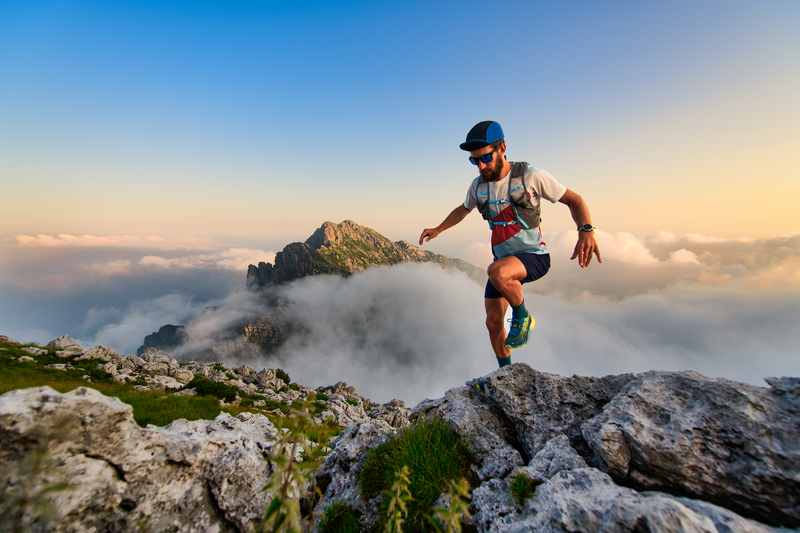
95% of researchers rate our articles as excellent or good
Learn more about the work of our research integrity team to safeguard the quality of each article we publish.
Find out more
ORIGINAL RESEARCH article
Front. Ecol. Evol. , 24 November 2023
Sec. Conservation and Restoration Ecology
Volume 11 - 2023 | https://doi.org/10.3389/fevo.2023.1246822
This article is part of the Research Topic Regeneration Mechanisms and Tradeoffs of Ecosystem Function after Drastic Environmental Changes View all 7 articles
Mining activities, while providing a huge material base for human society, have also caused great damage to the ecosystem. A large amount of mine waste is in urgent need of treatment and remediation. Phytoremediation, as a green and low-cost way of mine site restoration, has been researched by a large number of scholars. Ecological restoration, as a suitable alternative to phytoremediation, has also received extensive attention from scholars too. Field survey revealed that a native plant, Coriaria nepalensis, adapted to the abandoned sites of Pb-Zn mines for its adaptability to pollution and extreme habitats and its improvement of the surrounding microenvironment, with its formation of plant communities may contribute to the natural recovery of the abandoned sites of mines. For this reason, the present study was conducted on the nurse plant, C. nepalensis, which was naturally colonized in the abandoned land of the Pb-Zn mine in Mine Town, Huize County. The specific results of the study are as follows: Coriaria nepalensis promotes the stabilization of the soil structure under the canopy, and the local resources of the soil increase and the “fertilizer island” effect appears: (1) Improvement of physical properties: Compared with the herbaceous sample, the soil bulk density of the Coriaria nepalensis is significantly lower than that of the herbaceous sample. (2) Improve soil nutrition: the organic matter, total nitrogen and total phosphorus contents of the inter-root soil of the Coriaria nepalensis in large multi-diversity sites were higher than those of the herbaceous sample sites. (3) Reducing the toxicity of soil heavy metals to plants: although the total amount of heavy metals and the effective state of the Coriaria nepalensis were significantly higher than that of the herbaceous samples, the diversity and biomass of the plants under the Coriaria nepalensis were not affected, but were higher instead, which indicated that the Coriaria nepalensis mitigated the stress and toxicity of the heavy metals to the plants under the canopy, and allowed the plants to colonize and grow under the canopy. (4) Coriaria nepalensis in Pb-Zn mine abandoned sites can regulating soil microbial community structure, thus enabling plant community succession in degraded environments. Ascomycetes, Mycobacteriophages, Ascomycetes, and Stramenophages with higher abundance. (5) Coriaria nepalensis microbial community structure and increases the abundance of functions associated with nitrogen cycling and stress tolerance. There were higher abundances of bacterial functions related to nitrogen fixation, nitrate reduction, nitrogen respiration, nitrate respiration; and higher abundances of stress-tolerant, parthenogenetic anaerobic, biofilm-forming, aerobic, mobile protozoa-containing, and Gram-negative bacteria in the Coriaria nepalensis. In sum: C. nepalensis can have a nurse effect on its sub-canopy plants by improving microhabitat soil properties and regulating soil microbial community structure in abandoned sites of Pb-Zn mines, thus enabling plant community succession in degraded environments.
● Coriaria nepalensis has a good nurse effect on plants and can help other plant species to colonize under its canopy.
● Coriaria nepalensis promotes the stabilization of the soil structure under the canopy, and the local resources of the soil increase and the “fertilizer island” effect appears.
● Coriaria nepalensis in Pb-Zn mine abandoned sites can regulating soil microbial community structure, thus enabling plant community succession in degraded environments.
Mining activities provide basic physical energy security for humans and are one of the key sources of global economic growth (Zhu et al., 2018). Globally, approximately 350 × 109 tons of mining waste will be generated annually (Adiansyah et al., 2016). The country has maintained a dominant position in global mineral resource production and consumption (Pokhrel and Dubey, 2013). Mineral resources as an important material basis for economic development, the development and utilization of mining areas is extremely important for the modernization of China (Pan et al., 2014). Yunnan is known as the “kingdom of non-ferrous metals”, and 143 kinds of minerals have been discovered in Yunnan, accounting for 83% of the discovered minerals in China, including lead and zinc, which are among the most abundant in China and the world (Li, 2006). Among them, the Pb-Zn mining area in Huize County, which has the richest Pb-Zn ore taste in China, has a history of zinc refining for hundreds of years (Lei and Duan, 2008). As a famous collection and distribution area for clay-based Zn refining in China, most of the mining processes of Pb-Zn mines in Huize are characterized by large-scale mining of metal deposits with high grade and associated useful elements; long mining history; and low metal recovery and comprehensive utilization, so many mining abandoned sites leave a large amount of tailings and abandoned low-grade ores, which make the release of toxic and harmful heavy metal elements in them (Li et al., 2014; Chen et al., 2022), thus causing non-negligible secondary pollution to the local ecological environment.
