- 1Maine Cooperative Fish and Wildlife Research Unit, U.S. Geological Survey, University of Maine, Orono, ME, United States
- 2Department of Wildlife, Fisheries, and Conservation Biology, University of Maine, Orono, ME, United States
- 3School of Earth and Climate Sciences, University of Maine, Orono, ME, United States
- 4Biology Department, State University of New York, College at Oneonta, Oneonta, NY, United States
- 5Department of Environmental Science and Policy, University of Southern Maine, Gorham, ME, United States
- 6Maine Sea Grant College Program, School of Marine Sciences, University of Maine, Orono, ME, United States
More than a century of impoundments in the Penobscot River, Maine, USA, has contributed to population declines in migratory fish in the system. A decade of change, research, and monitoring has revealed direct and indirect ways that dams have influenced the river habitat, connectivity for migratory fish, and the food web. The removal of two main-stem dams (in 2012 and 2013) and bolstering of fish passage have been part of coordinated restoration efforts in the watershed. Integral to this undertaking was support for short- and long-term monitoring and research that included physical habitat, fish passage, and broad scale ecological assessments. Herein we discuss the seven interconnected and complex ways that dams have affected the Penobscot River ecosystem, particularly for migratory fish. These include familiar influences ascribed to dams: i) impaired access to habitat, ii) injury and mortality, and iii) delays of migration. Other ecological influences are less studied and more subtle: iv) facilitation of predation, v) community shifts, and vi) demographic shifts. Lastly, dams result in vii) a loss of ecosystem services that would otherwise be intact in an unimpounded system. We draw on both direct examples from the Penobscot River and broader information to characterize how impoundments have transformed this ecosystem for more than a century. Recent dam removals and mitigation efforts have reestablished some of these ecological functions.
Introduction
Human cultures are inextricably linked to river systems and people have shaped landscapes worldwide through damming. In the United States, there are more than 91,000 documented dams that serve significant functions for communities, including sources of water, navigation, and power generation (Roy et al., 2018). In the State of Maine alone, there are nearly 600 active dams (USACE, 2023) and many other uncatalogued impoundments. Biophysical processes have long been recognized to be fundamentally affected by dams and the impoundments they create. Rivers flows, thermal regimes, and sediment transport may all be affected (Poff et al., 1997; Petts et al., 2006). These endogenous factors influence river channel conditions that govern precipitation runoff and routing within the watershed’s hydrologic system in a manner that systematically modifies the river hydrograph. While evidence of changes to river flows caused by climate change has also been documented in Maine (Dudley and Hodgkins, 2002; Gerard, 2018), large scale land cover changes (from tree clearing, road construction, and dam construction) have been the most pervasive disturbances in the Penobscot River watershed over the past two centuries (Opperman et al., 2011).
The ecological result of dam-related perturbations has both human costs and ecological ramifications. The decisions made in management of coastal river systems result in socioeconomic tradeoffs that directly affect fish populations (Roy et al., 2018) and have a long and well-documented history of being in direct conflict with the livelihoods and life-ways of Indigenous Peoples, especially in New England (Bennett, 2017). While dams provide societal functions to meet human needs, their operation often conflicts with migratory fish conservation goals (Song et al., 2019). Many migratory species’ populations have declined due to dams (together with habitat destruction, overexploitation, and climate change; Wilcove, 2010) and now persist at greatly diminished levels (Greene et al., 2009; Limburg and Waldman, 2009; Waldman and Quinn, 2022). Mitigative steps such as operational guidance or fish passage may be implemented through the Federal Energy Regulatory Commission (FERC) in the United States but these multi-decade licenses may constrain both industrial and conservation potential (Vogel and Jansujwicz, 2022).
Conservation actions in the Penobscot River
Lessons learned after dam removals have been critical in understanding the subtle and synergistic ecological influences of damming. Those lessons complement a wealth of literature that is unequivocal as to the effects of dams. Dam removals may have immediate effects on river ecosystems (e.g., Catalano et al., 2007; Burroughs et al., 2010; Hitt et al., 2012; Poulos et al., 2014). These effects have been demonstrated in a tributary of the Penobscot River watershed (Gardner et al., 2013; Hogg et al., 2015) but recent changes to the main-stem of the River after dam removal have been extraordinary in scope. This river has been the focus of restoration efforts over the last several decades making its study a significant contributor to the advancement of river restoration ecology.
Central to the ancestral and current homelands of the Penobscot Nation, the Penobscot River is the second largest watershed in the New England states of the northeast USA, and the largest entirely within the State of Maine (approximately 22,300 km2). The river has five major tributaries, hundreds of smaller streams, and its basin includes approximately 330 km2 of lakes and ponds. This diversity in physical habitat continues to support runs of the full assemblage of native sea-run fish populations. Atlantic and shortnose sturgeons (Acipenser oxyrinchus and A. brevirostrum), striped bass (Morone saxatilis), rainbow smelt (Osmerus mordax), and tomcod (Microgadus tomcod) migrations are generally in the main-stem and estuary. Other sea-run species have longer migrations that often necessitate upstream and downstream passage at existing dams (Saunders et al., 2006). River herring (alewife, Alosa pseudoharengus and blueback herring, A. aestivalis), American eel (Anguilla rostrata), American shad (Alosa sapidissima), Atlantic salmon (Salmo salar), and sea lamprey (Petromyzon marinus) all have large amounts of required habitat located upstream of current dams (Trinko Lake et al., 2012). For many of these species, historic estimates (based in part on commercial catch data dating back to the 1800s) range into the millions of individuals per year with unknown levels prior to colonization. For alosine fishes, historic populations are estimated to be at least two orders of magnitude greater than they are today (Hall et al., 2011). The construction of main-stem dams initiated in the 1800s limited the upstream extent of migration (Saunders et al., 2006) and notably impacted harvest (Foster and Atkins, 1867).
Fisheries restoration efforts in the Penobscot River, which began in the mid-1800s (Moring et al., 1995), initially concentrated on the Atlantic Salmon, a culturally and economically iconic species (Schmitt, 2016). Despite the precarious status of this and many other diadromous species, present numbers of salmon in the Penobscot River are large relative to other northeastern USA rivers. In addition, relative to other large northeastern rivers, the Penobscot River watershed has less urban development and relatively fewer dams (Opperman et al., 2011). As a result, this river represents, and has represented for decades, a high priority for restoration of diadromous fish and associated ecological processes (e.g., Everhart and Cutting, 1968; Martin and Apse, 2011).
The Penobscot River Restoration Project
To resolve longstanding conflict over the licensing of hydropower operations on the Penobscot River, a multiparty settlement agreement was signed in June 2004 (Opperman et al., 2011). Parties included dam owners, federal and State of Maine partners, the Penobscot Indian Nation, several non-governmental organizations, and the Penobscot River Restoration Trust (PRRT; a non-profit organization established to implement the restoration project). The agreement filed with the FERC, outlined a plan (the Penobscot River Restoration Project; PRRP) to restore native sea-run fish through the i) purchase and removal of the two most seaward dams (Veazie and Great Works); ii) purchase, decommissioning, and construction of a nature-like bypass channel around a third dam (Howland); iii) maintenance of current energy generation through increased power generation at six existing dams (Gilman Fall, Stillwater, Orono, Weldon, West Enfield, and Milford); and iv) efforts to improve fish passage at four dams (Figure 1).
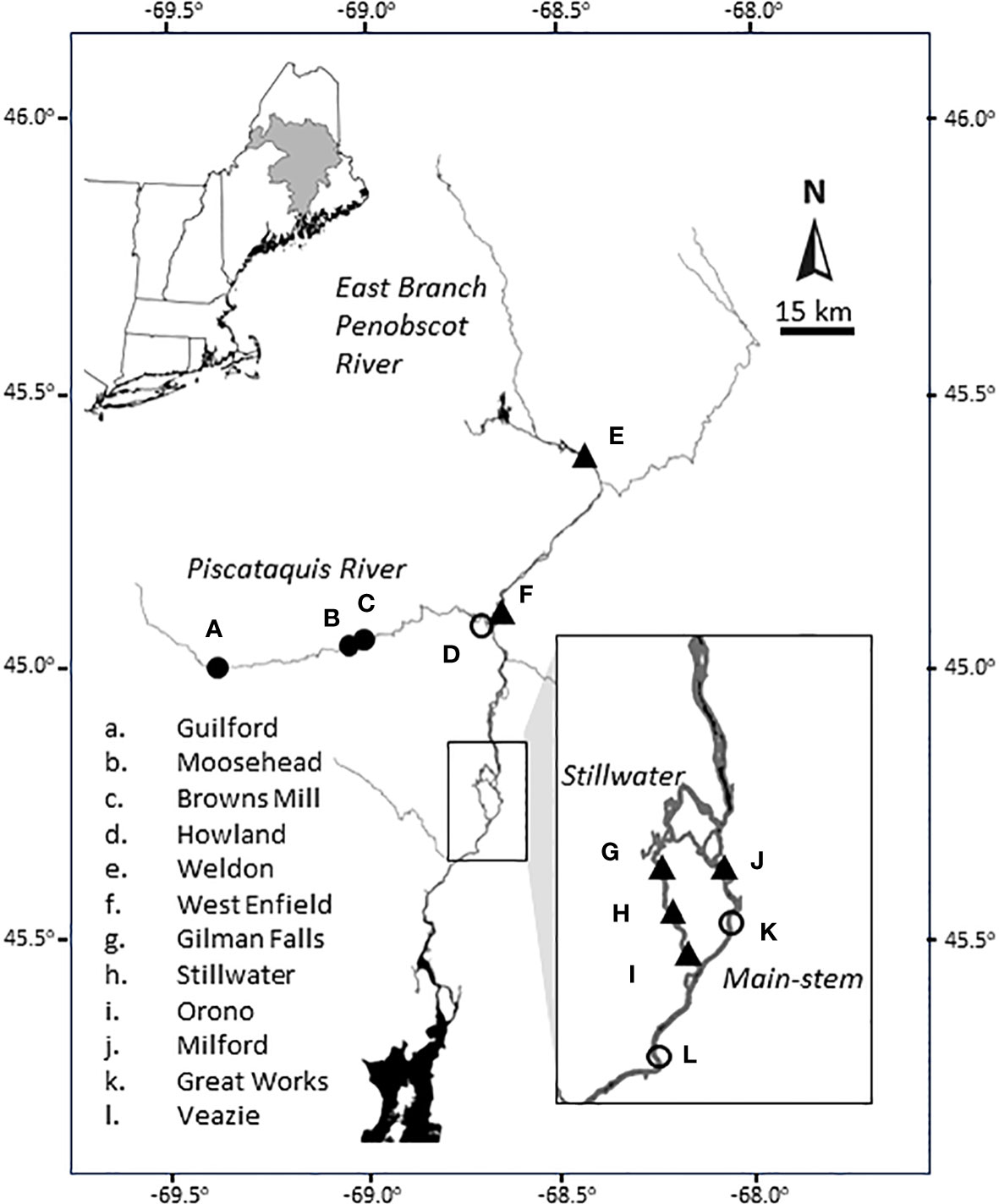
Figure 1 Map of the Penobscot River, Maine, USA with main-stem dams (others omitted for clarity). Upper left insert shows the New England region of the USA with the shaded area indicating the Penobscot River watershed for reference. Actions of the Penobscot River Restoration Project (PRRP) include the removal of the two most seaward dams (Veazie and Great Works) and decommissioning and construction of a nature-like fish way at a third dam (Howland) indicated by open circles. Energy generation (or water ponding) was increased at six existing dams (Weldon, West Enfield, Milford, Gilman Falls, Stillwater and Orono) shown by filled triangles. Dams not included in the PRRP are indicated by filled circles. Dam names are indicted by lower case letters “a” through “l”.
In 2012, the Great Works Dam was removed, followed by the Veazie Dam in 2013. Howland Dam was not removed but was instead decommissioned and a nature-like fish bypass built in 2016. Milford Dam (as of 2013 was the lowermost dam on the river) received a new fish lift to aid in fish passage, as well as two new turbines to offset energy production losses at other dams. We note that the PRRP resulted in minimal upstream passage at the Stillwater Branch (Figure 1), depending on a small fish lift and active trucking (Opperman et al., 2011).
While the PPRP has improved connectivity in the Penobscot River watershed, the physical influence of the dam removals is localized to the Veazie and Great Works Dam remnants, roughly 15 km of main-stem river access. It is important to note that while the project is often described as having “Opened up 2,000 miles of rivers and streams to sea-run fish” (NRCM, 2023), the more precise description frames it as “improved access” to 2,000 miles of habitat (or to 500 miles, as reported by Day, 2006) through both dam removals and efforts to improve fish passage. Recognizing a lack of monitoring associated with other dam removals, and congruent with calls for assessment (e.g., Hart et al., 2002), the PRRT began discussions about science and monitoring as early as 2004. This work critically informed the funding and course of research efforts, the results of which provide a unique opportunity to assess the effects of both dam removals as well as the persistent influences of those dams that remain.
Seven influences of dams on migratory fish
Herein we describe seven influences of dams on migratory fish and their ecosystems. The ecological outcomes we identify from dams and their impoundments are linked to one another, thereby producing a suite of effects that are synergistic in nature. Decades of study in the Penobscot River, and subsequent restorative actions through dam removal or mitigation, have helped to characterize both the obvious and subtle ways that dams influence the ecology of migratory fishes (Figure 2). We draw on specific examples of migratory fish in the Penobscot River, bolstered by literature, to identify the suite of ecological outcomes associated with the construction of dams in a riverine system: i) impaired access to habitat, ii) injury and mortality, iii) delay of migration, iv) facilitation of predation, v) community shifts, vi) demographic shifts, and vii) loss of ecosystem services.
We attempt to highlight the complexity and interconnected nature of these ecological influences as they present a critical challenge for managers and dam operators who wish to minimize and mitigate the influences of these structures. We would be remiss if we did not acknowledge that undammed rivers are neither homogenous nor universally passable to all fish. Migratory fish interact with many natural features in rivers that are partial or complete barriers to movement. These features (e.g., rapids, waterfalls, or natural lakes) may impose some (or all) of the influences we ascribe to anthropogenic structures. However, there are two fundamental distinctions between the influences of natural impediments and human created dams. Firstly, the construction of dams in North America has occurred in the last several hundred years, exerting selective pressures over abbreviated evolutionary time scales (Zarri et al., 2022). Secondly, the abundance of human-built structures on many coastal rivers is far greater than patterns of natural fragmentation in rivers (Freeman et al., 2003).
First: impaired access to habitat
Perhaps the most obvious effect of dams follows directly from their function of storing and controlling water. Dams divide free-flowing, continuous habitats into distinct, discontinuous fragments and create impounded waters. Riverine ecosystems rely upon basin-scale storage and transport of resources (Vannote et al., 1980) and the proliferation of damming has disrupted these processes by altering flow regimes and restricting the movement of aquatic fauna (Ward and Stanford, 1987). Habitat fragmentation may lead to local extirpation (Kiffney et al., 2009; Carvajal-Quintero et al., 2017), population declines (Limburg and Waldman, 2009), or extinction (Ricciardi and Rasmussen, 1999; Carvajal-Quintero et al., 2017).
Many of the Penobscot River’s migratory species are among those most vulnerable to the effects of damming globally, including alosines, lampreys, and eels (Liermann et al., 2012). Dams are migration barriers that exclude these species from important upstream habitats and caused some populations (e.g., Atlantic salmon, and American shad) to decline into single-digit abundances (Opperman et al., 2011; DMR, 2022). The depletion of Maine’s diadromous community mirrors the trend for these fishes across North America and globally (Waldman and Quinn, 2022). Three Penobscot River species have garnered federal protection (Atlantic sturgeon, shortnose sturgeon, and Atlantic salmon) under the Endangered Species Act (USFWS, 1967; NMFS, 2010; USFWS and NMFS, 2018) with rainbow smelt having been listed as a federal Species of Concern (Enterline et al., 2012). Dams are cited as a primary threat to these protected species within the Penobscot River (Atlantic and shortnose sturgeons; Fernandes et al., 2010; rainbow smelt, Enterline et al., 2012; Atlantic salmon, Rubenstein et al., 2023).
The removals of main-stem dams as part of the PRRP has revealed the direct relation between dams and restricted access to habitat. The PRRP and associated efforts have dramatically increased the abundance of diadromous fishes within the Penobscot River (Scherelis et al., 2020; DMR, 2022; Whittum et al., 2023). Before dam removal, the American shad population was of unknown size and limited to habitat downstream of Veazie Dam. Only 16 adults had passed through the fishway from 1978 to 2012 (Grote et al., 2014a; Grote et al., 2014b). Annual counts at Milford Dam have now surpassed 10,000 (in 2022) and the population has supported a growing recreational fishery (DMR, 2022). From a combination of passage improvements and adult stocking into spawning lakes, the 2023 river herring run has approached 6 million individuals, increased from tens of thousands of fish before dam removals (DMR, 2022; Figure 3). Shortnose sturgeon have been tracked moving upstream of the former Veazie Dam (Johnston et al., 2019) and several have been encountered at the Milford Dam fish lift. Atlantic salmon permeability through the region with the two dam remnants was greatly improved from critically poor passage (Holbrook et al., 2009) to that of an open river (Izzo et al., 2016). At a smaller scale, dam removal on a tributary of the Penobscot River, the Sedgeunkedunk Stream led to a rapid recolonization (within one year) by sea lamprey (Hogg et al., 2013) and other migratory fish (Hogg et al., 2015).
