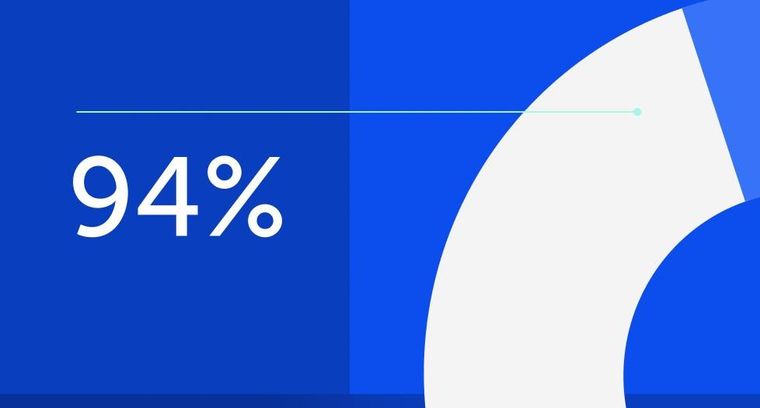
94% of researchers rate our articles as excellent or good
Learn more about the work of our research integrity team to safeguard the quality of each article we publish.
Find out more
REVIEW article
Front. Ecol. Evol., 12 December 2023
Sec. Population, Community, and Ecosystem Dynamics
Volume 11 - 2023 | https://doi.org/10.3389/fevo.2023.1279589
This article is part of the Research TopicEffects of Microplastics on Ecosystem Functioning of Eukaryotic Marine MicrobesView all 5 articles
Microplastics (MPs) are plastic particles <5 mm in size, that end up ultimately in marine and freshwater ecosystems, adversely affecting various ecological functions. With the multifaceted roles of fungi and their diverse modes of interaction such as saprobic, epibiotic, endobiotic, parasitic, and symbiotic or being a nutritionally enriched food source in aquatic ecosystems, the effect of MPs on fungi–plankton interactions is still less explored. Properties of MPs such as (i) size range similar to those of microeukaryotes, (ii) substrate for unique microbiota, (iii) ability to be transported from the source to faraway waterbodies, and (iv) sorption of pollutants, have adverse effects on various guilds of ecological organization. MPs also tend to alter nutrient cycling and inhibit efficient energy transfer through microbial and mycoloop in an ecosystem. This paper comprehensively reviews the effect of MPs at the population and community level on the complex ecological (fungi–phytoplankton–zooplankton) interactions with an emphasis on the role of fungi in the aquatic ecosystem. Examination of existing literature revealed that MPs can interfere in ecosystem functioning by acting in synergy with fungi, while antagonistically affecting the community and vice-versa. Herein, we explore current understanding of the effect of MPs on major components of microbial and mycoloop in the marine food web, elaborating MPs-mediated changes in the ecosystem functioning, identifying research gaps, and highlighting the need for future research.
● Microplastics (MPs) intervene in ecosystem functioning of aquatic microeukaryotes.
● MPs effects are seen at the community level via trophic transfer affecting microbial and mycoloop.
● MPs negatively affect the population of phytoplankton and zooplankton.
● MPs favor biofilm-forming bacteria, fungi, harmful algae, and invasive species by providing a stable artificial substrate.
● Plastic additives influence the outcomes of bacteria–fungi–plankton interactions.
● Integration of various trophic guilds and plastisphere communities remains to be prospected for biodegradation of MPs.
Microplastics (MPs) are synthetic organic polymers of particle size <5 mm that can be further degraded to particles <100 nm in size. They present a major challenge to the aquatic environment due to long-distance inter- and intra-environmental transport and being similar to the size of plankton. A substantial portion of the yearly global production of over 300 million tonnes (Mt) of plastics end up in inland water bodies and oceans, mainly through river transport (Lebreton et al., 2018; IUCN, 2021). Approximately 367 Mt plastic products had been produced till 2020, which has been increasing annually (Plastics Europe, 2021). Based on polymer type, plastics are mainly categorized as polystyrene, poly-ethylene, poly-propylene, poly-ethylene terephthalate, and poly-vinyl chloride. These plastics turn into MPs through various processes such as biological degradation, photochemical fragmentation, and mechanical wear and tear into various shapes such as fragments, films, fibers, foams and beads. Thus, MPs originate from two distinct pathways: primary and secondary sources. Primary sources of MPs are directly produced at micrometric size, such as plastic pellets, exfoliating cosmetics, and clothing fibers, whereas, secondary MPs originate from the breakdown of larger pieces (Li et al., 2018). Certain chemical additives such as flame retardants, stabilizers, softeners, plasticizers, and dyes are also used during the production of plastics to enhance its properties. These additives leach out of weathered plastic debris and are also known to have endocrine-disrupting activities and other hazardous effects in marine ecosystems. Additionally, their hydrophobicity, and high surface area-to-volume ratio, may facilitate MPs to continuously adsorb many hazardous substances, including organic pollutants and toxic metals (Rodrigues et al., 2019), and can adversely affect the aquatic biota (Ryan, 2016). In marine organisms that ingest MPs, chemicals adsorbed on the surface of MPs can accumulate in biological tissues and have harmful effects on marine organisms at different trophic levels. MPs have become a symptom of ongoing unsustainable production and consumption patterns. MPs are being widely reported from diverse aquatic ecosystems including deep sea (Van Cauwenberghe et al., 2013), Antarctic fjords (Garza et al., 2023), Himalayan glaciers (Zhang et al., 2021), the large ocean gyres in the Pacific Ocean (Do Sul et al., 2013; Eriksen et al., 2013; Law and Thompson, 2014), the Atlantic Ocean (Cózar et al., 2017), the Indian Ocean (Jeyasanta et al., 2023), and from coasts (Tiwari et al., 2019) to open seas (Pan et al., 2022). In 2020, a rough estimate of the amount of plastics in the ocean was 150×106 metric tons. So, along with other contaminants plastics are threatening global oceanic biodiversity and disrupting ecosystem functioning. It is relevant to mention that the majority of laboratory bioassay tests have not recorded any acute or inherently toxic effects of MPs, except a few studies (Jemec et al., 2016; Gray and Weinstein, 2017). On the other hand, significant chronic-sub-lethal effects on lower trophic organisms have been reported from long term, realistic environmental timescales, which points to effects on entire ecosystems through trophic cascades (Galloway et al., 2017; Botterell et al., 2019). Considering the steady rise in the production of plastics and the stability of MPs, the amount of MPs in the ocean is likely to increase while their average size will decrease (Jahnke et al., 2017) (Table 1). MPs, being similar to the size range of plankton and meiobenthic species that form the base of the food chain, pose an ecological threat to the marine environment by means of (i) ingestion of MPs by marine organisms like copepods, rotifers, mollusks, and fishes, (ii) trophic transfer, (iii) spread of diseases (Mei et al., 2020), and (iv) accumulation in biomass of marine organisms. Eventually, because of their pervasive nature and ever-increasing concentration, MPs are more likely to affect whole ecosystem functioning by impacting interactions, ingestions, providing substrate for colonization and biofilm formations by bacteria and fungi, incurring hazardous effects across food webs and entanglement causing physical hindrances to usual ecological activities of fungi, phytoplankton and zooplankton (Ziajahromi et al., 2017). Understanding how microplastic (MP) pollution affects fungal diversity and their interactions is paramount to predicting their ecological consequences. So far current understanding of ecological effects of MP pollution comes from laboratory studies applying single species bioassay tests, however, the environmental effects at ecosystem level cannot be simply explained by species-specific response of marine organisms against MPs. As, the alteration in the normal ecosystem functioning is linked to changes in the functional composition of the community, which in turn related to the changes in the structure of population (Rudolf and Rasmussen, 2013). The impacts depend upon how MPs alter the direct and indirect interaction of fungi with marine organisms. Herein, we review the current knowledge of the effects of MPs on major components of the microbial loop of the marine food web and MPs-mediated changes in the functioning of mycoloop (a trophic pathway, transferring carbon and nutrients to higher trophic levels through fungal zoospores) (Kagami et al., 2014). Based on the limited number of published papers, this review identifies research gaps and highlights the need for more detailed studies to determine the MPs-driven alterations in the outcome of interactions among fungi and other marine organisms. We also offer insights into how MPs affect interactions between fungi and marine zooplankton, while analyzing the possible long-standing ecological threats posed by MPs to marine ecosystems. This paper reviews available literature on the impacts of MPs on various ecosystem functions mediated by aquatic fungi and their role in the mitigation of plastic contamination in aquatic ecosystems. We have critically surveyed available literature on the role of fungi in shaping marine ecosystems through their diverse interactions with marine copepods and how the presence of MPs alters these ecological processes.
For the past five years, several studies have explored the impact of MPs on the health of individual organisms and the underlying mechanisms of population level effects e.g. MPs ingestion causes reduction in predator escape ability, and food intake capacity, blocks enzyme synthesis, causes retarded somatic growth, lower steroid hormone levels, and delayed ovulation leading to reproductive failure (Cole et al., 2011; Everaert et al., 2018; Athey and Erdle, 2022; Galgani et al., 2022; Wright et al., 2013; Ryan, 2015; Kumari et al., 2023). The most common manifestation of the ecological effects of any type of stress is an alteration in the (i) population growth trajectory of a species, and (ii) outcomes of population interactions.
Since both MPs and fungi are morphologically within the dietary niche breadth of zooplankton, they enter the food web and impact trophic structure and trophodynamics, intervening nutrient cycling. Even though some information on MP-mediated changes in energy uptake is available on zooplankton, which constitutes a central trophic link in pelagic food webs (Galloway et al., 2017; Macali and Bergami, 2020), only anecdotal information is available on fungal diversity and ecological function despite their critical ecological significance.
Numerous studies have reported the adverse effects of MPs exposure on single organism (Bergami et al., 2017; Lin et al., 2019; Kumari et al., 2023). However, at the higher levels of the ecological organization (community and ecosystem), and interactions, the MPs mediated impacts are still poorly understood. Ecological interactions such as parasitism, competition, and predation have a greater impact on the major functional components of an ecosystem (biogeochemical cycling, energy flow, ecological succession, and nutrient transfer) than on the fitness of a single species (Kumar, 2003). Biological interactions are also indicators of ecosystem health and the extent of ecological disturbance (Valiente-Banuet et al., 2015). In addition to direct effects, MPs also form a micro-ecosystem by providing artificial substrates for the colonization of diverse organisms (Rosato et al., 2020). These organisms are attached to the surface of MPs or in the extracellular matrix formed by other organisms. MPs constitute an important artificial substrate for fungal colonization as fungi are among the early colonizers of plastic (Jacquin et al., 2019). These fungi play prominent roles in biofilm formation, altering the plastisphere’s properties. A complex range of ecological niches from littoral to the deep sea, and pelagic to abyssal zones having diverse functional roles, is occupied by fungi. Fungi play a prominent role in environmental processes and are associated with organisms representing almost all trophic levels of the marine food web, being saprobic, epibiotic, endobiotic, parasitic, or symbiotic and being a nutritionally enriched food source.
Recent metagenomic studies (Breyer et al., 2022) have brought to light unknown and undetectable marine fungal diversity in widespread habitats, including the water column (Richards et al., 2015), ocean sediments (Raghukumar and Raghukumar, 1998), coral reefs (Golubic et al., 2005), the benthic vent ecosystem trench (Keeler et al., 2021) and animal hosts.
These phylogenetically diverse fungi are likely to be differentiated by geography, environmental conditions, and substrate, providing further insight into the high prevalence of marine fungal pathogens on phytoplankton in marine ecosystems. Understanding fungal interactions in marine ecosystems is based on concepts that are similar to those of their counterparts in terrestrial ecosystems. In spite of higher phylogenetic diversity and nifty metabolic potentials, the kingdom Fungi received little attention in earlier studies on epiplastic or plastisphere community. Significant efforts have been made to link the presence of a fungi to its functional role as detected through metagenomic surveys. Based on our current understanding of the kingdom Fungi, which can be found associated with every organism described so far, fungi have been associated with disease in seagrasses, corals, crustaceans, and higher marine organisms (Ramaiah, 2006). Extracellular enzymes and bioactive secondary metabolites released by fungi have a potential role in influencing microbial communities in marine ecosystems directly or indirectly (Gonçalves et al., 2021) as in terrestrial ecosystems (Kües, 2015). Further, fungi associated with phytoplankton as a pathogen or parasite have an implicit role in the microbial carbon cycle, regulating phytoplankton population and affecting the release of dissolved organic matter that eventually affects the microbial community structure. A clear distinction has been observed between the fungal community in marine, estuarine, and freshwater habitats (Mohamed and Martiny, 2011). According to earlier studies, most organisms are adapted to either freshwater or marine ecosystems. A study by Rojas-Jimenez et al. (2019) reported significant differences in fungal community structure above and below the salinity range of 8 PSU. However, some species, such as Aspergillus flavus, are widespread and can be found in various habitats (Ramírez-Camejo et al., 2012). Lately culture-independent methods of fungal inventory have elucidated several fungal groups including Ascomycota, Basidiomycota and Chytridiomycota and Cryptomycota with relatively higher fractions of Chytridiomycota in marine and freshwater ecosystems (Richards et al., 2015; Kettner et al., 2017), with significantly higher fungal richness and diversity were observed in epiplastic biofilm (Kettner et al., 2017) representing Chytridiomycota, Cryptomycota, Ascomycota and Basidiomycota. Furthermore, organic substances adsorbed to the plastic surface are likely to entice fungi, which Donlan (2002) referred as conditioning of plastic surface for colonization of saprotrophic fungi (Wurzbacher et al., 2011; Richards et al., 2012).
