valuable suggestions for lake management during the freezing period.
1 Introduction
In the northern hemisphere, lakes are notably characterized by their ice coverage in winter. Owing to climate change-induced alterations, the extent and persistence of ice cover have notably decreased, necessitating a comprehensive investigation of the ecological dynamics occurring beneath these ice cover (Beyene and Jain, 2020; Bartosiewicz et al., 2021). Subsequent to the formation of ice cover, there occur drastic shifts in both physical (e.g., a marked decrease in solar radiation, oxygen concentration, and water temperature) and chemical processes (including decreased flow and interaction of matter and energy), that critically modify a multitude of lake processes (Ptak and Sojka, 2021; Yang et al., 2021).
The impact of ice cover on lake ecosystems is significant, particularly in colder regions where latent heat from freezing and thawing plays a major role. This ice cover alters the climatic, ecological, and hydrological characteristics of the surrounding environment. The formation of ice stops the flux of momentum from the atmosphere into the lake, resulting in a laminar flow regime and reduced vertical diffusion. With the onset of ice cover, solar radiation decreases sharply, shifting the ecosystem to a winter mode. This also reduces the oxygen concentration in the water due to diminished atmospheric contact and the consumption of oxygen by the decomposition of organic materials. The ice cover isolates the water body from the external environment, significantly altering many processes such as hydrodynamics and light supply (Ptak and Sojka, 2021). Studies have shown that during ice formation, a majority of total dissolved solids are excluded from the ice, remaining in the water beneath (Leppäranta et al., 2003; Zhang et al., 2012). This leads to higher concentrations of nutrients in the underlying water layer during freeze-thaw cycles, as identified in glacial lakes. Additionally, during the ice-on period, both lake temperature and solar radiation are low, slowing down the matter and energy flow in the lake ecosystem. The formation of ice cover results in the consolidation of pure water into ice, while nutrients and impurities are concentrated in the water below (Zhang et al., 2012; Song et al., 2019). The changes in concentration under the ice profoundly affect the lake ecosystem during the winter period.
The pollution of aquatic ecosystems by heavy metals remains challenging due to their toxic effects, long-term presence, and bioaccumulative properties (Klavinš et al., 2000; Fu et al., 2014). It has been discovered that under the “concentration effect,” the sub-ice water stratum retains approximately 80% of all dissolved substances, resulting in the total manganese (TMn) concentration in the water layer becoming 0.27 times higher post-freezing (Leppäranta et al., 2003; Zhang et al., 2021). However, despite the known challenges posed by heavy metal pollution and the intriguing ‘concentration effect’ under ice, our current understanding of in-situ concentrations, migrations, and release characteristics during the ice cover phase is notably restricted due to limited research on sub-ice heavy metal concentrations.
The sediment-water interface (SWI) zone in lake ecosystems is a dynamic frontier characterized by significant vertical variations in physical and chemical parameters (Yuan et al., 2022). At this interface, mass transport and energy exchange constantly take place within confined spaces (Liu et al., 2022). Redox-sensitive metals, serving as electron acceptors, are released from the surface sediment strata into the overlying water at the SWI (Canfield, 1993). However, these metals in the overlying water can readily bind to suspended particulate matter, leading to their subsequent resettlement onto the surface sediments (Zhang et al., 2018).
Consequently, processes of deposition, mixing, and removal of various elements at the SWI play a pivotal role in the translocation of pollutants. Previous research using the Diffusive Gradients in Thin films (DGT) technique at the SWI has indicated that high labile Mn concentrations in the overlying water, reaching up to 22.3 μg L−1 (Yuan et al., 2018), with sediments identified as sources of heavy metals such as Pb, Zn, and Cd, particularly in the weakly mixed zone (Wang and Wang, 2017; Zhang H. et al., 2022; Liu et al., 2023).
Historically, research has primarily focused on the quantification of total metal content in solid forms rather than labile metal concentrations (Fan et al., 2019). Indeed, the bioavailability and mobility of heavy metals in sediments are the primary factors determining their potential toxicity (Zhang et al., 2001; Zhang M. et al., 2020). Prior studies on the migration and biological effects of heavy metals, using traditional methodologies like the European Community Bureau of Reference method, have been unable to fully capture the chemical and biological modifications in sediments throughout the collection, transportation, storage, and handling processes (Pan et al., 2019).
The diffusive gradients in thin films (DGT) methodology, an in-situ passive sampling technique, can effectively measure the bioavailable states of heavy metals, including free metal ions and unstable inorganic and organic complexes, with minimal environmental disruption and at low cost (Davison and Zhang, 1994; Eismann et al., 2020; Guan et al., 2021).
DGT has been widely employed as an in-situ passive sampling technique to assess the environmental metal chemistry at the SWI. The methodology has been extensively applied in various regions China, such as the Three Gorges Reservoir (Gao et al., 2018), in Lake Taihu (Yuan et al., 2018),in Lake Dianchi and WuliangSuhai (Wu et al., 2019; Li et al., 2022; Zhang H. et al., 2022; Zheng et al., 2022) in China. Nevertheless, limited research has been conducted on lakes in Northeast China, highlighting the need for increased focus on this geographical area.
The Lake Xingkai (LXK)/Khanka basin, the largest freshwater lake in Northeast Asia, situates on the Chinese–Russian border. It consists of LXK and Lake Xiao Xingkai (LXX) (Yuan et al., 2018). Given their contrasting sizes, eutrophication levels, and the number of inflowing rivers, these lakes present an ideal natural experiment for investigating variations in biogeochemical processes of nutrients and metals. Considering the globally observed decrease in ice cover frequency and duration in northern hemisphere lakes due to climate change, the concentrations of bioavailable metals at the SWI remain uncertain. Currently, there is limited research on heavy metals in the Xingkai Lake basin. Previous research indicates that Lake Xingkai and Lake Xiaoxingkai generally maintain uncontaminated levels with moderate ecological risks; however, compared to Xingkai Lake, Xiaoxingkai Lake exhibits higher contamination levels and potential ecological risks, primarily attributed to human activities and hydrological connectivity (Jiang et al., 2022).
A primary question that arises is whether the sediment acts as a source or sink for heavy metals during these periods. Additionally, the understanding of variations in the migration patterns and the driving factors influencing heavy metal concentrations between ice-covered and subsequent ice-free periods is lacking. This study proposes the hypothesis of a significant potential release of metals below ice due to the concentration effect. Our study aims to further illuminate the ecological implications of heavy metal distribution at the SWI, thereby providing crucial insights for the management of lakes during ice-covered periods.
2 Materials and methods
2.1 Study area
The LXK basin, is located in northeast China (44° 30′–45° 30′ N, 132° 00′–132° 50′ E; Figure 1), is part of the Sanjiang Plain and includes LXX, LXK and the inflowing rivers (e.g., River Chengzi and River Xidi) (Wu et al., 2013; Yuan et al., 2018). LXK covers a total surface area of 4380 km2, of which 1080 km2 is situated in China. It spans 90 km in length and 50 km in width, with the deepest point reaching 10 m and maintaining an average depth of 4.5 m. Meanwhile, LXX is 35 km long, 4.5 km wide, and has an average depth of 1.8 m. These two bodies of water are connected via two sluice gates (Sluice 1 and Sluice 2) (Yu et al., 2021).