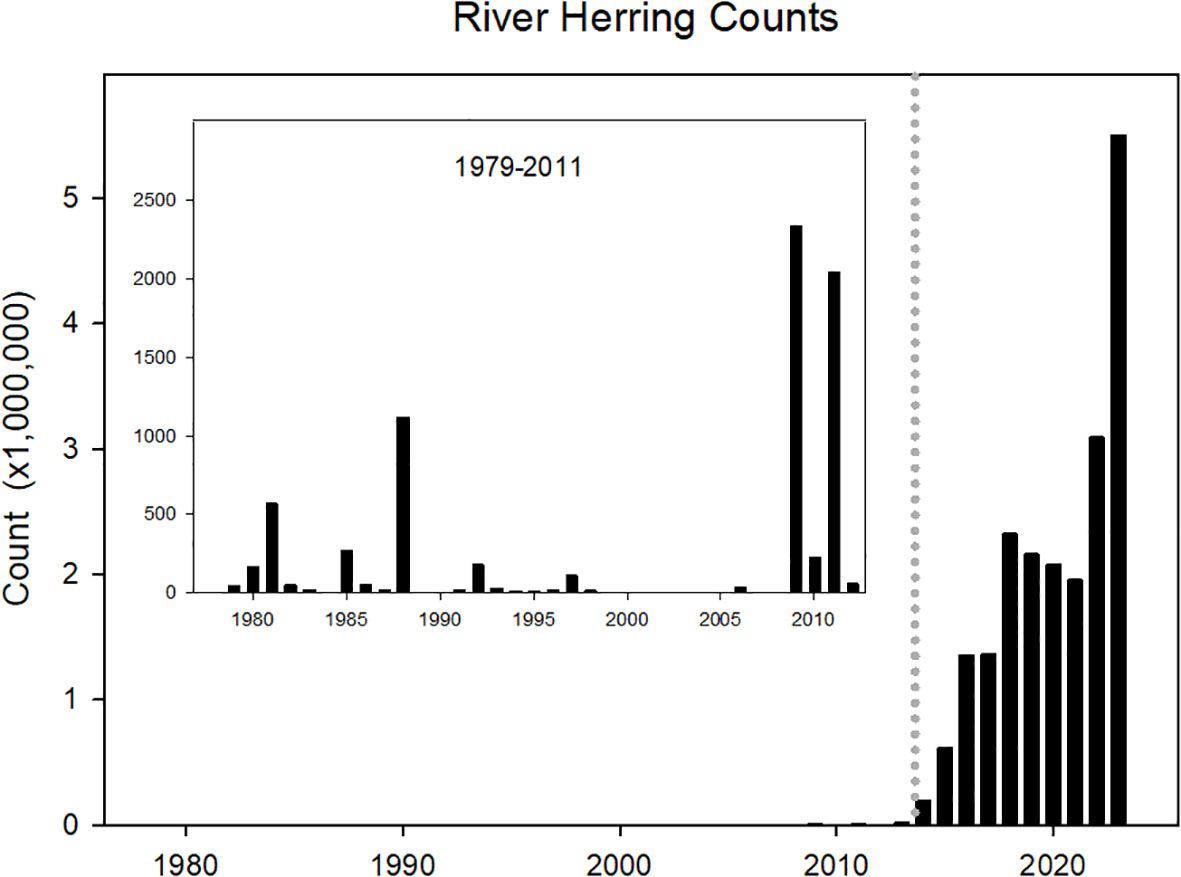
Figure 3 Estimated returns of river herring (Alosa pseudoharengus and A. aestivalis) to the main-stem of the Penobscot River, Maine, USA at Veazie Dam from 1979 to 2013, and aggregate at Milford Dam and Orono Dam thereafter post dam removal (indicated with vertical dotted grey line). The data show a rapid increase in river herring coincident with dam removal and coordinated upstream stocking (DMR, 2022).
However, many species have not recovered to target abundances (Opperman et al., 2011). Penobscot River runs of Atlantic salmon remain low (only 589 individuals were counted in 2021). River herring runs, although greatly improved, are likely still an order of magnitude less than runs pre-1600s (e.g., Hall et al., 2012). Despite dam removals, the Penobscot River remains a heavily impounded system. The two main-stem dam removals, while significant, had the limited influence of opening 15 km of river. Aspirational projections for Atlantic salmon (12,000), and American shad (2 million) are fully dependent on restoring connectivity between the ocean and important habitats in northern headwaters (Opperman et al., 2011). Fishways are often used to allow access to habitat that is otherwise constrained (Waldman and Quinn, 2022), and this is the case in the Penobscot River.
Most hydropower dams in the Penobscot River now have at least one form of fish passage that serves the general fish community, and several have also installed eel-specific bypasses (Opperman et al., 2011; Mensinger et al., 2021a; Molina-Moctezuma et al., 2021; Peterson, 2022). Overall upstream passage for adult Atlantic salmon at Milford Dam (now the lowermost dam), was relatively high (92%) over a span of six years (Izzo et al., 2016; Peterson, 2022), though with significant passage delays (days to weeks). Surviving downstream passage remains challenging for migrating juvenile Atlantic salmon (smolts) at Milford and other dams (Holbrook et al., 2011; Stich et al., 2014; Stich et al., 2015a). Downstream passage (for both juveniles and adults) is demonstrably critical for population persistence (e.g., American shad; Stich et al., 2019). In general, however, adult and non-salmonid juvenile downstream passage performance remains poorly characterized making such assessments important directions of future study.
Overall, however, fishway mediated access to upstream habitat falls far short of the capacity of unimpeded river systems (Zydlewski et al., 2021). Most fishways fail to effectively restore connectivity for all native species (Bunt et al., 2012; Noonan et al., 2012; Algera et al., 2020; Hershey, 2021). It is also common for fishways to be designed and evaluated for passage of salmonids (Noonan et al., 2012) and salmonids typically have the highest passage success through these structures (Noonan et al., 2012; Hershey, 2021). Importantly, migratory fish populations passing dams incur mortality, injury, and delay (Roscoe et al., 2011). Even the nature-like fishway at Howland Dam, while providing greatly improved passage (compared to when it was a functioning hydropower facility) remains distinguishable from free-flowing river reaches in terms of both passage delays and survival of Atlantic salmon smolts (Molina-Moctezuma et al., 2021).
Second: injury and mortality
Dams prevent access of some migrants to upstream habitat, but those that attempt to reach that habitat may face risk of injury or mortality. Upstream migrants must navigate fishways (or other paths) to move upstream. Passage attempts may lead to sub-lethal injuries (Castro-Santos et al., 2009) or mortality (Roscoe et al., 2011) due to engineered conditions or operational failures. While the run of American shad remains low in the Penobscot River, dozens to hundreds of dead adult American shad are removed from the fishway structure annually. Similar incidental losses for river herring and other migrants occur (Jason Valliere, Maine Department of Marine Resources, personal communication, August 31, 2023). Delays in passage may increase injury risk (McLaughlin et al., 2013) however it is difficult to assess what occurs within the fishway versus during searches for the fishway entrance.
Because dams are not freely permeable to movements in both directions, fish passage risks may be compounded by extensive searching periods. Migrating Atlantic salmon adults (Izzo et al., 2016; Maynard et al., 2017) and American shad (Grote et al., 2014b; Peterson, 2022) in the Penobscot River make wide-ranging upstream and downstream directional movements. Alewife are also known to “oscillate” in this way in other systems (McCartin et al., 2019). Such behaviors may put an individual at a great disadvantage even after successful upstream dam passage. These fish may suffer high mortality while moving back downstream (Castro-Santos and Letcher, 2010) being caught in an ecological trap that is confounded by the energetic cost of delay (Rubenstein et al., 2023). For iteroparous species, whether searching or not, fish that make it successfully past a dam must survive at least one downstream passage event.
Atlantic salmon are known to experience high mortality after completing spawning, but if successful in navigating dams moving downstream, they return to the sea (Maynard et al., 2018) either in the fall or following spring (Ruggles, 1980; Maynard et al., 2017). The population cost of losing these larger, multi-year fish may be significant due to their increased reproductive potential. Female Atlantic salmon may invest up to 25% of their body mass into egg production (Fleming, 1996). The loss of “big old fat female fish” may have significant population effects (sensu Hixon et al., 2014). Though Atlantic salmon restoration has focused on upstream passage more than multiyear spawning (USFWS and NMFS, 2018), the biological importance of these fish is clear (Fleming, 1996; Hixon et al., 2014).
Recruitment of juvenile anadromous fish spawned upstream of dams is entirely dependent upon the successful emigration downstream. Downstream migrating Atlantic salmon smolts have been extensively studied in the Penobscot River and river sections with dams are consistently identified as areas of high mortality (Holbrook et al., 2011; Stich et al., 2014; Stich et al., 2015a; Molina-Moctezuma et al., 2021; Molina-Moctezuma et al., 2022). As we explore later, sub-lethal injuries may partly explain why Atlantic salmon smolt mortality in the estuary is elevated both through delay and predation (Stich et al., 2015b; Stich et al., 2015c; Molina-Moctezuma et al., 2022).
For American eel, downstream migration is the culmination of up to decades of growth in fresh water before initiating fall migration. Migrants must locate a passage route and some individuals spend days searching, drawing on energy stores (Carr and Whoriskey, 2008; Piper et al., 2015; Eyler et al., 2016). Like other downstream migrating fish, adults risk impingement and impact-related injuries, as well as lethal and sub-lethal strikes by turbine blades in power generating stations (Piper et al., 2015; Eyler et al., 2016). In the Penobscot River, eel mortality rates are elevated at the two extant main-stem dams (West Enfield and Milford) but is indistinguishable from background mortality in reaches where Veazie and Great Works Dams were removed (Mensinger et al., 2021a). Injuries consistent with turbine blade strikes are commonly observed downstream of dams (Figure 4) supporting the assumption that entrainment through the turbines occurs – with negative outcomes for survival.
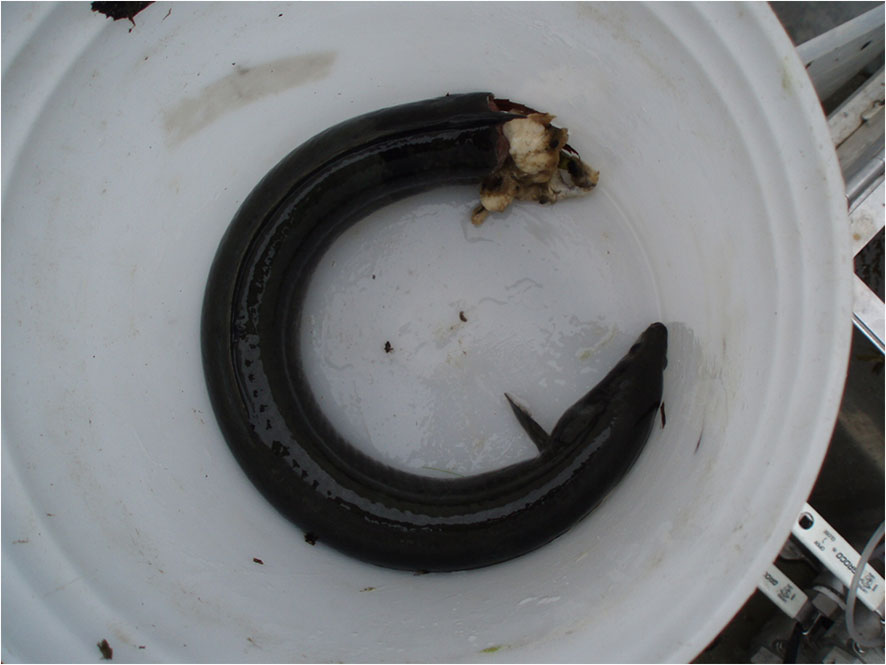
Figure 4 Injured American eels (Anguilla rostrata) are frequently encountered in the main stem of the Penobscot River, Maine, USA indicating that these downstream migrants pass through dam turbines and suffer injury. Severely injured fish may still be alive and may travel long distances (kilometers) from the site of injury, suggesting telemetry assessments may underestimate direct mortalities. (Photo credit, Zydlewski Laboratory, University of Maine).
Challenges of downstream passage are a partial driver of the decision to not pass sturgeon upstream of Milford Dam. The few shortnose sturgeon that entering the Milford fish lift annually are moved back downstream based on uncertainty in their historical range (assumed to be at the Milford Falls, although unclear [see Knight, 1985; Petersen and Sanger, 1986]) and resulting need to also move back downstream as adults or juveniles if spawning occurred (Jeff Murphy, NOAA’s National Marine Fisheries Service, personal communication). There mortality and injury have been demonstrated during downstream movements of sturgeon encountering dams during in other rivers systems (McDougall et al., 2013; McDougall et al., 2014; Jones and Cotel, 2023).
Changing conditions may also affect passage and survival at dams. At Milford Dam, water attraction conditions on the west shore of the Penobscot River tend to attract upstream migrants that are frequently left stranded in pools as river and operational conditions change (Jason Valliere, Maine Department of Marine Resources, personal communication, August 31, 2023). When noticed, this has led to concerted efforts to capture and move upstream migrating Atlantic salmon (Figure 5). Other species without federal protection, however, are not prioritized for such interventions. During the fall migration it is common to see stranded alosines that succumbed to low oxygen conditions (Figure 5).
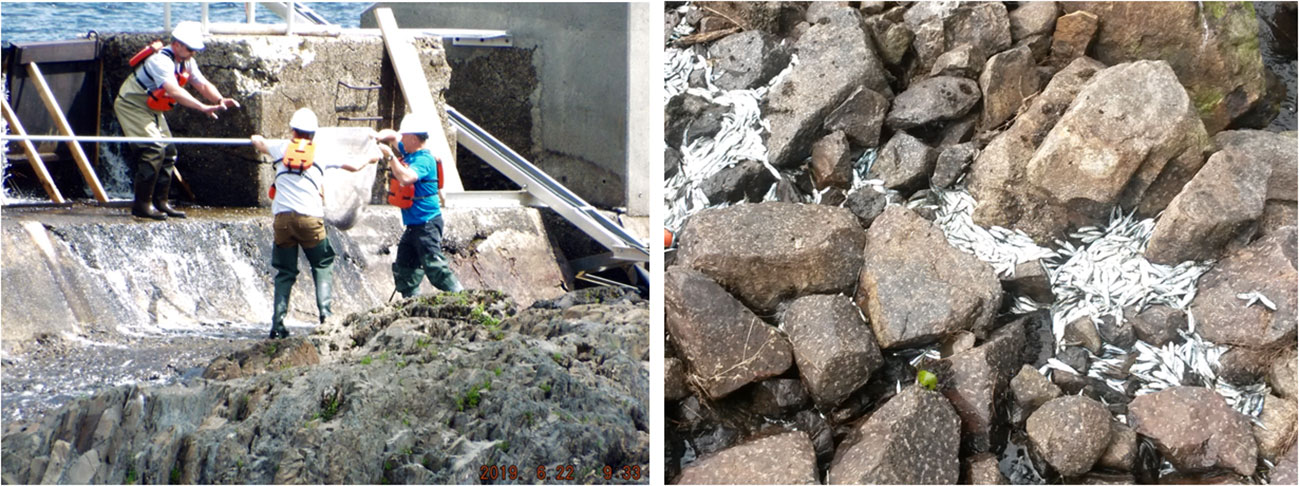
Figure 5 Changing environmental conditions in conjunction with operational changed may leave fish stranded as upstream or downstream migrants in the Penobscot River, Maine, USA. On the left, an endangered Atlantic salmon is rescued from a pool below Milford Dam that became isolated from the river while searching for an upstream route. On right, hundreds of alewife juvenile were stranded below a low-head dam as flows decreased. (Photo credits, Maine Department of Marine Resources).
Third: delays of migration
Rivers are the highways for migratory fish, and they are critical corridors for rapid movement. As we have discussed, dams restrict access to habitat. Engineering solutions (fishways) may facilitate movements and partly mitigate habitat fragmentation. Success, however, depends on three steps: attraction to the fishway entrance, entry, and successful transit. In the Penobscot River, the speed of movements and fish passage for both upstream and downstream migrating animals are slowed by dams in the system. This influence is obviated by the aggregations of migrating fish that may be observed below dams (Figure 6), but telemetry studies performed in the Penobscot River have provided more quantitative estimates of delay.