Mining activities in Pb-Zn mines have led to the accumulation and gradual diffusion of large amounts of toxic and hazardous substances into the environment, making mine waste sites a source of toxic and hazardous heavy metal elements, thus causing different levels of pollution (Rieuwerts et al., 2014). Soil acidification due to the massive export of potentially toxic elements and the oxidation of sulfides in mine spoils has resulted in reduced turnover of organic matter, increased soil toxicity, and loss of biodiversity in mine soils (Rodriguez-Seijo et al., 2020), and has caused soil degradation, lack of vegetation cover, soil structural damage, poor soil and water conservation, high soil heavy metal content, and soil nutrient depletion in mine waste sites (Mi et al., 2019). and soil nutrient depletion (Mi et al., 2019). Heavy metal contamination of abandoned sites in Pb-Zn mines is a serious hazard to plants, animals, microorganisms and humans (Cui et al., 2020). Meanwhile, these extreme environments can also prevent natural plant recolonization, which can lead to ecological damage (Stylianou et al., 2020; Li et al., 2022). Therefore, heavy metal contamination of abandoned sites in Pb-Zn mines has caused serious harm to flora and fauna, ecosystems and human health, and the treatment and remediation of abandoned sites should be carried out without delay.
In recent years, in order to improve the ecological environment of mining areas, a large number of research on mine remediation technologies have been carried out, and the main common methods of heavy metal remediation of mine waste sites are physical remediation, chemical remediation, waste site reuse and bioremediation (Mahar et al., 2016; Wei et al., 2021). However, most of the above restoration methods are costly, and a low-cost restoration method becomes the key to mine restoration at this time due to the large number of mine abandoned sites in China, the remote location of mine abandoned sites, and their low economic value. Phytoremediation method is widely suggested by many as a low cost method suitable for general promotion (Mahar et al., 2016; Khalid et al., 2017). Ecological restoration, as a suitable alternative to phytoremediation, has also received extensive attention from scholars too. Plant species selection is the key to the success of ecological restoration in mine waste sites (Chen et al., 2020). Conventional ecological restoration methods are to screen hyperaccumulator plants for large-scale planting, but hyperaccumulator plants generally have problems such as being confined to their native habitat, shallow roots, slow growth, small biomass, and metal selectivity (van der Ent et al., 2013; Sheoran et al., 2016), and are difficult to colonize mine waste sites. Even if it is colonized, its ability to form a long-term stable community and to continue succession has not been demonstrated (Saxena et al., 2020). In the past few years, the selection of ecological remediation plants has evolved from hyperaccumulation plants to native plant screening, but more studies have focused only on the uptake, transfer and accumulation effects of native plants on heavy metals and the short-term improvement of the soil environment, never on their effects on plant survival, plant community formation and plant colonization, and plant succession factors. Gastauer et al. (2018) said that perhaps native nurse plants can have greater ecological benefits relative to hyperaccumulation plants in extremely degraded environments such as mine waste sites. Nurse plants are stress-resistant species that not only grow rapidly in extremely degraded environments, but also trigger the restoration of essential ecosystem functions through ecological facilitation. In this study, a field survey at the abandoned site of Pb-Zn mine in Mine Town, Huize County, revealed that a native plant, Coriaria nepalensis, which is adapted to the abandoned site of Pb-Zn mine, may contribute to the natural restoration of the abandoned site of the mine by its adaptation to pollution and extreme habitats and its improvement of the surrounding microenvironment by forming a plant community. To this end, this study was conducted to investigate the effects of C. nepalensis on soil physicochemical properties, soil heavy metal storage characteristics, soil microbial community and plant colonization under the canopy under different years of abandonment by using field plant sample survey and soil physicochemical property determination. We also investigated the effects of C. nepalensis on soil physical and chemical properties, soil heavy metal fugacity, soil microbial community and under-canopy planting under different years of abandonment. It aims to provide a theoretical basis for restoration of abandoned land in Pb and Zn mining areas through ecological restoration.
Study area is located in the lead-zinc mining area of Mine Town, Huize County, Qujing City, Yunnan Province (103.70° E, 26.64° N, altitude 2500 m), which belongs to the Wumeng Mountain System. The region has a temperate highland monsoon climate with abundant rainfall, mild climate and unknown seasons, but a clear division between rainy and dry seasons. The average annual rainfall is 858.4 mm and the average annual temperature is 12.6°C. This Pb-Zn mining area is the richest area in China in terms of Pb-Zn ore taste and has a history of zinc refining for hundreds of years (Zhou et al., 2018), and it is a famous collection and distribution area for clay-based zinc refining in China, which is now a mine abandoned area with sparse vegetation. The area is dominated by yellow-brown soils with weakly alkaline soils, and the main vegetation types are herbs and shrubs (Zhou et al., 2018), and the study area and sampling sites are shown in Figure 1.
Figure 1 Geographical location of study sites (Drawing approval number is GS(2016)1600.), S1: 10 years of abandonment; S2: 20 years of abandonment; S3: 30 years of abandonment; S4: four types of study sites undisturbed by mining.
In early September 2021, a field survey was conducted on abandoned sites of Pb-Zn mines in mining town of Huize County: representative sample sites of Coriaria nepalensis were selected, and the soil heavy metal element range of each sample site was delineated by a portable soil heavy metal analyzer and based on the plant inspection of each sample site and the narrative of the mine management personnel, etc., four (> 7 ha) different sample sites of C. nepalensis were selected for this study, representing four types of study sites that were abandoned for 10 years, abandoned for 20 years, abandoned for 30 years, and disturbed by mining, respectively. The four sample plots are described in detail as follows: abandoned 10-year sample plot (20 ha); abandoned 20-year sample plot (12 ha); abandoned 30-year sample plot (15 ha); and undisturbed by mining sample plot (7 ha). These four plots were identified as the study plots (Figure 1), labeled S1, S2, S3, and S4, respectively, and were selected based on the following criteria: similar topography and slope < 3%, distance between any two plots > 2 km, and distance between any two plots within 5 km for similar topography and soil types and similar climatic conditions.