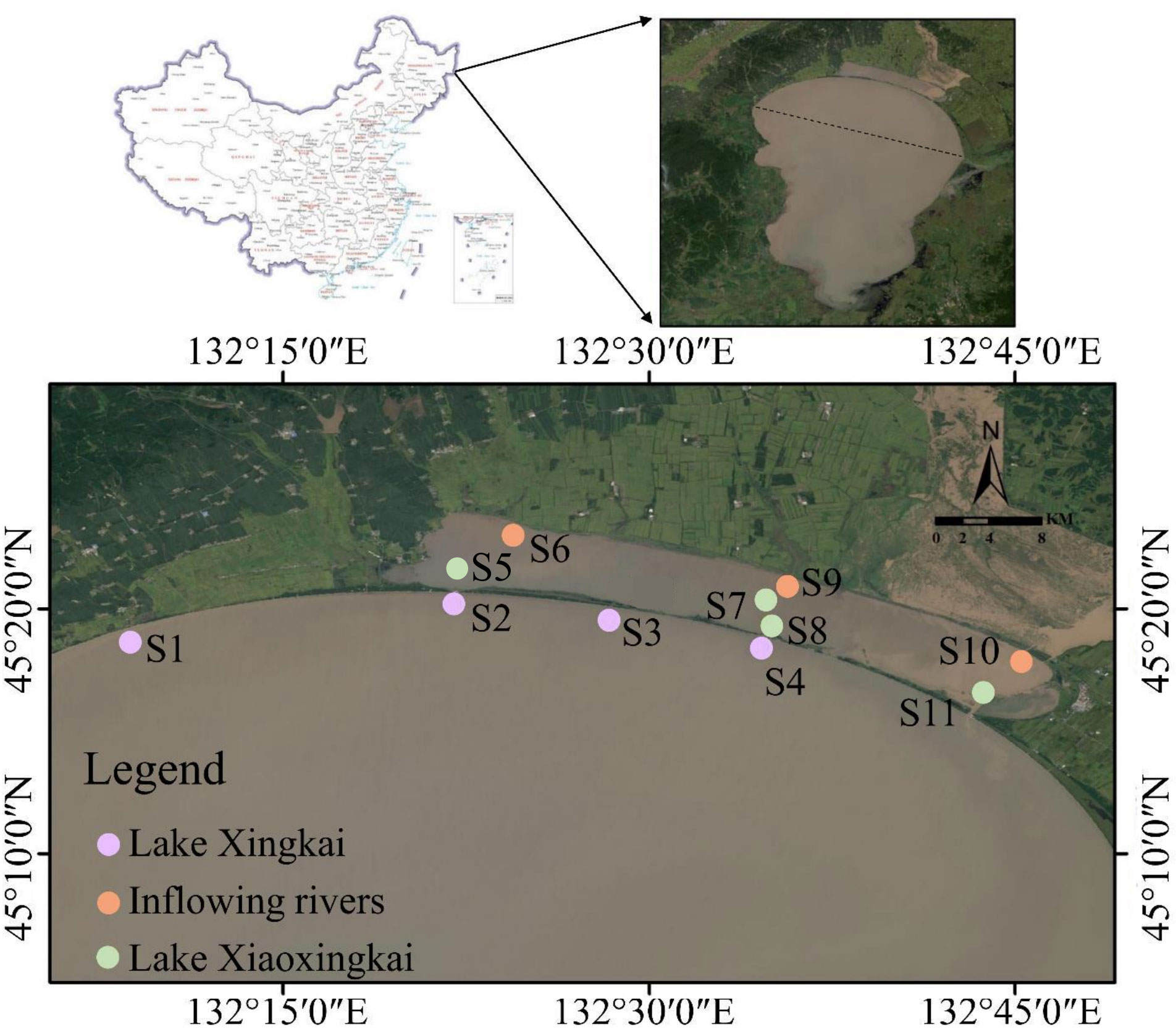
Figure 1 Location of sampling sites. S1 (Baipaozi), S2 (Xinkailiu beach in Lake Xingkai), S3 (Baiyutan) and S4 (the first gate in Lake Xingkai) are located in Lake Xingkai. S5 (Xinkailiu Wharf in Xiaoxingkai), S7 (center of Lake Xiao Xingkai), S8 (Sluice 1 in Lake Xiao Xingkai) and S11 (Sluice 2 in Lake Xiao Xingkai) are located in Lake Xiao Xingkai. S6 (River Chengzi), S9 (River Xidi) and S10 (Sanshiyilian) are located in the inflowing rivers.
This basin receives an annual precipitation volume averaging at 540 mm, with the majority (~70%) concentrated between June and September. Temperatures exhibit distinct seasonal fluctuations, with averages of 27°C in the summer and -19°C in the winter. Typically, ice formation occurs over both LXK and LXX from late October and extends through to next April (Sun et al., 2018).
2.2 Field sampling and analysis
2.2.1 Gathering of sediment cores
Eleven sediment cores were obtained across LXK (S1–S4), LXX (S5, S7, S8, and S11), and the inflowing rivers (S6, S9, and S10) during January and May 2021. This was accomplished using gravity corers equipped with plexiglass tubes (8 cm diameter, 60 cm long) (Figure 1). Ice drilling in January was facilitated by a gas-powered auger, and the collected sediment cores ranged from 30–40 cm in length, surmounted by approximately 20 cm of overlying water. These cores were securely sealed using rubber plugs before transportation to the laboratory for subsequent analysis. An EXO2 portable multiparametric water quality analyzer (YSI, Yellow Springs, OH, USA) was utilized to examine the water temperature, dissolved oxygen, conductivity, pH, chlorophyll-a, and redox potential (Eh). The concentration of dissolved organic carbon was determined by the total organic carbon analyzer (Shimadzu, Kyoto, Japan).
2.2.2 Preparation of DGT probes
All probes deployed in this study were supplied by EasySensor (Nanjing, China). The ZrO–Chelex–AgI binding gel was prepared following the protocol described by Xu et al. (2012). The DGT probe assembly included a ZrO–Chelex–AgI mixed binding gel (0.4 mm), an agarose diffusive gel (0.8 mm), and a Durapore membrane (0.1 mm) (Ding et al., 2016). Before deployment, the DGT probes were stored in an oxygen-free NaCl solution (0.01 mol L−1) and deoxygenated with nitrogen for over 16 hours (Gillmore, 2021).
2.2.3 DGT probe deployment and retrieval
After stabilizing for 24 hours, the sediment cores were subjected to a 72-hour vertical insertion of the DGT probe. At each sampling site, water temperature was measured in triplicate throughout the duration of the deployment period. Concurrently, the position of the SWI was documented and all probes were retrieved and cleaned with deionized water.
2.2.4 Sample analysis
The binding layers of the DGT probes were carefully separated, and then sliced at 2 mm intervals using a ceramic cutter. The gel samples were eluted using 1.8 mL HNO3 (1 mol L−1) for 24 hours in order to extract the cations accumulated on the membrane (Zhang et al., 2014). The linear concentration gradients of As, Cd, Cu, Mn, Pb and Zn through the diffusion layer were established (Li et al., 2019). During deployment, the labile metals, such as free ions and unstable complexes capable of dissolving to liberate mobile sediment components, migrate through the agarose diffusion layer and become securely anchored onto the binding gels. Consequently, based on Fick’s first law, CDGT can be determined through Equation 1, which is intrinsically linked to the establishment of a steady-state concentration gradient (μg L−1) (Zhang M. et al., 2020):
where M is the mass of the metal absorbed in the binding layer (μg), Δg represents the thickness of both the filter membrane and the diffusive layer (cm), A is the area of exposed gel in the DGT probes (cm2), D is the metal’s diffusive layer’s (cm2 s-1) diffusion coefficient, and t (s) is the deployment time.
The mass of metals (M) that has accumulated within the resin gel can be determined by Equation 2, which necessitates measuring the concentrations in the elution solution (Ce):
where Vgel is the volume of the binding gel (mL), Vacid is the volume of acid used for elution (mL) and fe is the elution factor (0.8) determined via an elution recovery test (Zhang et al., 2020a).
To estimate the direction and size of the metals’ diffusion, we evaluated the apparent flux between the sediment and the overlying water. The diffusive flux near the SWI was determined according to the method of (Ding et al., 2015). The total diffusive flux (F, μg cm−2 s−1) was determined by adding the diffusion fluxes originating from the overlying water to the SWI and those from the sediment to the SWI.
In this Equation 3, F (μg cm−2 s−1) represents the sum of the overlying water and surface sediment fluxes, while Fw (μg cm−2 s−1) and Fs (μg cm−2 s−1) correspond to the fluxes of labile heavy metals from the overlying water and the sediment to the SWI, respectively. and represent the concentration gradients in the overlying water and the sediment, respectively. We calculated the gradients based on a distance of 3 cm from the SWI (x = 0 cm). Dw (cm2 s−1) is the diffusion coefficient of metals in the overlying water. φ is the porosity in the surface sediment. Ds (cm2 s−1) is the diffusion coefficient of the metals in the sediment.
2.3 Statistical analysis
To explore the association of the heavy metals in January and May and assess if there was a significant effect among the various heavy metals, Pearson’s correlation analysis and one-way analysis of variance (ANOVA) were performed with SPSS 25. Using the vegan package in R (Version 4.1.1), redundancy analysis (RDA) was carried out to investigate the association between environmental conditions and labile metal concentrations. LXK (S1–S4), LXX (S5, S7, S8, and S11), and the inflowing rivers (S6, S9, and S10) during January and May 2021. For data processing, we calculated the average values for each group based on the data from the respective sampling points within that group. This approach ensures a comprehensive analysis of each region while maintaining clarity in data presentation.
3 Results
3.1 Profile distribution of heavy metals at the SWI in the ice-covered and ice-free periods
The physicochemical parameters of LXK, LXX and the inflowing rivers were shown in Table 1. The conductivity ranged from 61.31 to 289.7 S cm−1 (216.98 ± 58.92 S cm−1 in January; 108.58 ± 30.35 S cm−1 in May), dissolved oxygen (DO) from 8.83 to 14.69 mg L−1 (9.70 ± 0.58 mg L−1 in January; 13.33 ± 0.70 mg L−1 in May), pH from 7.1 to 9.01 (7.29 ± 0.07 in January; 8.63 ± 0.3 in May), turbidity from 8.27 to 79.9 FNU (28.72 ± 25.39 FNU in January; 45.06 ± 21.67 FNU in May) and oxidation-reduction potential from −13.6 to 84.3 mV (−5.49 ± 8.47 mV in January; 70.16 ± 9.27 mV in May).