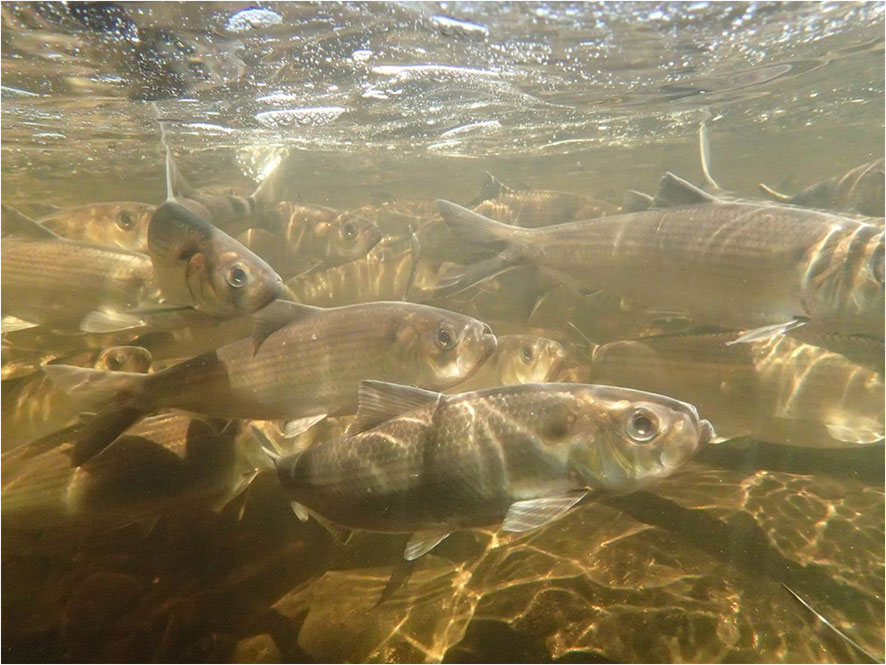
Figure 6 River herring are seen congregating below a dam on the Penobscot River, Maine, USA indicating the incomplete access provided to upstream habitat. (Photo credit, Zydlewski Laboratory).
Prior to the removal of Veazie and Great Works dams, upstream migrating Atlantic salmon adults were denied access to, or delayed in, reaching upstream habitat in the Penobscot River (Holbrook et al., 2009; Sigourney et al., 2015). For adult Atlantic salmon, completion of the dam removals as part of the PRRP allowed rapid passage through the reaches with the dam remnants. However, adults now experience substantial delays (often several weeks) at Milford Dam, a facility that operates with a fish elevator (Izzo et al., 2016) compared with the original Denil style fishway (a series of closely-spaced U-shaped baffles; Holbrook et al., 2009). Similarly, American shad adults approached Veazie Dam prior to the dam removals but did not pass in large numbers (Grote et al., 2014a). While passage of American shad at Milford Dam has increased (to more than 10,000 annually; DMR, 2022), telemetry assessment suggests that passage remains hampered by delays (Peterson, 2022). In contrast, sea lamprey adults now reach Milford Dam and are relatively successful in passing the dam (70–82%) with little delay (Peterson et al., 2023). While mean delay times for passage were low for successful fish (<48h), others experienced substantial delays (9–11 days) before abandoning upstream movements altogether.
Delays at dams are commonly observed in many impounded river systems. American shad (e.g., Castro-Santos et al., 2017; Weaver et al., 2019) and river herring (Haro et al., 1999; Noonan et al., 2012) have been demonstrated to have difficulty passing dams. Sea lamprey face delays and repeated failures to move through the fishways in the Connecticut River (Castro-Santos et al., 2017). Delays for salmonids have been documented in many river systems (Raymond, 1979; Wertheimer and Evans, 2005; Scruton et al., 2008; Keefer et al., 2012; Caudill et al., 2013; Nyqvist et al., 2017; Hagelin et al., 2021; Ohms et al., 2022). These delays may be biologically relevant (e.g., impacting ontogenic synchrony with nature) or even deadly. When fish are delayed by dams, they may be subjected to temperatures that reach lethal or near-lethal levels (Marschall et al., 2011). As discussed above, flows or water regulation decisions may also trap fish in areas where they are unable to escape or are susceptible to predation from natural or human predators (Figure 5). The longer they remain in the area, the more protracted the risk.
For adult upstream migrants, even modest delays may be energetically costly. Rubenstein et al. (2023) found that Atlantic salmon were delayed an average of 16–23 days at Milford Dam prior to passing and had lost 11–22% of initial fat reserves. These losses may be compounded by high water temperatures if thermal refugia are not available (Holbrook et al., 2009). Such delay-mediated energy losses are likely to be important during a migration (Thorstad et al., 2008). Returning adults cease consumption upon freshwater entry (Kadri et al., 1995) so that energy stores are the sole fuel for survival, migration, and spawning success.
For iteroparous species (e.g., Atlantic salmon, American shad, and river herring) protecting energy stores may contribute to post-spawn survival. Glebe and Leggett (1981) suggested that loss of more than 60% of energy reserves may serve as a constraint to iteroparity. For American shad, empirical evidence suggests that the threshold for post-spawn survival may be as low as 30–40% (Leonard and McCormick, 1999). Risks of both direct and indirect mortality through delay-mediated energy depletion are heightened when fish must pass multiple dams. This heightened mortality is consistent with the observed declines of American shad repeat spawners in the Connecticut River (New England) that fell from 49% (Walburg and Nichols, 1967; Carscadden and Leggett, 1975; Limburg et al., 2003), to 5% in 60 years after accessing habitat upstream of impoundments (Atlantic States Marine Fisheries Commission [ASMFC], 2020). This pattern of “forced semelparity” (Zydlewski et al., 2021) has obvious implications for population demographics. For semelparous species such as the sea lamprey, adult delays may likewise result in added energy losses that impair survival, migration, and spawning. For upstream migrating juvenile American eel, delays may functionally restrict their access to rearing habitat (Verdon and Desrochers, 2002) and may have differential individual effects based on variation in motivation (Mensinger et al., 2021b).
Delays are also observed at dams as fish migrate downstream. In the Penobscot River, Atlantic salmon smolts displayed slower movement rates in areas where dams were located (Spicer et al., 1995; Stich et al., 2015b). Studies also found that smolts arriving during the day experienced longer delays than those that arrived at night (Holbrook et al., 2011). Delays are directly associated with lower survival in the Penobscot River (Molina-Moctezuma et al., 2022) and elsewhere (Castro-Santos and Haro, 2003; Marschall et al., 2011; Nyqvist et al., 2017). This pattern is not held at all dams in the Penobscot River, however, as Browns Mill Dam (on the Piscataquis River, Maine, USA) had the lowest mortality (indistinguishable from in-river mortality) but the highest delays (up to 10 days; Molina-Moctezuma et al., 2021). Delays for downstream migrating smolts were reduced after construction of the nature-like fishway at Howland Dam in 2016, however, only about one third of the smolts used the bypass. Downstream delays from dams on the Penobscot River were also found to occur for adult American eels (Mensinger et al., 2021a), which may have a significant impact on spawning and population success as these are old, semelparous individuals.
Flow conditions strongly influenced the delays incurred by fish moving up or downstream at dams. Downstream migrating adult American eels were slowed at West Enfield and Milford dams, but this lag was erased individuals by higher flows during the migratory season (Mensinger et al., 2021a). Similarly, the Penobscot River experienced exceptionally high spring flows in 2017, 2018 and 2019. Atlantic salmon smolts tracked during these three years had greatly reduced delays and higher survival relative to lower flow years total cumulative survival of greater than 75% versus less than 50% in previous years (Molina-Moctezuma et al., 2022).
Delays may influence migrants through a disassociation of ontogenic processes with environmental windows of opportunity. Many migratory species develop physiological characteristics associated with anticipated shifts in habitat at the time of migration (Zydlewski and Wilkie, 2012). Smoltification in salmonids is an adaptive developmental stage that synchronizes the physiological capacity to osmoregulate in seawater with migratory behavior (Zydlewski and Wilkie, 2012; Stich et al., 2015c) and has been described as a window of opportunity to match physiological capacity with environmental conditions (McCormick et al., 2009). Delays may disrupt the match between migration and development. Consequently, fish may enter the ocean in suboptimal conditions (McCormick et al., 1998; Zydlewski et al., 2005; Marschall et al., 2011). All other factors being equal, salmonids migrating later in the season likely face greater physiological challenges than early migrants (McCormick et al., 1999). Overall, this can create greater physiological challenges, affect sensitivity to starvation, and adversely influence ocean survival (McCormick et al., 1999; Zydlewski et al., 2003; Wilson et al., 2022).
In contrast to the ontogenic development of seawater tolerance in migratory salmon, American shad develop tolerance to full strength seawater about three months in advance of their downstream migration (Zydlewski and McCormick, 1997a). However, juveniles lose their ability to regulate ions in fresh water, a change influenced by declining autumnal temperatures (Zydlewski and McCormick, 1997b). These developmental changes mean that entry into seawater late in the migratory season is physiologically challenging (Zydlewski et al., 2003) which may ultimately reduce survival of juvenile shad (Shrimpton et al., 2001). Whether migrating as a juvenile or as an adult, delays at dams consume critical energy for migration, cause a mismatch between developmental stages and the environment, and expose fish to unfavorable environmental conditions. We have shown how delays may lead to diminished survival and reduce biological fitness. Delays are also intimately intertwined with other risks, such as predation.
Fourth: facilitation of predation
Dam and fishway construction have been shown to create habitat suitable for opportunistic or ambush predators (Rieman and Beasmesderfer, 1991; Pasha et al., 1997). Fish that are aggregated near dams, delayed in passage, or disoriented by flow and turbulence, are increasingly vulnerable to predation (Ruggerone, 1986; Rieman and Beasmesderfer, 1991; Isaak and Bjornn, 1996; Blackwell and Juanes, 1998; Agostinho et al., 2012). Predator vulnerability of fish delayed near dams is obviated by seasonal diet shifts of predators incorporating more migrant prey species in their diets (Blackwell and Krohn, 1997; Fritts and Pearsons, 2006). This dietary shift has been observed in the Penobscot River where river herring have become an important seasonal prey item of smallmouth bass (Micropterus dolomieu) in the lower river (Watson et al., 2019).
Bald eagles (Haliaeetus leucocephalus), osprey (Pandion haliaetus) and double-crested cormorants (Phalacrocorax auritus) are known predators of diadromous species near impoundments (Ross and Follen, 1988; Call, 2015). In the Penobscot River, cormorants have been known to select seasonal foraging areas adjacent to dams to feed on migrating Atlantic salmon smolts in addition to other anadromous species such as rainbow smelt or river herring (Blackwell and Krohn, 1997). Prior to the PRRP, most avian predator diets in the upper Penobscot River were freshwater in source, although bald eagles were likely foraging on stocked Atlantic salmon smolts (Call, 2015). Presumably, avian predators now exploit the increased alosine forage base upstream of Milford Dam. Even large upstream migrants are vulnerable to avian predation. One of three adult salmon that passed Browns Mill Dam in 2020 was captured by a bald eagle while delayed in the 1 km head pond between dams Browns Mill and Moosehead Dams on the Piscataquis River (Peterson, 2022).
The role of in river predation for Atlantic salmon smolts has been of growing conservation concern. While marine mortality has been identified as being a critical source of loss for the species, freshwater and estuarine mortality may exceed coastal mortality for Atlantic salmon smolts (Kocik et al., 2009; Hawkes et al., 2019). In the Penobscot River, mortality rates for smolts are relatively low in free-flowing stretches of river but elevated near dams (Holbrook et al., 2011; Stich et al., 2015a; Molina-Moctezuma et al., 2022). Therefore, resolving the causal agent of mortality at dams is important for exploring possible mitigative actions. The development of acoustic predation tags provides a new tool for determining the disposition of tagged fish (Halfyard et al., 2017; Schultz et al., 2017). Recent acoustic telemetry data with predation sensors in the Penobscot River suggests that predation risk is 5-fold greater through impounded reaches (Mensinger et al., in press).
Atlantic salmon smolt mortalities in the estuary are also linked to their dam passage experiences (Stich et al., 2015a) likely because of increased delays (Molina-Moctezuma et al., 2022). Observed delays in transit rates at dams (Holbrook et al., 2011; Norrgård et al., 2013; Stich et al., 2015b) may result in loss of physiological smolt characteristics (McCormick et al., 1999; Budy et al., 2002; Ferguson, 2006) thereby reducing performance (Handeland et al., 1996). Thus, the asynchrony between the development of osmotic tolerance and timing of arrival in the estuary may contribute to mortality in the Penobscot estuary (Stich et al., 2015c). Such changes in salinity tolerance are exacerbated by descaling injuries as might occur at dams (Zydlewski et al., 2010) and may increase susceptibility to predation. Observations that predation risk in the estuary is nearly twice that of impounded areas (and 9-fold greater than in free-flowing river; Mensinger et al., in press) suggest a causal relation between dam delays and predation mortalities.
The slow rate of travel faced by downstream migrants in impoundments exposes migrants to fish, avian and mammalian predators. Weldon Dam’s impoundment is approximately 5 km long and is a reach of exceptionally high mortality risk for Atlantic salmon smolts (as high as 25%; Stich et al., 2015a; Molina-Moctezuma et al., 2022). It is notable that the gauntlet of predators in the impounded regions differs from the natural river. The changed lentic community favors predatory species including smallmouth bass, largemouth bass (Micropterus salmoides) and chain pickerel (Esox niger). While all these predators may be found throughout the Penobscot River, smallmouth bass are exceptionally widespread (Kramer, 2006; Kiraly et al., 2014). Smallmouth bass are a generalist and piscivorous fish that has been widely introduced (Loppnow et al., 2013) and implicated in declines of salmonids and other taxa (Magoulick, 2004; Fritts and Pearsons, 2006; Middaugh et al., 2016). As these predatory species are abundant in impoundments (Whittum et al., 2023), dam removal may notably reduce predation risk to juveniles by both reducing predator density and increasing speed of migration (Pasha et al., 1997).
The presence of predation in and near river impoundments is widely observed. In Pacific Northwest, migrating salmon (Oncorhynchus spp) are a key prey item for smallmouth bass near dams (Tabor et al., 1993; Fritts and Pearsons, 2004). Adult Atlantic salmon are preyed upon by the European catfish (Silurus glanis) near fishways in France (Boulêtreau et al., 2018) and in Brazil, redeye piranha (Serrasalmus rhombeus) prey on neotropical fish near fishway entrances (Agostinho et al., 2012). We note the presence of humans as another predator that are drawn to the aggregations of fish below dams (Jackson and Davies, 1988; Carey et al., 2011). Migratory fish in the Penobscot River are vulnerable enough near dams to warrant closure to “That area within 150 feet of any part of the Medway, West Enfield and Milford Dams, including fishways” (MDIFW, 2023). Milford Dam is highlighted as a place to fish for striped bass as their prey are congregated (Holyoke, 2021). Such angling pressure for striped bass and American shad results in the hooking of endangered adult Atlantic salmon (Jason Valliere, Maine Department of Marine Resources, personal observation, August 31, 2023).
Fifth: community shifts
Dams fundamentally change local biophysical conditions. Impoundments warm quickly, and shift from a lotic to lentic habitat. These changed conditions may disadvantage native species, providing a permissive environment for non-native species (Baxter, 1977; Ward and Stanford, 1987; Pess et al., 2008). Non-native fishes may find themselves well-positioned to outcompete or prey upon native salmonids and other fishes adapted to cold, free-flowing habitats. Impoundments may also tend to favor non-native minnow species (e.g., Whittum et al., 2023), which often arrive as bait-bucket introductions (Ludwig and Leitch, 1996). Dam-influenced fish assemblages are therefore not necessarily less diverse than those assemblages in free-flowing rivers (e.g., Burroughs et al., 2010; Hogg et al., 2015), but they are generally depleted in native species and enriched with non-native species. The Penobscot River is predominantly inhabited by macrohabitat generalist species (e.g., smallmouth bass) and riverine species (e.g., white sucker), and these species dominate the biomass in the main-stem Penobscot River.
Dam removals have been observed to result in rapid and often profound changes to riverine fish communities (Catalano et al., 2007; Burroughs et al., 2010; Hitt et al., 2012; Poulos et al., 2014). These changes have been demonstrated in the Sedgeunkedunk Stream, a tributary of the Penobscot River where persistent changes were observed within weeks of dam removal (Gardner et al., 2013; Hogg et al., 2015). In coastal systems, recolonization of diadromous fishes in newly available habitat represents a major shift (Hitt et al., 2012; Weigel et al., 2013; Hogg et al., 2015). Similarly, the fish community in the Penobscot River was substantially modified by the increased presence of migratory fish, but this influence diminishes with the number of dams that must be passed (Kiraly et al., 2014; Watson et al., 2018; Whittum et al., 2023).