Five C. nepalensis plants of similar size (C. nepalensis canopy diameter of about 1.5~2 m) were randomly selected in each sample site. Each C. nepalensis plant was used as a small sample point for the study, totaling 20 sample points. A sample square (3 m × 3 m) was set up at each sample site with the C. nepalensis as the geometric center, and there were five sample squares in each sample site. Five herbaceous samples were randomly selected in the area without C. nepalensis around the sample plots, and five herbaceous samples (3 m × 3 m) were set up (Figure 2), for a total of 40 samples. The cover, plant height, vegetation cover, name of herbaceous species, number of species, frequency of each sample, and the cover and height of five randomly selected plants of each species in the sample were counted and taken back to the laboratory for biomass determination. Plant names and species delineation were identified by consulting the Yunnan Central Wild Plant Manual and seeking assistance from a plant taxonomist. Soil sample collection: Soil samples were collected from 0–45 cm of the C. nepalensis sample (Y) and the herbaceous samples (N) using the five-point sampling method, and stored in two parts: one in a −80°C refrigerator for soil microbiological determination, and one for natural air-drying for the study of soil physical and chemical properties and heavy metal distribution under C. nepalensis.
Figure 2 Plant Sample Layout, S1: 10 years of abandonment; S2: 20 years of abandonment; S3: 30 years of abandonment; S4: four types of study sites undisturbed by mining.
We measured and analyzed 40 soil samples collected for soil bulk density (SBD), total nitrogen (TN), organic matter (SOM), total phosphorus (TP), and soil heavy metals (TPb, TZn, TCd, TMn). Soil bulk density (SBD, g/cm3) was determined by the ring-knife method (Marc and Jacques, 2006). Total soil carbon and total nitrogen (TC/TN, g/kg) were determined by combustion method (Zhang and Elser, 2017) using a vario TOC select type total organic carbon analyzer (Elementar Analysensysteme GmbH, Germany), and soil organic matter (SOM, g/kg) was derived by total soil nitrogen conversion. Soil organic matter (SOM, g/kg) was obtained by total soil nitrogen conversion. Soil total phosphorus (TP, g/kg) was determined by the sulfuric acid digestion-molybdenum antimony resistance method (Zhang et al., 2013). Total heavy metals in soil samples were determined by flame atomic absorption spectrometry (Moyan et al., 2017). The samples were determined on a flame atomic absorption spectrometer (Agilent AA240 spectrometer, Agilent Technologies, CA, USA).
Total DNA was extracted from 0.5 g of soil sample per tube, and the composition of soil bacterial, fungal and actinomycete communities was analyzed using high-throughput sequencing (Li et al., 2023).
Amplified by PCR using primers 338F: (5’-ACTCCTACGGGGAGGCAGCA-3’)/806R: (5’-GGACTACHVGGGGTWTCTAAT-3’) for the V3+V4 region of the bacterial 16S rRNA gene.
Fungi PCR amplification of the ITS1 region of the fungal ITS rRNA gene was performed using the primer ITS1F: (5’-CTTGGTCATTTAGAGGAAGTAA-3’)/ITS2: (5’-GCTGCGTTCTTCATCGATGC-3’).
Actinomycetes were amplified by PCR using primer 960F: (GGCTTAATTTGACTCAACRCG) NSR1438: (GGGCATCACAGACCTGTTAT) for the V7 region of the Actinomycetes 18S rRNA gene.
The qualified products were subjected to Illumina Miseq sequencing, which was entrusted to Beijing Baimike Biotechnology Co. The measured data (Raw Reads) were filtered by Trimmomatic v 0.33 software, primer identification and removal were performed by cutadapt1.9.1 software, followed by splicing of the samples by over lap and length filtering by Usearch v10 software, and finally denoising and removal of the chimeric sequences were performed by QIIME2 2020.6. chimeric sequences to obtain the final valid data.
(1) The relative interaction index (RII) and incremental species richness (ISR) for the C. nepalensis samples and surrounding herbaceous samples, (Cavieres et al., 2016) were calculated as follows:
Where: are mean values of species richness within the C. nepalensis samples and herbaceous samples, respectively; denotes species found only within the matsutake bush sample; denotes species found in two microhabitats.
(2) Plant diversity indices: richness index (R), Simpson diversity index (D), Shannon-Wiener diversity index (H), and Pielou evenness index (J) (Ma et al., 1997), calculated as follows:
Where:S denotes the number of species occurring within the sample square;Pi is the relative importance value, which is the proportion of the number of the ith species to the total number of all species.
(3) Biomass determination: 5 plants of each species randomly determined in each sample square were dug out with roots, marked and bagged according to different species in different squares, returned to the laboratory, washed, divided into above-ground and below-ground parts and then killed in the oven at 105°C for half an hour, then dried at 65°C to a constant weight and weighed with an electronic balance with an accuracy of 0.01 g to determine total biomass.
Excel 2020 software was used to count and organize the data; IBM SPSS 26.0 software was used to perform statistical analysis of the data. One-way analysis of variance (ANOVA) was used to assess differences in soil physicochemical properties, microbial diversity, and plant diversity and biomass across the same parties. Finally, Origin 2021 software was used for plotting. Structural equation models (SEM) were constructed using the lavaan software package in R (Rosseel, 2012; Hong et al., 2021), and the model parameter estimation method was based on the maximum probability method. SEM was created to estimate the contribution of the main influences and pathways in the presence of C. nepalensis (Branco et al., 2016). All the above statistical tests and illustrations were performed in R 4.1.3 or R 4.2.0 (R Core Team, 2018).
C. nepalensis can change the native habitat and improve the soil properties in C. nepalensis sample (Y) compared with the surrounding herbaceous sample (N). This indicates that the C. nepalensis promotes the stabilization of the soil structure under the canopy and increases the local resources of the soil with a “fertilizer island” effect. As shown in Figure 3, the soil improvement effects were reflected in the following aspects: (1) the soil bulk weight of C. nepalensis samples was significantly lower than that of herbaceous samples; (2) the organic matter of C. nepalensis samples was significantly higher than that of herbaceous samples in all samples except for S3 samples; (3) except for S3 sample, the total nitrogen of the C. nepalensis samples was significantly higher than that of the herbaceous samples; (4) except for S3 and S4 samples, the total phosphorus of the C. nepalensis samples was significantly higher than that of the herbaceous samples.
Figure 3 Effect of C. nepalensis on soil physicochemical properties; S1, S2, S3, S4 are different years of abandonment; Y is C. nepalensis samples; N is a herb samples; Different cases indicate significant difference between Y and N based on 95% confidence interval (p< 0.05).