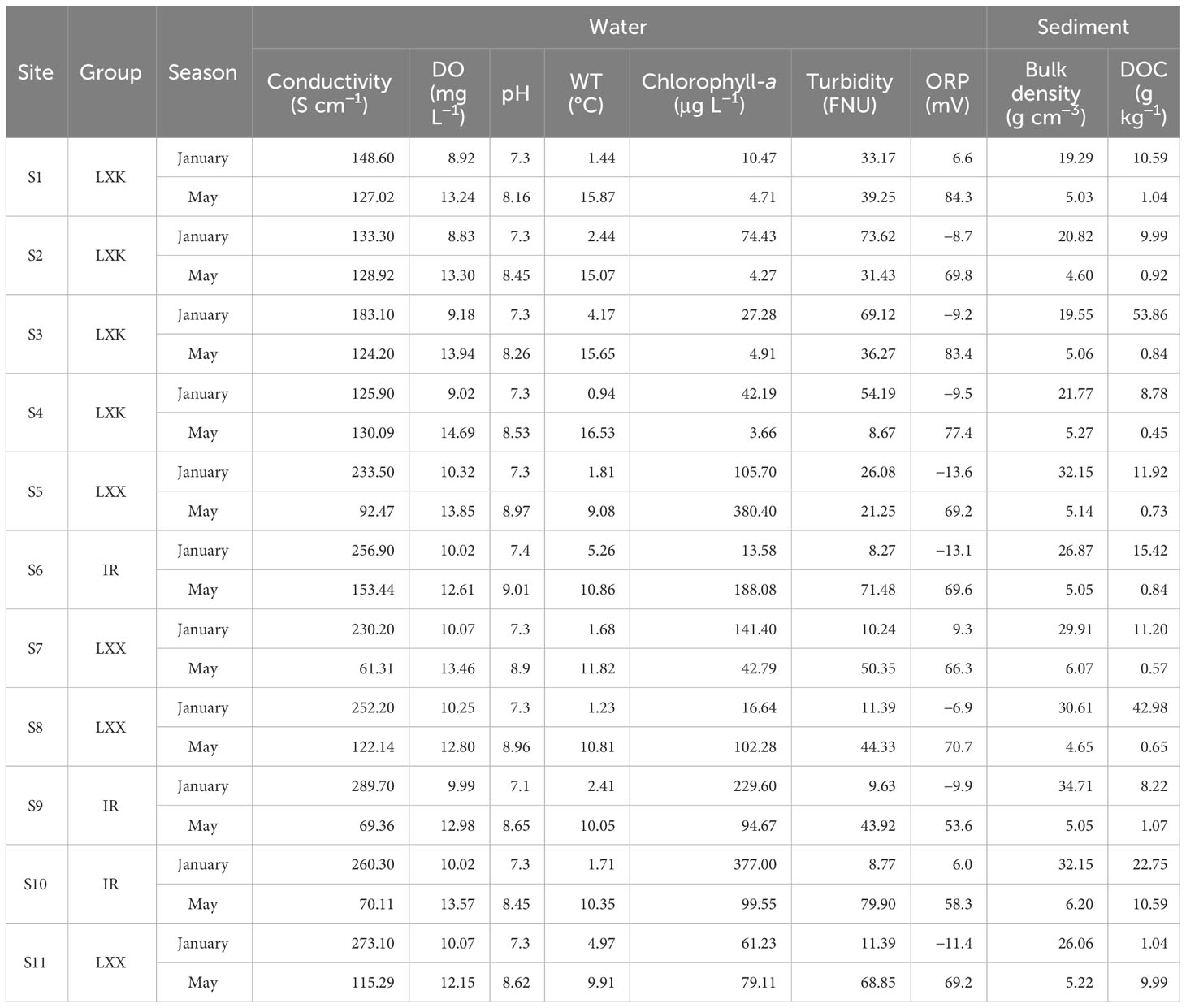
Table 1 Physicochemical parameters in Lake Xingkai (LXK), Lake Xiao Xingkai (LXX) and the inflowing rivers (IR) in winter and spring (Water and Sediment).
The concentrations of DGT-labile Mn (5.31–2432 μg L−1) and As (0.00–6.98 μg L−1) kept relatively stable in the overlying water when there was ice cover in January, but increased with depth in the sediment (Figure 2). Although the concentrations of DGT-labile Cd (0.01–0.11 μg L−1), Pb (0.00–6.98 μg L−1) and Zn (2.92–82.78 μg L−1) were relatively stable throughout the entire profile, their orders of magnitude differed. Apart from Cd, the levels of DGT-labile Cu, Mn, Pb and As were significantly highest in LXK. In terms of labile metal concentrations, no significant distinction was noted between LXX and the inflowing rivers, with the exception of Zn (P < 0.05) and Pb (P < 0.01). During the ice-free period in May, the levels of Mn (9.89–2958 μg L−1) and As (0.22–13.42 μg L−1) demonstrated similar variations to those observed in January. However, a significant difference was noted in the quantities of DGT-labile As (P < 0.05), with measurements in May showing a twofold increase (mean 2.9 ± 2.97 μg L−1) compared to those in January.
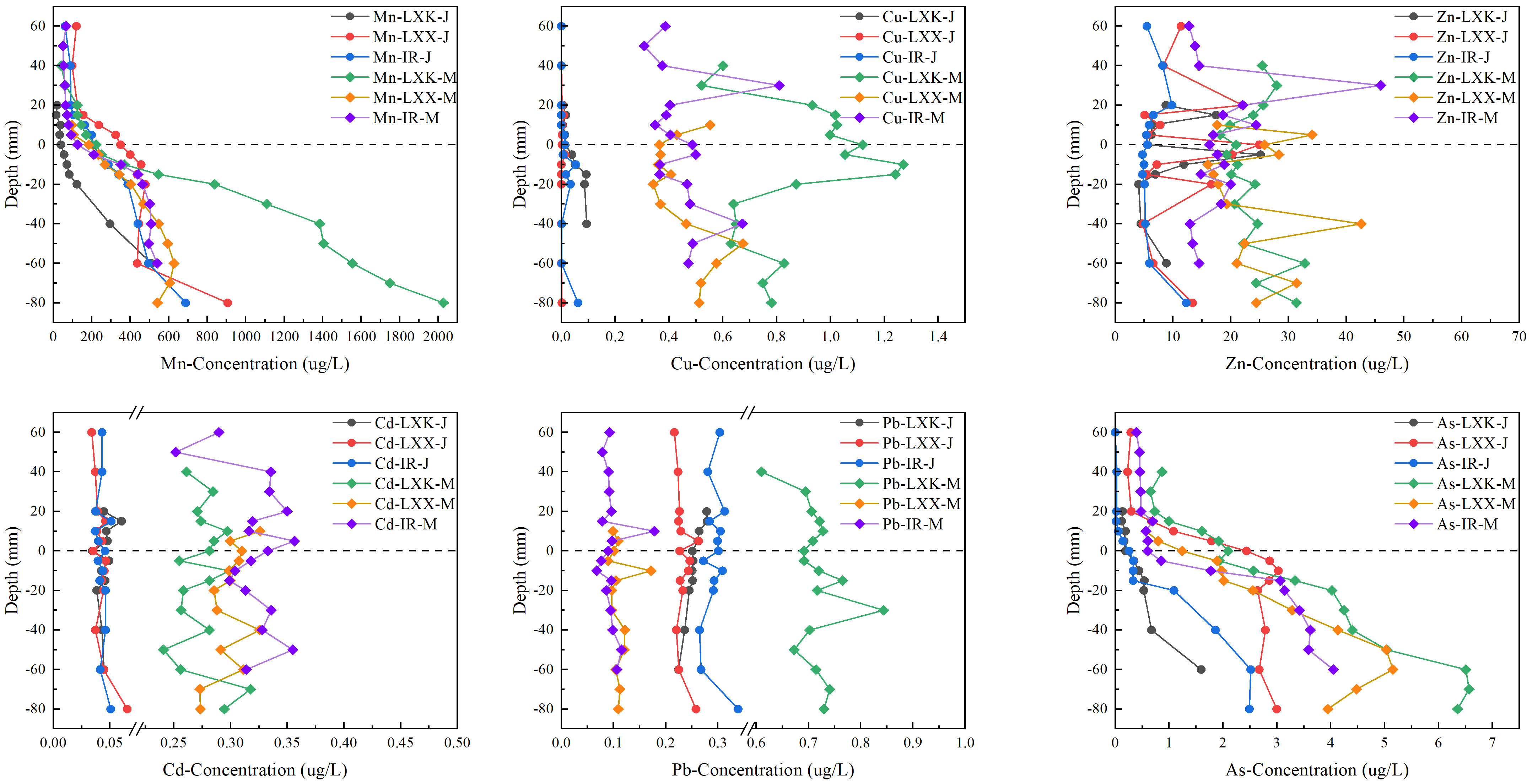
Figure 2 Distribution of labile Mn, Cu, Zn, Cd, Pb and As at the SWI in Lake Xingkai (LXK), Lake Xiao Xingkai (LXX) and inflowing rivers (IR) in January and May.
The concentrations of DGT-labile Cu (0.18–2.75 μg L−1), Zn (10.62–112.96 μg L−1), Cd (0.15–20.45 μg L−1) and Pb (0.06–0.39 μg L−1) in the overlying water increased with depth and remained stable from the SWI to the deep sediments, with higher values in May than in January (P < 0.05). Except As, the levels of DGT-labile Cd, Cu, Mn, Pb and Zn in LXK were significantly different from those in LXX and/or the inflowing rivers. Significant differences were evident in the levels of DGT-labile Mn, Cu, and Pb between LXK and the inflowing rivers (P < 0.01).