The Penobscot River remains a heavily impounded system with improved, but demonstrably imperfect fish passage. Although upgraded passage has allowed migratory species to attain greater upstream ranges, much of the community structure above the lowermost dam has remained similar to pre-dam removal conditions (Whittum et al., 2023). Upriver, the East Branch of the Penobscot River currently has had little to no presence of alosines, suggesting poor combined passage through Milford, West Enfield, and Weldon dams. Weldon Dam is a significant barrier (13.7 m; USACE, 2023) compared to downstream dams. Fish passage at Weldon Dam (pool and weir) and West Enfield Dam (vertical slot) are likely more selective than the fish elevator system at Milford Dam (Bunt et al., 2012).
In the Piscataquis River, the Howland nature-like fishway allows for fish passage (to and from the Penobscot River) without changing the impoundment. The solution of a nature-like fishway is desirable when there is high social or cultural value attached to the impoundment. Like other fishways, this structure leaves the habitat upstream of Howland Dam with little change after construction (Kiraly et al., 2014; Watson et al., 2018; Whittum et al., 2023). As might be anticipated, the extant impounded riverine reaches within the Penobscot River continue to favor cyprinid species and higher relative abundance of top predators, such as chain pickerel, smallmouth bass, and largemouth bass. As a result, any juvenile migrants above these dams may encounter an enhanced local density of piscivores when moving downriver. Migration delays at impounded locations may provide additional opportunity for predation from these reservoir species (Molina-Moctezuma et al., 2021). The risk is multiplied if passing several dams.
We would be remiss if we did not note that dam removal may also have unintended (and undesired) outcomes for the native fish community by facilitating the movement of non-native species. The expansion of some non-native fish such as the white catfish (Ameriuris catus) are likely the result of increased movement permeability in the system (Whittum, 2022). When contemplating the use of dam removal as a conservation approach, managers may benefit from considering how enhanced passage might influence non-native species (Cooper et al., 2021). Indeed, fears of the expansion of angler transported (and non-native) northern pike (Esox lucius) have prompted state proposed legislation to modify fishways on tributaries of the Penobscot River to exclude “invasives” (LD 1049, 131st Maine Legislature, An Act to Protect Maine’s Inland Fisheries from Invasive Fish). In principle, fishway modification may allow for differential passage (and therefore connectivity) for different species based on species-specific fishway passage performance (Noonan et al., 2012). This approach would, however, make fish passage more difficult for all species.
Sixth: demographic shifts
Dams have the potential to cause demographic shifts in populations due to a suite of influences on distinct ontogenetic stages and variable life histories within species. These influences may include shifts that result from selective pressures such as size selection in fish passage and mismatches between upstream access and downstream survival through dams for migrants. At the population level, shifts may manifest as changes in size and age structure, reductions in rates of iteroparity, or loss of life-history complexity and variability. While demographic shifts are increasingly well documented, the degree to which selective pressures influence local adaptation is poorly understood for many species.
Size selection in fish passage may limit access to spawning and rearing habitat by individuals of specific sizes, which alters average size of individuals while reducing variability in size. For anadromous species, selectivity in fish passage directly imposes selection on the component of adult spawners that access spawning habitat. This selection may operate on physiology, anatomy, or behavior (Mensinger et al., 2021b). Size-selective passage of Atlantic salmon has been observed in the Penobscot River (Sigourney et al., 2015; Maynard et al., 2017), whereby larger fish were less successful in passing dams to reach spawning habitat. Exclusion of the largest females from spawning grounds may affect both underlying phenotypes of spawners and survival in other life stages for a population. Trucking spawning fish around fishways, combined with conservation hatchery practices, may partly alleviate this selective pressure (Sigourney et al., 2015; Maynard et al., 2017).
Mensinger et al., 2021b observed that larger juvenile (glass) eels climbed faster than smaller ones. For catadromous species this size selection in passage may lead to differences in individual growth opportunities in freshwater habitats, or even skew sex ratios based on physiological limitations of habitat downstream of dams (Mensinger et al., 2021b). Size selection at challenging passage structures (anthropogenic or natural) may impose energetic limitations on rates of growth, maturation, and migration that have not been extensively studied. It may simply make some upstream habitat functionally inaccessible (Verdon and Desrochers, 2002).
Dams may also cause shifts in size structure and life history traits through mortality during downstream passage. If downstream survival of adult and juvenile fish through dams is not sufficiently high, upstream fish passage may become an ecological trap (Ohms et al., 2022). Because life history traits such as size, age at maturity, and iteroparity are co-inherited (Aykanat et al., 2019), downstream survival through dams may influence multiple population demographics simultaneously. Low survival through dams during downstream migration by post-spawn adults was associated with reduced iteroparity of Atlantic salmon in the Penobscot River (Maynard et al., 2018) and steelhead trout (Oncorhynchus mykiss) in the Snake and Columbia rivers in the northwestern USA (Wertheimer and Evans, 2005; Keefer et al., 2008). When adult downstream survival rates through dams were less than perfect (i.e., 100%), American shad age structures were predicted to be truncated through loss of older fish and repeat spawning rates were predicted to be reduced with increasing upstream fish passage (Castro-Santos and Letcher, 2010; Stich et al., 2019). Additive mortality incurred by passing multiple dams is compounded at the watershed scale with respect to changes in demographics such as abundance, size structure, and iteroparity (Castro-Santos and Letcher, 2010; Stich et al., 2019; Zydlewski et al., 2021).
The degree to which demographic shifts are realized may vary with damming intensity and upstream fish passage, environmental conditions, and life history variation. Abundance of American shad, for example, varies as a function of upstream passage, number of dams, configuration of spawning and rearing habitat relative to dams, as well as latitudinal clines in growth, maturation, fecundity, and iteroparity (Zydlewski et al., 2021). In the Penobscot River, where only 16 adults passed the lowermost dam from 1978 through 2012, fish exhibited repeat spawning rates as high as 75–95% (Grote et al., 2014b), though the population persisted at low abundance (Grote et al., 2014a) prior to the removal of Veazie Dam. Fish reached smaller maximum sizes and reached older ages despite elevated natural mortality estimates in the Penobscot River compared to other rivers in the northeastern part of their range (Gilligan-Lunda et al., 2021). In the Connecticut River, maximum age and repeat spawning rates have been reduced since implementation of upstream fish passage and despite closure of commercial fisheries and stable spawner abundances (ASMFC, 2020). These reductions in maximum age and repeat spawning have occurred even though Connecticut River American shad reached larger sizes and experienced lower mortality rates than in the Penobscot River (Gilligan-Lunda et al., 2021).
In species or populations with variable life histories, dams may influence population demographics through elimination of life history complexity, thereby reducing evolutionary stability of populations in variable environments. For example, coastal cutthroat trout (Oncorhynchus clarkii; Trotter, 1989) and steelhead trout (Thorpe et al., 1998; Satterthwaite et al., 2009; Hodge et al., 2016) exhibit high diversity in anadromous and freshwater resident life histories, in addition to variability in iteroparity. This diversity of life histories presumably reduces risk to extirpation through a portfolio effect (Moore et al., 2014) and includes genotypic and plastic responses (Satterthwaite et al., 2009; Whiteley et al., 2010). Whereas population structuring has been observed in steelhead trout upstream and downstream of barriers in the Elwha River, this structuring rapidly degraded following dam removal. Data suggest that overall genetic diversity was preserved within isolated freshwater and anadromous populations, indicating strong potential for recovery (Fraik et al., 2021). Similarly, a large body of research has demonstrated rapid adaptation of freshwater life histories in alewife following dam construction and land locking independently among many populations in Connecticut, USA (Palkovacs et al., 2008). Both phenotypic and genotypic responses in alewife populations are postulated to create eco-evolutionary feedbacks that drive rapid changes in populations (Palkovacs and Post, 2008). Recent evidence suggests that many of the underlying genomic changes can also be reversed through introgression with anadromous individuals following implementation of fish passage or dam removal (Reid and Goodman, 2020).
Finally, American shad exhibit parity on a continuum across their native range, with semelparous populations in southern rivers (south of 35° latitude) and increasingly high rates of iteroparity in northern rivers (Leggett and Carscadden, 1978) that correlate to differences in maturation (ASMFC, 2020), growth, and longevity (Gilligan-Lunda et al., 2021) and regional population structuring (Hasselman et al., 2010). Within the central range (35–41° latitude) of American shad, intermediate rates of iteroparity prevail, with multiple life histories present in some rivers (ASMFC, 2020). It remains unknown to what degree these life histories vary longitudinally within rivers. Work in undammed rivers (e.g., Delaware River, mid-Atlantic region of the USA) may aid in differentiating between the influence of migratory distance and damming.
Seventh: loss of ecosystem services
The six influences of dams discussed above are directly tied to interactions with migratory fishes. The ecological influences of dams, however, include changes in ecosystem function and human use that are indirectly linked to migrating fishes through ecosystem functions. We broadly identify these changes as “ecosystem services”. Inclusion of connections between migratory fishes and broader ecosystem function in this paper is consistent with shifts towards more holistic approaches to ecosystem and multispecies management (Larkin, 1996; Eriksson et al., 2011; Andersen et al., 2015). Ecosystem services have supported human well-being through history in both expected and unanticipated ways (Limburg and Waldman, 2009; Hall et al., 2012).
Dams have been the primary cause of migratory fish population declines across North America (Limburg and Waldman, 2009) and Europe (Wilson and Venerata, 2019). The direct loss of fisheries potential in the Penobscot watershed has been documented (Saunders et al., 2006). Hall et al. (2011) calculated that by 1850, river herring spawning habitat in Maine had been reduced to less than 5% of available habitat because of dam construction on small rivers, and to 1% of habitat by 1887 when the largest rivers were spanned by dams (Atkins, 1887). Impoundment of the Penobscot River has reduced American shad production potential nearly 90% (Stich et al., 2019), contributing to a dam-related coast-wide loss of 39% based on habitat access alone (Zydlewski et al., 2021). The loss of recreational angling for sea run fish also affects the region’s economy (Pinfold, 2011) and culture (Schmitt, 2016). The loss of fisheries has been particularly detrimental to indigenous communities.
The connection of Wabanaki people on the Penobscot River to once abundant sea run-fish species has been important for both sustenance and cultural connection (Speck, 1940; Harper and Ranco, 2009). The Penobscot Nation, penawahpkekeyak, are the people of the place of the white rocks, referring to a reach of river that bears their name. Similar connections are reinforced through stories and folklore (Kolodny, 2007). This federally recognized tribe has more than 2,400 enrolled members and is considered one of the oldest continuous governments in the world (Charlie Loring, Jr., Director, Department of Natural Resources, Penobscot Indian Nation, personal communication, August 31, 2023). Dam-mediated losses of sea run fish are viewed as a critical threat to the Penobscot Nation’s formative connection to the river, and the PRRP has been viewed as integral to the “reclamation of their cultural identity and sovereignty” (Frederick, 2006).
Dam-mediated losses of migratory fish runs may have effects beyond their local geography, either through trophic connections (as predator or prey) or range-wide population resilience. For the semelparous, panmictic American eel, adult downstream migrations are limited by access to upstream juvenile rearing habitat. Dam related loss of production from any river means that the entire population is diminished, particularly as adults from northern regions tend to be female (Wang and Tzeng, 1998; Jessop, 2010). Ames and Lichter (2013) assert that large, stable concentrations of young-of-the-year alosines influenced where resident and migrating gadid (cod) groups were located. The dam-associated loss of river herring and shad resulted in a loss of forage for ground fish such as Atlantic cod (Gadus morhua) haddock (Melanogrammus aeglefinus), pollock (Pollachius virens), and white hake (Urophyscus tenuis). These important northern coastal shelf fisheries collapsed coincident with the damming of rivers in the region (Lotze and Milewski, 2004). Striped bass also prey on blueback herring, alewife, and American shad, thereby benefiting from their abundance (Trent and Hassler, 1966; Nelson et al., 2003; Walter and Austin, 2003; Savoy and Crecco, 2004).
As dams alter fish assemblages, other interactions may directly or indirectly occur (Hanson and Curry, 2005; Kiffney et al., 2009). The presence of alewife and blueback herring in high numbers may benefit other species through substitution. As river herring populations rebound in the Penobscot River, it has been suggested that they may serve as a prey buffer for species such as the endangered Atlantic salmon (Saunders et al., 2006; Oke et al., 2020; Hare et al., 2021). These species are likely to become more important as a forage base as populations increase both in river and along the coast (Ames and Lichter, 2013). Mammalian predators such as the harbor seal (Phoca vitulina) and birds (e.g., bald eagles, osprey, double-crested cormorants) would also likely be limited by dam-mediated population losses (Able and Fahay, 2010; Call, 2015).
Predation pathways contribute important linkages among freshwater, marine, and terrestrial ecosystems by transference of energy and nutrients (e.g., Durbin et al., 1979; Petticrew et al., 2011; Willis et al., 2017; Barber et al., 2018). Anadromous species transfer freshwater-derived nutrients to marine environments during juvenile seaward migration (Willis et al., 2017; Barber et al., 2018). Then adults, which obtain a high proportion of their mass while feeding in marine environments, transfer “marine-derived nutrients” (hereafter MDN) to freshwater ecosystems during the spawning season through direct consumption, the release of gametes, excretion of metabolic wastes, and carcasses decay (e.g., Durbin et al., 1979; Twining et al., 2017; Barber et al., 2018). The pathways of MDN transference from anadromous fish to streams vary among their life history. While carcass decay is the principal input from semelparous species, excretion is the principal input from some iteroparous species such as alosines (Schindler et al., 2003; Post and Walters, 2009; Figure 7). Additionally, carcasses of anadromous fishes may be transferred to terrestrial ecosystems by water movement and terrestrial predators, thereby transferring MDN to riparian food webs (Hocking and Reynolds, 2011; Quinn et al., 2018). Catadromous species can generate the same transference of energy and nutrients in an opposite pathway (Saboret et al., 2021).
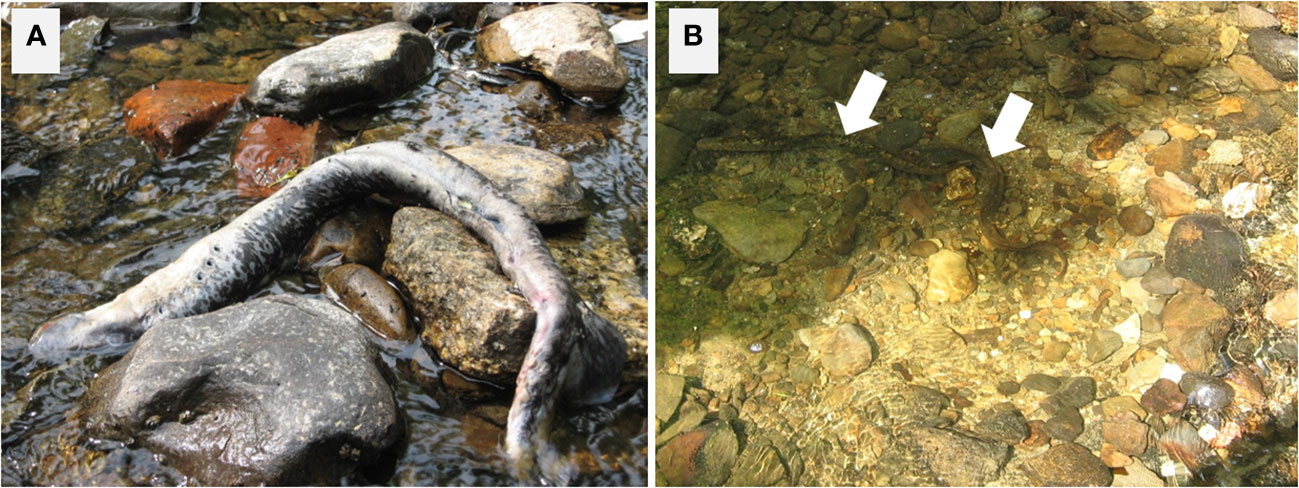
Figure 7 Migrating fish impact their ecosystems in complex ways. Sea lamprey (Petromyzon marinus) are native to the Penobscot River, Maine, USA. (A) As a semelparous species, lamprey carcasses provide nitrogen and phosphorous into deprived streams. (B) These fish have been shown to condition stream beds in ways that benefit other vertebrate and invertebrate taxa (lamprey are indicated with arrows, the nest that has been dug is in the center). (Photo credits, Zydlewski Laboratory, University of Maine).
Pulsed subsidies of MDN from anadromous fish increase the primary productivity of freshwater ecosystems through the bottom-up pathway of nutrient incorporation. In temperate regions, where freshwater ecosystems are less productive than marine ecosystems, MDN from anadromous species transfers nutrients to freshwater ecosystems increasing their primary productivity (Wipfli et al., 2010; Weaver et al., 2016; Weaver et al., 2018a). In the Penobscot River basin, both bottom-up and top-down pathways of MDN incorporation in freshwater ecosystems have been studied experimentally through carcass addition and theoretically through modeling (Guyette et al., 2013; Guyette et al., 2014; Weaver et al., 2015; Weaver et al., 2016; Weaver et al., 2018b; Weaver et al., 2018c; Zydlewski et al., 2021). Both producers and consumers incorporate MDN from anadromous fish subsidies (Guyette et al., 2013; Guyette et al., 2014; Weaver et al., 2016; Weaver et al., 2018b). Direct or indirect assimilation of carcass material may increase growth of young Atlantic salmon thereby bolstering survival (Guyette et al., 2013). Sea lamprey larvae assimilated nutrients found from the carcasses of their post-spawn adult conspecifics, which may improve their growth and enhance earlier metamorphosis (Weaver et al., 2018c).