On plastic surfaces, biofilms are formed through the release of extracellular matrix, forming a stable consortium on the surface by several microorganisms including fungi. It is possible that changes in functionality correlate with a change in taxonomic composition (Allison and Martiny, 2008). For example, the numerous Chytridiomycota present in the MPs biofilms may cause various types of saprotrophic or parasitic relationships with the inhabiting primary producers, mediating the movement of energy, carbon, and nutrients into the food chain (Agha et al., 2016). Fungal communities associated with the MPs were shown to be highly dependent on location and substrate types. For example, colonization study in the North Sea reported highly diverse pattern in fungal community structure between harbor and coastal site (De Tender et al., 2017). The association of fungi in MPs biofilm may be attributed to their higher affinity to attach on solid surfaces or to establish a relationship with the other organisms associated with biofilm or fungi may be attracted by organic substrates adsorbed by the MPs.
Unlike, natural substrate, MPs can persist in the environment for a long duration along with the attached biota. So far, the investigated epiplastic communities include prokaryotic bacteria (Stenger et al., 2021), cyanobacteria, and eukaryotic, unicellular and oligocellular-fungi (Reisser et al., 2014), which have been reported from marine and freshwater ecosystems globally (Florio Fumo et al., 2022; Sooriyakumar et al., 2022). Epiplastic fungal communities differ from those in surrounding water or on the natural substrate (Kettner et al., 2017).
The increasing accumulation of MPs and selective colonization of certain fungal groups could be transported to long distances facilitating the spread of fungi to diverse habitats. However, it is not yet clear, how stable are the fungi associated with the MPs and whether they are likely to be changed by local environmental factors or remain more resilient in their micro-niche on the MPs surface. The ecological consequences of distinct fungal communities on MP, however yet to be investigated. Frequent and diverse fungal occurrence and abundance in epiplastic community (Kim et al., 2022), points towards their important role and high relevance in plastisphere micro-ecosystems. The interaction between microbes and plastic surface also influences the effect of microplastics on ecosystems. The large surface area of plastics offers a favorable substrate for microbial colonization and biofilm formation, acting as a protective ecological niche known as plastisphere. Several fungal pathogens and associated production of appressoria (invasive structure used to adhere), biofilm formation, thigmotropism, and mucilage secretion are attributed to higher pathogens prevalence in the plastisphere (Harding et al., 2009). In a recent study of terrestrial ecosystems, 34 to 127 different fungal OTUs have been identified from a single plastic sample (Gkoutselis et al., 2021). Fungi have been reported to have the ability to adhere to plastic surfaces by increasing cell wall hydrophobicity through fungal proteins (Harding et al., 2009). Such effects are likely to take place in different biomes (Gkoutselis et al., 2021; Sheridan et al., 2022). All these attributes point to the modulating role of MPs on fungal population trajectories and predestination of pathogenic fungi to colonize the plastisphere.
Study by Schampera et al. (2021) shows the MPs effect on the chytrid infection on cyanobacterium Planktothrix agardhii, altering outcomes of host–parasite interactions. The modulation in the environment by the MPs had both direct and indirect impacts on the host’s fitness and susceptibility to infection. Aggregation of MPs around the host results in shading and reduced light availability impacting primary productivity, impeding the host growth, and increasing sedimentation. Seena et al. (2019) conducted a microcosm study to understand the impact of nano-polystyrene plastic (100 nm, 0–102.4 mg/L) on the process of litter decomposition by aquatic fungi (hyphomycetes). They recorded different tolerance levels to nano-polystyrene plastic by different fungal species. These studies have shown that species in the surrounding water column are different from the plastisphere (Oberbeckmann et al., 2016), and community composition and succession vary spatially and temporally. Thus, MPs constitutes a microhabitat with relatively higher affinity and flexibility. Such habitat preference and avoidance affect the population trajectory of epiplastic species, and fungi – being pathogens, parasites, symbionts or saprobes – are likely to shape up the community dynamics and cycling processes within plastisphere. Thus the kingdom Fungi with their versatile functional roles as parasites, pathogens, symbionts or saprobes are major determinants of community structure and cycling of materials among plastisphere community.
Being similar in size of autotrophic and heterotrophic protists (Tseng et al., 2011), zooplankton, and fungal spores, MPs are likely to alter the outcome of species interactions leading to alteration at the community level (Lehtiniemi et al., 2018). In consideration with symbiotic, commensal, parasitic, and saprobic association of fungi with prokaryotes, phytoplankton, zooplankton and their abundance in marine ecosystems (Gutiérrez et al., 2011; Bochdansky et al., 2017; Hassett et al., 2019), the role of fungi is not included in marine ecological models (Worden et al., 2015). According to Fryar et al. (2001) and Duarte et al. (2013), the results of species interactions within fungi depend on the availability of nutrients in the fungal substrate. Therefore, from an ecological perspective, it is necessary to take into account environmental factors such as MPs as well as substrate characteristics when studying community assemblages and fungal interactions. Furthermore, fungi and MPs interact (directly or indirectly) with different components of the food web (prokaryotes, phytoplankton and zooplankton), indicating diverse interactions that leads to concurrent changes in ecological process. Major goal of this review is to elucidate modifying role of MPs on fungi–zooplankton interactions. Provided that the majority of zooplankton are either bacterivorous or algaevorous the effects of fungi on prokaryotic bacteria, cyanobacteria and eukaryotic phytoplankton could substantially affect zooplankton community. To understand indirect effects of fungi on zooplankton via prokaryotes and algae, we also review effects of MPs on fungi-prokaryotes and fungi–phytoplankton interactions.
Prokaryotic bacteria and eukaryotic fungi often share microhabitats/substrate where they colonize and establish a dynamic co-evolved community. In addition to sorption of various organic and inorganic compounds, the MPs surface also offer a favorable artificial substrate for biofilm formation, thus facilitate colonization of diverse microbial communities (Zettler et al., 2013; Hoellein et al., 2017; Dussud et al., 2018), which constitutes a preferred ecological niche, continuously expanding due to the discharge of land driven MPs and plastic debris into marine ecosystems.
All the studies on plastisphere community have described coexistence of microbial species representing a wide diversity of fungal and bacterial families (González-Pleiter et al., 2021; Li et al., 2021). MPs surface is colonized by bacteria such as Bacteroidetes, Cyanobacteria, Proteobacteria, Alphaproteobacteria, Gammaproteobacteria, Acinetobacter etc. algae and fungi. They are major recyclers, decomposers and cornerstone communities playing pivotal roles in the functioning of marine ecosystems, regulating biogeochemical cycles. In addition to constituting an artificial island for colonization and biofilm formation, MPs were found to be dispersed in different parts of aquatic habitats including pristine unaffected regions and leach dissolved organic matter (DOM) through physical, chemical, and biological degradation (Suhrhoff and Scholz-Böttcher, 2016; Gewert et al., 2018; Amaral-Zettler et al., 2020). Along with providing physical barrier/substrate, leachate from degraded MPs may affect bacterial population growth due to hazardous compounds added to synthetic polymers. Many of such plastic additives are hydrophobic organic compounds with a property to sorb to polymers. These toxic additives may harm, and potentially bio-magnify to higher trophic levels by bacterivorous organisms. Bacterial response to plastic leachate depends on (i) the natural molecular composition of DOM and (ii) the ability of bacteria to use plastic leachate. Conversely, leachate may be beneficial to bacteria in ambient waters with (i) highly labile DOM, and (ii) providing DOM similar to naturally occurring DOM. These plastic leachates are used as a source of energy by bacterial community and are transferred towards higher trophic levels through food web. Synthetic polymers (Chen et al., 2020) and lignin (Janusz et al., 2017) are gradually degraded by prokaryotes. The fungal diversity on plastic wastes is influenced by the colonizing mechanisms of the microbes (Miao et al., 2023). Fungal species have also been reported in inhabiting other plastic colonising microorganisms (Webb et al., 2000; Gkoutselis et al., 2021). Some Hypocreales and Pleosporales species are also known to obtain their nutrients from other fungi, either by commensalism or parasitism (Sun et al., 2019). Several species in the order Hypocreales and Pleosporales have been reported to have low Polycaprolactone (PCL) degradation ability, indicating that they have alternative sources for nutrients. Because of the composition and amounts of additives, the outcomes of fungi-bacteria interactions are likely to be modified attributed to differential sensitivity to additives for different species. By impacting carbonyl indices, microbial colonization affects the properties of plastic surfaces that in turn determine the colonization and species composition in biofilm. Both, the material type and size determine the biofilm formation and microbial community composition on MPs (Parrish and Fahrenfeld, 2019).
Fungi belonging to the group Chytridiomycota are known to infect phytoplankton. These parasites commonly act as prey either by concomitant predation (get consumed by a predator along with the infected host) or through direct consumption of their free-swimming life forms. A study in the upwelling zone near Central Chile of the Humboldt current system established the presence of mycoloop in the marine food web (Gutiérrez et al., 2016). Food web models also highlight the significance of mediating carbon and energy transfers by chytrid infections, around 20% of gross primary production is conveyed by chytrid parasitism and satisfy about 38% of zooplankton nutritional needs (Grami et al., 2011; Haraldsson et al., 2018). During bloom conditions, large inedible fungi sink from the euphotic zone resulting in a loss of nutrients. During chytrid infection, the nutrient within the host gets consumed by the chytrid and released as free-swimming zoospores which are preferable sources of food in terms of shape and size for the zooplankton. In addition, chytrid infection leads to the fragmentation of large colonies of inedible phytoplankton into smaller ones that become readily edible to zooplankton. This not only reclaims and repackages the lost nutrient but also supplements the diet of zooplankton with additional nutrients as fungal zoospores are rich in cholesterols and polyunsaturated fatty acids that are required for the growth and development of crustaceans in marine environments (Hartwich et al., 2013). Microbial and fungal diversity have also been reported to increase the concentration of blue-green algae after leachate addition. Furthermore, some microbial, fungal, and phytoplankton may be well suited to exploit compounds derived from plastics and eliminate them from the aquatic ecosystem.
The interaction between fungi and zooplankton can be both, direct and indirect (Huang et al., 2020). In the marine ecosystem, fungi are exposed to a degree of fluctuations, which can be reflected by changes in community structure and abundance. It is believed that fungi, with an array of multiple nutritional strategies, can have a notable role in regulating taxon dynamics. Including mutualistic and pathogenic lifestyles, marine fungi are also saprotrophic or symbionts (Jobard et al., 2010). The biotic interactions of aquatic fungi, however, go further than these simple interactions. They may participate in cross-feeding networks, engage in resource competition with different species, or even manufacture allelopathic chemicals. Additionally, it has been demonstrated that spores and fungal tissue serve as a significant source of food for a variety of marine organisms (Cleary and Durbin, 2016). A temporal study by Banos et al. (2020) reported that marine fungi are embedded in the microbial food web through highly complex and manifold interactions. Based on the molecular identification techniques, they revealed that the majority of fungi have a saprotrophic lifestyle, while the other fungi are negatively correlated with zooplankton, especially copepods. Copepods provide a fundamental role in marine productivity and energy transfer, consuming energy from phytoplankton and transferring it to a higher level of organization in the food chain. The links between fungi and copepods were found to be mainly negative, furthermore, fungal assemblage structure differ with copepod species composition (Banos et al., 2020). For instance, Acartia sp., has been recorded to be associated with fungal groups such as Ustillaginomyctes, Malasseziales, Leotiomyctes and Eurotiales, whereas, Calanus sp., was associated with fungal groups Pleosporales, Cyclopoida with Leotiomyctes and Harpellales. However, the most prominent fungi were zoosporic Chytridiomycetes and Cryptomycota, and the filamentous group of Pleosporales (Banos et al., 2020). This could be perceived as top-down regulation with regard to the structure of the marine food web and nutrient/carbon cycle, where one organism becomes more abundant at the cost of the other.
Fungi–zooplankton interaction is vital when blooms of inedible algae persist in natural setup. Phytoplankton species that are resistant to zooplankton grazing, e.g., colonial or filamentous cyanobacteria and diatoms dominate phytoplankton communities in marine ecosystems (Fontana et al., 2007; Perga et al., 2013; Rollwagen-Bollens et al., 2013). The effective transfer of carbon and energy from lower trophic levels to higher levels could be limited by a reduction in grazing, causing trophic bottlenecks. Moreover, the bioavailability and ingestion of MPs by copepods and other zooplankton elevate the impacts depending upon the frequency and extent of the MPs exposure, size, and type (Table 2).