As can be seen from Figure 4, total soil Pb in the C. nepalensis samples was not significantly different from that in the herbaceous samples. The total soil Zn and Cd in the C. nepalensis samples were significantly higher than that in herbaceous samples in the S1, S2, S4 samples, while the opposite was true in Sample S3. In the C. nepalensis samples, soil total Cd was significantly higher in S2 and S4 than in herbaceous samples, while the opposite was true in S3. In the C. nepalensis samples, soil total Mn was significantly higher in S1 and S2 than in herbaceous samples, while the opposite was true in S3.
Figure 4 Effect of C. nepalensis on soil heavy metals; S1, S2, S3, S4 are different years of abandonment; Y is C. nepalensis samples; N is a herb samples;Different cases indicate significant difference between Y and N based on 95% confidence interval (p< 0.05).
The results of NMDS analysis based on Bray-Curtis similarity coefficients for bacterial, fungal, and actinomycete community data from each site soil sample are shown in Figure 5.
Figure 5 Non-metric multidimensional scale (NMDS) ranking plots based on Bray-Curtis distances for (A) bacterial, (B) fungal and (C) actinomycete community samples for all loci.
As seen in Figure 6, For the bacterial community, Chao1 index was significantly higher in S1 and S3 samples for C. nepalensis samples than for the herbaceous samples, while the opposite was true for S4 samples; Shannon index was significantly higher in S2 and S4 samples for C. nepalensis samples than for the herbaceous samples. For the fungal community, the fungal Chao1 index was significantly higher in the C. nepalensis samples than in the herbaceous samples in the S2, S3, S4 samples, and the fungal Shannon index was significantly higher in the C. nepalensis samples than in the herbaceous samples in the S3 and S4 sample sites. For actinomycetes, the Chao1 index and Shannon index of actinomycetes were significantly higher in the C. nepalensis samples than in the herbaceous samples in the three sample sites, except for the S3 sample site.
Figure 6 Alpha diversity indices of bacteria, fungi and actinomycetes in different loci. Top: Chao1 indices of bacteria, fungi and actinomycetes. Bottom: Shannon indices of bacteria, fungi and actinomycetes. Different lowercase letters indicate significant differences (p< 0.05) based on 95% confidence intervals for C. nepalensis samples and herbaceous samples.
As shown in Figure 7, the composition of bacterial and fungal communities were similar for each site, but the abundance of each phylum differed. In the C. nepalensis samples, it could be found that the abundance of Proteobacteria was higher in each sample site than in the herbaceous sample sites; except for the S3 sample site, the abundance of Bacteroidetes was higher in the herbaceous sample sites; in each sample site, the abundance of Chloroflexi was higher in the herbaceous samples than in the C. nepalensis samples. For the fungal community composition, In the C. nepalensis samples, it could be found that the abundance of Ascomycota was higher than that of herbaceous sample sites in all sample sites except S4; the abundance of Basidiomycota was higher than that of herbaceous samples in all sample sites except S3; and the abundance of Glomeromycota and Chytridiomycota was higher than that of herbaceous samples in all sample sites.
Figure 7 Relative abundance (%) of bacterial and fungal taxa at the phylum level in different equations. (A) Bacterial taxa; (B) fungal taxa.
By comparing with the FAPROTAX database, we found that the bacterial functions related to nitrogen fixation and nitrate reduction were higher in the C. nepalensis samples than in the herbaceous samples in all the sample plots; the bacterial functions related to nitrogen respiration and nitrate respiration were higher in the C. nepalensis samples than in the herbaceous samples except for the S4 sample plots; the bacterial functions related to predation or ectoparasitism were higher in the herbaceous samples than in the C. nepalensis samples except for the S3 sample plots (Figure 8). The bacterial functions related to predation or ectoparasitism were higher in the herbaceous samples than in the C. nepalensis samples except for S3 (Figure 8). By comparing with the Bugbase database, we found that more parthenogenic anaerobic bacteria and stress tolerance were present in the C. nepalensis samples in all the sample plots; greater abundance of potentially pathogenic bacteria and biofilm formation were present in the C. nepalensis samples in all the sample plots except S1; greater abundance of stress tolerance function was present in the C. nepalensis samples in all the sample plots except S2; greater abundance of aerobic bacteria and Gram-negative bacteria were present in the C. nepalensis samples in all the sample plots except S3; and greater abundance of aerobic bacteria was present in the C. nepalensis samples in all the sample plots except S4. In addition to S3, C. nepalensis samples had greater abundance of mobile elements and Gram-negative bacteria; except for S4, C. nepalensis samples had greater abundance of aerobic bacteria. In contrast, higher abundance of Gram-positive bacteria was found in herbaceous samples in all the plots except S3 (Figure 8).
Figure 8 Microbial function prediction in different parties (A) based on bacterial function prediction in different parties of FAPROTAX; (B) based on bacterial function prediction in different parties of Bugbase.
As shown in Table 1, the number of species unique to the C. nepalensis samples were significantly higher than that of the herbaceous sample in all the sample sites, and C. nepalensis had more of its endemic species under its canopy. This indicates that in the abandoned sites of Pb-Zn mines, C. nepalensis has a good nurse effect on plants and can help other plant species to colonize under its canopy.
As shown in Figure 2, the relative interaction index (RII) and incremental species richness (ISR) were calculated for each sample site based on the number of species and unique species in each sample site (Table 2). As shown in Table 2, the relative interaction index (RII) was greater than 0 for each site, indicating that the species richness increased at the patch scale in each sample site. At the community level, the proportional increase in species richness from the C. nepalensis can be expressed by the incremental species richness (ISR) index, which was greater than 20%.
As shown in Figure 9, In C. nepalensis sample, the Shannon diversity index and Simpson index were higher in the S2 and S4 samples than in the herbaceous sample. The Pielou index of the herbaceous sample without C. nepalensis sample was significantly higher than that of the C. nepalensis sample in the S1 sample.
Figure 9 Plant diversity indices for the different equal sides. Different letters indicate significant differences based on 95% confidence intervals for different squares (p< 0.05).
The biomass of each sample was measured and the results are shown in Figure 10. For the same site, the biomass of the C. nepalensis sample was found to be significantly higher than that of the surrounding herbaceous samples in all four sample sites.
Figure 10 Plant biomass in different equal squares. Different letters indicate significant differences (p< 0.05) based on 95% confidence intervals for the different equations.