Consumption of MDN from anadromous fish subsidies may also transfer beneficial biomolecules to in-stream consumers. Anadromous fishes accumulate high contents of vital dietary biomolecules such as ω-3 highly unsaturated fatty acids (hereafter ω-3 HUFAs) in their tissues while feeding in marine food webs; therefore, they transfer them from ω-3 HUFAs-rich (marine) to ω-3 HUFAs-poor food webs (freshwater) through their subsidies (Fuiman et al., 2015; Figueroa-Muñoz et al., 2022; Závorka et al., 2023). Evidence of transference of eicosapentaenoic acid (EPA) and docosahexaenoic acid (DHA) to in-stream consumers following the consumption of anadromous fish subsidies (i.e., carcasses and eggs) has been documented both experimentally and in natural systems (Heintz et al., 2004; Landsman et al., 2018; Figueroa-Muñoz et al., 2022). Indeed, pulsed subsidies of MDN from anadromous fishes, especially eggs, can constitute an important source of ω-3 HUFAs to predators in the Penobscot River.
The absence of migratory fish from habitat in the Penobscot River has deprived the ecosystem of other services as well. Atlantic salmon and sea lamprey are considered “ecosystem engineers”: they physically change benthic habitats during their nest construction, thereby affecting other organisms in streams (Moore, 2006; Hogg et al., 2014). Spawning sea lamprey generate changes in the streambed during nest construction, making it more complex and benefitting benthic invertebrates (Hogg et al., 2014; Weaver et al., 2018b; Figure 7). Furthermore, nest construction may benefit drift-feeding species (e.g., brook trout and Atlantic salmon) by providing them with energetically profitable foraging habitats (Fausch and Northcote, 1992) or by improved spawning habitat through removal of fine sediments (Saunders et al., 2006; Hogg et al., 2014).
The interruption of migratory fishes by dams also interrupts the life cycle of a myriad of parasitic organisms. While impacts on some species may not initially be recognized as a lost opportunity cost for society (e.g., a host for freshwater copepods; Powell et al., 1999), other interactions are readily recognized as valuable from the human perspective. Freshwater mussels are one of the most imperiled groups of animals in North America (Master et al., 1998) with many species federally listed or extinct (Bogan, 1998). As a result, two species – the yellow lamp mussel (Lampsilis cariosa) and tidewater mucket (Leptodea ochracea) – were listed as Threatened under the Maine Endangered Species Act in 1997. Within watersheds, dams constrain fish movement and limit freshwater mussel dispersal through their fish-host dependent life history. Glochidia – the parasitic larval stage of freshwater mussels – generally require a vertebrate host to complete development (Kat, 1984; Kneeland and Rhymer, 2008). It is easy to see, therefore, how limitations in fish movement also may limit distribution patterns of mussels. Such examples demonstrate how the loss of migratory fish on one system may influence local and regional ecosystems in complex ways.
Summary
Both the removals of dams and the continued operations of other dams in the Penobscot River have allowed us to better understand the direct and indirect ways that these structures fundamentally alter the river ecosystem. The ecological influences imposed by dams on migratory fishes are both complex and interconnected. Fish populations are affected by direct exclusion, injury, and delay. We have also highlighted less obvious effects such as predation, community changes, demographic shifts, and loss of ecosystem services. The loss of ecosystem services is a diminution of the ecological links native fish species have with the physical, biological, and cultural aspects of the watershed. This complexity makes comprehensive assessment of these seven factors challenging to assess, especially in isolation from one another.
Because dams are constructed and operated to serve human needs, their presence represents a tradeoff with their influence on ecological function (Roy et al., 2018; Song et al., 2019; Roy et al., 2020). In some cases, direct comparisons of alternative approaches may be instructive in weighing costs and benefits of hydropower (Sharma and Waldman, 2021). Such analysis is hampered, however, by the inherent challenges in quantifying the value of an intact river (WWF, 2022), leading conventional economic analyses to chronically undervalue natural resources (Odum, 2007).
Data from the Penobscot River and elsewhere demonstrate that fishways are consistently incomplete in restoring habitat connectivity (Hershey, 2021), and likely fall short in the capacity for restoring populations to the levels possible in intact rivers (Zydlewski et al., 2021). However, fishways are frequently seen as the de-facto “solution” for fragmentation (Waldman and Quinn, 2022) despite their generally poor performance (Noonan et al., 2012). Where socioeconomic tradeoffs are possible, complete removal of dams has been repeatedly demonstrated as an effective tool for restoring the ecological functions that have been diminished (Magilligan et al., 2016; Waldman and Quinn, 2022). The removal of the Edwards Dam in Kennebec River in Maine (1999) was one of the first targeted dam removals for migratory fish restoration (Crane, 2009). Decades later migratory fish have returned to that river, in the millions for some species (Wippelhauser, 2021). Within the Penobscot River, dam removals have allowed the full suite of native fishes to recolonize parts of their historic ranges (Trinko Lake et al., 2012; Hogg et al., 2013; Izzo et al., 2016; Johnston et al., 2019; Whittum et al., 2023), with populations of some species also numbering in the millions now. The Penobscot River remains heavily impounded and ecological processes are impaired, but rehabilitation has brought a suite of migratory fish back to waters that been without them for generations.
Author contributions
JZ: Writing – original draft, Writing – review & editing. SC: Writing – original draft. CD: Writing – original draft, Writing – review & editing. GF-M: Writing – original draft, Writing – review & editing. CM: Writing – original draft, Writing – review & editing. SS: Writing – original draft. RS: Writing – original draft, Writing – review & editing. DS: Writing – original draft, Writing – review & editing. SV: Writing – original draft, Writing – review & editing. KW: Writing – original draft, Writing – review & editing. GZ: Writing – review & editing.
Acknowledgments
Logistic support was provided by the University of Maine Department of Wildlife, Fisheries, and Conservation. In-kind support was provided by the U.S. Geological Survey, Maine Cooperative Fish and Wildlife Research Unit. Support was provided by the Maine Agricultural and Forestry Experiment Station. The authors appreciate the insights of Molly Payne Wynne and the review of an early draft of the document. Lara Katz provided expertise in mapping. Any use of trade, firm, or product names is for descriptive purposes only and does not imply endorsement by the U.S. Government. This work did not use vertebrate animals.
Conflict of interest
The authors declare that the research was conducted in the absence of any commercial or financial relationships that could be construed as a potential conflict of interest.
Publisher’s note
All claims expressed in this article are solely those of the authors and do not necessarily represent those of their affiliated organizations, or those of the publisher, the editors and the reviewers. Any product that may be evaluated in this article, or claim that may be made by its manufacturer, is not guaranteed or endorsed by the publisher.
References
Able K. W., Fahay M. P. (2010). Ecology of estuarine fishes (Baltimore, MD: Johns Hopkins University Press).
Agostinho A. A., Agostinho C. S., Pelicice F. M., Marques. E. E. (2012). Fish ladders: safe fish passage or hotspot for predation? Neotropical Ichthyol. 10, 687–696. doi: 10.1002/nvsm.1427
Algera D. A., Rytwinski T., Taylor J. J., Bennett J. R., Smokorowski K. E., Harrison P. M., et al. (2020). What are the relative risks of mortality and injury for fish during downstream passage at hydroelectric dams in temperate regions? A systematic review. Environ. Evidence 9, 1–36. doi: 10.1186/s13750-020-0184-0
Ames E. P., Lichter J. (2013). Gadids and alewives: structure within complexity in the Gulf of Maine. Fisheries Res. 141, 70–78. doi: 10.1016/j.fishres.2012.09.011
Andersen K. H., Brander K., Ravn-Jonsen L. (2015). Trade-offs between objectives for ecosystem management of fisheries. Ecol. Appl. 25 (5), 1390–1396. doi: 10.1890/14-1209.1
Atkins C. G. (1887). The river fisheries of Maine. fisheries fishery industries United States 1, 673–728.
Atlantic States Marine Fisheries Commission (ASMFC) (2020). American shad Benchmark Stock Assessment and Peer Review (Arlington, VA: ASMFC).
Aykanat T., Ozerov M., Vähä J. P., Orell P., Niemelä E., Erkinaro J., et al. (2019). Co-inheritance of sea age at maturity and iteroparity in the Atlantic salmon vgll3 genomic region. J. Evolutionary Biol. 32 (4), 343–355. doi: 10.1111/jeb.13418
Barber B. L., Gibson A. J., O’Malley A. J., Zydlewski J. (2018). Does what goes up also come down? Using a recruitment model to balance alewife nutrient import and export. Mar. Coast. Fisheries 10 (2), 236–254. doi: 10.1002/mcf2.10021
Baxter R. M. (1977). Environmental effects of dams and impoundments. Annu. Rev. Ecol. systematics 8 (1), 255–283. doi: 10.1146/annurev.es.08.110177.001351
Bennett Z. M. (2017). “A means of removing them further from us”: the struggle for waterpower on New England’s eastern frontier. New Engl. Q. 90 (4), 540–560. doi: 10.1162/tneq_a_00640
Blackwell B. F., Juanes F. (1998). Predation on Atlantic salmon smolts by striped bass after dam passage. North Am. J. Fisheries Manage. 18 (4), 936–939. doi: 10.1577/1548-8675(1998)018<0936:POASSB>2.0.CO;2
Blackwell B. F., Krohn W. B. (1997). Spring foraging distribution and habitat selection by double-crested cormorants on the Penobscot River, Maine USA. Colonial Waterbirds 20 (1), 66. doi: 10.2307/1521765
Bogan A. E. (1998). “Freshwater molluscan conservation in North America: Problems and practices,” in Molluscan Conservation: A Strategy for the 21st Century. Eds. Killeen I. J., Seddon M. B., Holmes A. M., Conchol J. 2, 223–230.
Boulêtreau S., Gaillagot A., Carry L., Tétard S., De Oliveira E., Santoul. F. (2018). Adult Atlantic salmon have a new freshwater predator. PloS One 13 (4), e0196046. doi: 10.1371/journal.pone.0196046
Budy P., Thiede G. P., Bouwes N., Petrosky C. E., Schaller H. (2002). Evidence linking delayed mortality of Snake River salmon to their earlier hydrosystem experience. North Am. J. Fisheries Manage. 22 (1), 35–51. doi: 10.1577/1548-8675(2002)022<0035:ELDMOS>2.0.CO;2
Bunt C. M., Castro-Santos T., Haro A. (2012). Performance of fish passage structures at upstream barriers to migration. River Res. Appl. 28 (4), 457–478. doi: 10.1002/rra.1565
Burroughs B. A., Hayes D. B., Klomp K. D., Hansen J. F., Mistak J. (2010). The effects of the Stronach Dam removal on fish in the Pine River, Manistee County, Michigan. Trans. Am. Fisheries Soc. 139 (5), 1595–1613. doi: 10.1577/T09-056.1
Call E. (2015). River birds as indicators of change in riverine ecosystems (Orono, ME: The University of Maine).
Carey M. P., Sanderson B. L., Friesen T. A., Barnas K. A., Olden J. D. (2011). Smallmouth bass in the Pacific Northwest: a threat to native species; a benefit for anglers. Rev. Fisheries Sci. 19 (3), 305–315. doi: 10.1080/10641262.2011.598584
Carr J. W., Whoriskey F. G. (2008). Migration of silver American eels past a hydroelectric dam and through a coastal zone. Fisheries Manage. Ecol. 15 (5-6), 393–400. doi: 10.1111/j.1365-2400.2008.00627.x
Carscadden J. E., Leggett W. C. (1975). Life history variations in populations of American shad, Alosa sapidissima (Wilson), spawning in tributaries of the St John River, New Brunswick. J. Fish Biol. 7 (5), 595–609. doi: 10.1111/j.1095-8649.1975.tb04633.x
Carvajal-Quintero J. D., Januchowski-Hartley S. R., Maldonado-Ocampo J. A., Jezequel C., Delgado J., Tedesco P. A. (2017). Damming fragments species’ ranges and heightens extinction risk. Conserv. Lett. 10 (6), 708–716. doi: 10.1111/conl.12336
Castro-Santos T., Cotel A.L.I.N.E., Webb P. W. (2009). “Fishway evaluations for better bioengineering: an integrative approach,” in Challenges for diadromous fishes in a dynamic global environment, vol. 69. (Bethesda, MD: American Fisheries Society, Symposium), 557–575.
Castro-Santos T., Haro A. (2003). Quantifying migratory delay: a new application of survival analysis methods. Can. J. Fisheries Aquat. Sci. 60 (8), 986–996. doi: 10.1139/f03-086
Castro-Santos T., Letcher B. H. (2010). Modeling migratory energetics of Connecticut River American shad (Alosa sapidissima): Implications for the conservation of an iteroparous anadromous fish. Can. J. Fisheries Aquat. Sci. 67 (5), 806–830. doi: 10.1139/F10-026
Castro-Santos T., Shi X., Haro A. (2017). Migratory behavior of adult sea lamprey and cumulative passage performance through four fishways. Can. J. Fisheries Aquat. Sci. 74 (5), 790–800. doi: 10.1139/cjfas-2016-0089
Catalano M. J., Bozek M. A., Pellett T. D. (2007). Effects of dam removal on fish assemblage structure and spatial distributions in the Baraboo River, Wisconsin. North Am. J. Fisheries Manage. 27 (2), 519–530. doi: 10.1577/M06-001.1
Caudill C. C., Keefer M. L., Clabough T. S., Naughton G. P., Burke B. J., Peery C. A. (2013). Indirect effects of impoundment on migrating fish: temperature gradients in fish ladders slow dam passage by adult chinook salmon and steelhead. PloS One 8 (12), e85586. doi: 10.1371/journal.pone.0085586
Cooper A. R., Infante D. M., O'Hanley J. R., Yu H., Neeson T. M., Brumm K. J. (2021). Prioritizing native migratory fish passage restoration while limiting the spread of invasive species: a case study in the Upper Mississippi River. Sci. total Environ. 791, 148317. doi: 10.1016/j.scitotenv.2021.148317
Crane J. (2009). “Setting the river free”: The removal of the Edwards dam and the restoration of the Kennebec River. Water History 1, 131–148. doi: 10.1007/s12685-009-0007-2
Day L. R. (2006). Restoring native fisheries to Maine’s largest watershed: the Penobscot River Restoration Project. J. Contemp. Water Res. Educ. 134), 29–33.
DMR. (2022). Historical trap counts. Maine Department of Marine Resources. Available at: https://www.maine.gov/dmr/sites/maine.gov.dmr/files/inline-files/Trap%20Count%20Archive%202022.pdf.
Dudley R. W., Hodgkins G. A. (2002). Trends in streamflow, river ice, and snowpack for coastal river basins in Maine during the 20th century: U.S. Geological Survey Water-Resources Investigations Report 02–4245. 26 (Augusta, ME: U.S. Geological Survey).
Durbin A. G., Nixon S. W., Oviatt C. A. (1979). Effects of the spawning migration of the alewife, Alosa pseudoharengus, on freshwater ecosystems. Ecology 60, 8–17. doi: 10.2307/1936461
Enterline C. L., Chase B. C., Carloni J. M., Mills K. E. (2012). A regional conservation plan for anadromous Rainbow smelt in the US Gulf of Maine NOAA Species of Concern Grant Program Award #NA06NMF4720249A.
Eriksson B. K., Sieben K., Eklöf J., Ljunggren L., Olsson J., Casini M., et al. (2011). Effects of altered offshore food webs on coastal ecosystems emphasize the need for cross-ecosystem management. Ambio 40, 786–797. doi: 10.1007/s13280-011-0158-0
Everhart W. H., Cutting R. E. (1968). The Penobscot River: Atlantic Salmon Restoration, Key to a Model River (Brewer, ME: Penobscot County Conservation Association).
Eyler S. M., Welsh S. A., Smith D. R., Rockey M. M. (2016). Downstream passage and impact of turbine shutdowns on survival of silver American eels at five hydroelectric dams on the Shenandoah River. Trans. Am. Fisheries Soc. 145 (5), 964–976. doi: 10.1080/00028487.2016.1176954
Ferguson A. (2006). “Genetics of sea trout, with particular reference to Britain and Ireland,” in Sea trout: Biology, conservation and management Eds. Harris G. S., Milner N. J. (Oxford, England: Blackwell Publishing), 157–182.