No discernible information is available on the effect of MPs contamination on fungi–zooplankton interaction, where the zooplanktonic communities are likely to be more affected as the particle size of MPs coincides with dietary niche (phytoplankton, fungal spores) and also comparable to their ability to entrain particles (Lehtiniemi et al., 2018). This interaction is further crucial for the filter feeding zooplankton, given their position in marine food webs and major roles in trophic cascading. So far, interaction between fungi and zooplankton is concerned, MPs are likely to alter outcomes of interaction by disrupting the trophic transfer of nutrients and energy through the food chain and by interfering microbial loop and mycoloop.
Until recently, it was believed by marine ecologists that aquatic food webs are relatively simple, comprising of phytoplankton–zooplankton–planktivorous fish–piscivorous fish assisted by microbial loop. The presence of fungi–zoospores, fungal actions in changing the properties of dietary phytoplankton, and entry of MPs into these food webs in aquatic ecosystems were unknown. Recent studies in field and laboratories, and simulation studies with MPs have brought to light that a substantial amount of organic matter in marine and freshwater ecosystems is processed through the complex trophic interactions among fungi, fungi–bacteria, and fungi–algae interaction, which constitute the concept of mycoloop in nearly all aquatic ecosystems (Amend et al., 2019; Breyer et al., 2022). Mycoloop describes a trophic pathway transferring carbon and nutrients to higher trophic levels through the constitution of fungal zoospores in an alternate trophic link between primary and secondary production (Kagami et al., 2014).
In case of diatoms and cyanobacterial blooms, where large inedible algae, dominate phytoplankton assemblages, zooplankton can still gain from fungi through direct consumption, concomitant predation, and free-swimming chytrid zoospores as a food source. Parasitic fungi are ecologically very important in the marine ecosystem, as they regulate the host population, mediate intraspecific competition between infected and uninfected hosts, and interspecific competition between host and other organisms affecting overall community structure. Zooplankton can acquire chytrid fungi (concomitant predation) by ingesting sporangia attached to edible hosts, or by consuming free-swimming chytrid zoospores. Experimental evidence points towards the sustenance of zooplankton growth and reproduction, by zoospores, when poorly edible filamentous cyanobacteria or large inedible diatoms dominate the phytoplankton communities (Kagami et al., 2014). The energy transfer across the trophic levels in the pelagic food web could be greatly enhanced by this mechanism. Additionally, chytrids also increase the digestibility of pollen grains, upgrading the allochthonous organic matter, by making the inaccessible pollen PUFA more accessible (Kagami et al., 2017; Masclaux et al., 2013). However, it is unclear whether nutritional enrichment occurs when carbon is taken up from phytoplankton following chytrid infection. It has been reported that, as zooplankton suffers from essential fatty acid deficiencies, growth and reproduction are reduced during cyanobacteria blooms (Elert et al., 2003; Martin-Creuzburg et al., 2008; Kagami et al., 2011). Polyunsaturated fatty acids (PUFA) and sterols are not synthesized by crustacean zooplankton but acquired from their natural diet. These are essential for the modulation, and formation of cell membranes (Müller-Navarra et al., 2000; Valentine and Valentine, 2004; Martin-Creuzburg et al., 2008). Sterols, especially cholesterol, a major sterol in animals, which act as precursors to steroid hormones (Goad, 1981), and components of the eukaryotic cell membranes. Chytrid parasites produce PUFA, essential lipids, and sterols de novo, which could supplement the zooplankton with lipid in abundance, when taxa lacking essential lipids (such as cyanobacteria), dominate the phytoplankton community.
The rapid increase in the MPs concentration in marine ecosystems could have a negative effect on the material and energy transfer by mycoloop. The presence of MPs has been reported to alter the community composition of both, phytoplankton (Hitchcock, 2022; Cheng et al., 2023) and fungi followed by alteration in the chytrid infection influencing the outcomes (Figure 1). Adverse effects of MPs on the organisms include altered community structure, growth impairment, and hetero-aggregation, impacting parasite fitness and reducing disease prevalence (Wan et al., 2018). Schampera et al. (2021) demonstrated that the presence of nano-sized plastic particles alters the host–parasite environment. Plastic particles form a hetero-aggregation around the phytoplankton inhibiting their growth by reducing photosynthetic productivity and also inhibiting the chytrid infection by acting as a physical barrier to infection and, may also hamper parasite chemotaxis. In the context of mycoloop, this reduced intensity of infection indicates reduced disease transmission that would result in increased phytoplankton bloom with a higher abundance of inedible microalgae and lower zoospore formation which is a critical part of the mycoloop. In combination with the detrimental effects on zooplankton, MPs are likely to exert diverse cascading effects on ecosystem functioning.
Figure 1 Schematic diagram of microplastics-mediated alteration in mycoloop: The conceptual model showing that the microplastics in the environment act as a physical barrier in parasitism, which in turn negatively impacts the fungal action by hampering chemotaxis, increasing oxidative stress and toxicity. The reduced fungal parasitism and lower production of fungal zoospores hamper the transfer of carbon and nutrients to higher trophic levels, having a cascading effects on ecosystem functioning (Kagami et al., 2014).
Apart from its interaction via entanglement and ingestion of MPs, plastics may contain stabilizers, plasticizers, and dyes that could leach, on weathering or aging of plastics, which could affect the biological activities of the zooplankton. Additionally, studies have shown that MPs in association with certain pollutants, owing to the interaction with inorganic and organic compounds, through adsorption is important determinant of the degradability, bioavailability, fate, toxicity, and dispersal, which could lead to adverse implications at various levels of biological organization (Table 2). At the molecular level, reactive oxygen species (ROS) levels rise as a result of increased oxidative stress, and inflammation due to the ingestion of MPs inside the body. Studies have reported that excessive ROS production causes oxidative damage to biomolecules such as lipids, proteins, and DNA (Solomando et al., 2020; Tagorti and Kaya, 2022). Further, Imhof et al. (2017) reported that increased oxidative stress downregulates Hsp70 (Heat shock protein 70) gene expression, responsible for the enhanced transport of proteins in the nucleus, which, in turn impairs the DNA repair mechanism leading to DNA damage.
Intrinsic factors such as properties of MPs, and environmental components, and extrinsic factors like pH, temperature, and ionic strength determine the adsorption of organic biomolecules in the environment. The substances adsorbed on the particulate called Corona and it constitute both “hard” and “soft” corona layer (Lynch and Dawson, 2008; Cao et al., 2022). Unlike the stable hard corona, the components of the soft corona can quickly exchange with the surrounding environment (Cao et al., 2022; Camil Rex et al., 2023). The biomolecular-coated outer layer of natural organic matter (NOM) called ecocorona interacts with plastisphere reacting with biological systems (biocorona) profoundly influence the ecosystem dynamics, bioavailability, degradation, transport and toxicity. Organismic and sub-organismic level effects on aquatic biota incurred by ecocorna are oxidative damage, retarded growth, organelle damage, early mortality, decreased food uptake, inflammation, immune malfunctions and aberrant behavior are the major detrimental effects of MPs on the aquatic biota (Benson et al., 2022; Gao et al., 2022; Camil Rex et al., 2023).
The potential effects of compounds like heavy metals, antibiotics, persistent organic pollutants, and hazardous toxins, present on MPs surfaces could include, increased production of ROS, enhanced toxicity, disrupt metabolism, and impairment of biofilm formation on MPs surfaces. Two factors make the surface of MPs conducive for biofilm formation, (i) the physiochemical attributes of MPs, and (ii) the status of environmental parameters such as nutrient level, salinity, temperature, and location (Sooriyakumar et al., 2022). Colonization of organisms on the surface of MPs makes it readily ingestible by zooplankton, and also adds some nutritional value to the MPs, due to the biofilm adjacent to it. The ingestion of colonized MPs, facilitates the transport (horizontal and vertical) and dispersal of pathogens and parasites through MPs. Interaction of adsorbed compounds such as DMS (dimethyl sulphonamide) on the surface of MPs, may also increase their bioavailability for the organism ingesting the MPs. Synthetic polymers are generally considered non-biodegradable and plastics encompass a number of labile and bioavailable additives – for example, colorants, antioxidants, and plasticizers. These additives, released from plastics after degradation, are likely to accumulate in surface waters at higher concentrations than natural DOM (Jacquin et al., 2019). However, data on the chemical structure and fate of leachate are scarce in comparison to natural DOM, especially in the marine environment.
The impact of MPs on zooplankton depends upon species encounter, interaction, and susceptibility of the MPs to the organism. It has been hypothesized that marine copepod mistakenly identifies plastics as food sources due to the presence of MPs in the organism’s food size range and similarity to prey. Recent evidence also suggests chemosensory cues as a probable factor influencing feeding habits and promoting ingestion of MPs by zooplankton. A variety of zooplankton including copepods have been reported to show foraging behavior and locate the prey density in the presence of chemosensory cues such as DMS. Copepods utilize both chemo and mechanical reception feeding mechanisms to identify and locate prey species.
Certain species of marine algae are known to produce a sulfonium compound, as dissolved dimethyl sulfonio propionate (DMSP) in high concentrations in the surroundings. This algae-associated, particulate DMSP, when released are readily degraded into DMS through enzymatic cleavage of DMSP into the marine environment. DMSP arises primarily from the marine phytoplankton and is also produced by salt marsh cordgrasses and by certain species of macroalgae as an osmo-protectant. A study by Bacic and Yoch (1998) shows that Fusarium elaterium isolated from marine sand salt marsh and other fungal decomposers from group ascomycetes have DMSP lyase activity (enzymatic release of dimethyl sulfide) involved in the elevation of DMS concentration resulted in increased grazing. Exposure of MPs to DMS creates an olfactory trap that could stimulate the foraging behavior of zooplankton for MPs. Recently, a grazing experiment by Procter et al. (2019) reported that the DMS-infused MPs were readily ingested by the Calanus helgolandicus as compared to DMS-free MPs. There was a 0.7 to 3-fold increase in the intake rendering them more palatable to copepods and influencing the framework of the microbial food web. The study also highlighted the absorption of DMS and pollutants by MPs from the surroundings. Further studies have shown that weathered MPs were able to adsorb significantly higher DMS than virgin MPs increasing the detrimental impact on the zooplankton (Breckels et al., 2013). Research by Vroom et al. (2017) showed that the proportion of individuals and number of plastic ingestion depends upon species, life stage, and size of plastic particles. MPs were ingested by all the stages of Calanus finmarchicus. Whereas, juvenile copepodites of Acartia longiremis females and Calanus finmarchicus adults preferred aged MPs compared to pristine ones. This was accredited to the formation of a biofilm that may contain similar prey as the copepods encountered in the water column and secreting chemical oozes that acted as cue for copepods. It is yet, not fully understood whether variation in shapes, sizes, and colors of MPs can influence their ingestion rates in copepod and if the presence of a DMS predominates this process of selection. DMS production by bacteria, the biofilm being inhabited by algae, and fungi having DMS ligase activity, are ways by which the significance of the existence of a biofilm can be accounted. Further research on the influence of biofilm formation, on copepod ingestion rates, is required.
It is becoming evident that MPs are not limited to the ocean’s surface and that some way or another, these particles eventually find their way to the ocean floor. Studies have noted the presence of MPs in marine snow and organics aggregates (Long et al., 2015; Porter et al., 2018) representing the movement of MPs from the surface layer to the deep ocean and sediments. Marine snow act as a downward flux transferring carbon and energy from the pelagic zone to the benthic biota as well as to other organism in the water column contributing to the global carbon cycle. The movement of particulate organic matter from the surface to the deep sea is composed of fecal pellets from pelagic organisms, phyto-detritus, and organic aggregates. Interaction between zooplankton and marine snow may influence the pelagic food web through direct feeding on aggregates and repackaging into dense fecal pellets or fragmentation of aggregates on interaction resulting in alteration in size, density, and sinking velocity (Turner, 2015). Thus, influencing the nutrient flux by zooplankton. A study by Van Der Jagt et al. (2020) showed that aggregate feeding by arctic filter-feeding copepods Calanus sp. and Pseudocalanus sp. on settling aggregates reduced around 60–65% carbon. Further, grazing experiments by Cawley et al. (2021) using gut pigments and stable isotope methods on the ingestion of the copepod Calanus pacificus, on both marine aggregates and phytoplankton shows that marine snow can be an important source of nutrient for copepod, comparable to individual phytoplankton. It has been reported that the marine snow in the bathypelagic realm of the marine ecosystem is dominated by fungi and labyrinthulomyctes in terms of biomass (Bochdansky et al., 2017). Fungi reported from marine snow were majorly saprotrophic, and are known to decompose detritus material in water and have the potential to degrade recalcitrant organics matter and contribute to particulate organic matter flux which can create a nutrient-rich environment that supports the copepod population. The presence of fungi shows its tolerance to high hydrostatic pressure and cold temperature. Further, the distribution and functionality of marine snow are influenced by the presence of MPs. Research by Kvale et al. (2020) presented an estimate of the transfer of MPs from the pelagic zone to the deep through biological fouling and de-fouling. The uptake and release of marine snow by the zooplankton not only redistribute the MPs but also potentially increases the bioavailability through bioconcentration processes. This led to various issues like how the existence of MPs in the marine snow impacts the carbon transfer to the bottom of the ocean from the surface, the degradation of recalcitrant organic matter by fungi and other microorganisms, a potential vector for infection, and the influence of plastics on the ecological health of the organism consuming marine snow.