In this study, structural equation modeling was used to explore the key factors affecting plant biomass within the sample plots (Figure 11). The herbaceous sample square (SN) was used as a control. In the SY sample, the effects can be divided into four main pathways: (i) TN→Bio (λ = 0.28), (ii) ACT-S→Bio (λ = 0.40) and (iii) SBD→Bio (λ = −0.50), (iiii) SBD→ACT-S (λ = 0.35)→Bio (λ = 0.40). In the SN sample, there is a major pathway: (i) ACT-S→Bio (λ = 0.62), while there is a nitrogen recharge pathway: SOM→TN (λ = 0.56) in the SN sample due to nitrogen deficiency. In the C. nepalensis complex, TN, ACT-S and SBD are direct factors affecting plant biomass within the sample, while the effect of SBD on ACT-S also affects plant biomass within the sample as an indirect factor. When in herbaceous samples, ACT-S can directly affect plant biomass within the sample, while herbaceous samples will increase N content through SOM due to N deficiency, but have no significant effect on biomass. Overall, N may be the main factor limiting plant colonization and survival in degraded habitats in mining areas.
Figure 11 Structural equation modeling (SEM) showing the effects of C. nepalensis drivers on sample biomass. Total soil nitrogen content (TN), biomass (Bio), soil organic matter (SOM), soil bulk density (SBD), and actinomycete Shannon diversity index (ACT-S). For each endogenous variable, the amount of variance explained by the model (R2) is labeled above it, and the metric value of the overall model fit is labeled above the box. Standardized path coefficients are adjacent to the arrows, (*: 0.01< p< 0.05; **: 0.001< p< 0.01; ***: p< 0.001). Red arrows indicate positive correlations, black arrows indicate negative correlations, the stronger the correlation, the thicker the arrow, and dashed lines indicate non-significant paths. (A) Indicates C. nepalensis-like (SY); (B) indicates herb-like (SN).
The promotion of sub-canopy species by conservation plants is primarily through intermediates (soils) (Hunter and Price, 1992). Soil nutrient sharing may provide long-term benefits to nurse plants and may be a neglected mechanism for maintaining plant community coexistence and increasing phylogenetic diversity (Anthelme et al., 2017; Montesinos-Navarro et al., 2017). Wright et al. (2017) showed that in degraded systems, the impact of nurse plants on soil characteristics was mainly in the form of improved microhabitat (Tapella et al., 2021) through microclimate changes induced within the canopy by nurse plants and in the form of nutrient enrichment. In the C. nepalensis sample soil properties outside the canopy were also improved compared to the herbaceous sample, indicating that the C. nepalensis sample contributed to the stabilization of the sub-canopy soil structure and the increase of local soil resources and the “fertilizer island” effect. Firstly, the soil tolerance of C. nepalensis sample was significantly lower than that of herbaceous sample sites, which is consistent with Tapella et al. (2021). As a symbiotic nitrogen-fixing actinomycete, C. nepalensis fixes nitrogen in the air and increases the soil total nitrogen content of C. nepalensis sample, thus improving the soil nutrition of the patches, which is consistent with the study of Wang et al. (2017), and C. nepalensis sample enriches nitrogen near C. nepalensis due to its strong nitrogen fixation ability, thus promoting plant growth and increasing community biomass. Meanwhile, it was found that the organic matter in the C. nepalensis sample in large diverse sites was significantly higher than that in herbaceous sample sites. This may be due to the greater plant biomass under the C. nepalensis sample, the accumulation of apoplastic material from the plants under the cluster and the C. nepalensis’s own apoplastic material, which made the organic matter content significantly higher than that of the bare ground, thus contributing to the increase of soil nutrients.
The presence of C. nepalensis can increase soil nutrients, Meanwhile significantly reduce heavy metal spillage. Soil organic matter easily forms complexes with metals and acts as a sorbent (Pezzarossa and Petruzzelli, 2003), and high molecular weight humic acids form very stable complexes with metals, removing toxic and hazardous metals from the environment and reducing their bioavailability (Wang et al., 2010). Therefore, the increase of soil organic matter under the C. nepalensis sample further avoids the escape of toxic and harmful metals from the soil and reduces their toxic effects on organisms. It has also been shown that C. nepalensis can form complexes with heavy metals due to its own functional groups such as carboxyl or hydroxyl groups, and it can be used as the best detergent for remediation of heavy metal soils when the pH is acidic, while in the present study, the mine waste site is mainly alkaline, and C. nepalensis mainly shows complexation for heavy metals, and C. nepalensis can form complexes with heavy metals to reduce the toxicity of heavy metals to plants under the canopy (Cao et al., 2017).
In microbial ecology, it is generally believed that co-nutritional microorganisms are closely associated with r-strategies, while oligotrophic microorganisms are associated with k-strategies (Zhou et al., 2017; Loayza et al., 2018). Microbial trophic partitioning may help to understand the results of this study (Ken et al., 2009; Mihoc et al., 2016). Both Proteobacteria and Bacteroidetes belong to the r-strategic bacteria (Zeng et al., 2018). Previous studies have suggested that an increase in easily decomposable organic matter such as glucose and root secretions in soil stimulates a rapid increase in the abundance of r-strategic bacteria (Bashan et al., 2017), which in turn initiates an excitation effect to accelerate soil organic matter decomposition (Bernard et al., 2007). In the study, the abundance of Proteobacteria and Bacteroidetes was found to be higher in each sample site than in the herbaceous sample sites, which justified the higher organic matter in the C. nepalensis sample in this study, and the increase in organic matter led to the increase in the abundance of r-strategic bacteria and the faster decomposition of organic matter in the C. nepalensis sample sites. Meanwhile, the presence of C. nepalensis increased the abundance and diversity of soil actinomycetes, probably mainly due to high inter-root organic matter content, faster decomposition, loose soil and good moisture conditions, which allowed more actinomycetes to colonize. Ascomycota and Basidiomycota have the ability to decompose cellulose and hemicellulose. Among them, Ascomycota belongs to r-strategic fungi, and the abundance of Ascomycota in C. nepalensis sample was greater than that of herbaceous samples in the abandoned sites of metal mining areas, which may indicate that C. nepalensis sample are significantly more prone to decompose organic matter than herbaceous samples, and C. nepalensis sample decompose organic matter more rapidly. In contrast, Basidiomycota plays a major role in the decomposition of refractory organic matter, and an increase in the abundance of refractory organic matter in the composition of apoplankton will lead to an increase in Basidiomycota abundance (Yan et al., 2020). In the study, the abundance of Basidiomycota was greater in the C. nepalensis sample than in the herbaceous sample sites because of the high plant species richness and biomass of the C. nepalensis sample, which resulted in a complex composition of the C. nepalensis sample with a higher content of refractory organic matter than in the herbaceous sample sites. It was also found that the abundance of Chloroflexi in the bacterial community and Glomeromycota and Chytridiomycota in the fungal community was higher in the herbaceous sample sites than in the C. nepalensis sample, reflecting their ability to tolerate extreme conditions and support lower substrate utilization (Eilers et al., 2010).