Fernandes S. J., Zydlewski G. B., Zydlewski J. D., Wippelhauser G. S., Kinnison M. T. (2010). Seasonal distribution and movements of shortnose sturgeon and Atlantic sturgeon in the Penobscot River estuary, Maine. Trans. Am. Fisheries Soc. 139 (5), 1436–1449. doi: 10.1577/T09-122.1
Figueroa-Muñoz G., Arismendi I., Urzúa Á., Guzmán-Rivas F., Fierro P., Gomez-Uchida D. (2022). Consumption of marine-derived nutrients from invasive Chinook salmon (Oncorhynchus tshawytscha) transfer ω-3 highly unsaturated fatty acids to invasive resident rainbow trout (O. mykiss). Sci. Total Environment. 844, 157077. doi: 10.1016/j.scitotenv.2022.157077
Fleming I. A. (1996). Reproductive strategies of Atlantic salmon: ecology and evolution. Rev. fish Biol. fisheries 6, 379–416. doi: 10.1007/BF00164323
Foster N. W., Atkins C. G. (1867). “Report of Commission on fisheries,” in Twelfth annual report of the Secretary of the Maine Board of Agriculture (Augusta, Maine: Stevens and Sayward Printers to the State).
Fraik A. K., McMillan J. R., Liermann M., Bennett T., McHenry M. L., McKinney G. J., et al. (2021). The impacts of dam construction and removal on the genetics of recovering steelhead (Oncorhynchus mykiss) populations across the Elwha River watershed. Genes 12 (1), 89. doi: 10.3390/genes12010089
Frederick K. L. (2006). Resurrecting a River and its People: An Environmental History of the Penobscot River and the Contemporary Efforts to Facilitate Environmental Change on the Penobscot River (Orono, ME: The University of Maine).
Freeman M. C., Pringle C. M., Greathouse E. A., Freeman B. J. (2003). Ecosystem-level consequences of migratory faunal depletion caused by dams Vol. 35 (Bethesda, MD: American Fisheries Society Symposium), 255–266.
Fritts A. L., Pearsons T. N. (2004). Smallmouth bass predation on hatchery and wild salmonids in the Yakima River, Washington. Trans. Am. Fisheries Soc. 133 (4), 880–895. doi: 10.1577/T03-003.1
Fritts A., Pearsons T. N. (2006). Effects of predation by nonnative smallmouth bass on native salmonid prey: the role of predator and prey size. Am. Fisheries Soc. 135, 853–860. doi: 10.1577/T05-014.1
Fuiman L. A., Connelly T. L., Lowerre-Barbieri S. K., McClelland J. W. (2015). Egg boons: central components of marine fatty acid food webs. Ecology 96, 362–372. doi: 10.1890/14-0571.1
Gardner C., Coghlan S. M. Jr., Zydlewski J., Saunders R. (2013). Distribution and abundance of stream fishes in relation to barriers: implications for monitoring stream recovery after barrier removal. River Res. Appl. 29 (1), 65–78. doi: 10.1002/rra.1572
Gerard B. (2018). Stream Dynamics in the Headwaters of Post-Glacial Watershed Systems (Orono, ME: The University of Maine).
Gilligan-Lunda E. K., Stich D. S., Mills K. E., Bailey M. M., Zydlewski J. D. (2021). Climate change may cause shifts in growth and instantaneous natural mortality of American Shad throughout their native range. Trans. Am. Fisheries Soc. 150 (3), 407–421. doi: 10.1002/tafs.10299
Glebe B. D., Leggett W. C. (1981). Latitudinal differences in energy allocation and use during the freshwater migrations of American shad (Alosa sapidissima) and their life history consequences. Can. J. Fisheries Aquat. Sci. 38 (7), 806–820. doi: 10.1139/f81-109
Greene K. E., Zimmerman J. L., Laney R. W., Thomas-Blate J. C. (2009). Atlantic coast diadromous fish habitat: a review of utilization, threats, recommendations for conservation, and research needs. Atlantic States Mar. Fisheries Commission Habitat Manage. Ser. 464, 276.
Grote A. B., Bailey M. M., Zydlewski J. D. (2014b). Movements and demography of spawning American Shad in the Penobscot River, Maine, prior to dam removal. Trans. Am. Fisheries Soc. 143 (2), 552–563. doi: 10.1080/00028487.2013.864705
Grote A. B., Bailey M. M., Zydlewski J. D., Hightower J. E. (2014a). Multibeam sonar (DIDSON) assessment of American shad (Alosa sapidissima) approaching a hydroelectric dam. Can. J. Fisheries Aquat. Sci. 71 (4), 545–558. doi: 10.1139/cjfas-2013-0308
Guyette M. Q., Loftin C. S., Zydlewski J. (2013). Carcass analog addition enhances juvenile Atlantic salmon (Salmo salar) growth and condition. Can. J. Fisheries Aquat. Sci. 70, 860–870. doi: 10.1139/cjfas-2012-0496
Guyette M. Q., Loftin C. S., Zydlewski J., Cunjak R. (2014). Carcass analogues provide marine subsidies for macroinvertebrates and juvenile Atlantic salmon in temperate oligotrophic streams. Freshw. Biol. 59, 392–406. doi: 10.1111/fwb.12272
Hagelin A., Museth J., Greenberg L., Kraabøl M., Calles O., Bergman E. (2021). Upstream fishway performance by Atlantic salmon (Salmo salar ) and brown trout ( Salmo trutta ) spawners at complex hydropower dams—Is prior experience a success criterion? Can. J. Fisheries Aquat. Sci. 78 (2), 124–134. doi: 10.1139/cjfas-2019-0271
Halfyard E. A., Webber D., Del Papa J., Leadley T., Kessel S. T., Colborne S. F., et al. (2017). Evaluation of an acoustic telemetry transmitter designed to identify predation events. Methods Ecol. Evol. 8 (9), 1063–1071. doi: 10.1111/2041-210X.12726
Hall C. J., Jordaan A., Frisk. M. G. (2011). The historic influence of dams on diadromous fish habitat with a focus on river herring and hydrologic longitudinal connectivity. Landscape Ecol. 26, 95–107. doi: 10.1007/s10980-010-9539-1
Hall C. J., Jordaan A., Frisk M. G. (2012). Centuries of anadromous forage fish loss: consequences for ecosystem connectivity and productivity. BioScience 62, 723–731. doi: 10.1525/bio.2012.62.8.5
Handeland S. O., Järvi T., Fernö A., Stefansson S. O. (1996). Osmotic stress, antipredatory behaviour, and mortality of Atlantic salmon (Salmo salar) smolts. Can. J. Fisheries Aquat. Sci. 53 (12), 2673–2680. doi: 10.1139/f96-227
Hanson S. D., Curry R. A. (2005). Effects of size structure on trophic interactions between age-0 smallmouth bass and juvenile anadromous alewives. Trans. Am. Fisheries Soc. 134 (2), 356–368. doi: 10.1577/T03-067.1
Hare J. A., Borggaard D. L., Alexander M. A., Bailey M. M., Bowden A. A., Damon-Randall K., et al. (2021). A review of river herring science in support of species conservation and ecosystem restoration. Mar. Coast. Fish. 13, 627–664. doi: 10.1002/mcf2.10174
Haro A., Odeh M., Castro-Santos T., Noreika J. (1999). Effect of slope and headpond on passage of American shad and blueback herring through simple and deepened Alaska steeppass fishways. North Am. J. Fisheries Manage. 19, 51–58. doi: 10.1577/1548-8675(1999)019<0051:EOSAHO>2.0.CO;2
Harper B., Ranco D. (2009). Wabanaki traditional cultural lifeways exposure scenario (Washington, DC: Environmental Protection Agency).
Hart D. D., Johnson T. E., Bushaw-Newton K. L., Horwitz R. J., Bednarek A. T., Charles D. F., et al. (2002). Dam removal: challenges and opportunities for ecological research and river restoration. Bioscience 52, 669–681. doi: 10.1641/0006-3568(2002)052[0669:DRCAOF]2.0.CO;2
Hasselman D. J., Bradford R. G., Bentzen P. (2010). Taking stock: defining populations of American shad (Alosa sapidissima) in Canada using neutral genetic markers. Can. J. Fisheries Aquat. Sci. 67 (6), 1021–1039. doi: 10.1139/F10-031
Hawkes J. P., Goullete G. S., Moctezuma A. M., Atkinson E. J., Cox O. N. (2019). Supplementation of Atlantic salmon in the southern extent of their range: evaluation of age-1 hatchery smolt stocking in a small coastal watershed. North Pacific Anadromous Fish Commission Tech. Rep. No. 15, 197–199. doi: 10.23849/npafctr15/197.199
Heintz R. A., Nelson B. D., Hudson J., Larsen M., Holland L., Wipfli M. (2004). Marine subsidies in freshwater: effects of salmon carcasses on lipid class and fatty acid composition of juvenile coho salmon. Trans. Am. Fisheries Soc. 133, 559–567. doi: 10.1577/T03-035.1
Hershey H. (2021). Updating the consensus on fishway efficiency: A meta-analysis. Fish Fisheries 22 (4), 735–748. doi: 10.1111/faf.12547
Hitt N. P., Eyler S., Wofford J. E. (2012). Dam removal increases American eel abundance in distant headwater streams. Trans. Am. Fisheries Soc. 141 (5), 1171–1179. doi: 10.1080/00028487.2012.675918
Hixon M. A., Johnson D. W., Sogard S. M. (2014). BOFFFFs: on the importance of conserving old-growth age structure in fishery populations. ICES J. Mar. Sci. 71 (8), 2171–2185. doi: 10.1093/icesjms/fst200
Hocking M. D., Reynolds J. D. (2011). Impacts of salmon on riparian plant diversity. Science 331, 1609–1612. doi: 10.1126/science.1201079
Hodge B. W., Wilzbach M. A., Duffy W. G., Quiñones R. M., Hobbs J. A. (2016). Life history diversity in Klamath River steelhead. Trans. Am. Fish. Soc 145 (2), 227–238.
Hogg R., Coghlan S. M. Jr., Zydlewski J. (2013). Anadromous sea lampreys recolonize a Maine coastal river tributary after dam removal. Trans. Am. Fisheries Soc. 142 (5), 1381–1394. doi: 10.1080/00028487.2013.811103
Hogg R. S., Coghlan S. M. Jr., Zydlewski J., Gardner C. (2015). Fish community response to a small-stream dam removal in a maine coastal river tributary. Trans. Am. Fisheries Soc. 144 (3), 467–479. doi: 10.1080/00028487.2015.1007164
Hogg R. S., Coghlan S. M., Zydlewski J., Simon K. S. (2014). Anadromous sea lampreys (Petromyzon marinus) are ecosystem engineers in a spawning tributary. Freshw. Biol. 59, 1294–1307. doi: 10.1111/fwb.12349
Holbrook C. M., Kinnison M. T., Zydlewski J. (2011). Survival of migrating atlantic salmon smolts through the Penobscot River, Maine: A pre-restoration assessment. Trans. Am. Fisheries Soc. 140 (5), 1255–1268. doi: 10.1080/00028487.2011.618356
Holbrook C. M., Zydlewski J., Gorsky D., Shepard S. L., Kinnison M. T. (2009). Movements of pre-spawn adult Atlantic salmon near hydroelectric dams in the lower Penobscot River, Maine. North Am. J. Fisheries Manage. 29 (2), 495–505. doi: 10.1577/M08-042.1
Holyoke (2021). Available at: https://www.bangordailynews.com/2021/07/24/outdoors/heres-some-places-where-you-can-fish-for-striped-bass/.
Isaak D. J., Bjornn T. C. (1996). Movement of northern squawfish in the tailrace of a lower Snake River dam relative to the migration of juvenile anadromous salmonids. Trans. Am. Fisheries Soc. 125 (5), 780–793. doi: 10.1577/1548-8659(1996)125<0780:MONSIT>2.3.CO;2
Izzo L. K., Maynard G. A., Zydlewski J. (2016). Upstream movements of atlantic salmon in the lower Penobscot River, Maine following two dam removals and fish passage modifications. Mar. Coast. Fisheries 8 (1), 448–461. doi: 10.1080/19425120.2016.1185063
Jackson D. C., Davies W. D. (1988). Environmental factors influencing summer angler effort on the Jordan Dam tailwater, Alabama. North Am. J. Fisheries Manage. 8 (3), 305–309. doi: 10.1577/1548-8675(1988)008<0305:EFISAE>2.3.CO;2
Jessop B. M. (2010). Geographic effects on American eel (Anguilla rostrata) life history characteristics and strategies. Can. J. Fisheries Aquat. Sci. 67 (2), 326–346. doi: 10.1139/F09-189
Johnston C., Zydlewski G. B., Smith S., Zydlewski J., Kinnison M. T. (2019). River reach restored by dam removal offers suitable spawning habitat for endangered Shortnose Sturgeon. Trans. Am. Fisheries Soc. 148 (1), 163–175. doi: 10.1002/tafs.10126
Jones K., Cotel A. J. (2023). A hydrodynamics-based framework to evaluate the impact of fishways on drifting lake sturgeon larvae. J. Great Lakes Res. 49 (1), 332–338. doi: 10.1016/j.jglr.2022.11.006
Kadri S., Metcalfe N. B., Huntingford F. A., Thorpe J. E. (1995). What controls the onset of anorexia in maturing adult female Atlantic salmon? Funct. Ecol., 790–797.
Keefer M. L., Taylor G. A., Garletts D. F., Helms C. K., Gauthier G. A., Pierce T. M., et al. (2012). Reservoir entrapment and dam passage mortality of juvenile Chinook salmon in the Middle Fork Willamette River: Chinook salmon entrapment and mortality. Ecol. Freshw. Fish 21 (2), 222–234. doi: 10.1111/j.1600-0633.2011.00540.x
Keefer M. L., Wertheimer R. H., Evans A. F., Boggs C. T., Peery C. A. (2008). Iteroparity in Columbia River summer-run steelhead (Oncorhynchus mykiss): Implications for conservation. Can. J. Fisheries Aquat. Sci. 65 (12), 2592–2605. doi: 10.1139/F08-160
Kiffney P. M., Pess G. R., Anderson J. H., Faulds P., Burton K., Riley S. C. (2009). Changes in fish communities following recolonization of the Cedar River, WA, USA by Pacific salmon after 103 years of local extirpation. River Res. Appl. 25 (4), 438–452. doi: 10.1002/rra.1174
Kiraly I. A., Coghlan S. M. Jr., Zydleswki J., Hayes D. (2014). An assessment of fish assemblage structure in a large river. River Res. Appl. 31 (3), 301–312. doi: 10.1002/rra.2738
Kneeland S. C., Rhymer J. M. (2008). Determination of fish host use by wild populations of rare freshwater mussels using a molecular identification key to identify glochidia. J. North Am. Benthological Soc. 27 (1), 150–160. doi: 10.1899/07-036.1
Knight J. A. (1985). Differential Preservation of Calcified Bone at the Hirundo Site, Alton, Maine (Orono, ME: University of Maine). M.S. Thesis.
Kocik J. F., Hawkes J. P., Sheehan T. F., Music P. A., Beland K. F. (2009). Assessing estuarine and coastal migration and survival of wild Atlantic salmon smolts from the Narraguagus River, Maine using ultrasonic telemetry Vol. 69 (Bethesda, MD: American Fisheries Society Symposium), 293–310.
Kolodny A. (2007). “Rethinking the" Ecological Indian": A penobscot precursor,” in Interdisciplinary Studies in Literature and Environment . (Oxford, UK: Oxford University Press), 1–23.
Kramer G. (2006). Penobscot River Smallmouth Bass Management. Fishery Interim Summary Report Series (No. 06-05) (Augusta, ME: Maine Department of Inland Fisheries and Wildlife). Available at: https://digitalmaine.com/cgi/viewcontent.cgi?article=1114andcontext=ifw_docs.
Landsman S. J., Samways K. M., Hayden B., Knysh K. M., Heuvel M.R.v. d. (2018). Assimilation of marine-derived nutrients from anadromous Rainbow Smelt in an eastern North American riverine food web: evidence from stable-isotope and fatty acid analysis. Freshw. Sci. 37, 747–759. doi: 10.1086/700598
Larkin P. A. (1996). Concepts and issues in marine ecosystem management. Rev. fish Biol. fisheries 6, 139–164. doi: 10.1007/BF00182341
Leggett W. C., Carscadden J. E. (1978). Latitudinal variation in reproductive characteristics of American shad (Alosa sapidissima): evidence for population specific life history strategies in fish. J. Fish Board Canada 35 (11), 1469–1478.
Leonard J. B., McCormick S. D. (1999). Effects of migration distance on whole-body and tissue-specific energy use in American shad (Alosa sapidissima). Can. J. Fisheries Aquat. Sci. 56 (7), 1159–1171. doi: 10.1139/f99-041
Liermann C. R., Nilsson C., Robertson J., Ng R. Y. (2012). Implications of dam obstruction for global freshwater fish diversity. BioScience 62 (6), 539–548. doi: 10.1525/bio.2012.62.6.5
Limburg K. E., Hattala K. A., Kahnle A. (2003). American shad in its native range Vol. 35 (Bethesda, MD: American Fisheries Society Symposium), 125–140.