The relationship and driving factors between the physicochemical parameters of heavy metals in water and those in sediment were determined by RDA analysis. Dissolved oxygen exerted a predominant control on the migration of heavy metals, accounting for 45.6% (P = 0.01) of the effect (Figure 3). The first and second axes explained 55.18% and 0.39% of the variation of heavy metals in January. In May, the first and the second axes explained 33.17% and 0.90% of the variation in heavy metals. The water temperature explained 52.9% (P = 0.006) of variation in heavy metals and was the most important driving factor.
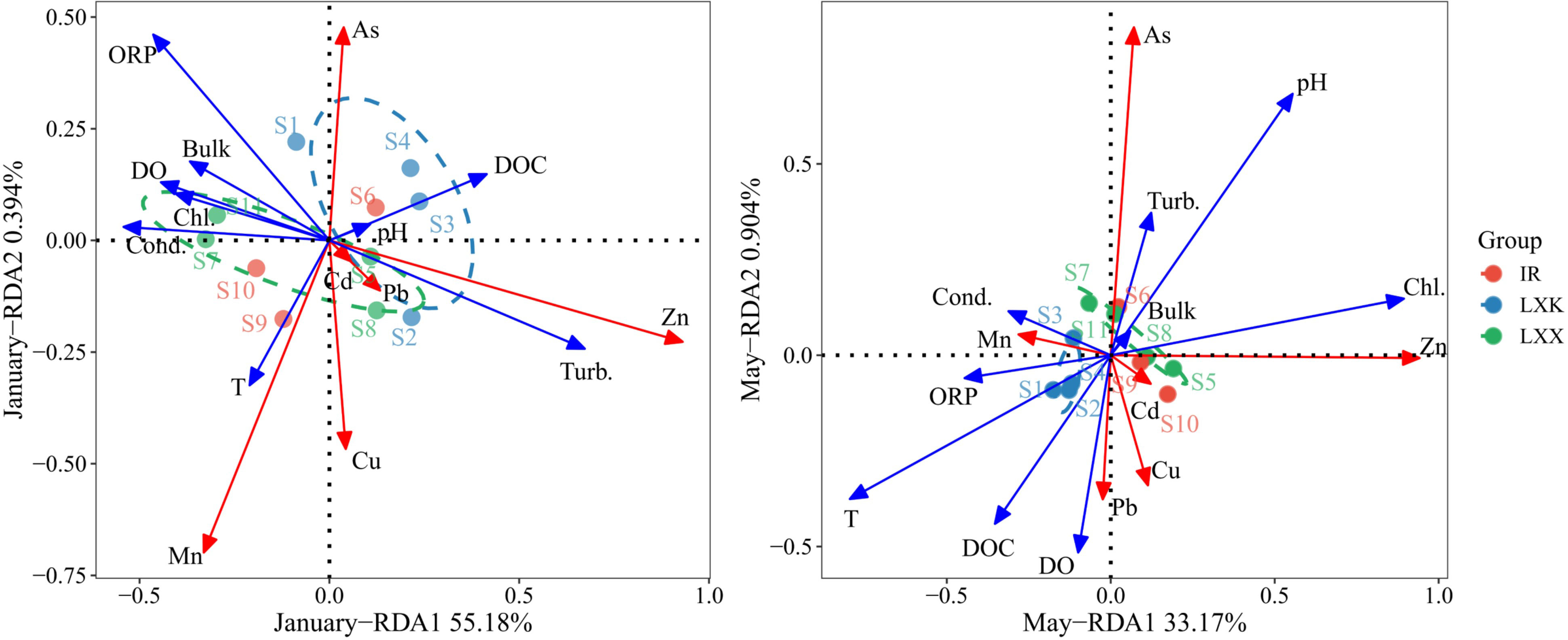
Figure 3 Redundancy analysis (RDA) diagram for heavy metals and sediment properties during ice-covered (January) and ice-free (May) periods showing AX1 and AX2 of the RDA, constrained by the selection of environmental variables obtained through forward selection. The environmental variables are depicted as blue lines with directional arrows, and the heavy metals are represented by red lines with arrows.
3.2 Diffusion flux of heavy metals across SWI
The upward flow to the overlying water and the downward flux to the sediment are shown by the positive and negative numbers, respectively. In January (with ice cover), the positive apparent diffusion fluxes of Mn (413.82 ± 518.70 mg·m−2·day−1), As (3.53 ± 4.92 mg·m−2·day−1) and Cu (0.03 ± 0.12 mg·m−2·day−1) indicated that the sediment served as a source of these metals (Figure 4). Conversely, the fluxes of Zn (−2.36 ± 117.59 mg·m−2·day−1), Cd (−0.04 ± 0.09 mg·m−2·day−1) and Pb (−0.02 ± 0.11 mg·m−2·day−1) indicated that the sediment served as a reservoir for these metals (Figure 4).
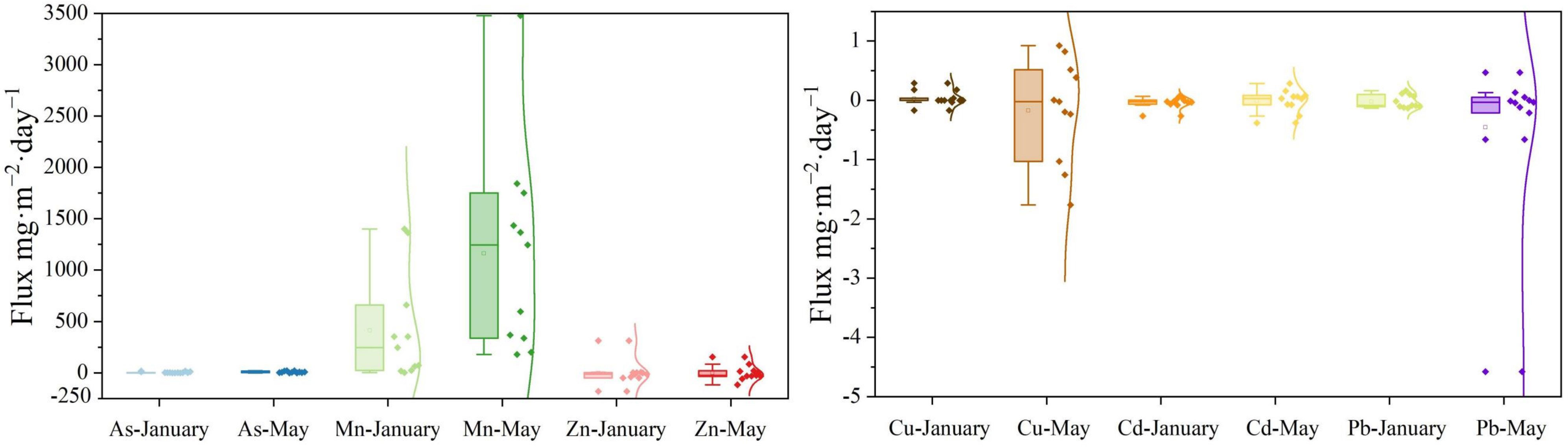
Figure 4 During ice-covered and ice-free periods, the heavy metals (As, Cd, Cu, Mn, Pb, and Zn) diffusion flux at the SWI. The diamond indicates the concentrations of heavy metals (As, Cd, Cu, Mn, Pb, and Zn); the square indicates the mean value; and the curve line is fitted by Kernel Smoother.
The source–sink characteristics of Mn (1163.25 ± 991.27 mg·m−2·day−1) and As (8.12 ± 7.59 mg·m−2·day−1) in May were consistent with those in January, but with much higher fluxes (P < 0.05). The values of the diffusive fluxes of Zn (−1.80 ± 72.84 mg·m−2·day−1), Pb (−0.46 ± 1.39 mg·m−2·day−1), Cu (−0.17 ± 0.86 mg·m−2·day−1) and Cd (−0.01 ± 0.19 mg·m−2·day−1) in May tended to 0 mg·m−2·day−1, indicating a weak sink of these metals in the sediment.
4 Discussion
4.1 Heavy metal distribution at SWI in ice-covered and ice-free periods
The distribution of heavy metals revealed a strong spatial heterogeneity in the profiles in the LXK basin (Figure 2). Throughout the ice-covered season, DGT-labile Mn and As profiles exhibited a strong similarity with concentrations peaking in the sediment and displaying decreased levels in the overlying water (Figure 2). This can be attributed to the reductive dissolution of Mn/Fe oxides under lower redox potential conditions in the deep sediments, which subsequently increases the concentrations of Mn and As below the sediment-water interface (SWI) (Wu et al., 2015; Jiang et al., 2022).