Meanwhile, in this study, it was found that N was significantly higher in the C. nepalensis sample than in the herbaceous sample in a large diversity of sites, and the prediction of bacterial functions based on FAPROTAX revealed that the abundance of bacterial functions related to nitrogen fixation, nitrate reduction, nitrogen respiration, and nitrate respiration was higher in the C. nepalensis sample in a large diversity of sites, so C. nepalensis may have recruited more functional genes related to nitrogen cycling to improve the inter-root soil nitrogen cycle, which needs to be Further studies are needed to prove this. Bugbase’s prediction of bacterial functions revealed that more biofilm-forming, parthenogenic anaerobic, stress-tolerant, aerobic, mobile progenitor-containing and Gram-negative bacteria were present in the C. nepalensis sample in a large diversity of sites. The inclusion of mobile elements could play an important role in horizontal transfer, thereby increasing microbial adaptation to the environment, while Gram-negative bacteria were shown to be more metal-tolerant, so C. nepalensis may recruit more well-adapted and tolerant microorganisms.
Nurse plants (e.g., leguminous shrubs and trees, matted plants, etc.) can induce microenvironmental changes within the canopy that provide a benign environment more conducive to seed germination and/or seedling recruitment than the surrounding environment, promoting the colonization, growth, and development of other plant species under their canopy, thereby increasing species richness, diversity, and species coexistence (Ellison, 2019). The present study showed that C. nepalensis could improve sub-canopy soil nutrition, reduce the toxicity of heavy metals to sub-canopy plants and recruit microorganisms that favor plant growth, resulting in more species and biomass under the canopy. This is in agreement with the findings of Joshi et al. (2001) in eroded sites and Mourya et al. (2019) in central Himalaya on C. nepalensis. In conclusion, C. nepalensis has a good nurse role in mining waste sites and C. nepalensis can help the establishment of other plants, thus restoring species populations and ecological interactions, which in turn makes it possible to restore the relevant ecosystem functions.
In summary, C. nepalensis has a good nurse role as a naturally colonized plant in Pb-Zn mine waste sites. It can improve the microhabitat soil properties and regulate the soil microbial community structure to conserve the under-canopy plants in the abandoned areas of Pb-Zn mines, thus enabling plant community succession in degraded environments. A comprehensive analysis of the environmental improvement effectiveness and mechanism of mulberry in Pb-Zn mine abandoned sites aims to provide a theoretical basis for revegetation and restoration of Pb-Zn mine abandoned sites.
The original contributions presented in the study are included in the article/supplementary material. Further inquiries can be directed to the corresponding author.
JY: conceptualization, methodology, software, writing –original draft, visualization, and investigation. HT: investigation and software. C-QD: methodology, validation, revision, review and editing, supervision, and funding acquisition. X-QY, S-CW, LH, and L-YL: investigation and methodology. All authors contributed to the article and approved the submitted version.
The author(s) declare financial support was received for the research, authorship, and/or publication of this article. This research was supported by the National Natural Science Foundation of China (U2002208 and 32260315) and China Yunnan Provincial R&D Programs (202101AS070033, 202201AS070016, and 202201BF070001-002).
The authors declare that the research was conducted in the absence of any commercial or financial relationships that could be construed as a potential conflict of interest.
All claims expressed in this article are solely those of the authors and do not necessarily represent those of their affiliated organizations, or those of the publisher, the editors and the reviewers. Any product that may be evaluated in this article, or claim that may be made by its manufacturer, is not guaranteed or endorsed by the publisher.
Adiansyah J. S., Rosano M., Vink S., Keir G. (2016). A framework for a sustainable approach to mine tailings management: disposal strategies. J. Cleaner Production 108, 1050–1062. doi: 10.1016/j.jclepro.2015.07.139
Anthelme F., Meneses R. I., Valero N. N. H., Pozo P., Dangles O. (2017). Fine nurse variations explain discrepancies in the stress-interaction relationship in alpine regions. Oikos 126 (8), 1173–1183. doi: 10.1111/oik.04248
Bashan Y., Salazar B., Puente M. E., Bacilio M., Linderman R. (2017). Enhanced establishment and growth of giant cardon cactus in an eroded field in the Sonoran Desert using native legume trees as nurse plants aided by plant growth-promoting microorganisms and compost. Biol. Fertility Soils 45 (6), 585–594.
Bernard L., Mougel C., Maron P. A., Nowak V., Leveque J., Henault C., et al. (2007). Dynamics and identification of soil microbial populations actively assimilating carbon from C-13-labelled wheat residue as estimated by DNA- and RNA-SIP techniques. Environ. Microbiol. 9 (3), 752–764. doi: 10.1111/j.1462-2920.2006.01197.x
Branco P., Ribeiro R. P., Torgo L. (2016). UBL: an R package for utility-based learning. arXiv. doi: 10.48550/arXiv.1604.08079. [Preprint].
Cao Y. R., Zhang S. R., Wang G. Y., Huang Q. L, Xu X. X. (2017). Removal of Pb, Zn, and Cd from contaminated soil by new washing agent from plant material. Environ. Sci. pollut. Res. 24 (9), 8525–8533. doi: 10.1007/s11356-017-8542-3
Cavieres L. A., Hernandez-Fuentes C., Sierra-Almeida A., Kikvidze. (2016). Facilitation among plants as an insurance policy for diversity in Alpine communities. Funct. Ecol. 30 (1), 52–59. doi: 10.1111/1365-2435.12545
Chen X. W., Wong J. T. F., Wang J. J., Ming H. (2020). Vetiver grass-microbe interactions for soil remediation. Crit. Rev. Environ. Sci. Technol. 51 (9), 897–938.