The effects of the ingestion of MPs by the copepods can be reflected in other trophic levels that are directly or indirectly associated with them. Recent evidence shows that ingestion of MPs by the copepods results in a reduction in the size of fecal pellets and a decrease in the organic contents (Cole et al., 2016) which are utilized by the organisms dwelling in the water column as well as benthic inhabitants as an important source of energy and nutrient to the deep waters. Further incorporation of MPs in fecal pellets could change the size and shape of fecal pellets (Figure 2) and alter their rate of sinking.
Figure 2 Photograph of fecal pellets of invasive snail Physa acuta. (A) Fecal pellet without the presence of microplastics. (B) Fecal pellet in presence of microplastics. Study reported that the microplastic ingestion by the organism cause altered shape and size of fecal pellets as compared to control. Adapted from Kumari et al. (2023).
A study by Coppock et al. (2019) shows that the incorporation of high-density significantly increases the sinking rate, while the presence of low-density MPs decreases the sinking rate, and increases buoyancy. Reduced sinking rate and lateral advection rather than the vertical flux of fecal pellets could enhance the probability of further disintegration by zooplankton and colonization of fungi, bacteria, and other microorganisms facilitating remineralization in the pelagic zone only. This could strain the process of nutrient recycling, moving the balance from the water column to the surface, and influencing the flow of carbon to the benthic zone (Figure 3). Future research is essential to determine the possible effects of this biogeochemical cascade on the oceanic carbon cycle and the capacity of the benthic zone to absorb organic carbon fixed in the pelagic zone.
Figure 3 A conceptual model of microplastics mediated alterations in ecological functioning of the aquatic environment.
With the potential of long-distance transportation, research has shown that MPs could serve as physical vectors, spreading pathogens (Kirstein et al., 2016; Virsek et al., 2017). It has been widely explained that in marine ecosystems, MPs quickly develop a conditioning film that markedly differs from the surroundings with the increasing incidence of the presence of pathogenic microorganisms in these MPs surfaces (Zettler et al., 2013). Thus, in ocean, MPs act as a raft for pathogenic bacteria, invasive species and fungi. This along with the long-range transport raises concerns about the increase in disease outbreaks. Fungi are found to act as pathogens and can infect various zooplankton including copepods. In a study by Seki and Fulton (1969) it was observed that the tissues of copepods were infected by Metschnikowia species. Physiological characteristics suggested terrestrial origin of yeast and are adapted to marine ecosystems. A recent study on nanoplastics by Mavrianos et al. (2023) showed how plastic pollution affects the infection of yeast in diverse ways. There was an increase in the proportion of host infection under plastic exposure, whereas, reduced disease prevalence was observed due to increase in host mortality under higher plastic concentration. Research by Bosch et al. (2021) stated that the presence of MPs increases the incidence of chytridiomycosis, a disease caused by the chytrid fungus Batrachochytrium dendrobatidis to amphibian larvae. Thus, growing evidence suggests that MPs act as a carrier of pathogens and parasites, whereas, the factors promoting the association with MPs are still unknown, and future research is required.
It is possible that changes in functionality correlate with a change in taxonomic composition (Allison and Martiny, 2008). For example, the numerous Chytridiomycota present in the MPs biofilms may exhibit parasitism or saprotrophism with primary producers, which are also present, mediating the movement of carbon, nutrients, and energy into the food webs (Agha et al., 2016). The presence of polysaccharide-rich diatom biofilms is being reported in marine MPs (Lacerda et al., 2019), this may elucidate the occurrence and alliance of fungi with biofilms on plastics surfaces (Kettner et al., 2017) and also MPs modulated roles of fungi in ecosystem functioning. Essentially, there are two key selection forces that determine the community structure in the marine ecosystem and to which the life forms have to adapt are salinity and hydrodynamic forcing. Salinity causes osmotic stress, whereas, hydrodynamic forcing affects habitat shifting and physical imbalance (Tisthammer et al., 2016). The existence of the high-osmolarity-glycerol signaling pathway, the regulation of salt efflux, and the synthesis of osmolytes compatible with cellular functions are major adaptations of fungi to the marine ecosystem (Gladfelter et al., 2019). Besides this, various morphological adaptations (e.g., in chytrids) in fungal structure and spore-dispersion strategies are present to survive in high salinity levels (Greenwood, 2007) and turbulent oceans. Essentially, in the marine ecosystem, the interaction of organisms is also influenced by the hydrodynamic forcing of the water. Studies have shown that encounter rates are increased in a turbulent flow, which is characterized by unpredictable variations in velocity gradients and directions (Mahjoub et al., 2012). The impact of hydrodynamic forcing has been studied for various organisms especially focusing on prey–predator interactions (Rothschild and Osborn, 1988), while in the case of marine fungi, no study reports the effects of hydrodynamic forcing on the survival and fitness of fungi. However, very limited researches have investigated the effect of hydrodynamic forcing on fungal spores. They were found to adhere to the substratum under turbulent conditions. This adherence is assumed to be facilitated by rapid mucilage production from zoospores (Hyde et al., 1998), accompanied by an increase in the attachment of the spore wall/appendages to the substratum. The low turbulence level enhances MPs ingestion rates in zooplankton by increasing the encounter probability between zooplankton and MPs particles. Secondary consumers (predatory copepods, chaetognaths, and luciferids) are probably more affected by MPs because they could ingest microplastics via contaminated prey or accidentally ingest them owing to confusion in the motion signals, especially under turbulent conditions. Turbulence induces higher encounters and may also cause the entanglement of MPs in the copepods body because of their complex morphology. Encounters between zooplankton and microplastics do not always result in ingestion or entanglement, but the higher encounters escalate risk for the species.
The higher concentration of leachate from plastics than natural DOM is likely to favour bacterioplankton concentrations in surface waters. Further, the compositional differences in plastic leachate and natural DOM is also supposed to favour the bacterial multiplication by supplying more niches for degradation and decomposition (Jacquin et al., 2019). There is information on the effect plastic leachates on bacterial growth, but data on molecular composition of DOM and plastic leachates are lacking. Bacteria and fungi as decomposers are important in maintaining ecosystem stability and resilience by regulating the cycling of materials in the ecosystem. Except few publications, information is lacking on the interlinkage between MPs and decomposers. MPs in aquatic ecosystems may interrupt nitrogen cycling by altering microbial productivity, and community structure (Shen et al., 2022). Recent discovery of diverse and active bacteria and fungi from plastispheres as compared to the surrounding water column hints to MPs mediated larger impacts on the global biogeochemical cycles. Furthermore, the position of planktonic and colonized fungi in the food chain makes them more susceptible to threats imposed by MPs due to their small size of the planktonic stages (Botterell et al., 2019; Franzellitti et al., 2019). Thus, it is crucial to study these new classes of pollutants and their effects on ecological functions, and plankton diversity.
Both plastic wastes and their leachates affect bacterial and fungal populations in marine ecosystems. The early colonizers like bacteria facilitate polymer oxidation, that in turn increases the hydrophilicity, weakens their chemical bonds, and enables secondary colonizers like fungi to access the MPs surface (Oberbeckmann and Labrenz, 2020). Various fungal species have been reported to colonize plastic waste and participate in polymer degradation. Kim et al. (2022) collected fungal communities from plastic waste samples from the sea coast and conducted a degradation assay to see the potential of these fungi to degrade PCL. In that study, highly abundant species (Acremonium cf. fuci, Pleosporales sp., and Paradendryphiella arenariae) recorded lower level of PCL degradation ability. These authors reported that fungal species on plastic waste, which play diverse roles, were the more prevalent species in the plastisphere, are weak plastic degraders, and grow on plastic waste by using resources predominantly degraded by various environmental factors. Fungal species that showed higher PCL degradation potential in the laboratory such as, Phaeophleospora eucalypticola and C. xanthochromaticum were less abundant in plastisphere (Kim et al., 2022). Additionally, fungal species such as Aspergillus sp., Fusarium sp., Penicillium simplissimum, and other plastic-deteriorating fungi have been identified and studied in the landfill (terrestrial) plastisphere (Yamada-Onodera et al., 2001; Zahra et al., 2010; Kanelli et al., 2015; Muhonja et al., 2018) that might be prospected to degrade plastic in marine environment. Integration of different trophic compartments encountered by MPs is likely to yield better results towards biodegradation of MPs. For instance, epicorona and biocorona formation determines bioavailability, internalization by organisms, biofilm formation determining plastisphere community. Ingestion by pelagic and benthic micro-eukaryotes alters the surface property, size, stability, surface charge, and digestion of soft corona enhancing biodegradability by bacteria and fungi.
In the present review, we present three aspects of MPs effects on marine ecosystem functioning, (i) MPs-mediated alteration in outcomes on the interaction between fungi and other planktonic groups, (ii) MPs as a vehicle of spreading disease pathogens, leachates, and toxic additives to marine organisms and (iii) environmental application of biota in plastisphere biofilm in mitigation of plastic contamination. To summarize, the occurrence of MPs in various concentrations has been reported in oceans (Table 1), estuaries, and rivers around the world. Because of the diversity in types, polymers, shapes, and sizes, they have harmful effects on living organisms from the molecular level to the disruption of ecosystem processes. These MPs enter the organism via various routes and interfere with their physiological functions such as locomotion, digestion, and swimming resulting in pseudo satiety, weakness, and nutrient deficiencies resulting in population-level adverse outcomes. Further entanglement and interference by MPs are causing severe damage to biodiversity and unknown impacts that have not yet been analyzed. At present, much information is available for individual or population but impacts at the community level and ecological interactions are still missing. Researchers have focused primarily on single species or trophic transfer of MPs through short-term experiments at far more than realistic environmental concentrations, thereby undermining the ecological relevance. Through interaction between fungi and zooplankton, it has been perceived that MPs not only disrupt the trophic transfer of nutrients and energy through the food chain but also through microbial loop, mycoloop, and transfer of energy to benthos via marine snow (Figure 3). Initial colonizers of plastic surface prokaryotes alter the substrate attributes through biofouling, increasing hydrophilicity and roughness, which facilitate colonization by fungal populations including plastic degrading fungi. Thus, comprehensive investigation combining various trophic compartments directly exposed to MPs need to be undertaken to understand the role of pelagic organisms, benthic organisms, pioneer colonizers of plastic surface such as bacteria, and stable plastisphere community including eukaryotic yeast, fungi, and protists.
It is more important to investigate the coastal ecosystem, estuaries, lagoons, and beaches that are inhibited by intertidal species and meiobenthic species that could be impacted by MPs more intensely. So far, only anecdotal information is available on MPs-mediated impacts on the population, ecosystem, and community level. Comparative data on the effects of MPs on fungi, when present alone, or in community, or as part of the plastisphere community is scarce to understand impacts at different ecological levels. The understanding of the impact of increasing bioavailability of MPs and biofilm formation is still underdeveloped. Further investigation is required to explore the degradation of compounds on MPs surfaces, along with insights on the difference in microbial community structure in virgin MPs and naturally degraded MPs. In order to develop strategy to harvest the ecological function of fungi and mitigation of MPs contamination species specific potential of degrading differently aged plastics is very essential.
Furthermore, several intriguing questions remain to be answered that demands further well-planned investigation to elicit the role of MPs in altering the ecosystem services performed by fungi in marine ecosystems. A large number of published papers either describe MPs concentration in different regions of the ocean or the plastisphere community and biofilm formation. Many studies on plastic interaction and plastisphere focused mainly on bacteria. However, other microorganisms including fungi have rarely been investigated. The additional interactions through mycoloop may play a vital role in ecosystem metabolism. The chemical constitution of plastic leachates and their effects on the plastisphere community has rarely been investigated; molecular characterization of DOM and leachates is essentially required to understand the differential impacts of leachates and DOM on microbial multiplication. On the other hand, microbes with a potential of utilizing leachates may differ from plastic-degrading bacteria. No comparative study is available so far. Further, the interactions among fungal species on plastic substrata have not yet been studied. No information is available on fungal species/communities associated with a particular plastic surface at a particular time as a determinant of the plastisphere biofilm community structure in the plastisphere.
The carbon cycle at the core of aquatic biological pump plays a key role in nutrient recycling. In this process, phytoplankton-driven photosynthesis draws carbon from the atmosphere into the ocean. This organic carbon is largely utilized by microbes and transported to the benthos or higher trophic levels through various food chains (Fuhrman, 1992). Despite the abundant evidence on the assimilation capacity of marine fungi, and their ability to translocate essential amounts of DOM (Cunliffe et al., 2017), they are largely neglected from the existing structure of microbial loop, which includes archaea, eukaryotic protists, and bacteria (Worden et al., 2015). In addition to altering fungal community structure, MPs have been shown to impact the ecological functioning of fungi.