During the freezing period, average labile concentrations of Cd, Pb, and Zn in the overlying water were observed to be higher relative to ice-free conditions (Figure 2). At the same depth, concentrations of Cd and Cu in all three groups (LXK, LXX, IR) were significantly higher in May compared to January. Figure 2 revealed distinctive concentration peaks at specific depths for both Zn-IR-J and Zn-IR-M. Notably, Zn-IR-J exhibited a significant concentration peak of 9.81 μg L−1 at a depth of 20 mm, while Zn-IR-M displayed a pronounced peak of 45.97 μg L−1 at a depth of 30 mm. This may suggest a concentration gradient-driven diffusion of these heavy metals towards the ice-water interface. Based on principles from crystallography and the theory of liquid-solid phase equilibrium, the formation of ice may subsequently force these heavy metals into the water beneath the ice (Zhang et al., 2012; Tang et al., 2020; Jansen et al., 2021). Moreover, under lower redox potential conditions beneath the ice cover, the reduction of Fe/Mn oxides in the sediment would occur, leading to the release of adsorbed Cd, Pb, and Zn back into the overlying water (Huo et al., 2015; Zhang et al., 2020b). Furthermore, the notable rise in arsenic, lead, and manganese concentrations in surface waters from January to May could be linked to increased agricultural runoff, as the region’s intensified farming practices involve fertilizers and pesticides known to contain specific heavy metals like arsenic and lead (Tang et al., 2010; Deng et al., 2016; Zhang R. et al., 2020; Zhang J. et al., 2022).
The variations in Mn and Cd levels show a consistent fluctuation with changes in sediment depth. This substantiates the assertion that the mobilization of Cd is governed by the Mn redox cycle. Correlative analysis of redox potential and Cd content validates this inference, demonstrating that Cd is discharged during the reductive dissolution of manganese oxide concomitant with a reduction in redox potential. Furthermore, in contrast to January, the month of May exhibits an augmented overall concentration of Cd and Cu, attributed to exogenous inputs from agricultural practices and microbial perturbations during this period (Chen et al., 2021). The concentrations of Mn, As, and Cu at the sediment-water interface exhibit a significant positive correlation with temperature. This is attributed to the elevated temperature promoting both the reduction reactions of oxides and the spontaneous sediment adsorption-desorption process (Sun Q. et al., 2015; Wang et al., 2024). In May, a substantial negative correlation is observed between Mn and Cu concentrations and pH, aligning with prior investigations. This phenomenon is attributed to the diminishing pH, which entails a concurrent escalation in H+ competition and a reduction in surface electronegativity, thereby repelling cations. Consequently, this results in their leaching and partial release into the solution (Guthrie et al., 2005). The elevated pH in May resulted in a reduction in cations leaching; however, this did not lead to a decrease in heavy metal concentrations. Therefore, it can be concluded that pH is not the primary determinant of heavy metal concentrations. The dominant factors contributing to the rise in heavy metal concentrations are still the increase in temperature and external inputs from agricultural activities.
In January, the driving force of labile concentrations of heavy metals was the dissolved oxygen (45.6%, P=0.01), with a negative correlation (Figure 3). The ice-cover acts as a barrier isolating water from the atmosphere, thus inhibiting vertical mixing and photosynthesis. This isolation, coupled with continuous oxygen consumption by subaquatic organisms, may result in a dissolved oxygen deficit (Golosov et al., 2016). Hence, the reduction process of Fe/Mn oxides and sulfides at low redox potential conditions consequently lead to the co-release of metals into the pore and overlying waters (Wu et al., 2015; Zhang et al., 2020b).
In contrast, the distinct hydrodynamics in May caused sediment resuspension, transforming the speciation of metals from stable to labile fractions, and leading to elevated labile concentrations of Cu, Zn, Cd and As (Figure 2). Water temperature was found to account for 52.9% of the variation in labile metals throughout the profile in May. The spontaneous sediment adsorption process is governed by adsorption-desorption theory (Sun W. et al., 2015; Xie et al., 2019; Zhang et al., 2019). As both ΔG0 and ΔH0 are negative, the adsorption of heavy metals based on ΔG0 = ΔH0 – T × ΔS0 is exothermic, whereas the desorption process is endothermic (Jin et al., 1992; Adebowale et al., 2008). According to this principle, temperature elevations facilitate the migration of heavy metals from sediments into lake water, reducing their sedimentary concentrations (Zhao et al., 2014). Consequently, heavier metal loads at the SWI during summer relative to winter were observed. Interactions with organic matter could potentially limit DGT gel absorption of these metals (Davison et al., 2015). Bioturbation and physical disturbances could enhance pore spaces in sediments, promoting sediment particle resuspension, and hence liberating heavy metals from sediments into the overlying water (Atkinson et al., 2007).
In the LXK basin, the Mn concentration was observed to be notably high, 23 times greater than values recorded from a prior study of heavy metals at the SWI during ice-covered periods in the Chagan Lake (Yuan et al., 2022). This substantial Mn concentration suggests a predominance of natural lithogeochemical processes over anthropogenic sources and points to ongoing diagenetic processes in the LXK basin (Förstner et al., 1981; Yuan et al., 2014). Previous studies documented diagenetic processes whereby MnOx is rapidly converted to Mn(Ca)CO3 and deposited near the SWI, a process catalyzed by the reductive dissolution of abundant MnOx by sulfides and microbial activity at the SWI (Liu et al., 2009; Lee et al., 2011; Henkel et al., 2019). Whereas the concentrations of most other heavy metals (As, Cd, Cu, Pb, and Zn) in the LXK basin were lower than those in Chagan Lake and other aquatic systems (Table 2). Owing to its geographical remoteness, the LXK basin experiences comparatively lower heavy metal contaminant levels, particularly during harsh winter periods.
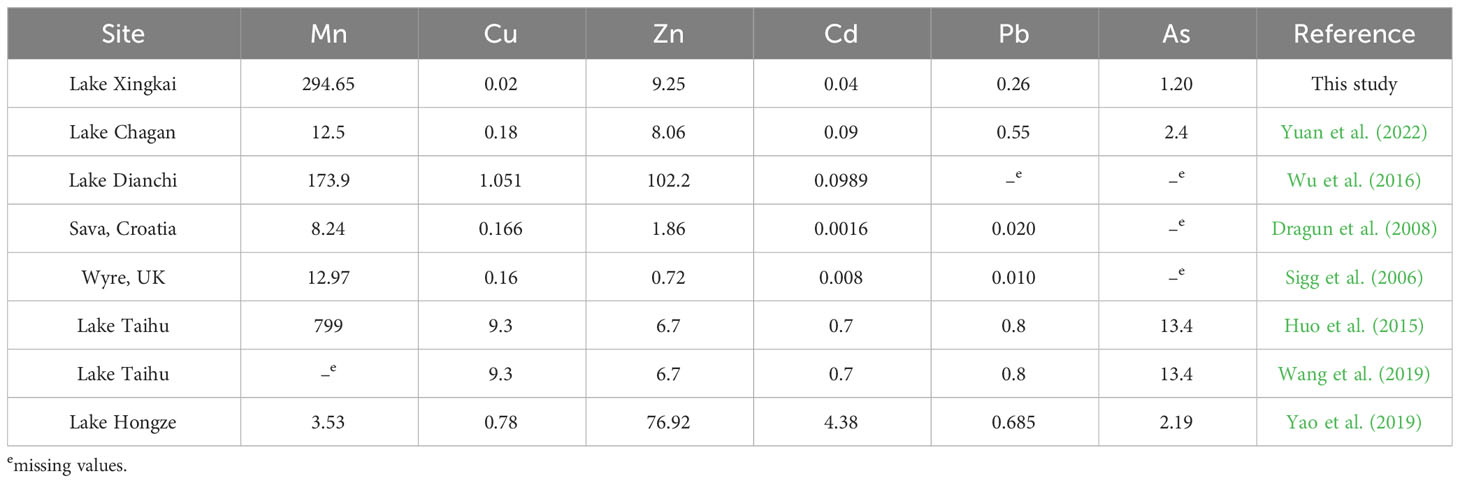
Table 2 Comparation of metal concentrations (μg L−1) with other lakes (Sigg et al., 2006; Dragun et al., 2008; Huo et al., 2015; Wu et al., 2016; Wang et al., 2019; Yao et al., 2019; Yuan et al., 2022).
4.2 Apparent fluxes across the SWI during the ice-covered and ice-free periods
By measuring the metal fluxes from pore waters to the DGT resin, it is possible to determine the levels of the labile fractions present in pore waters or estimate the flux of remobilized metals (Wu et al., 2011). The labile levels of Mn, As and Zn by DGT indicated that the LXK basin represented a potential risk of heavy metal release (Figure 4).
The elevated concentration of labile Mn can likely be attributed to the larger reservoir of Mn present in the LXK basin compared to other regions, as previous studies have reported an average soil concentration of 764 mg kg−1 in this area (Zhang et al., 2021). The Fe/Mn (hydro)oxides possess expansive specific surface areas and exhibit a pronounced attraction towards metals such as As and Zn. This propensity facilitates the establishment of robust surface complexes between Zn/As and Fe/Mn oxides (Chen et al., 2021). Under ice-covered conditions, the reduction of Fe/Mn (hydro)oxides plays a role in the potential release of Zn and As fluxes into the water above (Liu et al., 2022). As spring temperatures rise, the melting of the lake’s ice cover initiates, leading to the transition of the sediment environment from reducing to oxidizing conditions. In the presence of oxidizing conditions, Mn and As in the sulfidic phases can be transferred from the poorly soluble organic matter and sulfide-bound fractions to the more soluble exchangeable and carbonate-bound fractions (Ho et al., 2012). The activity of aerobic microorganisms experienced an increase, alongside an elevated rate of oxidative degradation of organic matter. As a result, the release of Mn and As fluxes in spring surpasses those observed in winter, driven by the degradation of organic matter and the oxidation of sulfides to sulfates (Thamdrup et al., 1994; Duan et al., 2019).