Limburg K. E., Waldman J. R. (2009). Dramatic declines in North Atlantic diadromous fishes. BioScience 59, 955–965. doi: 10.1525/bio.2009.59.11.7
Loppnow G. L., Vascotto K., Venturelli P. A. (2013). Invasive smallmouth bass (Micropterus dolomieu): history, impacts, and control. Manage. Biol. Invasions 4 (3), 191. doi: 10.3391/mbi.2013.4.3.02
Lotze H. K., Milewski I. (2004). Two centuries of multiple human impacts and successive changes in a North Atlantic food web. Ecol. Appl. 14, 1428–1447.
Ludwig H. R. Jr., Leitch J. A. (1996). Interbasin transfer of aquatic biota via anglers' bait buckets. Fisheries 21 (7), 14–18.
Magilligan F. J., Graber B. E., Nislow K. H., Chipman J. W., Sneddon C. S., Fox C. A. (2016). River restoration by dam removal: Enhancing connectivity at watershed scales. Elementa 4, 000108. doi: 10.12952/journal.elementa.000108
Magoulick D. D. (2004). Effect of predation risk on habitat selection by water column fish, benthic fish and crayfish in stream pools. Hydrobiologia 527, 2009–2221. doi: 10.1023/B:HYDR.0000043302.32382.59
Marschall E. A., Mather M. E., Parrish D. L., Allison G. W., McMenemy J. R. (2011). Migration delays caused by anthropogenic barriers: Modeling dams, temperature, and success of migrating salmon smolts. Ecol. Appl. 21 (8), 3014–3031. doi: 10.1890/10-0593.1
Martin E. H., Apse C. D. (2011). “Northeast aquatic connectivity: An assessment of dams on northeastern rivers,” in The Nature Conservancy, Eastern Freshwater Program (Brunswick, ME).
Master L. L., Flack S. R., Stein B. A. (Eds.) (1998). Rivers of life: critical watersheds for protecting freshwater. Arlington, VA: Nature Conservancy.
Maynard G. A., Izzo L. K., Zydlewski J. D. (2018). Movement and mortality of Atlantic salmon kelts (Salmo salar) released into the Penobscot River, Maine. Fishery Bull. 116 (3-4), 281–291. doi: 10.7755/FB.116.3-4.6
Maynard G. A., Kinnison M. T., Zydlewski J. D. (2017). Size selection from fishways and potential evolutionary responses in a threatened Atlantic salmon population. River Res. Appl. 33 (7), 1004–1015. doi: 10.1002/rra.3155
McCartin K., Jordaan A., Sclafani M., Cerrato R., Frisk M. G. (2019). A new paradigm in Alewife migration: oscillations between spawning grounds and estuarine habitats. Trans. Am. Fisheries Soc. 148 (3), 605–619. doi: 10.1002/tafs.10155
McCormick S. D., Cunjak R. A., Dempson B., O’Dea M. F., Carey J. B. (1999). Temperature-related loss of smolt characteristics in Atlantic salmon (Salmo salar) in the wild. Can. J. Fisheries Aquat. Sci. 56 (9), 1649–1667. doi: 10.1139/f99-099
McCormick S. D., Hansen L. P., Quinn T. P., Saunders R. L. (1998). Movement, migration, and smolting of Atlantic salmon (Salmo salar). Can. J. Fisheries Aquat. Sci. 55 (S1), 77–92. doi: 10.1139/d98-011
McCormick S. D., Lerner D. T., Monette M. Y., Nieves-Puigdoller K., Kelly J. T., Thrandur Björnsson B. (2009). Taking it with you when you go: how perturbations to the freshwater environment, including temperature, dams, and contaminants, affect marine survival of salmon. Am. Fisheries Soc. Symposium 69, 195–214.
McDougall C. A., Anderson W. G., Peake S. J. (2014). Downstream passage of lake sturgeon through a hydroelectric generating station: route determination, survival, and fine-scale movements. N Am. J. Fish Manage 34, 546–558. doi: 10.1080/02755947.2014.892547
McDougall C. A., Blanchfield P. J., Peake S. J., Anderson W. G. (2013). Movement patterns and size-class influence entrainment susceptibility of lake sturgeon in a small hydroelectric reservoir. Tran Am. Fish Soc 142, 1508–1521. doi: 10.1080/00028487.2013.815659
McLaughlin R. L., Smyth E. R., Castro-Santos T., Jones M. L., Koops M. A., Pratt T. C., et al. (2013). Unintended consequences and trade-offs of fish passage. Fish Fisheries 14 (4), 580–604. doi: 10.1111/faf.12003
MDIFW (2023). Available at: https://www.maine.gov/ifw/fishing-boating/fishing/laws-rules/special-laws.html.
Mensinger M. A., Blomberg E. J., Zydlewski J. D. (2021a). The consequences of dam passage for downstream-migrating American eel in the Penobscot River, Maine. Can. J. Fisheries Aquat. Sci. 78 (8), 1181–1192. doi: 10.1139/cjfas-2020-0402
Mensinger M. A., Brehm A. M., Mortelliti A., Blomberg E. J., Zydlewski J. D. (2021b). American eel personality and body length influence passage success in an experimental fishway. J. Appl. Ecol. 58 (12), 2760–2769. doi: 10.1111/1365-2664.14009
Mensinger M., Hawkes J., Goulette G., Mortelliti A., Blomberg E., Zydlewski J. (in press). Dams facilitate predation during Atlantic salmon smolt migration. Can. J. Fish. Aquat. Sci.
Middaugh C. R., Kessinger B., Magoulick D. D. (2016). Climate-induced seasonal changes in smallmouth bass growth rate potential at the southern range extent. Ecol. Freshw. Fish 27 (1), 19–29. doi: 10.1111/eff.12320
Molina-Moctezuma A., Peterson E., Zydlewski J. D. (2021). Movement, survival, and delays of atlantic salmon smolts in the Piscataquis River, maine, USA. Trans. Am. Fisheries Soc. 150 (3), 345–360. doi: 10.1002/tafs.10289
Molina-Moctezuma A., Stich D. S., Zydlewski J. D. (2022). Effects of dam-induced delays on system-wide survival of Atlantic salmon smolts during high-flow, high-survival years in the Penobscot River, Maine, USA. Can. J. Fisheries Aquat. Sci. 79 (12), 2237–2250. doi: 10.1139/cjfas-2022-0055
Moore J. W. (2006). Animal ecosystem engineers in streams. BioScience 56, 237. doi: 10.1641/0006-3568(2006)056[0237:AEEIS]2.0.CO;2
Moore J. W., Yeakel J. D., Peard D., Lough J., Beere M. (2014). Life-history diversity and its importance to population stability and persistence of a migratory fish: steelhead in two large North American watersheds. J. Anim. Ecol. 83 (5), 1035–1046. doi: 10.1111/1365-2656.12212
Moring J. R., Marancik J., Griffiths F. (1995). Changes in stocking strategies for Atlantic salmon restoration and rehabilitation in Maine 1871-1993 Vol. 15 (Bethesda, MD: American Fisheries Society Symposium).
Nelson G. A., Chase B. C., Stockwell J. (2003). Food habits of striped bass (Morone saxatilis) in coastal waters of Massachusetts. J. Northwest Atlantic Fishery Sci. 32, 1–25. doi: 10.2960/J.v32.a1
NMFS (2010). Endangered and threatened wildlife and plants; proposed listing determinations for three distinct population segments of atlantic sturgeon in the northeast region. Federal Register 75 (193), 61872–61904.
Noonan M. J., Grant J. W., Jackson C. D. (2012). A quantitative assessment of fish passage efficiency. Fish Fisheries 13 (4), 450–464. doi: 10.1111/j.1467-2979.2011.00445.x
Norrgård J. R., Greenberg L. A., Piccolo J. J., Schmitz M., Bergman E. (2013). Multiplicative loss of landlocked Atlantic salmon Salmo salar L. smolts during downstream migration through multiple dams. River Res. Appl. 29 (10), 1306–1317. doi: 10.1002/rra.2616
Northcote T. G. (1992). Migration and residency in stream salmonids- some ecological considerations and evolutionary consequences. Nordic J. Freshw. Res. Drottningholm 67, 5–17.
NRCM. (2023). Penobscot River Restoration Project. Natural Resources Council of Maine. Available at: https://www.nrcm.org/programs/waters/penobscot-river-restoration-project/.
Nyqvist D., Greenberg L. A., Goerig E., Calles O., Bergman E., Ardren W. R., et al. (2017). Migratory delay leads to reduced passage success of Atlantic salmon smolts at a hydroelectric dam. Ecol. Freshw. Fish 26 (4), 707–718. doi: 10.1111/eff.12318
Odum H. T. (2007). Environment, power, and society for the twenty-first century: the hierarchy of energy (New York, NY: Columbia University Press).
Ohms H. A., Chargualaf D. N., Brooks G., Hamilton C., Palkovacs E. P., Boughton D. A. (2022). Poor downstream passage at a dam creates an ecological trap for migratory fish. Can. J. Fisheries Aquat. Sci. 79 (12), 2204–2215. doi: 10.1139/cjfas-2022-0095
Oke K. B., Cunningham C. J., Westley P. A. H., Baskett M. L., Carlson S. M., Clark J., et al. (2020). Recent declines in salmon body size impact ecosystems and fisheries. Nat. Commun. 11, 4155. doi: 10.1038/s41467-020-17726-z
Opperman J. J., Royte J., Banks J., Day L. R., Apse C. (2011). The Penobscot River, Maine, USA: A basin-scale approach to balancing power generation and ecosystem restoration. Ecol. Soc. 16 (3), 7. doi: 10.5751/ES-04117-160307
Palkovacs E. P., Dion K. B., Post D. M., Caccone A. (2008). Independent evolutionary origins of landlocked alewife populations and rapid parallel evolution of phenotypic traits. Mol. Ecol. 17 (2), 582–597. doi: 10.1111/j.1365-294X.2007.03593.x
Palkovacs E. P., Post D. M. (2008). Eco-evolutionary interactions between predators and prey: can predator-induced changes to prey communities feedback to shape predator foraging traits? Evolutionary Ecol. Res. 10 (5), 699–720.
Pasha M. F. K., Yeasmin D., Rentch J. M. (1997). Dam-lake operation to optimize fish habitat. Environ. Process 2, 631–645. doi: 10.1007/s40710-015-0106-2
Pess G. R., McHenry M. L., Beechie T. J., Davies J. (2008). Biological impacts of the Elwha River dams and potential salmonid responses to dam removal. Northwest Sci. 82 (sp1), 72–90. doi: 10.3955/0029-344X-82.S.I.72
Petersen J. B., Sanger D. (1986). Archaeological Phase II Testing at the Eddington Bend site (74-8). Penobscot County, Maine (Orono, ME: Bangor Hydro-Electric Company by the University of Maine at Orono).
Peterson E. (2022). The Long-term Impact of Dam Removals on Penobscot River Migratory Fishes (Orono, ME: The University of Maine).
Peterson E., Thors R., Frechette D., Zydlewski J. (2023). Efficiency of adult sea lamprey approach and passage at the Milford Dam fishway, Penobscot River, Maine, United States (North American Journal of Fisheries Management) 43, 1052–1065. doi: 10.1002/nafm.10919
Petticrew E. L., Rex J. F., Albers S. J. (2011). Bidirectional delivery of organic matter between freshwater and marine systems: the role of flocculation in Pacific salmon streams. J. North Am. Benthol. Soc 30 (3), 779–786.
Petts G. E., Morales Y., Sadler J. P. (2006). Linking hydrology and biology in assessing water needs for riverine ecosystems. Hydrological Processes 20 (10), 2247–2251. doi: 10.1002/hyp.6223
Pinfold G. (2011). Economic impact analysis, Dalhousie University (Halifax, Nova Scotia, Canada: Dalhousie University).
Piper A. T., Manes C., Siniscalchi F., Marion A., Wright R. M., Kemp P. S. (2015). Response of seaward-migrating European eel (Anguilla Anguilla) to manipulated flow fields. Proc. R. Soc. B: Biol. Sci. 282 (1811), 20151098. doi: 10.1098/rspb.2015.1098
Poff N. L., Allan J. D., Bain M. B., Karr J. R., Prestegaard K. L., Richter B. D., et al. (1997). The natural flow regime. BioScience 47 (11), 769–784. doi: 10.2307/1313099
Post D. M., Walters A. W. (2009). Nutrient excretion rates of anadromous alewives during their spawning migration. Trans. Am. Fisheries Soc. 138, 264–268. doi: 10.1577/T08-111.1
Poulos H. M., Miller K. E., Kraczkowski M. L., Welchel A. W., Heineman R., Chernoff B. (2014). Fish assemblage response to a small dam removal in the Eightmile River system, Connecticut, USA. Environ. Manage. 54, 1090–1101. doi: 10.1007/s00267-014-0314-y
Powell K., Trial J. G., Dubé N., Opitz M. (1999). External parasite infestation of sea-run Atlantic salmon (Salmo salar) during spawning migration in the Penobscot River, Maine. Northeast. Nat., 363–370.
Quinn T. P., Helfield J. M., Austin C. S., Hovel R. A., Bunn A. G. (2018). A multidecade experiment shows that fertilization by salmon carcasses enhanced tree growth in the riparian zone. Ecology 99, 2433–2441. doi: 10.1002/ecy.2453
Raymond H. L. (1979). Effects of dams and impoundments on migrations of juvenile chinook salmon and steelhead from the Snake river 1966 to 1975. Trans. Am. Fisheries Soc. 108 (6), 505–529. doi: 10.1577/1548-8659(1979)108<505:EODAIO>2.0.CO;2
Reid S. B., Goodman D. H. (2020). Natural recolonization by Pacific lampreys in a Southern California coastal drainage: Implications for their biology and conservation. North Am. J. Fisheries Manage. 40 (2), 335–341. doi: 10.1002/nafm.10412
Ricciardi A., Rasmussen J. B. (1999). Extinction rates of North American freshwater fauna. Conserv. Biol. 13 (5), 1220–1222. doi: 10.1046/j.1523-1739.1999.98380.x
Rieman B. E., Beasmesderfer R. C. (1991). Estimated loss of juvenile salmonids to predation by northern squawfish, walleyes and smallmouth bass in john day reservoir, Columbia river. Trans. Am. Fisheries Soc. 120, 448–458. doi: 10.1577/1548-8659(1991)120<0448:ELOJST>2.3.CO;2
Roscoe D. W., Hinch S. G., Cooke S. J., Patterson D. A. (2011). Fishway passage and post-passage mortality of up-river migrating sockeye salmon in the Seton River, British Columbia. River Res. Appl. 27 (6), 693–705. doi: 10.1002/rra.1384
Ross D. A., Follen D. G. Sr. (1988). Bald eagles wintering at the Petenwell Dam, Wisconsin. Passenger Pigeon 50 (2), 99–106.
Roy S. G., Daigneault A., Zydlewski J., Truhlar A., Smith S., Jain S., et al. (2020). Coordinated river infrastructure decisions improve net social-ecological benefits. Environ. Res. Lett. 15 (10), 104054. doi: 10.1088/1748-9326/abad58
Roy S. G., Uchida E., de Souza S. P., Blachly B., Fox E., Gardner K., et al. (2018). A multiscale approach to balance trade-offs among dam infrastructure, river restoration, and cost. Proc. Natl. Acad. Sci. 115 (47), 12069–12074. doi: 10.1073/pnas.1807437115
Rubenstein S. R., Peterson E., Christman P., Zydlewski J. D. (2023). Adult Atlantic salmon (Salmo salar) delayed below dams rapidly deplete energy stores. Can. J. Fisheries Aquat. Sci. 80 (1), 170–182. doi: 10.1139/cjfas-2022-0008
Ruggerone G. T. (1986). Consumption of migrating juvenile salmonids by gulls foraging below a Columbia River dam. Trans. Am. Fisheries Soc. 115 (5), 736–742. doi: 10.1577/1548-8659(1986)115<736:COMJSB>2.0.CO;2
Ruggles C. P. (1980). A review of the downstream migration of Atlantic salmon. Can. Tech. Rep. Fish. Aquat. Sci. 952, 1–39.
Saboret G., Buckle D. J., King A. J., Douglas M. M., Crook D. A. (2021). Partial migration in diadromous fishes drives the allocation of subsidies across the freshwater-marine ecotone. Anim. Migrations 8, 40–55. doi: 10.1515/ami-2020-0108
Satterthwaite W. H., Beakes M. P., Collins E. M., Swank D. R., Merz J. E., Titus R. G., et al. (2009). Steelhead life history on California's central coast: insights from a state-dependent model. Trans. Am. Fisheries Soc. 138 (3), 532–548. doi: 10.1577/T08-164.1
Saunders R., Hachey M. A., Fay C. W. (2006). Maine’s diadromous fish community: past, present, and implications for Atlantic salmon recovery. Fisheries 31, 537–547. doi: 10.1577/1548-8446(2006)31[537:MDFC]2.0.CO;2
Savoy T. F., Crecco V. A. (2004). Factors affecting the recent decline of blueback herring and American shad in the Connecticut River. Am. Fisheries Soc. Monograph 9, 361–377.