Chen L., Zhou M. X., Wang J. Z., Zhang Z. Q., Duan C. J., Wang X. X., et al. (2022). A global meta-analysis of heavy metal(loid)s pollution in soils near copper mines: Evaluation of pollution level and probabilistic health risks. Sci. Total Environ. 835, 155441. doi: 10.1016/j.scitotenv.2022.155441
Cui J. L., Zhao Y. P., Chan T. S., Zhang L. L., Daniel C. W., Li X. D. (2020). Spatial distribution and molecular speciation of copper in indigenous plants from contaminated mine sites:Implication for phytostabilization. J. Hazardous Materials 381, 121208.
Eilers K. G., Lauber C. L., Knight R., Fierer N. (2010). Shifts in bacterial community structure associated with inputs of low molecular weight carbon compounds to soil. Soil Biol. Biochem. 42 (6), 896–903. doi: 10.1016/j.soilbio.2010.02.003
Ellison A. M. (2019). Foundation species, non-trophic interactions, and the value of being common. Iscience 13, 254–268. doi: 10.1016/j.isci.2019.02.020
Gastauer M., Silva J. R., Caldeira C. F., Ramos S. J., Martins S. F., Pedro W., et al. (2018). Mine land rehabilitation: Modern ecological approaches for more sustainable mining. J. Cleaner Production 172, 1409–1422. doi: 10.1016/j.jclepro.2017.10.223
Hong Y. S., Chen Y. Y., Shen R. L., Chen S., Xu G., Cheng H., et al. (2021). Diagnosis of cadmium contamination in urban and suburban soils using visible-to-near-infrared spectroscopy. Environ. pollut. 291, 118128. doi: 10.1016/j.envpol.2021.118128
Hunter M. D., Price P. W. (1992). Playing chutes and ladders: heterogeneity and the relative roles of bottom-up and top-down forces in natural communities. Ecology 73 (3), 724–732. doi: 10.2307/1940152
Joshi B., Singh S. P., Rawat Y. S., Goel D. (2001). Facilitative effect of Coriaria Nepalensis on species diversity and growth of herbs on severely eroded hill slopes. Curr. Sci. 80 (5), 678–682.
Ken E. G., Ernst W., McGrath S. P. (2009). Heavy metals and soil microbes. Soil Biology and Biochemistry 41 (10), 2031–2037. doi: 10.1016/j.soilbio.2009.04.026
Khalid S., Shahid M., Niazi N. K., Murtaza B., Bibi I., Dumat C. (2017). A comparison of technologies for remediation of heavy metal contaminated soils. J. Geochemical Explor. 182, 247–268. doi: 10.1016/j.gexplo.2016.11.021
Lei D. M., Duan C. Q. (2008). Restoration potential of pioneer plants growing on lead-zinc mine tailings in Lanping, southwest China. J. Environ. Sci. 20 (10), 1202–1209. doi: 10.1016/S1001-0742(08)62210-X
Li M. S. (2006). Ecological restoration of mineland with particular reference to the metalliferous mine wasteland in China: A review of research and practice. Sci. Total Environ. 357, (1–3)38-53.
Li J., Huang X. B., Li S. F., Tang R., Su J. R. (2023). Microbial network complexity and diversity together drive the soil ecosystem multifunctionality of forests during different woodland use intensity in dry and wet season. For. Ecol. Manage. 542, 121086. doi: 10.1016/j.foreco.2023.121086
Li Z. Y., Ma Z. W., van der Kuijp T. J., Yuan Z. W., Huang L. (2014). A review of soil heavy metal pollution from mines in China: Pollution and health risk assessment. Sci. Total Environ. 468, 843–853. doi: 10.1016/j.scitotenv.2013.08.090
Li T., Wu M. H., Duan C. Q., Li S. Y., Liu C. E. (2022). The effect of different restoration approaches on vegetation development in metal mines. Sci. Total Environ. 806 (3), 150626.
Loayza A. P., Herrera-Madariaga M. A., Carvajal D. E., Garcia-Guzman P., Squeo F., Pugnaire F. (2018). Conspecific plants are better ‘nurses’ than rocks: consistent results revealing intraspecific facilitation as a process that promotes establishment in a hyper-arid environment. Aob Plants 9, plx056.
Ma K. P., Huang J. H., Yu S. L. (1997). Plant community diversity in dongling mountain,Beijing,China II species abundance relations of several types of forest communities. Acta Ecologica Sin. 17, 573–583.
Mahar A., Wang P., Ali A., Awasthi M. K., Lahori A. H., Wang Q., et al. (2016). Challenges and opportunities in the phytoremediation of heavy metals contaminated soils: A review. Ecotoxicology Environ. Saf. 126, 111–121. doi: 10.1016/j.ecoenv.2015.12.023
Marc P., Jacques G. (2006). Handbook of Soil Analysis [M] (Mineralogical, Organic and Inorganic Methods).
Mi J. X., Liu R., Zhang S. L., Hou H. P., Yang Y. J., Chen F. Y., et al. (2019). Vegetation patterns on a landslide after five years of natural restoration in the Loess Plateau mining area in China. Ecol. Eng. 136, 46–54. doi: 10.1016/j.ecoleng.2019.05.022
Mihoc M. A. K., Gimenez-Benavides L., Pescador D. S., et al. (2016). Soil under nurse plants is always better than outside: a survey on soil amelioration by a complete guild of nurse plants across a long environmental gradient. Plant Soil 408 (1-2), 31–41. doi: 10.1007/s11104-016-2908-z
Montesinos-Navarro A., Verdu M., Querejeta J. I. (2017). Nurse plants transfer more nitrogen to distantly related species. Ecology. 98 (5), 1300–1310.
Mourya N. R., Bargali K., Bargali S. S. (2019). Impacts of Coriaria Nepalensis colonization on vegetation structure and regeneration dynamics in a mixed conifer forest of Indian Central Himalaya. J. Forestry Res. 30 (1), 305–317. doi: 10.1007/s11676-018-0613-x
Moyan W., Zi Q. M., Daniel B. G., et al. (2017). Heavy metals in agricultural soil in China: A systematic review and meta-analysis. Eco-Environment Health 1 (4), 219–228.
Pan H. J., Zhou G. H., Cheng Z. Z., et al. (2014). Advances in geochemical survey of mine tailings project in China. J. Geochemical Explor. 139, 193–200. doi: 10.1016/j.gexplo.2013.07.012
Pezzarossa B., Petruzzelli G. (2003). Selenium contamination in soil: sorption and desorption. Heavy Metals Release Soils. 978-1-56670-531-8.