4.3 Management implications from freezing periods
Our work suggests that the existence of ice cover could cause the enrichment of labile heavy metals (e.g., Mn, Zn and As) at the SWI, but at low levels of contamination and potential ecological risk, consistent with our prior investigation on the overall metal content (Jiang et al., 2022). The mean concentration of labile Mn (294 μg L−1) exceeded the threshold of the Environmental Quality Standards for Surface Water (GB 3838-2002, 100 μg L−1), which might pose a potential risk to the benthic and aquatic biota. During ice-covered and ice-free periods, the redox-sensitive Mn/Fe phases also affected the availability of associated nutrients, such as Fe and P, in lake ecosystems. Variations in redox conditions significantly impact the release and migration dynamics of heavy metals(Huo et al., 2015; Wu et al., 2015). For instance, our results indicates that the reduction of Fe/Mn oxides within sediment actively promotes the liberation of adsorbed Cd, Pb, and Zn back into the overlying water. Different hydrodynamic conditions can either facilitate or impede the diffusion and transportation of heavy metals (Geng et al., 2022). Turbulent flows enhance metal dispersion and interaction with sediment particles (Hayter and Gu, 2000). Resuspending sediments, especially in turbulence, alters sediment properties, affecting heavy metal distribution and promoting their release into the water. Conversely, steady currents reduce sediment interaction, thereby slowing down metal transport (Sheng and Lick, 1979).The management of lakes for hydrology and eutrophication in winter should therefore consider redox and hydrodynamic processes, in addition to reducing the use of products containing heavy metals.
5 Conclusion
The present study employed an in situ, high-resolution DGT technique to investigate the dynamics of bioavailable heavy metals (e.g., Mn, Zn, As, Cd, Cu, and Pb) at the SWI during ice-covered and ice-free periods in the Lake Xingkai basin. Our findings demonstrate the accumulation of labile heavy metals at the SWI during ice-covered conditions, albeit at lower concentrations compared to other areas. Notably, labile Mn exhibited significantly higher concentrations in the LXK basin than in other lakes, displaying distinctive source characteristics in both winter and spring. Additionally, based on Fick’s first law, the sediments exhibited a role as a source of Mn and As, while weakly sequestering Zn, Cd, and Pb during both ice-covered and ice-free periods. Furthermore, we observed lower concentrations of labile metals in winter compared to spring, except for As. The composition and distribution of metals at the SWI were governed by different driving mechanisms, with dissolved oxygen being a prominent factor during ice-covered periods and water temperature influencing the system during ice-free periods. This comprehensive study provides significant insights and a solid theoretical foundation for mitigating heavy metal pollution in the LXK basin.
Data availability statement
The raw data supporting the conclusions of this article will be made available by the authors, without undue reservation.
Author contributions
XZ: Conceptualization, Funding acquisition, Methodology, Resources, Supervision, Writing – original draft, Project administration. ZW: Data curation, Investigation, Software, Visualization, Writing – original draft. XH: Investigation, Writing – original draft. ZY: Investigation, Writing – original draft. XD: Software, Visualization, Writing – review & editing. YY: Supervision, Writing – review & editing. NW: Supervision, Writing – review & editing. ZQ: Supervision, Writing – review & editing. CW: Supervision, Writing – review & editing.
Funding
The author(s) declare financial support was received for the research, authorship, and/or publication of this article. This research was financially supported by Natural Science Foundation of Jilin Province (No. YDZJ202201ZYTS480), the National Natural Science Foundation of China (No. 42101071, 42271129).
Acknowledgments
The authors extend gratitude to the Heilongjiang Xingkai Lake Wetland Ecosystem National Observation and Research Station for fieldwork support, Fuzhi Li for fieldwork assistance, and the Wetland Ecosystem Management Group for their insightful suggestions.
Conflict of interest
The authors declare that the research was conducted in the absence of any commercial or financial relationships that could be construed as a potential conflict of interest.
Publisher’s note
All claims expressed in this article are solely those of the authors and do not necessarily represent those of their affiliated organizations, or those of the publisher, the editors and the reviewers. Any product that may be evaluated in this article, or claim that may be made by its manufacturer, is not guaranteed or endorsed by the publisher.
References
Adebowale K. O., Agunbiade F. O., Olu-Owolabi B. I. (2008). Fuzzy comprehensive assessment of metal contamination of water and sediments in Ondo Estuary, Nigeria. Chem. Ecol. 24, 269–283. doi: 10.1080/02757540802255600
Atkinson C. A., Jolley D. F., Simpson S. L. (2007). Effect of overlying water pH, dissolved oxygen, salinity and sediment disturbances on metal release and sequestration from metal contaminated marine sediments. Chemosphere 69, 1428–1437. doi: 10.1016/j.chemosphere.2007.04.068
Bartosiewicz M., Ptak M., Woolway R. I., Sojka M. (2021). On thinning ice: Effects of atmospheric warming, changes in wind speed and rainfall on ice conditions in temperate lakes (Northern Poland). J. Hydrol. 597, 125724. doi: 10.1016/j.jhydrol.2020.125724
Beyene M. T., Jain S. (2020). Climate-related thresholds in lake ice and the associated environmental and social systems. Water Secur. 10, 100065. doi: 10.1016/j.wasec.2020.100065
Canfield D. E. (1993). "Organic matter oxidation in marine sediments," in Interactions of C, N, P, and S Biogeochemical Cycles and Global Change NATO ASI Series, Eds. Wollast R., Mackenzie F. T., Chou L. (Berlin, Heidelberg: Springer), 333–363. doi: 10.1007/978-3-642-76064-8_14
Chen M., Ding S., Li C., Tang Y., Fan X., Xu H., et al. (2021). High cadmium pollution from sediments in a eutrophic lake caused by dissolved organic matter complexation and reduction of manganese oxide. Water Res. 190, 116711. doi: 10.1016/j.watres.2020.116711
Davison W., Lin C., Gao Y., Zhang H. (2015). Effect of gel interactions with dissolved organic matter on DGT measurements of trace metals. Aquat. Geochem. 21, 281–293. doi: 10.1007/s10498-014-9244-9
Davison W., Zhang H. (1994). In situ speciation measurements of trace components in natural waters using thin-film gels. Nature 367, 546–548. doi: 10.1038/367546a0
Deng J., Wang Y., Liu X., Hu W., Zhu J., Zhu L. (2016). Spatial distribution and risk assessment of heavy metals and As pollution in the sediments of a shallow lake. Environ. Monit. Assess. 188, 296. doi: 10.1007/s10661-016-5301-8
Ding S., Han C., Wang Y., Yao L., Wang Y., Xu D., et al. (2015). In situ, high-resolution imaging of labile phosphorus in sediments of a large eutrophic lake. Water Res. 74, 100–109. doi: 10.1016/j.watres.2015.02.008
Ding S., Wang Y., Wang D., Li Y. Y., Gong M., Zhang C. (2016). In situ, high-resolution evidence for iron-coupled mobilization of phosphorus in sediments. Sci. Rep. 6, 24341. doi: 10.1038/srep24341
Dragun Z., Raspor B., Roje V. (2008). The labile metal concentrations in Sava River water assessed by diffusive gradients in thin films. Chem. Speciation Bioavailability 20, 33–46. doi: 10.3184/095422908X299164
Duan L., Song J., Liang X., Yin M., Yuan H., Li X., et al. (2019). Dynamics and diagenesis of trace metals in sediments of the Changjiang Estuary. Sci. Total Environ. 675, 247–259. doi: 10.1016/j.scitotenv.2019.04.190
Eismann C. E., Menegário A. A., Gemeiner H., Williams P. N. (2020). Predicting trace metal exposure in aquatic ecosystems: evaluating DGT as a biomonitoring tool. Expo. Health 12, 19–31. doi: 10.1007/s12403-018-0280-3
Fan X., Ding S., Chen M., Gao S., Fu Z., Gong M., et al. (2019). Peak chromium pollution in summer and winter caused by high mobility of chromium in sediment of a eutrophic lake: in situ evidence from high spatiotemporal sampling. Environ. Sci. Technol. 53, 4755–4764. doi: 10.1021/acs.est.8b07060
Förstner U., Wittmann G., Prosi F., Lierde J. (1981). Metal pollution in the aquatic environment 110–196. doi: 10.1007/978-3-642-96511-1_8. XF2006212406.
Fu J., Zhao C., Luo Y., Liu C., Kyzas G. Z., Luo Y., et al. (2014). Heavy metals in surface sediments of the Jialu River, China: Their relations to environmental factors. J. Hazard. Mater. 270, 102–109. doi: 10.1016/j.jhazmat.2014.01.044
Gao L., Gao B., Peng W., Xu D., Yin S. (2018). Assessing potential release tendency of As, Mo and W in the tributary sediments of the Three Gorges Reservoir, China. Ecotox. Environ. Safe. 147, 342–348. doi: 10.1016/j.ecoenv.2017.08.036
Geng N., Bai Y., Pan S. (2022). Research on heavy metal release with suspended sediment in Taihu Lake under hydrodynamic condition. Environ. Sci. pollut. Res. 29, 28588–28597. doi: 10.1007/s11356-021-17666-1
Gillmore M. (2021). Assessing the risk of sediment-associated nickel exposure to benthic marine biota in Southeast Asia and Melanesia. Philosophy Thesis School of Earth, Atmospheric and Life Sciences, University of Wollongong. Available at: https://ro.uow.edu.au/theses1/1429.