Scherelis C., Zydlewski G. B., Brady D. C. (2020). Using hydroacoustics to relate fluctuations in fish abundance to river restoration efforts and environmental conditions in the Penobscot River, Maine. River Res. Applications 36(2) pp, 234–246. doi: 10.1002/rra.3560
Schindler D. E., Scheuerell M. D., Moore J. W., Gende S. M., Francis T. B., Palen W. J. (2003). Pacific salmon and the ecology of coastal ecosystems. Front. Ecol. Environ. 1, 31–37. doi: 10.1890/1540-9295(2003)001[0031:PSATEO]2.0.CO;2
Schultz A. A., Afentoulis V. B., Yip C. J., Johnson M. N. (2017). Efficacy of an acoustic tag with predation detection technology. North Am. J. Fisheries Manage. 37 (3), 574–581. doi: 10.1080/02755947.2017.1290720
Scruton D. A., Pennell C., Ollerhead L. M. N., Alfredsen K., Stickler M., Harby A., et al. (2008). A synopsis of “hydropeaking” studies on the response of juvenile Atlantic salmon to experimental flow alteration. Hydrobiologia 609, 263–275. doi: 10.1007/s10750-008-9409-x
Sharma S., Waldman J. (2021). Potential solar replacement of hydroelectricity to reopen rivers: Maine as a case example. Fisheries 46 (8), 383–390. doi: 10.1002/fsh.10619
Shrimpton J. M., Zydlewski J. D., McCormick S. D. (2001). The Stress Response of Juvenile American Shad to Handling and Confinement is Greater during Migration in Freshwater than in Seawater. Trans. Am. Fisheries Soc. 130 (6), 1203–1210. doi: 10.1577/1548-8659(2001)130<1203:TSROJA>2.0.CO;2
Sigourney D. B., Zydlewski J. D., Hughes E., Cox O. (2015). Transport, dam passage, and size selection of adult Atlantic salmon in the Penobscot River, Maine. North Am. J. Fisheries Manage. 35 (6), 1164–1176. doi: 10.1080/02755947.2015.1099578
Song C., Omalley A., Roy S. G., Barber B. L., Zydlewski J., Mo W. (2019). Managing dams for energy and fish tradeoffs: what does a win-win solution take? Sci. Total Environ. 669, 833–843. doi: 10.1016/j.scitotenv.2019.03.042
Speck F. G. (1940). Penobscot man: The life history of a forest tribe in Maine. (Philadelphia, PA: University of Pennsylvania Press), 325.
Spicer A. V., Moring J. R., Trial J. G. (1995). Downstream migratory behavior of hatchery-reared, radio-tagged Atlantic salmon (Salmo salar) smolts in the Penobscot River, Maine, USA. Fisheries Res. 23 (3–4), 255–266. doi: 10.1016/0165-7836(94)00352-W
Stich D. S., Bailey M. M., Holbrook C. M., Kinnison M. T., Zydlewski J. D. (2015a). Catchment-wide survival of wild-and hatchery-reared Atlantic salmon smolts in a changing system. Can. J. Fisheries Aquat. Sci. 72 (9), 1352–1365. doi: 10.1139/cjfas-2014-0573
Stich D. S., Bailey M. M., Zydlewski J. D. (2014). Survival of Atlantic salmon Salmo salar smolts through a hydropower complex. J. Fish Biol. 85 (4), 1074–1096. doi: 10.1111/jfb.12483
Stich D. S., Kinnison M. T., Kocik J. F., Zydlewski J. D. (2015b). Initiation of migration and movement rates of Atlantic salmon smolts in fresh water. Can. J. Fisheries Aquat. Sci. 72 (9), 1339–1351. doi: 10.1139/cjfas-2014-0570
Stich D. S., Sheehan T. F., Zydlewski J. D. (2019). A dam passage performance standard model for American shad. Can. J. Fisheries Aquat. Sci. 76 (5), 762–779. doi: 10.1139/cjfas-2018-0008
Stich D. S., Zydlewski G. B., Zydlewski J. (2015c). Effects of physiological preparedness and saltwater tolerance on behavioural preferences and thresholds. Migration. J. Fish Biol. 88 (2), 595–617. doi: 10.1111/jfb.12853
Tabor R. A., Shively R. S., Poe. T. P. (1993). Predation on Juvenile Salmonids by Smallmouth Bass and sic [Northern Squawfish] in the Columbia River near Richmond, Washington. North Am. J. Fisheries Manage. 13, 831–838. doi: 10.1577/1548-8675(1993)013<0831:POJSBS>2.3.CO;2
Thorpe J. E., Mangel M., Metcalfe N. B., Huntingford F. A. (1998). Modelling the proximate basis of salmonid life-history variation, with application to Atlantic salmon, Salmo salar L. Evolutionary Ecol. 12, 581–599. doi: 10.1023/A:1022351814644
Thorstad E. B., Fleming I. A., McGinnity P., Soto D., Wennevik V., Whoriskey F. (2008). Incidence and impacts of escaped farmed Atlantic salmon Salmo salar in nature. NINA Special Report 36, 114 pp.
Trent L., Hassler W. W. (1966). Feeding behavior of adult striped bass, Roccus saxatilis, in relation to stages of sexual maturity. Chesapeake Sci. 7 (4), 189–192. doi: 10.2307/1350432
Trinko Lake T. R., Ravana K. R., Saunders R. (2012). Evaluating changes in diadromous species distributions and habitat accessibility following the Penobscot River Restoration Project. Mar. Coast. Fisheries 4 (1), 284–293. doi: 10.1080/19425120.2012.675971
Trotter P. C. (1989). Coastal cutthroat trout: a life history compendium. Trans. Am. Fisheries Soc. 118 (5), 463–473. doi: 10.1577/1548-8659(1989)118<0463:CCTALH>2.3.CO;2
Twining C. W., Palkovacs E. P., Friedman M. A., Hasselman D. J., Post D. M. (2017). Nutrient loading by anadromous fishes: species-specific contributions and the effects of diversity. Can. J. Fisheries Aquat. Sci. 74, 609–619. doi: 10.1139/cjfas-2016-0136
USACE. (2023). National Inventory of Dams. US Army Corps of Engineers. Available at: https://nid.sec.usace.army.mil/ (Accessed 15 January 2020).
USFWS, NMFS. (2018). Recovery Plan for the Gulf of Maine Distinct Population Segment of Atlantic Salmon (Salmo salar). (East Orland, ME: U.S. Fish and Wildlife Service), 74pp.
Vannote R. L., Sweeney B. W. (1980). Geographic analysis of thermal equilibria: a conceptual model for evaluating the effect of natural and modified thermal regimes on aquatic insect communities. Am. Nat. 115 (5), 667–695.
Verdon R., Desrochers D. (2002). “Upstream migratory movements of American eel (Anguilla rostrata) between the Beauhornois and Moses-Saunders power dams on the St. Lawrence River,” in Biology, management, and protection of Catadromous Eels. Ed. Dixon D.A. (Bethesda, MD: American Fishery Society Symposium) 33, pp. 139-151.
Vogel S., Jansujwicz J. (2022). Navigating fish passage decisions during regulatory dam relicensing in Maine. Fisheries Manage. Ecol. 29 (1), 69–87. doi: 10.1111/fme.12513
Walburg C. H., Nichols P. R. (1967). Biology and management of the American shad and status of the fisheries, Atlantic coast of the United States 1960(No. 550) (Bureau of Commercial Fisheries: US Department of the Interior).
Waldman J. R., Quinn T. P. (2022). North American diadromous fishes: Drivers of decline and potential for recovery in the Anthropocene. Sci. Adv. 8, eabl5486. doi: 10.1126/sciadv.abl5486
Walter J. F., Austin H. M. (2003). Diet composition of large striped bass (Morone saxatilis) in Chesapeake Bay. Fishery Bull. 101 (2), 414.
Wang C. H., Tzeng W. N. (1998). Interpretation of geographic variation in size of American eel Anguilla rostrata elvers on the Atlantic coast of North America using their life history and otolith ageing. Mar. Ecol. Prog. Ser. 168, 35–43. doi: 10.3354/meps168035
Ward J. V., Stanford J. A. (1987). “The ecology of regulated streams: past accomplishments and directions for future research,” in Regulated streams: advances in ecology (Boston, MA: Springer US), 391–409.
Watson J. M., Coghlan S. M. Jr., Zydlewski J., Hayes D. B., Kiraly I. A. (2018). Dam removal and fish passage improvement influence fish assemblages in the Penobscot River, Maine. Trans. Am. Fisheries Soc. 147 (3), 525–540. doi: 10.1002/tafs.10053
Watson J. M., Coghlan S. M. Jr., Zydlewski J., Hayes D. B., Stich D. S. (2019). “Role of recovering river herring Alosa spp,” in Population on Smallmouth Bass Diet and Growth. Am. Fish. Soc. Symp. (Bathesda MD: American Fisheries Society), 87.
Weaver D. M., Brown M., Zydlewski J. D. (2019). Observations of American Shad Alosa sapidissima Approaching and Using a Vertical Slot Fishway at the Head-of-Tide Brunswick Dam on the Androscoggin River, Maine. North Am. J. Fisheries Manage. 39 (5), 989–998. doi: 10.1002/nafm.10330
Weaver D. M., Coghlan S. M., Greig H. S., Klemmer A. J., Perkins L. B., Zydlewski J. (2018b). Subsidies from anadromous sea lamprey (Petromyzon marinus) carcasses function as a reciprocal nutrient exchange between marine and freshwaters: Cross-ecosystem sea lamprey subsidies. River Res. Applications. 34, 824–833. doi: 10.1002/rra.3291
Weaver D. M., Coghlan S. M., Zydlewski J. (2016). Sea lamprey carcasses exert local and variable food web effects in a nutrient-limited Atlantic coastal stream. Can. J. Fisheries Aquat. Sci. 73, 1616–1625. doi: 10.1139/cjfas-2015-0506
Weaver D. M., Coghlan S. M. Jr., Zydlewski J. (2018a). The influence of nutrients from carcasses of sea lamprey (Petromyzon marinus) on larval growth and spawner abundance. FB 116, 142–152. doi: 10.7755/FB.116.2.3
Weaver D. M., Coghlan S. M., Zydlewski J. (2018c). Effects of sea lamprey substrate modification and carcass nutrients on macroinvertebrate assemblages in a small Atlantic coastal stream. J. Freshw. Ecol. 33, 19–30. doi: 10.1080/02705060.2017.1417168
Weaver D. M., Coghlan S. M., Zydlewski J., Hogg R. S., Canton M. (2015). Decomposition of sea lamprey Petromyzon marinus carcasses: temperature effects, nutrient dynamics, and implications for stream food webs. Hydrobiologia 760, 57–67. doi: 10.1007/s10750-015-2302-5
Weigel D. E., Connolly P. J., Martens K. D., Powell M. S. (2013). Colonization of steelhead in a natal stream after barrier removal. Trans. Am. Fisheries Soc. 142 (4), 920–930. doi: 10.1080/00028487.2013.788560
Wertheimer R. H., Evans A. F. (2005). Downstream passage of steelhead kelts through hydroelectric dams on the lower snake and Columbia rivers. Trans. Am. Fisheries Soc. 134 (4), 853–865. doi: 10.1577/T04-219.1
Whiteley A. R., Hastings K., Wenburg J. K., Frissell C. A., Martin J. C., Allendorf F. W. (2010). Genetic variation and effective population size in isolated populations of coastal cutthroat trout. Conserv. Genet. 11, 1929–1943.
Whittum K. A. (2022). Evaluation of Fish Assemblage Composition and the Expansion of an Invasive Species Following Dam Removal and Upgraded Fish Passage in the Penobscot River, Maine (Orono, ME: The University of Maine).
Whittum K., Zydlewski J., Coghlan S. Jr, Hayes D., Watson J., Kiraly I. (2023). Fish assemblages in the Penobscot river: A decade after dam removal. Mar. Coast. Fisheries 15, e10227. doi: 10.1002/mcf2.10227
Wilcove D. S. (2010). No way home: the decline of the world's great animal migrations (Washington, DC, United States: Island Press).
Willis T. V., Wilson K. A., Johnson B. J. (2017). Diets and stable isotope derived food web structure of fishes from the inshore gulf of maine. Estuaries Coasts 40, 889–904. doi: 10.1007/s12237-016-0187-9
Wilson K., Veneranta L. (2019). Data-limited diadromous species – review of European status. ICES Coop. Res. Rep (CRR).
Wilson S., Patterson D., Moore J. (2022). Intra- and inter-population variation in sensitivity of migratory sockeye salmon smolts to phenological mismatch. Mar. Ecol. Prog. Ser. 692, 119–136. doi: 10.3354/meps14070
Wipfli M. S., Hudson J. P., Caouette J. P., Mitchell N. L., Lessard J. L., Heintz R. A., et al. (2010). Salmon carcasses increase stream productivity more than inorganic fertilizer pellets: A test on multiple trophic levels in streamside experimental channels. Trans. Am. Fisheries Society. 139, 824–839. doi: 10.1577/T09-114.1
Wippelhauser G. (2021). Recovery of diadromous fishes: A Kennebec River case study. Trans. Am. Fisheries Soc. 150 (3), 277–290. doi: 10.1002/tafs.10292
WWF (World Wildlife Fund) (2022). Living Planet Report 2022 – Building a Nature-Positive society. Eds. Almond R. E. A., Grooten M., Bignoli D. J., Petersen D. T. (WWF, Gland). Available at: https:/livingplanet.panda.org. (Accessed 7 October 2023)
Zarri L. J., Palkovacs E. P., Post D. M., Therkildsen N. O., Flecker A. S. (2022). The evolutionary consequences of dams and other barriers for riverine fishes. BioScience 72 (5), 431–448. doi: 10.1093/biosci/biac004
Závorka L., Blanco A., Chaguaceda F., Cucherousset J., Killen S. S., Liénart C., et al. (2023). The role of vital dietary biomolecules in eco-evo-devo dynamics. Trends Ecol. Evol. 38, 72–84. doi: 10.1016/j.tree.2022.08.010
Zydlewski G. B., Haro A., McCormick S. D. (2005). Evidence for cumulative temperature as an initiating and terminating factor in downstream migratory behavior of Atlantic salmon (Salmo salar) smolts. Can. J. Fisheries Aquat. Sci. 62 (1), 68–78. doi: 10.1139/f04-179
Zydlewski J., McCormick S. D. (1997a). The ontogeny of salinity tolerance in the American shad, Alosa sapidissima. Can. J. Fisheries Aquat. Sci. 54 (1), 182–189. doi: 10.1139/f96-251
Zydlewski J., McCormick S. D. (1997b). The loss of hyperosmoregulatory ability in migrating juvenile American shad, Alosa sapidissima. Can. J. Fisheries Aquat. Sci. 54 (10), 2377–2387. doi: 10.1139/f97-144
Zydlewski J., McCormick S. D., Kunkel J. G. (2003). Late migration and seawater entry is physiologically disadvantageous for American shad juveniles: seawater entry and physiology in American shad. J. Fish Biol. 63 (6), 1521–1537. doi: 10.1111/j.1095-8649.2003.00264.x
Zydlewski J., Stich D. S., Roy S., Bailey M., Sheehan T., Sprankle K. (2021). What have we lost? Modeling dam impacts on American shad populations through their native range. Front. Mar. Sci. 8. doi: 10.3389/fmars.2021.734213
Zydlewski J., Wilkie M. P. (2012). “Freshwater to seawater transitions in migratory fishes,” in Fish physiology, vol. 32. (Cambridge, MA: Academic Press), 253–326.
Keywords: fish passage, dam, river, restoration, migration, anadromous, catadromous
Citation: Zydlewski J, Coghlan S, Dillingham C, Figueroa-Muñoz G, Merriam C, Smith S, Smith R, Stich D, Vogel S, Wilson K and Zydlewski G (2023) Seven dam challenges for migratory fish: insights from the Penobscot River. Front. Ecol. Evol. 11:1253657. doi: 10.3389/fevo.2023.1253657
Received: 05 July 2023; Accepted: 29 September 2023;
Published: 16 October 2023.
Edited by:
Rebecca McCaffery, United States Department of the Interior, United StatesReviewed by:
Keith Nislow, Forest Service (USDA), United StatesWilliam Wyatt Hoback, Oklahoma State University, United States
Copyright © 2023 Zydlewski, Coghlan, Dillingham, Figueroa-Muñoz, Merriam, Smith, Smith, Stich, Vogel, Wilson and Zydlewski. This is an open-access article distributed under the terms of the Creative Commons Attribution License (CC BY). The use, distribution or reproduction in other forums is permitted, provided the original author(s) and the copyright owner(s) are credited and that the original publication in this journal is cited, in accordance with accepted academic practice. No use, distribution or reproduction is permitted which does not comply with these terms.
*Correspondence: Joseph Zydlewski, am9zZXBoekBtYWluZS5lZHU=