Pokhrel L. R., Dubey B. (2013). Global scenarios of metal mining, environmental repercussions, public policies, and sustainability: A review. Crit. Rev. Environ. Sci. Technol. 43 (21), 2352–2388. doi: 10.1080/10643389.2012.672086
R Core Team (2018). R: A Language and Environment for Statistical Computing. (Vienna: R Foundation for Statistical Computing).
Rieuwerts J. S., Mighanetara K., Braungardt C. B., et al. (2014). Geochemistry and mineralogy of arsenic in mine wastes and stream sediments in a historic metal mining area in the UK. Sci. Total Environ. 472, 226–234. doi: 10.1016/j.scitotenv.2013.11.029
Rodriguez-Seijo A., Lourenco J., Arenas-Lago D., et al. (2020). Chemical availability versus bioavailability of potentially toxic elements in mining and quarry soils. Chemosphere 251, 126421. doi: 10.1016/j.chemosphere.2020.126421
Rosseel Y. (2012). lavaan: an R package for structural equation modeling. J. Stat. Software 48, 1–36. doi: 10.18637/jss.v048.i02
Saxena G., Purchase D., Mulla S. I., et al. (2020). Phytoremediation of heavy metal-contaminated sites: eco-environmental concerns, field studies, sustainability issues, and future prospects. Rev. Environ. Contamination Toxicol. 249, 71–131.
Sheoran V., Sheoran A. S., Poonia P. (2016). Factors affecting phytoextraction: A review. Pedosphere 26 (2), 148–166. doi: 10.1016/S1002-0160(15)60032-7
Stylianou M., Gavriel I., Vogiatzakis I. N., et al. (2020). Native plants for the remediation of abandoned sulphide mines in Cyprus: A preliminary assessment. J. Environ. Manage. 274, 110531. doi: 10.1016/j.jenvman.2020.110531
Tapella M. P., Marcora P. I., Tecco P. A. (2021). Reciprocal interactions between a non-native shrub and the dominant native trees of a high mountain woodland: who benefits? Biol. Invasions 23 (1), 53–67. doi: 10.1007/s10530-020-02355-w
van der Ent A., Baker A. J. M., Reeves R. D., et al. (2013). Hyperaccumulators of metal and metalloid trace elements: Facts and fiction. Plant Soil 362 (1-2), 319–334. doi: 10.1007/s11104-012-1287-3
Wang D., He H. L., Gao Q., et al. (2017). Effects of short-term N addition on plant biomass allocation and C and N pools of the Sibiraea angustata scrub ecosystem. Eur. J. Soil Sci. 68 (2), 212–220. doi: 10.1111/ejss.12414
Wang Q., Li Z., Cheng S. P., et al. (2010). Effects of humic acids on phytoextraction of Cu and Cd from sediment by Elodea nuttallii. Chemosphere 78 (5), 604–608. doi: 10.1016/j.chemosphere.2009.11.011
Wei Z. H., Le Q. V., Peng W. X., et al. (2021). A review on phytoremediation of contaminants in air, water and soil. J. Hazardous Materials 403, 123658. doi: 10.1016/j.jhazmat.2020.123658
Wright A. J., Wardle D. A., Callaway R., et al. (2017). The overlooked role of facilitation in biodiversity experiments. Trends Ecol. Evol. 32 (5), 383–390. doi: 10.1016/j.tree.2017.02.011
Yan B. S., Sun L. P., Li J. J., et al. (2020). Change in composition and potential functional genes of soil bacterial and fungal communities with secondary succession in Quercus liaotwigensis forests of the Loess Plateau, western China. Geoderma 364, 114199. doi: 10.1016/j.geoderma.2020.114199
Zeng Q. C., An S. S., Liu Y. (2018). Soil bacterial community response to vegetation succession after fencing in the grassland of China. Sci. Total Environ. 609, 2–10. doi: 10.1016/j.scitotenv.2017.07.102
Zhang J., Elser J. J. (2017). Carbon : nitrogen:Phosphorus stoichiometry in fungi: A meta-analysis. Front. Microbiol. 8, 01281. doi: 10.3389/fmicb.2017.01281
Zhang N. Y., Guo R., Song P. A., et al. (2013). Effects of warming and nitrogen deposition on the coupling mechanism between soil nitrogen and phosphorus in Songnen Meadow Steppe, northeastern China. Soil Biol. Biochem. 65, 96–104. doi: 10.1016/j.soilbio.2013.05.015
Zhou Z. H., Wang C. K., Jiang L. F., et al. (2017). Trends in soil microbial communities during secondary succession. Soil Biol. Biochem. 115, 92–99. doi: 10.1016/j.soilbio.2017.08.014
Zhou J. X., Xiang Z. Z., Zhou M. F., et al. (2018). The giant Upper Yangtze Pb-Zn province in SW China: reviews, new advances and a new genetic model. J. Asian Earth Sci. 154, 280–315. doi: 10.1016/j.jseaes.2017.12.032
Keywords: nurse plant, heavy metal, Pb-Zn mine, Coriaria nepalensis, ecological restoration
Citation: Yang J, Tang H, Duan C-q, Wang S-c, Yuan X-q, Huang L and Li L-y (2023) Nurse role and mechanism of Coriaria nepalensis in abandoned land of Pb-Zn mining area. Front. Ecol. Evol. 11:1246822. doi: 10.3389/fevo.2023.1246822
Received: 24 June 2023; Accepted: 10 November 2023;
Published: 24 November 2023.
Edited by:
Kang Di, China West Normal University, ChinaCopyright © 2023 Yang, Tang, Duan, Wang, Yuan, Huang and Li. This is an open-access article distributed under the terms of the Creative Commons Attribution License (CC BY). The use, distribution or reproduction in other forums is permitted, provided the original author(s) and the copyright owner(s) are credited and that the original publication in this journal is cited, in accordance with accepted academic practice. No use, distribution or reproduction is permitted which does not comply with these terms.
*Correspondence: Chang-qun Duan, Y2hxZHVhbkB5bnUuZWR1LmNu
Disclaimer: All claims expressed in this article are solely those of the authors and do not necessarily represent those of their affiliated organizations, or those of the publisher, the editors and the reviewers. Any product that may be evaluated in this article or claim that may be made by its manufacturer is not guaranteed or endorsed by the publisher.
Research integrity at Frontiers
Learn more about the work of our research integrity team to safeguard the quality of each article we publish.