Golosov S., Terzhevik A., Zverev I., Kirillin G., Engelhardt C. (2016). Climate change impact on thermal and oxygen regime of shallow lakes. Tellus A: Dyn. Meteorol. Oceanogr. 68, 17264. doi: 10.3402/tellusa.v64i0.17264
Guan D.-X., He S.-X., Li G., Teng H. H., Ma L. Q. (2021). Application of diffusive gradients in thin-films technique for speciation, bioavailability, modeling and mapping of nutrients and contaminants in soils. Crit. Rev. Environ. Sci. Technol. 52, 3035–3079. doi: 10.1080/10643389.2021.1900765
Guthrie J. W., Hassan N. M., Salam M. S. A., Fasfous I. I., Murimboh C. A., Murimboh J., et al. (2005). Complexation of Ni, Cu, Zn, and Cd by DOC in some metal-impacted freshwater lakes: a comparison of approaches using electrochemical determination of free-metal-ion and labile complexes and a computer speciation model, WHAM V and VI. Anal. Chim. Acta 528, 205–218. doi: 10.1016/j.aca.2004.10.003
Hayter E. J., Gu R. (2000). “Prediction of contaminated sediment transport in the Maurice River-Union Lake, New Jersey, USA,” in Proceedings in Marine Science Coastal and Estuarine Fine Sediment Processes. Eds. McAnally W. H., Mehta A. J. (Amsterdam: Elsevier), 439–458. doi: 10.1016/S1568-2692(00)80136-9
Henkel J. V., Dellwig O., Pollehne F., Herlemann D. P. R., Leipe T., Schulz-Vogt H. N. (2019). A bacterial isolate from the Black Sea oxidizes sulfide with manganese(IV) oxide. Proc. Natl. Acad. Sci. U.S.A. 116, 12153–12155. doi: 10.1073/pnas.1906000116
Ho H. H., Swennen R., Cappuyns V., Vassilieva E., Van Gerven T., Tran T. V. (2012). Potential release of selected trace elements (As, Cd, Cu, Mn, Pb and Zn) from sediments in Cam River-mouth (Vietnam) under influence of pH and oxidation. Sci. Total Environ. 435–436, 487–498. doi: 10.1016/j.scitotenv.2012.07.048
Huo S., Zhang J., Yeager K. M., Xi B., Qin Y., He Z., et al. (2015). Mobility and sulfidization of heavy metals in sediments of a shallow eutrophic lake, Lake Taihu, China. J. Environ. Sci. 31, 1–11. doi: 10.1016/j.jes.2014.12.003
Jansen J., Macintyre S., Barrett D. C., Chin Y. P., Cortés A., Forrest A. L., et al (2021). Winter limnology: how do hydrodynamics and biogeochemistry shape ecosystems under ice? J. Geophys. Res.: Biogeosci. 26, e2020JG006237 doi: 10.1029/2020JG006237
Jiang M., Wang Q., Tian X., Zhu X., Dong X., Wu Z., et al. (2022). Spatiotemporal variation and ecological risk assessment of sediment heavy metals in two hydrologically connected lakes. Front. Ecol. Evol. 10. doi: 10.3389/fevo.2022.1005194
Jin X., Xu N., Zhang Y. (1992). Sediment pollution chemistry (Beijing: China Environmental Science Press).
Klavinš M., Briede A., Rodinov V., Kokorite I., Parele E., Klavina I. (2000). Heavy metals in rivers of Latvia. Sci. Total Environ 262, 175–183. doi: 10.1016/S0048-9697(00)00597-0
Lee J.-H., Kennedy D. W., Dohnalkova A., Moore D. A., Nachimuthu P., Reed S. B., et al. (2011). Manganese sulfide formation via concomitant microbial manganese oxide and thiosulfate reduction: Manganese sulfide precipitated by Shewanella oneidensis MR-1. Environ. Microbiol. 13, 3275–3288. doi: 10.1111/j.1462-2920.2011.02587.x
Leppäranta M., Tikkanen M., Virkanen J. (2003). Observations of ice impurities in some Finnish lakes/Soome jarvede jaas esinevate lisandite vaatlus. Proc. Estonian Acad. Sci. Chem. 52, 59–75. doi: 10.3176/chem.2003.2.02
Li C., Ding S., Yang L., Wang Y., Ren M., Chen M., et al. (2019). Diffusive gradients in thin films: devices, materials and applications. Environ. Chem. Lett. 17, 801–831. doi: 10.1007/s10311-018-00839-9
Li J., Zuo Q., Feng F., Jia H. (2022). Occurrence and ecological risk assessment of heavy metals from Wuliangsuhai Lake, Yellow River Basin, China. Water 14, 1264. doi: 10.3390/w14081264
Liu Q., Jia Z., Liu G., Li S., Hu J. (2023). Assessment of heavy metals remobilization and release risks at the sediment-water interface in estuarine environment. Mar. pollut. Bull. 187, 114517. doi: 10.1016/j.marpolbul.2022.114517
Liu J., Liu L., Qu X. (2009). Discussion on origin of clay minerals in outcropped sandstone from Lower Cretaceous Chengzihe Formation and Muling Formation in Jixi Basin. Global Geol. 12, 1–4.
Liu W., Lu G., Wang W.-X. (2022). In situ high-resolution two-dimensional profiles of redox sensitive metal mobility in sediment-water interface and porewater from estuarine sediments. Sci. Total Environ. 820, 153034. doi: 10.1016/j.scitotenv.2022.153034
Pan F., Liu H., Guo Z., Cai Y., Gao A. (2019). Metal/metalloid and phosphorus characteristics in porewater associated with manganese geochemistry: A case study in the Jiulong River Estuary, China. Environ. Pollut 255, 113134. doi: 10.1016/j.envpol.2019.113134
Ptak M., Sojka M. (2021). The disappearance of ice cover on temperate lakes (Central Europe) as a result of climate warming. Geogr. J. 187, 200–213. doi: 10.1111/geoj.12385
Sheng Y. P., Lick W. (1979). The transport and resuspension of sediments in a shallow lake. J. Geophys. Res.: Oceans 84, 1809–1826. doi: 10.1029/JC084iC04p01809
Sigg L., Black F., Buffle J., Cao J., Cleven R., Davison W., et al. (2006). Comparison of analytical techniques for dynamic trace metal speciation in natural freshwaters. Environ. Sci. Technol. 40, 1934–1941. doi: 10.1021/es051245k
Song S., Li C., Shi X., Zhao S., Tian W., Li Z., et al. (2019). Under-ice metabolism in a shallow lake in a cold and arid climate. Freshw. Biol. 64, 1710–1720. doi: 10.1111/fwb.13363
Sun W., Jiang B., Wang F., Xu N. (2015). Effect of carbon nanotubes on Cd(II) adsorption by sediments. Chem. Eng. J. 264, 645–653. doi: 10.1016/j.cej.2014.11.137
Sun Q., Zhang L., Ding S., Li C., Yang J., Chen J., et al. (2015). Evaluation of the diffusive gradients in thin films technique using a mixed binding gel for measuring iron, phosphorus and arsenic in the environment. Environ. Sci.: Processes Impacts 17, 570–577. doi: 10.1039/C4EM00629A
Sun W., Zhang E., Liu E., Chang J., Shen J. (2018). Linkage between Lake Xingkai sediment geochemistry and Asian summer monsoon since the last interglacial period. Palaeogeogr. Palaeoclimatol. Palaeoecol. 512, 71–79. doi: 10.1016/j.palaeo.2018.06.026
Tang W., Shan B., Zhang H., Mao Z. (2010). Heavy metal sources and associated risk in response to agricultural intensification in the estuarine sediments of Chaohu Lake Valley, East China. J. Hazard. Mater. 176, 945–951. doi: 10.1016/j.jhazmat.2009.11.131
Tang Y., Zhang Y., Zhao W., Liu T., Liu Y. (2020). The migration law of iron during the process of water icing. Water 12, 441. doi: 10.3390/w12020441
Thamdrup B., Fossing H., Jørgensen B. B. (1994). Manganese, iron and sulfur cycling in a coastal marine sediment, Aarhus bay, Denmark. Geochim. Cosmochim. Acta 58, 5115–5129. doi: 10.1016/0016-7037(94)90298-4
Wang S., Ding S., Zhao H., Chen M., Yang D., Li C. (2024). Seasonal variations in spatial distribution, mobilization kinetic and toxicity risk of arsenic in sediments of Lake Taihu, China. J. Hazard. Mater. 463, 132852. doi: 10.1016/j.jhazmat.2023.132852
Wang W., Wang W.-X. (2017). Trace metal behavior in sediments of Jiulong River Estuary and implication for benthic exchange fluxes. Environ. pollut. 225, 598–609. doi: 10.1016/j.envpol.2017.03.028
Wang W., Wang S., Chen J., Jiang X., Zheng B. (2019). Combined use of diffusive gradients in thin film, high-resolution dialysis technique and traditional methods to assess pollution and bioavailability of sediment metals of lake wetlands in Taihu Lake Basin. Sci. Total Environ. 671, 28–40. doi: 10.1016/j.scitotenv.2019.03.053