Further, reducing disease prevalence as free-living chytrid zoospores use photosynthetic oozes as chemotaxis to spread infection. The aggregation of MPs, around the host and parasite forms a physical barrier, inhibiting direct contact between the host and parasite that is necessary for infection prevalence. However, the direction of the outcomes of ecosystem interaction between parasite/pathogen, MPs, and host are dictated by differences in infection strategies and by the more complex interactions between importantly, coexisting with naturally occurring predators, such as protists, rotifers and crustaceans. These plankton have the potential to use zoospores as a food source and also have the capacity to capture MPs from the water column. It is unknown to what extent it happens and whether it deteriorates or reduces the parasite infection, but it needs to be investigated. This review work can now serve as a basis for future research with an objective of revealing ecological functions by specific functional group of interest such as plastic degraders, pathogen careers and algal parasites.
This article presents a succinct overview of available scientific information on the environmental behavior of MPs, including interactions with biomolecules, myco, and microbial loop. Understanding and evaluating the influence of MPs contamination on the environment, and the ecosystem processes, are topics of significant interest. MPs enter the ecosystem via variety of pathways, due to their non-biodegradable nature and can persist in the ecosystem for decades or even millennia. These MPs become part of the community and affect organisms at all trophic levels, from bacteria to piscivorous fish. The outcome of MPs interactions at the community level will be different from impacts at the molecular, cellular, or individual levels. The biological consequences of MPs on marine zooplankton have received a lot of attention recently, but research on MPs’ effects on marine fungi is still in its early stages, and little is known about their diversity, community structure or how the MPs affect it. There is no doubt that fungi play a pivotal role in shaping the eukaryotic microbial community via diverse interaction. In the view of adverse impact of MPs on the marine ecosystem, additional and comprehensive studies are required.
1. To address the contribution of MPs to the spread of pathogens and disease outbreaks. Research needs to be focused on the hitch-hike of pathogens on MPs and their transport to different compartments of the marine ecosystem. The shapes and concentrations of MPs in the environment also influence their transport and fate. Proper ecological risk assessment by MPs cannot be achieved by using only particular kinds or MPs types as the study model. So, polymer-type and shape-specific studies on ecological risk are also required.
2. To elucidate the role of the physico-chemical attributes of the plastisphere in the development of microbial communities on their surface and in shaping the community structure of the surrounding environment.
3. To investigate the effects of the concurrent presence of the zooplankton along with microbial community on the plastisphere such as degrading bacteria, fungi (plastics degrading species), phytoplankton, diatoms, and meiobenthos at the bottom, affect the plastic degradation through digestion, enzymatic secretion, biomass accumulation and acting as a sink.
4. To elucidate the impact of MPs coupled with other contaminants and stressors on marine fungi and copepods, assessing the ecological risk that persists from MPs pollution.
5. There could be further studies on the context of the role of mycoloop on the feeding and life history traits of copepods, considering their survival and reproduction. The feeding preferences and longevity are mostly phenotypic manifestations of the effect of surroundings, which would generate more inclusive information about MPs-mediated changes in the ecosystem functioning of fungi and copepods.
Thus, future research should concentrate on ecosystem-level studies involving species from various trophic levels giving more mechanistic insights into the biological effects and environmental behavior of MPs along with their implications on the ecosystem processes.
DY: Data curation, Formal Analysis, Investigation, Methodology, Writing – original draft. RK: Conceptualization, Methodology, Resources, Supervision, Visualization, Writing – review & editing.
The author(s) declare that no financial support was received for the research, authorship, and/or publication of this article.
Authors thank Prof. T. R. Rao for reviewing and valuable comments on the first draft of the manuscript. DKY thank UGC for a Senior Research fellowship and CUSB for providing facilities.
The authors declare that the research was conducted in the absence of any commercial or financial relationships that could be construed as a potential conflict of interest.
The author(s) declared that they were an editorial board member of Frontiers, at the time of submission. This had no impact on the peer review process and the final decision.
All claims expressed in this article are solely those of the authors and do not necessarily represent those of their affiliated organizations, or those of the publisher, the editors and the reviewers. Any product that may be evaluated in this article, or claim that may be made by its manufacturer, is not guaranteed or endorsed by the publisher.
Agha R., Saebelfeld M., Manthey C., Rohrlack T., Wolinska J. (2016). Chytrid parasitism facilitates trophic transfer between bloom-forming cyanobacteria and zooplankton (Daphnia). Sci. Rep. 6 (1), 35039. doi: 10.1038/srep35039
Allison S. D., Martiny J. B. H. (2008). Resistance, resilience, and redundancy in microbial communities. Proc. Natl. Acad. Sci. U.S.A. 105 (supplement_1), 11512–11519. doi: 10.1073/pnas.0801925105
Amaral-Zettler L. A., Zettler E. R., Mincer T. J. (2020). Ecology of the plastisphere. Nat. Rev. Microbiol. 18 (3), 139–151. doi: 10.1038/s41579-019-0308-0
Amend A., Burgaud G., Cunliffe M., Edgcomb V. P., Ettinger C. L., Gutiérrez M. H., et al. (2019). Fungi in the marine environment: open questions and unsolved problems. mBio 10 (2), e01189–e01118. doi: 10.1128/mBio.01189-18. Edited by D.A. Garsin.
Athey S. N., Erdle L. M. (2022). Are we underestimating anthropogenic microfiber pollution? A critical review of occurrence, methods, and reporting. Environ. Toxicol. Chem. 41 (4), 822–837. doi: 10.1002/etc.5173
Bacic M. K., Yoch D. C. (1998). In vivo characterization of dimethylsulfoniopropionate lyase in the fungus fusarium lateritium. Appl. Environ. Microbiol. 64 (1), 106–111. doi: 10.1128/AEM.64.1.106-111.1998
Banos S., Gysi D. M., Richter-Heitmann T., Glöckner F. O., Boersma M., Wiltshire K. H., et al. (2020). Seasonal dynamics of pelagic mycoplanktonic communities: interplay of taxon abundance, temporal occurrence, and biotic interactions. Front. Microbiol. 11. doi: 10.3389/fmicb.2020.01305
Beiras R., Muniategui-Lorenzo S., Rodil R., Tato T., Montes R., López-Ibáñez S., et al. (2019). Polyethylene microplastics do not increase bioaccumulation or toxicity of nonylphenol and 4-MBC to marine zooplankton. Sci. Total Environ. 692, 1–9. doi: 10.1016/j.marpolbul.2018.11.029
Bellas J., Gil I. (2020). Polyethylene microplastics increase the toxicity of chlorpyrifos to the marine copepod Acartia tonsa. Environ. pollut. 260, 114059. doi: 10.1016/j.envpol.2020.114059
Benson N. U., Agboola O. D., Fred-Ahmadu O. H., Dela-Torre G. E., Oluwalana A., Williams A. B. (2022). Micro(nano)plastics prevalence, food web interactions, and toxicity assessment in aquatic organisms: A review. Front. Mar. Sci. 9. doi: 10.3389/fmars.2022.851281
Bergami E., Pugnalini S., Vannuccini M. L., Manfra L., Faleri C., Savorelli F., et al. (2017). Long-term toxicity of surface-charged polystyrene nanoplastics to marine planktonic species Dunaliella tertiolecta and Artemia franciscana. Aquat. Toxicol. 189, 159–169. doi: 10.1016/j.aquatox.2017.06.008
Bochdansky A. B., Clouse M. A., Herndl G. J. (2017). Eukaryotic microbes, principally fungi and labyrinthulomycetes, dominate biomass on bathypelagic marine snow. ISME J. 11 (2), 362–373. doi: 10.1038/ismej.2016.113
Bosch J., Thumsová B., López-Rojo N., Pérez J., Alonso A., Fisher M. C., et al. (2021). Microplastics increase susceptibility of amphibian larvae to the chytrid fungus Batrachochytrium dendrobatidis. Sci. Rep. 11 (1), 22438. doi: 10.1038/s41598-021-01973-1
Botterell Z. L., Beaumont N., Dorrington T., Steinke M., Thompson R. C., Lindeque P. K. (2019). Bioavailability and effects of microplastics on marine zooplankton: A review. Environ. pollut. 245, 98–110. doi: 10.1016/j.envpol.2018.10.065
Breckels M., Bode N., Codling E., Steinke M. (2013). Effect of grazing-mediated dimethyl sulfide (DMS) production on the swimming behavior of the copepod calanus helgolandicus. Mar. Drugs 11 (7), 2486–2500. doi: 10.3390/md11072486
Breyer E., Zhao Z., Herndl G. J., Baltar F. (2022). Global contribution of pelagic fungi to protein degradation in the ocean. Microbiome 10 (1), 143. doi: 10.1186/s40168-022-01329-5
Camil Rex M., Abhrajit Debroy M., Nirmala J., Mukherjee A. (2023). Ecotoxicological significance of bio-corona formation on micro/nanoplastics in aquatic organisms. RSC Adv. 13, 22905–22917. doi: 10.1039/d3ra04054b
Cao J., Yang Q., Jiang J., Dalu T., Kadushkin A., Singh J., et al. (2022). Coronas of micro/nano plastics: a key determinant in their risk assessments. Part. Fibre Toxicol. 19, 55. doi: 10.1186/s12989-022-00492-9
Cawley G. F., Décima M., Mast A., Prairie J. C. (2021). The effect of phytoplankton properties on the ingestion of marine snow by Calanus pacificus. J. Plankton Res. 43 (6), 957–973. doi: 10.1093/plankt/fbab074
Chen C. C., Dai L., Ma L., Guo R. T. (2020). Enzymatic degradation of plant biomass and synthetic polymers. Nat. Rev. Chem. 4 (3), 114–126. doi: 10.1038/s41570-020-0163-6
Cheng S., Yoshikawa K., Cross J. S. (2023). Effects of nano/microplastics on the growth and reproduction of the microalgae, bacteria, fungi, and Daphnia magna in the microcosms. Environ. Tech. Inno. 31, 103211. doi: 10.1016/j.eti.2023.103211
Cleary A. C., Durbin E. G. (2016). Unexpected prevalence of parasite 18S rDNA sequences in winter among Antarctic marine protists. J. Plankton Res. 38 (3), 401–417. doi: 10.1093/plankt/fbw005
Cole M., Lindeque P. K., Fileman E., Clark J., Lewis C., Halsband C., et al. (2016). Microplastics alter the properties and sinking rates of zooplankton faecal pellets. Environ. Sci. Technol. 50 (6), 3239–3246. doi: 10.1021/acs.est.5b05905
Cole M., Lindeque P., Halsband C., Galloway T. S. (2011). Microplastics as contaminants in the marine environment: a review. Mar. pollut. Bull. 62 (12), 2588–2597. doi: 10.1016/j.marpolbul.2011.09.025