Wu Z., He M., Lin C. (2011). In situ measurements of concentrations of Cd, Co, Fe and Mn in estuarine porewater using DGT. Environ. pollut. 159, 1123–1128. doi: 10.1016/j.envpol.2011.02.015
Wu Z., Jiao L., Wang S. (2016). The measurement of phosphorus, sulfide and metals in sediment of Dianchi Lake by DGT (diffusive gradients in thin films) probes. Environ. Earth Sci. 75, 193. doi: 10.1007/s12665-015-4978-2
Wu H., Lu X., Wu D., Song L., Yan X., Liu J. (2013). Ant mounds alter spatial and temporal patterns of CO2, CH4 and N2O emissions from a marsh soil. Soil Biol. Biochem. 57, 884–891. doi: 10.1016/j.soilbio.2012.10.034
Wu Z., Wang S., He M., Wu F. (2015). The measurement of metals by diffusive gradients in thin films (DGT) at sediment/water interface (SWI) of bay and remobilization assessment. Environ. Earth Sci. 73, 6283–6295. doi: 10.1007/s12665-014-3851-z
Wu Z., Wang S., Ji N. (2019). Phosphorus (P) release risk in lake sediment evaluated by DIFS model and sediment properties: A new sediment P release risk index (SPRRI). Environ. pollut. 255, 113279. doi: 10.1016/j.envpol.2019.113279
Xie F., Dai Z., Zhu Y., Li G., Li H., He Z., et al. (2019). Adsorption of phosphate by sediments in a eutrophic lake: Isotherms, kinetics, thermodynamics and the influence of dissolved organic matter. Colloids Surf. A: Physicochem. Eng. Aspects 562, 16–25. doi: 10.1016/j.colsurfa.2018.11.009
Xu D., Ding S., Sun Q., Zhong J., Wu W., Jia F. (2012). Evaluation of in situ capping with clean soils to control phosphate release from sediments. Sci. Total Environ 438, 334–341. doi: 10.1016/j.scitotenv.2012.08.053
Yang F., Cen R., Feng W., Zhu Q., Leppäranta M., Yang Y., et al. (2021). Dynamic simulation of nutrient distribution in lakes during ice cover growth and ablation. Chemosphere 281, 130781. doi: 10.1016/j.chemosphere.2021.130781
Yao Y., Wang P., Wang C. (2019). The influence on contaminant bioavailability and microbial abundance of lake Hongze by the south-to-north water diversion project. Int. J. Environ. Res. Public Health 16, 3068. doi: 10.3390/ijerph16173068
Yu S., Li X., Wen B., Chen G., Hartleyc A., Jiang M., et al. (2021). Characterization of water quality in Xiao Xingkai Lake: implications for trophic status and management. Chin. Geogr. Sci. 31, 558–570. doi: 10.1007/s11769-021-1199-3
Yuan Y., Jiang M., Liu X., Yu H., Otte M. L., Ma C., et al. (2018). Environmental variables influencing phytoplankton communities in hydrologically connected aquatic habitats in the Lake Xingkai basin. Ecol. Indic. 91, 1–12. doi: 10.1016/j.ecolind.2018.03.085
Yuan Z., Taoran S., Yan Z., Tao Y. (2014). Spatial distribution and risk assessment of heavy metals in sediments from a hypertrophic plateau lake Dianchi, China. Environ. Monit. Assess. 186, 1219–1234. doi: 10.1007/s10661-013-3451-5
Yuan Y., Wang Q., Dong X., Zhu Y., Wu Z., Yang Q., et al. (2022). In situ, high-resolution evidence of metals at the sediment-water interface under ice cover in a seasonal freezing lake. Front. Ecol. Evol. 10. doi: 10.3389/fevo.2022.956903
Zhang C., Ding S., Xu D., Tang Y., Wong M. H. (2014). Bioavailability assessment of phosphorus and metals in soils and sediments: a review of diffusive gradients in thin films (DGT). Environ. Monit. Assess. 186, 7367–7378. doi: 10.1007/s10661-014-3933-0
Zhang Y., Li C., Shi X., Li C. (2012). The migration of total dissolved solids during natural freezing process in Ulansuhai Lake: The migration of total dissolved solids during natural freezing process in Ulansuhai Lake. J. Arid Land 4, 85–94. doi: 10.3724/SP.J.1227.2012.00085
Zhang T., Li L., Xu F., Chen X., Du L., Wang X., et al. (2020a). Assessing the remobilization and fraction of cadmium and lead in sediment of the Jialing River by sequential extraction and diffusive gradients in films (DGT) technique. Chemosphere 257, 127181. doi: 10.1016/j.chemosphere.2020.127181
Zhang T., Li L., Xu F., Chen X., Du L., Wang X., et al. (2020b). Evaluation of the environment risk, fractions and mobilization of nickel (Ni) in sediments of the Jialing River by sediment quality guidelines, sequential extraction and Chelex-AgI gel DGT probe. Appl. Geochem. 118, 104634. doi: 10.1016/j.apgeochem.2020.104634
Zhang M., Li C., Yang L., Ding S., Ma X., Zhang Y., et al. (2020). Application of DGT/DIFS combined with BCR to assess the mobility and release risk of heavy metals in the sediments of Nansi Lake, China. Environ. Geochem. Health 42, 3765–3778. doi: 10.1007/s10653-020-00638-8
Zhang H., Liang P., Liu Y., Wang X., Bai Y., Xing Y., et al. (2022). Spatial distributions and intrinsic influence analysis of Cr, Ni, Cu, Zn, As, Cd and Pb in sediments from the Wuliangsuhai Wetland, China. Int. J. Environ. Res. Public Health 19, 10843. doi: 10.3390/ijerph191710843
Zhang J., Liu Y., Wen M., Zheng C., Chai S., Huang L., et al. (2022). Distribution characteristics and ecological risk assessment of nitrogen, phosphorus, and some heavy metals in the sediments of Yueliang Lake in Western Jilin Province, Northeast China. Water 14, 3306. doi: 10.3390/w14203306
Zhang Y., Yang Z., Dai H., Liu G., Xu J., Xiao H. (2021). Geochemical evaluation of land quality in muling river-xingkai lake plain. Resour. Geol. 30(1), 62–70.
Zhang D., Yang S., Wang Y., Yang C., Chen Y., Wang R., et al. (2019). Adsorption characteristics of oxytetracycline by different fractions of organic matter in sedimentary soil. Environ. Sci. pollut. Res. 26, 5668–5679. doi: 10.1007/s11356-018-4028-1
Zhang R., Zhang Y., Liu L., Wang Y., Song Z., Wang X., et al. (2020). Occurrence and risk assessment of heavy metals in an urban river supplied by reclaimed wastewater. Water Environ. Res. 92, 1888–1898. doi: 10.1002/wer.1341
Zhang H., Zhao F.-J., Sun B., Davison W., Mcgrath S. P. (2001). A new method to measure effective soil solution concentration predicts copper availability to plants. Environ. Sci. Technol. 35, 2602–2607. doi: 10.1021/es000268q
Zhang J., Zhou F., Chen C., Sun X., Shi Y., Zhao H., et al. (2018). Spatial distribution and correlation characteristics of heavy metals in the seawater, suspended particulate matter and sediments in Zhanjiang Bay, China. PloS One 13, e0201414. doi: 10.1371/journal.pone.0201414
Zhao S., Shi X., Li C., Zhang H., Wu Y. (2014). Seasonal variation of heavy metals in sediment of Lake Ulansuhai, China. Chem. Ecol. 30, 1–14. doi: 10.1080/02757540.2013.841894
Keywords: heavy metals, sediment-water interface, ice-covered lakes, diffusive fluxes, diffusive gradients in thin films
Citation: Zhu X, Wu Z, Han X, Yang Z, Dong X, Yuan Y, Wang N, Qu Z and Wang C (2024) In situ, high-resolution evidence for the release of heavy metals from lake sediments during ice-covered and free periods. Front. Ecol. Evol. 11:1326818. doi: 10.3389/fevo.2023.1326818
Received: 24 October 2023; Accepted: 18 December 2023;
Published: 08 January 2024.
Edited by:
Arnaldo Marín, University of Murcia, SpainReviewed by:
Jianwei Lin, Shanghai Ocean University, ChinaMingke Luo, Chinese Research Academy of Environmental Sciences, China
Copyright © 2024 Zhu, Wu, Han, Yang, Dong, Yuan, Wang, Qu and Wang. This is an open-access article distributed under the terms of the Creative Commons Attribution License (CC BY). The use, distribution or reproduction in other forums is permitted, provided the original author(s) and the copyright owner(s) are credited and that the original publication in this journal is cited, in accordance with accepted academic practice. No use, distribution or reproduction is permitted which does not comply with these terms.
*Correspondence: Xiaoyan Zhu, emh1eHkwNjEzQDEyNi5jb20=
†These authors have contributed equally to this work and share first authorship