Coppock R. L., Galloway T. S., Cole M., Fileman E. S., Queirós A. M., Lindeque P. K. (2019). Microplastics alter feeding selectivity and faecal density in the copepod, Calanus helgolandicus. Sci. Total Environ. 687, 780–789. doi: 10.1016/j.scitotenv.2019.06.009
Courtene-Jones W., Van Gennip S., Penicaud J., Penn E., Thompson R. C. (2022). Synthetic microplastic abundance and composition along a longitudinal gradient traversing the subtropical gyre in the North Atlantic Ocean. Mar. pollut. Bull. 185, 114371. doi: 10.1016/j.marpolbul.2022.114371
Cózar A., Martí E., Duarte C. M., García-de-Lomas J., Van Sebille E., Ballatore T. J., et al. (2017). The Arctic Ocean as a dead end for floating plastics in the North Atlantic branch of the Thermohaline Circulation. Sci. Adv. 3 (4), e1600582. doi: 10.1126/sciadv.1600582
Cunliffe M., Hollingsworth A., Bain C., Sharma V., Taylor J. D. (2017). Algal polysaccharide utilisation by saprotrophic planktonic marine fungi. Fungal Ecol. 30, 135–138. doi: 10.1016/j.funeco.2017.08.009
Desforges J.-P. W., Galbraith M., Dangerfield N., Ross P. S. (2014). Widespread distribution of microplastics in subsurface seawater in the NE Pacific Ocean. Mar. pollut. Bull. 79 (1–2), 94–99. doi: 10.1016/j.marpolbul.2013.12.035
De Tender C., Devriese L. I., Haegeman A., Maes S., Vangeyte J., Cattrijsse A., et al. (2017). Temporal dynamics of bacterial and fungal colonization on plastic debris in the North sea. Environ. Sci. Technol. 51 (13), 7350–7360. doi: 10.1021/acs.est.7b00697
Donlan R. M. (2002). Biofilms: Microbial life on surfaces. Emerg. Infect. Dis. 8, 881–890. doi: 10.3201/eid0809.020063
Do Sul J. A., Costa M. F., Barletta M., Cysneiros F. J. A. (2013). Pelagic microplastics around an archipelago of the Equatorial Atlantic. Mar. Poll. Bull. 75 (1–2), 305–309. doi: 10.1016/j.marpolbul.2013.07.040
Drago C., Weithoff G. (2021). Variable fitness response of two rotifer species exposed to microplastics particles: the role of food quantity and quality. Toxics 9 (11), 305. doi: 10.3390/toxics9110305
Duarte S., Fernandes I., Nogueira M. J., Cássio F., Pascoal C. (2013). Temperature alters interspecific relationships among aquatic fungi. Fungal Ecol. 6 (3), 187–191. doi: 10.1016/j.funeco.2013.02.001
Dussud C., Meistertzheim A. L., Conan P., Pujo-Pay M., George M., Fabre P., et al. (2018). Evidence of niche partitioning among bacteria living on plastics, organic particles and surrounding seawaters. Environ Pollut 236, 807–816. doi: 10.1016/j.envpol.2017.12.027
Elert E. V., Martin-Creuzburg D., Le Coz J. R. (2003). Absence of sterols constrains carbon transfer between cyanobacteria and a freshwater herbivore (Daphnia galeata). Proc. R. Soc Lond. B. 270 (1520), 1209–1214. doi: 10.1098/rspb.2003.2357
Eriksen M., Mason S., Wilson S., Box C., Zellers A., Edwards W., et al. (2013). Microplastic pollution in the surface waters of the Laurentian Great Lakes. Mar. pollut. Bull. 77 (1–2), 177–182. doi: 10.1016/j.marpolbul.2013.10.007
Everaert G., Van Cauwenberghe L., De Rijcke M., Koelmans A. A., Mees J., Vandegehuchte M., et al. (2018). Risk assessment of microplastics in the ocean: Modelling approach and first conclusions. Environ. pollut. 242, 1930–1938. doi: 10.1016/j.envpol.2018.07.069
Felten V., Toumi H., Masfaraud J. F., Billoir E., Camara B. I., Férard J. F. (2020). Microplastics enhance Daphnia magna sensitivity to the pyrethroid insecticide deltamethrin: Effects on life history traits. Sci. Total Environ. 714, 136567. doi: 10.1016/j.scitotenv.2020.136567
Florio Furno M., Poli A., Ferrero D., Tardelli F., Manzini C., Oliva M., et al. (2022). The culturable mycobiota of sediments and associated microplastics: from a harbor to a marine protected area, a comparative study. Jo. F. 8 (9), 927. doi: 10.3390/jof8090927
Fontana A., d’Ippolito G., Cutignano A., Romano G., Lamari N., Massa Gallucci A., et al. (2007). LOX-induced lipid peroxidation mechanism responsible for the detrimental effect of marine diatoms on zooplankton grazers. ChemBioChem 8 (15), 1810–1818. doi: 10.1002/cbic.200700269
Franzellitti S., Canesi L., Auguste M., Wathsala R. H., Fabbri E. (2019). Microplastic exposure and effects in aquatic organisms: a physiological perspective. Environ. Toxicol. Pharmacol. 68, 37–51. doi: 10.1016/j.etap.2019.03.009
Fryar S. C., Yuen T. K., Hyde K. D., Hodgkiss I. J. (2001). The Influence of Competition between Tropical Fungi on wood colonization in Streams. Microb. Ecol. 41 (3), 245–251. doi: 10.1007/s002480000075
Frydkjær C. K., Iversen N., Roslev P. (2017). Ingestion and egestion of microplastics by the cladoceran Daphnia magna: effects of regular and irregular shaped plastic and sorbed phenanthrene. Bull. Environ. Contam. Toxicol. 99, 655–661. doi: 10.1007/s00128-017-2186-3
Fuhrman J. (1992). “Bacterioplankton Roles in Cycling of Organic Matter: The Microbial Food Web,” in Primary Productivity and Biogeochemical Cycles in the Sea. Eds. Falkowski P. G., Woodhead A. D., Vivirito K. (Boston, MA: Springer US), 361–383. doi: 10.1007/978-1-4899-0762-2_20
Galgani L., Goßmann I., Scholz-Böttcher B., Jiang X., Liu Z., Scheidemann L., et al. (2022). Hitchhiking into the Deep: How Microplastic Particles are Exported through the Biological Carbon Pump in the North Atlantic Ocean. Environ. Sci. Technol. 56 (22), 15638–15649. doi: 10.1021/acs.est.2c04712
Galloway T. S., Cole M., Lewis C. (2017). Interactions of microplastic debris throughout the marine ecosystem. Nat. Ecol. Evol. 1 (5), 116. doi: 10.1038/s41559-017-0116
Gao D., Liu X., Junaid M., Liao H., Chen G., Wu Y., et al. (2022). Toxicological impacts of micro(nano)plastics in the benthic environment. Sci. Total Environ. 836 (2022), 155620. doi: 10.1016/j.scitotenv
Garza T. N., Barnes D. K. A., Scourse J. D., Whitaker J. M., Janosik A. M. (2023). Quantifying microplastics in fjords along the Western Antarctic Peninsula. Mar. Poll. Bull. 193, 115144. doi: 10.1016/j.marpolbul.2023.115144
Gewert B., Plassmann M., Sandblom O., MacLeod M. (2018). Identification of chain scission products released to water by plastic exposed to ultraviolet light. Environ. Sci. Technol. Lett. 5 (5), 272–276. doi: 10.1021/acs.estlett.8b00119
Gkoutselis G., Rohrbach S., Harjes J., Obst M., Brachmann A., Horn M. A., et al. (2021). Microplastics accumulate fungal pathogens in terrestrial ecosystems. Sci. Rep. 11 (1), 13214. doi: 10.1038/s41598-021-92405-7
Gladfelter A. S., James T. Y., Amend A. S. (2019). Marine fungi. Curr. Biol. 29 (6), R191–R195. doi: 10.1016/j.cub.2019.02.009
Goad L. J. (1981). Sterol biosynthesis and metabolism in marine invertebrates. Pure Appl. Chem. 53 (4), 837–852. doi: 10.1351/pac198153040837
Golubic S., Radtke G., Campion-Alsumard T. L. (2005). Endolithic fungi in marine ecosystems. Trends Microbiol. 13 (5), 229–235. doi: 10.1016/j.tim.2005.03.007
Gonçalves M. F. M., Hilário S., Van De Peer Y., Esteves A. C., Alves A. (2021). Genomic and Metabolomic Analyses of the Marine Fungus Emericellopsis cladophorae: Insights into Saltwater Adaptability Mechanisms and Its Biosynthetic Potential. Jo. F. 8 (1), 31. doi: 10.3390/jof8010031
González-Pleiter M., Velázquez D., Casero M. C., Tytgat B., Verleyen E., Leganés F., et al. (2021). Microbial colonizers of microplastics in an Arctic freshwater lake. Sci. Total Environ. 795, 148640. doi: 10.1016/j.scitotenv.2021.148640
Grami B., Rasconi S., Niquil N., Jobard M., Saint-Béat B., Sime-Ngando T. (2011). Functional effects of parasites on food web properties during the spring diatom bloom in lake pavin: A linear inverse modeling analysis. PloS One 6 (8), e23273. doi: 10.1371/journal.pone.0023273. Edited by J.A. Gilbert.
Gray A. D., Weinstein J. E. (2017). Size-and shape-dependent effects of microplastic particles on adult daggerblade grass shrimp (Palaemonetes pugio). Environ. Toxicol. Chem. 36 (11), 3074–3080. doi: 10.1002/etc.3881
Greenwood M. F. D. (2007). Nekton community change along estuarine salinity gradients: Can salinity zones be defined? Estuaries Coasts 30 (3), 537–542. doi: 10.1007/BF03036519
Gutiérrez M. H., Jara A. M., Pantoja S. (2016). Fungal parasites infect marine diatoms in the upwelling ecosystem of the Humboldt current system off central Chile: Fungal parasites on marine diatoms in upwelling ecosystems. Environ. Microbiol. 18 (5), 1646–1653. doi: 10.1111/1462-2920.13257
Gutiérrez R. A., Latasa M., Scharek R., Massana R., Vila G., Gasol J. M. (2011). Growth and grazing rate dynamics of major phytoplankton groups in an oligotrophic coastal site. Estuarine Coast. Shelf Sci. 95 (1), 77–87. doi: 10.1016/j.ecss.2011.08.008
Hansen J., Hildebrandt L., Zimmermann T., El Gareb F., Fischer E. K., Pröfrock D. (2023). Quantification and characterization of microplastics in surface water samples from the Northeast Atlantic Ocean using laser direct infrared imaging. Mar. Poll. Bull. 190, 114880. doi: 10.1016/j.marpolbul.2023.114880
Haraldsson M., Gerphagnon M., Bazin P., Colombet J., Tecchio S., Sime-Ngando T., et al. (2018). Microbial parasites make cyanobacteria blooms less of a trophic dead end than commonly assumed. ISME J. 12 (4), 1008–1020. doi: 10.1038/s41396-018-0045-9
Harding M. W., Marques L. L. R., Howard R. J., Olson M. E. (2009). Can filamentous fungi form biofilms? Trends Microbiol. 17 (11), 475–480. doi: 10.1016/j.tim.2009.08.007
Hartwich M., Martin-Creuzburg D., Wacker A. (2013). Seasonal changes in the accumulation of polyunsaturated fatty acids in zooplankton. J. Plankton Res. 35 (1), 121–134. doi: 10.1093/plankt/fbs078
Hassett B. T., Borrego E. J., Vonnahme T. R., Rämä T., Kolomiets M. V., Gradinger R. (2019). Arctic marine fungi: biomass, functional genes, and putative ecological roles. ISME J. 13 (6), 1484–1496. doi: 10.1038/s41396-019-0368-1
Hitchcock J. N. (2022). Microplastics can alter phytoplankton community composition. Sci. Total Environ. 819, 153074. doi: 10.1016/j.scitotenv.2022.153074
Hoellein T. J., McCormick A. R., Hittie J., London M. G., Scott J. W., Kelly J. J. (2017). Longitudinal patterns of microplastic concentration and bacterial assemblages in surface and benthic habitats of an urban river. Freshw Sci 36(3), 491–507. doi: 10.1086/693012
Huang Q., Lin Y., Zhong Q., Ma F., Zhang Y. (2020). The impact of microplastic particles on population dynamics of predator and prey: implication of the lotka-Volterra model. Sci. Rep. 10 (1), 4500. doi: 10.1038/s41598-020-61414-3
Hyde K. D., Jones E. B. G., Leaño E., Pointing S. B., Poonyth A. D., Vrijmoed L. L. P. (1998). Role of fungi in marine ecosystems. Biodivers. Conserv. 7 (9), 1147–1161. doi: 10.1023/A:1008823515157
Imhof H. K., Rusek J., Thiel M., Wolinska J., Laforsch C. (2017). Do microplastic particles affect Daphnia magna at the morphological, life history and molecular level? PloS One 12 (11), e0187590. doi: 10.1371/journal.pone.0187590
IUCN, International Union for Conservation of Nature (2021) Marine plastic pollution. Available at: http://www.iucn.org/theme/marine-and-polar/our-work/close-plastic-tap-programme/reports (Accessed 14/07/2023).
Jacquin J., Cheng J., Odobel C., Pandin C., Conan P., Pujo-Pay M., et al. (2019). Microbial ecotoxicology of marine plastic debris: A review on colonization and biodegradation by the “Plastisphere”. Front. Microbiol. 10. doi: 10.3389/fmicb.2019.00865
Jahnke A., Arp H. P. H., Escher B. I., Gewert B., Gorokhova E., Kühnel D., et al. (2017). Reducing uncertainty and confronting ignorance about the possible impacts of weathering plastic in the marine environment. Environ. Sci. Technol. Lett. 4 (3), 85–90. doi: 10.1021/acs.estlett.7b00008
Janusz G., Pawlik A., Sulej J., Świderska-Burek U., Jarosz-Wilkołazka A., Paszczyński A. (2017). Lignin degradation: microorganisms, enzymes involved, genomes analysis and evolution. FEMS Microbiol. Rev. 41 (6), 941–962. doi: 10.1093/femsre/fux049
Jemec A., Horvat P., Kunej U., Bele M., Kržan A. (2016). Uptake and effects of microplastic textile fibers on freshwater crustacean Daphnia magna. Environ. pollut. 219, 201–209. doi: 10.1016/j.envpol.2016.10.037
Jeyasanta K. I., Laju R. L., Patterson J., Jayanthi M., Bilgi D. S., Sathish N., et al. (2023). Microplastic pollution and its implicated risks in the estuarine environment of Tamil Nadu, India. Sci. Total Environ. 861, 160572. doi: 10.1016/j.scitotenv.2022.160572
Jobard M., Rasconi S., Sime-Ngando T. (2010). Diversity and functions of microscopic fungi: a missing component in pelagic food webs. Aquat. Sci. 72 (3), 255–268. doi: 10.1007/s00027-010-0133-z
Kagami M., Helmsing N. R., Van Donk E. (2011). Parasitic chytrids could promote copepod survival by mediating material transfer from inedible diatoms. Hydrobiologia 659 (1), 49–54. doi: 10.1007/s10750-010-0274-z
Kagami M., Miki T., Takimoto G. (2014). Mycoloop: chytrids in aquatic food webs. Front. Microbiol. 5. doi: 10.3389/fmicb.2014.00166
Kagami M., Motoki Y., Masclaux H., Bec A. (2017). Carbon and nutrients of indigestible pollen are transferred to zooplankton by chytrid fungi. Freshw. Biol. 62 (5), 954–964. doi: 10.1111/fwb.12916
Kanelli M., Vasilakos S., Nikolaivits E., Ladas S., Christakopoulos P., Topakas E. (2015). Surface modification of poly (ethylene terephthalate)(PET) fibers by a cutinase from Fusarium oxysporum. Process Biochem. 50 (11), 1885–1892. doi: 10.1016/j.procbio.2015.08.013
Kang H. M., Byeon E., Jeong H., Lee Y., Hwang U. K., Jeong C. B., et al. (2021). Arsenic exposure combined with nano-or microplastic induces different effects in the marine rotifer Brachionus plicatilis. Aquat. Toxicol. 233, 105772. doi: 10.1016/j.aquatox.2021.105772
Keeler E., Burgaud G., Teske A., Beaudoin D., Mehiri M., Dayras M., et al. (2021). Deep-sea hydrothermal vent sediments reveal diverse fungi with antibacterial activities. FEMS Microbiol. Ecol. 97 (8), fiab103. doi: 10.1093/femsec/fiab103
Kettner M. T., Rojas-Jimenez K., Oberbeckmann S., Labrenz M., Grossart H.-P. (2017). Microplastics alter composition of fungal communities in aquatic ecosystems: Fungal communities on microplastics. Environ. Microbiol. 19(11), 4447–4459. doi: 10.1111/1462-2920.13891
Kim S. H., Lee J. W., Kim J. S., Lee W., Park M. S., Lim Y. W. (2022). Plastic-inhabiting fungi in marine environments and PCL degradation activity. Antonie van Leeuwenhoek 115 (12), 1379–1392. doi: 10.1007/s10482-022-01782-0
Kirstein I. V., Kirmizi S., Wichels A., Garin-Fernandez A., Erler R., Löder M., et al. (2016). Dangerous hitchhikers? Evidence for potentially pathogenic Vibrio spp. on microplastic particles. Mar. Environ. Res. 120, 1–8. doi: 10.1016/j.marenvres.2016.07.004
Kües U. (2015). Fungal enzymes for environmental management. Curr. Opin. Biotechnol. 33, 268–278. doi: 10.1016/j.copbio.2015.03.006
Kumar R. (2003). Effects of mesocyclops thermocyclopoides (Copepoda: cyclopoida) predation on the population growth patterns of different prey species. J. Freshw. Ecol. 18 (3), 383–393. doi: 10.1080/02705060.2003.9663974
Kumari N., Samantaray B. P., Patel A., Kumar R. (2023). Microplastics Affect Rates of Locomotion and Reproduction via Dietary Uptake in Globally Invasive Snail Physa acuta. Water 15 (5), 928. doi: 10.3390/w15050928
Kvale K., Prowe A. E. F., Chien C.-T., Landolfi A., Oschlies A. (2020). The global biological microplastic particle sink. Sci. Rep. 10 (1), 16670. doi: 10.1038/s41598-020-72898-4
Lacerda A. L. D. F., Rodrigues L. D. S., Van Sebille E., Rodrigues F. L., Ribeiro L., Secchi E. R., et al. (2019). Plastics in sea surface waters around the Antarctic Peninsula. Sci. Rep. 9 (1), 3977. doi: 10.1038/s41598-019-40311-4
Law K. L., Thompson R. C. (2014). Microplastics in the seas. Science 345 (6193), 144–145. doi: 10.1126/science.1254065
Lebreton L., Slat B., Ferrari F., Sainte-Rose B., Aitken J., Marthouse R., et al. (2018). Evidence that the Great Pacific Garbage Patch is rapidly accumulating plastic. Sci. Rep. 8 (1), 4666. doi: 10.1038/s41598-018-22939-w
Lehtiniemi M., Hartikainen S., Näkki P., Engström-Öst J., Koistinen A., Setälä O. (2018). Size matters more than shape: Ingestion of primary and secondary microplastics by small predators. Food Webs 17, e00097. doi: 10.1016/j.fooweb.2018.e00097
Li J., Gao F., Zhang D., Cao W., Zhao C. (2022). Zonal distribution characteristics of microplastics in the southern Indian ocean and the influence of ocean current. JMSE 10 (2), 290. doi: 10.3390/jmse10020290
Li J., Liu H., Chen J.P. (2018). Microplastics in freshwater systems: A review on occurrence, environmental effects, and methods for microplastics detection. Water Res. 137, 362–374. doi: 10.1016/j.watres.2017.12.056
Li D., Liu K., Li C., Peng G., Andrady A. L., Wu T., et al. (2020). Profiling the vertical transport of microplastics in the west pacific ocean and the east Indian ocean with a novel in situ filtration technique. Environ. Sci. Technol. 54 (20), 12979–12988. doi: 10.1021/acs.est.0c02374
Li C., Wang L., Ji S., Chang M., Wang L., Gan Y., et al. (2021). The ecology of the plastisphere: microbial composition, function, assembly, and network in the freshwater and seawater ecosystems. Water Res. 202, 117428. doi: 10.1016/j.watres.2021.117428
Li C., Zhu L., Wang X., Liu K., Li D. (2022). Cross-oceanic distribution and origin of microplastics in the subsurface water of the South China Sea and Eastern Indian Ocean. Sci. Total Environ. 805, 150243. doi: 10.1016/j.scitotenv.2021.150243
Lin W., Jiang R., Xiong Y., Wu J., Xu J., Zheng J., et al. (2019). Quantification of the combined toxic effect of polychlorinated biphenyls and nano-sized polystyrene on Daphnia magna. J. Hazard. Mater. 364, 531–536. doi: 10.1016/j.jhazmat.2018.10.056
Long M., Moriceau B., Gallinari M., Lambert C., Huvet A., Raffray J., et al. (2015). Interactions between microplastics and phytoplankton aggregates: Impact on their respective fates. Mar. Chem. 175, 39–46. doi: 10.1016/j.marchem.2015.04.003
Lusher A. L., Tirelli V., O’Connor I., Officer R. (2015). Microplastics in Arctic polar waters: the first reported values of particles in surface and sub-surface samples. Sci. Rep. 5 (1), 14947. doi: 10.1038/srep14947
Lynch I., Dawson K. A. (2008). Protein-nanoparticle interactions. Nano Today 3, 40–47. doi: 10.1016/S1748-0132(08)70014-8
Macali A., Bergami E. (2020). Jellyfish as innovative bioindicator for plastic pollution. Ecol. Indic. 115, 106375. doi: 10.1016/j.ecolind.2020.106375
Mahjoub M.-S., Kumar R., Souissi S., Schmitt F. G., Hwang J.-S. (2012). Turbulence effects on the feeding dynamics in European sea bass (Dicentrarchus labrax) larvae. J. Exp. Mar. Biol. Ecol. 416–417. doi: 10.1016/j.jembe.2012.02.005
Martin-Creuzburg D., Von Elert E., Hoffmann K. H. (2008). Nutritional constraints at the cyanobacteria- Daphnia magna interface: The role of sterols. Limnol. Oceanogr. 53 (2), 456–468. doi: 10.4319/lo.2008.53.2.0456
Masclaux H., Perga M. E., Kagami M., Desvilettes C., Bourdier G., Bec A. (2013). How pollen organic matter enters freshwater food webs. Limnol Oceanogr 58 (4), 1185–1195. doi: 10.4319/lo.2013.58.4.1185
Mavrianos S., Manzi F., Agha R., Azoubib N., Schampera C., Wolinska J. (2023). Nanoplastics modulate the outcome of a zooplankton–microparasite interaction. Freshw. Bio. 68 (5), 847–859. doi: 10.1111/fwb.14068
Mei W., Chen G., Bao J., Song M., Li Y., Luo C. (2020). Interactions between MPs and organic compounds in aquatic environments: a mini review. Sci. Total Environ. 736, 139472. doi: 10.1016/j.scitotenv.2020.139472
Miao L., Li W., Adyel T. M., Yao Y., Deng Y., Wu J., et al. (2023). Spatio-temporal succession of microbial communities in plastisphere and their potentials for plastic degradation in freshwater ecosystems. Water Res. 229, 119406. doi: 10.1016/j.watres.2022.119406
Mohamed D. J., Martiny J. B. (2011). Patterns of fungal diversity and composition along a salinity gradient. ISME J. 5 (3), 379–388. doi: 10.1038/ismej.2010.137
Muhonja C. N., Makonde H., Magoma G., Imbuga M. (2018). Biodegradability of polyethylene by bacteria and fungi from Dandora dumpsite Nairobi-Kenya. PloS One 13 (7), e0198446. doi: 10.1371/journal.pone.0198446
Müller-Navarra D. C., Brett M. T., Liston A. M., Goldman C. R. (2000). A highly unsaturated fatty acid predicts carbon transfer between primary producers and consumers. Nat 403 (6765), 74–77. doi: 10.1038/47469
Oberbeckmann S., Labrenz M. (2020). Marine microbial assemblages on microplastics: diversity, adaptation, and role in degradation. Ann. Rev. Mar. Sci. 12, 209–232. doi: 10.1146/annurev-marine-010419-010633
Oberbeckmann S., Osborn A. M., Duhaime M. B. (2016). Microbes on a bottle: substrate, season and geography influence community composition of microbes colonizing marine plastic debris. PloS One 11 (8), e0159289. doi: 10.1371/journal.pone.0159289
Pan Z., Guo H., Chen H., Wang S., Sun X., Zou Q., et al. (2019). Microplastics in the Northwestern Pacific: Abundance, distribution, and characteristics. Sci. Total Environ. 650, 1913–1922. doi: 10.1016/j.scitotenv.2018.09.244
Pan Z., Liu Q., Sun X., Li W., Zou Q., Cai S., et al. (2022). Widespread occurrence of microplastic pollution in open sea surface waters: Evidence from the mid-North Pacific Ocean. Gondwana Res. 108, 31–40. doi: 10.1016/j.gr.2021.10.024
Parrish K., Fahrenfeld N. L. (2019). Microplastic biofilm in fresh-and wastewater as a function of microparticle type and size class. Environ. Sci. Water Res. Technol. 5, 495–505. doi: 10.1039/c8ew00712h
Perga M. E., Domaizon I., Guillard J., Hamelet V., Anneville O. (2013). Are cyanobacterial blooms trophic dead ends? Oecologia 172 (2), 551–562. doi: 10.1007/s00442-012-2519-1
Plastics Europe (2021) Plastics – the Facts 2021, An analysis of European plastics production, demand and waste data. Available at: https://plasticseurope.org/knowledge-hub/plastics-the-facts-2021/ (Accessed 28/07/2023).
Porter A., Lyons B. P., Galloway T. S., Lewis C. (2018). Role of marine snows in MPs fate and bioavailability. Environ. Sci. Tech. 52 (12), 7111–7119. doi: 10.1021/acs.est.8b01000
Procter J., Hopkins F. E., Fileman E. S., Lindeque P. K. (2019). Smells good enough to eat: Dimethyl sulfide (DMS) enhances copepod ingestion of MPs. Mar. pollut. Bull. 138, 1–6. doi: 10.1016/j.marpolbul.2018.11.014
Raghukumar C., Raghukumar S. (1998). Barotolerance of fungi isolated from deep-sea sediments of the Indian Ocean. Aquat. Microb. Ecol. 15 (2), 153–163. doi: 10.3354/ame015153
Ramaiah N. (2006). A review on fungal diseases of algae, marine fishes, shrimps and corals. Indian J. Mar. Sci. 35 (4), 380–387.
Ramírez-Camejo L. A., Zuluaga-Montero A., Lázaro-Escudero M., Hernández-Kendall V., Bayman P. (2012). Phylogeography of the cosmopolitan fungus Aspergillus flavus: is everything everywhere? Fungal Bio. 116 (3), 452–463. doi: 10.1016/j.funbio.2012.01.006
Rehse S., Kloas W., Zarfl C. (2018). Microplastics reduce short-term effects of environmental contaminants. Part I: effects of bisphenol A on freshwater zooplankton are lower in presence of polyamide particles. Int. J. Environ. Res. Public Health 15 (2), 280. doi: 10.3390/ijerph15020280
Reisser J., Shaw J., Hallegraeff G., Proietti M., Barnes D. K., Thums M., et al. (2014). Millimeter-sized marine plastics: a new pelagic habitat for microorganisms and invertebrates. PloS One 9 (6), e100289. doi: 10.1371/journal.pone.0100289
Richards T. A., Jones M. D. M., Leonard G., Bass D. (2012). Marine fungi: their ecology and molecular diversity. Ann. Rev. Mar. Sci. 4, 495–522. doi: 10.1146/annurev-marine-120710-100802
Richards T. A., Leonard G., Mahé F., Del Campo J., Romac S., Jones M. D., et al. (2015). Molecular diversity and distribution of marine fungi across 130 European environmental samples. Proc. R. Soc B 282 (1819), 20152243. doi: 10.1098/rspb.2015.2243
Rodrigues M. O., Abrantes N., Gonçalves F. J. M., Nogueira H., Marques J. C., Gonçalves A. M. M. (2019). Impacts of plastic products used in daily life on the environment and human health: What is known? Environ. Toxicol. Pharmacol. 72, 103239. doi: 10.1016/j.etap.2019.103239
Rojas-Jimenez K., Rieck A., Wurzbacher C., Jürgens K., Labrenz M., Grossart H. P. (2019). A salinity threshold separating fungal communities in the Baltic Sea. Front. Microbiol. 10. doi: 10.3389/fmicb.2019.00680
Rollwagen-Bollens G., Bollens S. M., Gonzalez A., Zimmerman J., Lee T., Emerson J. (2013). Feeding dynamics of the copepod Diacyclops thomasi before, during and following filamentous cyanobacteria blooms in a large, shallow temperate lake. Hydrobiologia 705, 101–118. doi: 10.1007/s10750-012-1385-5
Rosato A., Barone M., Negroni A., Brigidi P., Fava F., Xu P., et al. (2020). Microbial colonization of different MPs types and biotransformation of sorbed PCBs by a marine anaerobic bacterial community. Sci. Total Environ. 705, 135790. doi: 10.1016/j.scitotenv.2019.135790
Rothschild B. J., Osborn T. R. (1988). Small-scale turbulence and plankton contact rates. J. plankton Res. 10 (3), 465–474. doi: 10.1093/plankt/10.3.465
Rudolf V. H., Rasmussen N. L. (2013). Population structure determines functional differences among species and ecosystem processes. Nat. Commun. 4 (1), 2318. doi: 10.1038/ncomms3318
Ryan P. G. (2015). A brief history of marine litter research. Mar. anthropogenic litter 78, 1–25. doi: 10.1007/978-3-319-16510-3_1
Ryan P. G. (2016). Ingestion of plastics by marine organisms. Hazard. chem. associated plastics Mar. Environ. 78, 235–266. doi: 10.1007/698_2016_21
Schampera C., Wolinska J., Bachelier J. B., de Souza MaChado A. A., Rosal R., González-Pleiter M., et al. (2021). Exposure to nanoplastics affects the outcome of infectious disease in phytoplankton. Environ. pollut. 277, 116781. doi: 10.1016/j.envpol.2021.116781
Seena S., Graça D., Bartels A., Cornut J. (2019). Does nanosized plastic affect aquatic fungal litter decomposition? Fungal Ecol. 39, 388–392. doi: 10.1016/j.funeco.2019.02.011
Seki H., Fulton J. (1969). Infection of marine copepods by Metschnikowia sp. Mycopathol. mycol. applicata 38 (1-2), 61–70. doi: 10.1007/bf02051676
Shen M., Song B., Zhou C., Hu T., Zeng G., Zhang Y. (2022). Advanced oxidation processes for the elimination of microplastics from aqueous systems: Assessment of efficiency, perspectives and limitations. Sci. Total Environ. 842, 156723. doi: 10.1016/j.scitotenv.2022.156723
Sheridan E. A., Fonvielle J. A., Cottingham S., Zhang Y., Dittmar T., Aldridge D. C., et al. (2022). Plastic pollution fosters more microbial growth in lakes than natural organic matter. Nat. Commun. 13 (1), 4175. doi: 10.1038/s41467-022-31691-9
Solomando A., Capó X., Alomar C., Álvarez E., Compa M., Valencia J. M., et al. (2020). Long-term exposure to microplastics induces oxidative stress and a pro-inflammatory response in the gut of Sparus aurata Linnaeu. Environ. pollut. 266, p.115295. doi: 10.1016/j.envpol.2020.115295
Sooriyakumar P., Bolan N., Kumar M., Singh L., Yu Y., Li Y., et al. (2022). Biofilm formation and its implications on the properties and fate of microplastics in aquatic environments: a review. J. Hazard. Mater. Adv. 6, 100077. doi: 10.1016/j.hazadv.2022.100077
Stenger K. S., Wikmark O. G., Bezuidenhout C. C., Molale-Tom L. G. (2021). Microplastics pollution in the ocean: Potential carrier of resistant bacteria and resistance genes. Environ. pollut. 291, 118130. doi: 10.1016/j.envpol.2021.118130
Suaria G., Perold V., Lee J. R., Lebouard F., Aliani S., Ryan P. G. (2020). Floating macro-and microplastics around the Southern Ocean: Results from the Antarctic Circumnavigation Expedition. Environ. Inter. 136, 105494. doi: 10.1016/j.envint.2020.105494
Suhrhoff T. J., Scholz-Böttcher B. M. (2016). Qualitative impact of salinity, UV radiation and turbulence on leaching of organic plastic additives from four common plastics—A lab experiment. Mar. pollut. Bull. 102 (1), 84–94. doi: 10.1016/j.marpolbul.2015.11.054
Sun J. Z., Liu X. Z., McKenzie E. H., Jeewon R., Liu J. K., Zhang X. L., et al. (2019). Fungicolous fungi: terminology, diversity, distribution, evolution, and species checklist. Fungal Divers. 95, 337–430. doi: 10.1007/s13225-019-00422-9
Sun Y., Qian Y., Geng S., Wang P., Zhang L., Yang Z. (2023). Joint effects of microplastics and ZnO nanoparticles on the life history parameters of rotifers and the ability of rotifers to eliminate harmful phaeocystis. Chemosphere 310, 136939. doi: 10.1016/j.chemosphere.2022.136939
Tagorti G., Kaya B. (2022). Genotoxic effect of microplastics and COVID-19: The hidden threat. Chemosphere 286, 131898. doi: 10.1016/j.chemosphere.2021.131898
Tisthammer K. H., Cobian G. M., Amend A. S. (2016). Global biogeography of marine fungi is shaped by the environment. Fungal Ecol. 19, 39–46. doi: 10.1016/j.funeco.2015.09.003
Tiwari M., Rathod T. D., Ajmal P. Y., Bhangare R. C., Sahu S. K. (2019). Distribution and characterization of microplastics in beach sand from three different Indian coastal environments. Mar. Poll. Bull. 140, 262–273. doi: 10.1016/j.marpolbul.2019.01.055
Tseng L. C., Kumar R., Chen Q. C., Hwang J. S. (2011). Summer distribution of Noctiluca scintillans and mesozooplankton in the Western and Southern East China Sea prior to the Three Gorges Dam operation. Hydrobiologia 666, 239–256. doi: 10.1007/s10750-010-0587-y
Turner J. T. (2015). Zooplankton fecal pellets, marine snow, phytodetritus and the ocean’s biological pump. Prog. Oceanogr. 130, 205–248. doi: 10.1016/j.pocean.2014.08.005
Valentine R. C., Valentine D. L. (2004). Omega-3 fatty acids in cellular membranes: a unified concept. Prog. Lipid Res. 43 (5), 383–402. doi: 10.1016/j.plipres.2004.05.004
Valiente-Banuet A., Aizen M. A., Alcántara J. M., Arroyo J., Cocucci A., Galetti M., et al. (2015). Beyond species loss: the extinction of ecological interactions in a changing world. Funct. Ecol. 29 (3), 299–307. doi: 10.1111/1365-2435.12356. Edited by M. Johnson.
Van Cauwenberghe L., Vanreusel A., Mees J., Janssen C. R. (2013). Microplastic pollution in deep-sea sediments. Environ. Poll. 182, 495–499. doi: 10.1016/j.envpol.2013.08.013
Van Der Jagt H., Wiedmann I., Hildebrandt N., Niehoff B., Iversen M. H. (2020). Aggregate feeding by the copepods calanus and pseudocalanus controls carbon flux attenuation in the arctic shelf sea during the productive period. Front. Mar. Sci. 7. doi: 10.3389/fmars.2020.543124
Viršek M. K., Lovšin M. N., Koren Š., Kržan A., Peterlin M. (2017). Microplastics as a vector for the transport of the bacterial fish pathogen species Aeromonas salmonicida. Mar. Poll. Bull. 125 (1–2), 301–309. doi: 10.1016/j.marpolbul.2017.08.024
Vroom R. J. E., Koelmans A. A., Besseling E., Halsband C. (2017). Aging of microplastics promotes their ingestion by marine zooplankton. Environ. Poll. 231, 987–996. doi: 10.1016/j.envpol.2017.08.088
Wan J.-K., Chu W.-L., Kok Y.-Y., Lee C.-S. (2018). “Distribution of Microplastics and Nanoplastics in Aquatic Ecosystems and Their Impacts on Aquatic Organisms, with Emphasis on Microalgae,” in Reviews of Environmental Contamination and Toxicology, vol. 246 . Ed. De Voogt P. (Cham, Switzerland: Springer International Publishing), 133–158. Reviews of Environmental Contamination and Toxicology. doi: 10.1007/398_2018_14
Webb J. S., Nixon M., Eastwood I. M., Greenhalgh M., Robson G. D., Handley P. S. (2000). Fungal colonization and biodeterioration of plasticized polyvinyl chloride. App. Environ. Microbiol. 66 (8), 3194–3200. doi: 10.1038/s41570-020-0163-6
Worden A. Z., Follows M. J., Giovannoni S. J., Wilken S., Zimmerman A. E., Keeling P. J. (2015). Rethinking the marine carbon cycle: Factoring in the multifarious lifestyles of microbes. Science 347 (6223), 1257594. doi: 10.1126/science.1257594
Wright S. L., Thompson R. C., Galloway T. S. (2013). The physical impacts of microplastics on marine organisms: a review. Environ. pollut. 178, 483–492. doi: 10.1016/j.envpol.2013.02.031
Wurzbacher C., Kerr J., Grossart H.-P. (2011). Aquatic fungi. In The Dynamical Processes of Biodiversity - Case Studies of Evolution and Spatial Distribution. Eds. Grillo O., Venora G. (London, United Kingdom: InTech), 227–258. Available at: http://www.intechopen.com/books/the-dynamical-processes-of-biodiversity-case-studies-of-evolution-and-spatialdistribution/aquatic-fungi.
Xue Y. H., Sun Z. X., Feng L. S., Jin T., Xing J. C., Wen X. L. (2021). Algal density affects the influences of polyethylene microplastics on the freshwater rotifer Brachionus calyciflorus. Chemosphere 270, 128613. doi: 10.1016/j.chemosphere.2020.128613
Yamada-Onodera K., Mukumoto H., Katsuyaya Y., Saiganji A., Tani Y. (2001). Degradation of polyethylene by a fungus, Penicillium simplicissimum YK. Polym. Degrad. Stab. 72 (2), 323–327. doi: 10.1016/S0141-3910(01)00027-1
Zahra S., Abbas S. S., Mahsa M. T., Mohsen N. (2010). Biodegradation of low-density polyethylene (LDPE) by isolated fungi in solid waste medium. Waste Manage. 30 (3), 396–401. doi: 10.1016/j.wasman.2009.09.027
Zettler E. R., Mincer T. J., Amaral-Zettler L. A. (2013). Life in the “Plastisphere”: microbial communities on plastic marine debris. Environ. Sci. Technol. 47 (13), 7137–7146. doi: 10.1021/es401288x
Zhang Y., Gao T., Kang S., Allen S., Luo X., Allen D. (2021). Microplastics in glaciers of the Tibetan Plateau: Evidence for the long-range transport of microplastics. Sci. Total Environ. 758, 143634. doi: 10.1016/j.scitotenv.2020.143634
Zhang P., Yan Z., Lu G., Ji Y. (2019). Single and combined effects of microplastics and roxithromycin on Daphnia magna. Environ. Sci. Pollut. Res. 26, 17010–17020. doi: doi :10.1007/s11356-019-05031-2
Keywords: Chytridiomycota, ecological interactions, ecosystem functioning, microplastics, mycoloop
Citation: Yadav DK and Kumar R (2023) Microplastic effects in aquatic ecosystems with special reference to fungi–zooplankton interaction: identification of knowledge gaps and prioritization of research needs. Front. Ecol. Evol. 11:1279589. doi: 10.3389/fevo.2023.1279589
Received: 18 August 2023; Accepted: 09 November 2023;
Published: 12 December 2023.
Edited by:
Yixin Zhang, Soochow University, ChinaReviewed by:
Andrea Augusto Sfriso, University of Ferrara, ItalyCopyright © 2023 Yadav and Kumar. This is an open-access article distributed under the terms of the Creative Commons Attribution License (CC BY). The use, distribution or reproduction in other forums is permitted, provided the original author(s) and the copyright owner(s) are credited and that the original publication in this journal is cited, in accordance with accepted academic practice. No use, distribution or reproduction is permitted which does not comply with these terms.
*Correspondence: Ram Kumar, cmFta3VtYXJAY3ViLmFjLmlu
Disclaimer: All claims expressed in this article are solely those of the authors and do not necessarily represent those of their affiliated organizations, or those of the publisher, the editors and the reviewers. Any product that may be evaluated in this article or claim that may be made by its manufacturer is not guaranteed or endorsed by the publisher.
Research integrity at Frontiers
Learn more about the work of our research integrity team to safeguard the quality of each article we publish.